- 1School of Agricultural Biotechnology, Punjab Agricultural University, Ludhiana, India
- 2Pulses Section, Department of Plant Breeding and Genetics, Punjab Agricultural University, Ludhiana, India
- 3Punjab Agricultural University, Regional Research Station, Faridkot, India
- 4Department of Biotechnology-Assam Agricultural University Centre, Assam Agricultural University, Jorhat, India
The gram pod borer Helicoverpa armigera is a major constraint to chickpea (Cicer arietinum L.) production worldwide, reducing crop yield by up to 90%. The constraint is difficult to overcome as chickpea germplasm including wild species either lacks pod borer resistance or if possessing resistance is cross-incompatible. This study describes conversion of elite but pod borer-susceptible commercial chickpea cultivars into resistant cultivars through introgression of cry1Ac using marker-assisted backcross breeding. The chickpea cultivars (PBG7 and L552) were crossed with pod borer-resistant transgenic lines (BS 100B and BS 100E) carrying cry1Ac that led to the development of BC1F1, BC1F2, BC1F3, BC2F1, BC2F2, and BC2F3 populations from three cross combinations. The foreground selection revealed that 35.38% BC1F1 and 8.4% BC1F2 plants obtained from Cross A (PBG7 × BS 100B), 50% BC1F1 and 76.5% BC1F2 plants from Cross B (L552 × BS 100E), and 12.05% BC2F2 and 82.81% (average) BC2F3 plants derived from Cross C (PBG7 × BS 100E) carried the cry1Ac gene. The bioassay of backcross populations for toxicity to H. armigera displayed up to 100% larval mortality. BC1F1 and BC1F2 populations derived from Cross B and BC2F3 population from Cross C segregated in the Mendelian ratio for cry1Ac confirmed inheritance of a single copy of transgene, whereas BC1F1 and BC1F2 populations obtained from Cross A and BC2F2 population from Cross C exhibited distorted segregation ratios. BC1F1 plants of Cross A and Cross B accumulated Cry1Ac protein ranging from 11.03 to 11.71 µgg−1 in leaf tissue. Cry1Ac-positive BC2F2 plants from Cross C demonstrated high recurrent parent genome recovery (91.3%) through background selection using SSR markers and phenome recovery of 90.94%, amongst these 30% plants, were homozygous for transgene. The performance of BC2F3 progenies derived from homozygous plants was similar to that of the recurrent parent for main agronomic traits, such as number of pods and seed yield per plant. These progenies are a valuable source for H. armigera resistance in chickpea breeding programs.
Introduction
Chickpea (Cicer arietinum L., 2n = 16), belonging to the family Leguminoseae, is an economical source of protein (18–22%), minerals, fiber, β-carotene, and unsaturated fatty acids (Jukanti et al., 2012). The crop is grown in nearly 57 countries with India, Australia, Myanmar, Ethiopia, Turkey, and Russia as the major producers (Merga and Haji 2019). The crop production is severely affected by various biotic and abiotic stresses leading up to 90% yield losses (Kumar et al., 2018). Among biotic stresses, gram pod borer Helicoverpa armigera (Hübner) [Lepidoptera: Noctuidae] causes significant crop damage annually (90%) estimated at US $330 million worldwide (Rao et al., 2013; Patil et al., 2017). H. armigera is difficult to control as it has migratory behavior, numerous generations per year, adaptability to different environmental conditions, high fecundity, and insecticidal resistance (Fitt 1989). Furthermore, the biopesticides used to control the insect have high production costs coupled with poor product quality control systems (Cherry et al., 2000; Jenkins and Grzywacz 2000). The development of pod borer-resistant chickpea cultivars through conventional breeding is hampered due to the narrow crop genetic base and crossability barriers between cultivated chickpea and wild Cicer species (Mallikarjuna et al., 2007).
The pod borer larvae have been effectively controlled through specific insecticidal crystal proteins of Bacillus thuringiensis, and Cry1Ac is the most effective toxin against H. armigera (Chakrabarti et al., 1998). Cry1Ac protein acts by targeting the insect midgut in which the prevalence of high pH solubilizes the protein; the activated protein forms a pore complex in the insect epithelial membrane causing lysis and eventually larval death (Bravo et al., 2008). The pod borer attack has been countered efficiently by transgenic chickpea plants carrying cry1Ac, cry1Ab, cry2Aa, and cry1Aa3 (Kar et al., 1997; Sanyal et al., 2005; Acharjee et al., 2010; Mehrotra et al., 2011; Khatodia et al., 2014). The introgression of cry genes from transgenic plants into elite cultivars/lines through marker-assisted backcross breeding leads to precise trait transfer, for e.g., enhanced resistance against striped stem borer in rice by introgression of cry1Ab (Wang et al., 2012), improved resistance against corn borer with cry1A.105 and cry2ab2 in maize inbred lines (Venkatesh et al., 2015), increased tolerance to fruit/shoot borer in eggplant following cry1Ac transfer (Ripalda et al., 2012), and improved insect resistance in cotton via cryIA introgression (Guo et al., 2005), etc. Marker-assisted backcross breeding, an effective molecular breeding technique, enables the transfer of desirable genes from an agronomically inferior donor into an elite recipient in a few generations, without linkage drag and in a smaller population size (Hospital and Charcosset 1997).
The introgression of cry genes from transgenic chickpea lines to commercial chickpea cultivars for imparting resistance against Helicoverpa following marker-assisted backcross breeding is not reported so far. In the present study, an attempt was made to convert two elite but pod borer-susceptible chickpea cultivars, namely, PBG7 and L552, into resistant cultivars by introgressing cry1Ac from pod borer-resistant transgenic lines, namely, BS 100B and BS 100E through marker-assisted backcross breeding. PBG7 is a high-yielding cultivar of desi chickpea, whereas L552 is a bold-seeded high-yielding cultivar of kabuli chickpea; both cultivars are recommended for commercial cultivation in the North Indian state, Punjab, and possess good cooking quality (Sandhu et al., 2012; Singh et al., 2015). The backcross populations were analyzed for the presence of transgene, evaluated for Cry1Ac concentration, and bioassayed for toxicity to H. armigera. The highlighting feature of this study was the introgression of cry1Ac in BC1F1 populations and its subsequent transmission to BC1F2, BC1F3, BC2F2, and BC2F3 that displayed up to 100% H. armigera larval mortality, and agronomic performance of selected BC2F2 and BC2F3 plants was similar to that of the recurrent parent.
Materials and Methods
Plant Material
T5 seeds (15 in number) for each of two transgenic chickpea lines, namely, BS 100B and BS 100E expressing cry1Ac gene under the control of the Arabidopsis Rubisco small subunit gene promoter and tobacco SSU terminator (Supplementary Figure S1), were procured from the Department of Biotechnology-Assam Agricultural University Centre, Assam Agricultural University, Jorhat, Assam, India, during 2013. The transgenic lines carrying cry1Ac at a single locus were used as donor (male) parents in chickpea backcrossing program; the lines are reported to accumulate a high level of Cry1Ac protein (˃ 50 μg g−1 leaf tissue) that causes 80–100% neonatal H. armigera larval mortality (Hazarika et al., 2019). The high-yielding commercial cultivars PBG7 (desi) and L552 (kabuli) were used as recipient (female) parents. F1 plants of PBG7 × BS 100B (designated as Cross A), L552 × BS 100E (Cross B), and PBG7 × BS 100E (Cross C) were backcrossed with their respective recipient parents to obtain BC1F1 seeds that were sown to generate BC1F1 populations. F1 plants, BC1F1, BC1F2, BC1F3, BC2F1, BC2F2, and BC2F3 populations were raised under contained conditions (Supplementary Figure S2; Supplementary Table S1) in a net house (30-mesh screen) at Experimental Farms, Department of Plant Breeding & Genetics, Punjab Agricultural University (PAU), Ludhiana. The populations were grown in plots comprising 25 rows with 2 m length and row-to-row distance of 40 cm following normal agronomic practices during the month of October. The schematic overview of marker assisted-backcross breeding of commercial chickpea cultivars with transgenic lines is shown in Figure 1.
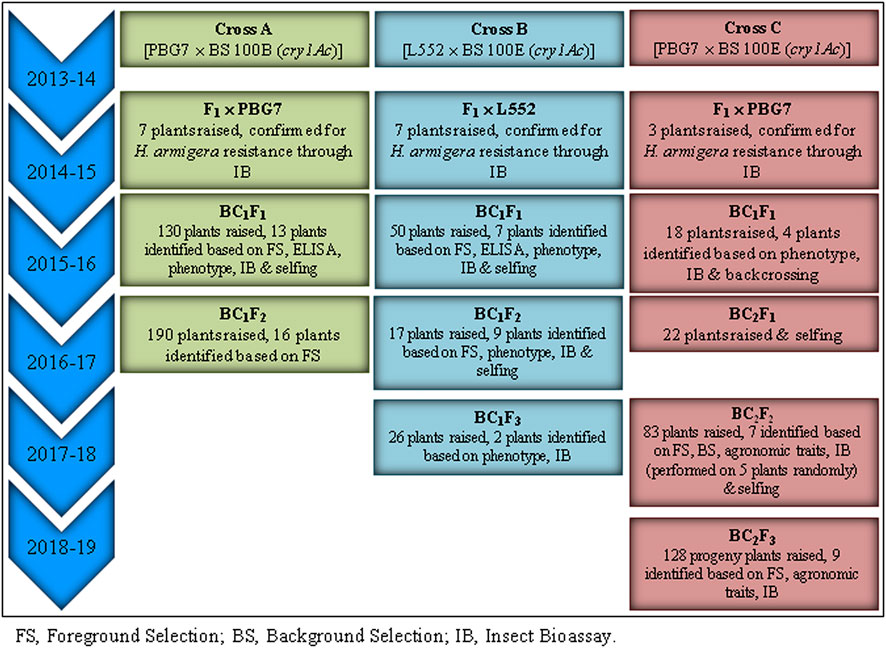
FIGURE 1. Schematic overview of marker-assisted backcross breeding of commercial chickpea cultivars × transgenic lines.
DNA Extraction
Genomic DNA was extracted from tender twigs of 20-day-old BC1F1, BC1F2, BC2F2, and BC2F3 populations, transgenic donor parents BS 100B and BS 100E, and non-transgenic recipient parents PBG7 and L552 according to the miniprep method. For quantification, the extracted DNA was electrophoresed on 0.8% (w/v) agarose gel using PowerPacHC (Bio-Rad, United States) at 50 V for 2 h; ethidium bromide-stained gel was visualized under UV light and photographed on a 110 V AlphaImager HP imaging system (ProteinSimple, United Kingdom).
Foreground Selection for cry1Ac-Positive Plants
The foreground selection of backcross populations was carried out through PCR using cry1Ac-specific (Accession Number M11068, Hazarika et al., 2019), internal forward 5-TATCTTTGGTCCATCTCAATGGG-3 and reverse 5-GTGTCCAGACCAGTAATACTC-3 primers to amplify 757 bp transgene. PCR mixture (20 µl) contained 50 ng genomic DNA (2 µl), 10 µM of each primer (0.6 µl), 1 mM dNTPs (4 µl), 25 mM MgCl2 (1.5 µl), 5 × Green GoTaq Flexi buffer (4 µl), 5 units GoTaq DNA polymerase (1 µl) [Promega, United States] and nuclease-free water (6.3 µl). The reaction mixtures were placed in a GeneAmp PCR System 9700 (Thermo Fisher Scientific, United States) programmed for an initial denaturation at 94 C for 4 min, followed by 35 cycles of denaturation at 94 C for 50 s, annealing at 58 C for 1 min, extension at 72 C for 1 min, and concluded by a final extension at 72 C for 7 min and held at 4 C prior to storage. The amplicons were resolved on 1.5% (w/v) agarose gel, visualized, and photographed. The statistical significance for cry1Ac segregation data was determined by Chi-square analysis using the formula: χ2 = (O-E)2/E, where O is the observed value and E is the expected value.
Enzyme-Linked Immunosorbent Assay
Cry1Ac expression in BC1F1 plants and transgenic donor and non-transgenic recipient parents was quantified through ELISA using Cry1Ac QuantiPlate kit (EnviroLogix, United States) according to the manufacturer’s instructions. A total of two leaflets (10 mg) of each plant were homogenized in an Eppendorf grinding tube for 20–30 s by adding 500 µl of 1 × extraction buffer. Each leaf tissue sample (50 µl) was diluted in a 1:11 ratio by adding 550 µl of 1 × extraction buffer; thereafter, 100 µl each of diluted sample, negative control, and positive calibrator was dispensed in the ELISA plate, followed by parafilm masking and incubation at an ambient temperature for 15 min. The assay was performed in triplicate. Cry1Ac-enzyme conjugate (100 µl) was added to each well, and the plate was again covered with parafilm and incubated for 1 h. After incubation, the parafilm mask was removed and well contents were agitated vigorously to decant the wells. The vacant wells were flooded with washing buffer and agitated to decant; the washing step was performed thrice. Then substrate (100 µl) was added to each well and mixed thoroughly, followed by plate covering with parafilm and incubation for 20 min. The reaction was terminated by adding 100 µl of stop solution to each well. The ELISA plate was read in a 96-well ELISA plate reader Infinite 200 Pro (Tecan, Switzerland) at 450 and 600 nm. The optical density (OD) values of samples and positive calibrators were analyzed using a Microsoft Excel sheet to generate a linear scale graph of the mean OD of each calibrator against its Cry1Ac concentration (Supplementary Table S2). The amount of Cry1Ac protein in each leaf tissue sample (µg g−1) was determined using the formula {(OD of sample - mean OD of negative control) - 0.425/0.127} × dilution factor 1 (38.46) × dilution factor 2 (11)/1,000 (Supplementary Table S2). The data were analyzed for mean ± standard deviation using Microsoft Excel 2007 software at default settings.
Background Selection for Recurrent Parent Genome Recovery
The background selection of cry1Ac-positive BC2F2 plants was carried out using Simple Sequence Repeat (SSR) markers. As a preliminary step, polymorphism analysis was undertaken on parents PBG7 and BS 100E using 210 markers belonging to the following series: CGMM, CaM, GA, GAA, TA, TAA, TS, TR, NCPGR, H, and CaSTMS 11 (Supplementary Table S3). The amplified products were resolved on 6% (w/v) PAGE, and marker data were scored based on differential separation of amplicon(s). BC2F2 plants possessing maximum recurrent parent genome were identified with reproducible polymorphic SSR markers (Supplementary Table S3). The percent recurrent parent genome recovery in a BC2F2 plant was calculated as the sum of the number of alleles corresponding to recurrent parent detected by polymorphic markers divided by the total number of alleles detected by polymorphic and cry1Ac-specific markers.
Assessment for Agronomic Traits
The agronomic performance of BC2F2 population was assessed for plants analyzed for recurrent parent genome recovery, and of BC2F3 population was based on three progeny plants (from each BC2F2 plant) having phenotype similar to the recurrent parent. The data were recorded on days to 50% flowering, number of branches per plant, days to maturity, plant height, number of pods per plant, number of seeds per plant, 100-seed weight, biological yield, seed yield per plant, and harvest index, compared with the recurrent parent and analyzed for percent phenome recovery in BC2F2 plants and for mean ± standard deviation in BC2F3 plants.
Bioassay for Determining Toxicity to H. armigera
Four-month-old morphologically healthy plants were analyzed for toxicity to H. armigera using two approaches, i.e., detached leaf bioassay and whole plant bioassay given by Sharma et al. (2005a), Sharma et al. (2005b) with modifications. Detached leaf bioassay: The terminal twigs having fully expanded leaflets were plucked from F1, backcross population (BC1F1, BC1F2 and BC2F2), transgenic donor parent and non-transgenic recipient parent plants, and placed on 3% (w/v) agar (HiMedia, India) medium slants in sterile 500 ml plastic cups and used for bioassay. H. armigera larvae (3rd to 4th instar) collected in February from chickpea fields were reared individually in bioassay cups and maintained initially on non-transformed tender chickpea twigs, followed by growth on a semi-synthetic diet (Armes et al., 1992) until pupation. The pupae were kept on moist sponges covered with filter paper (Whatman, United States) in plastic containers till the emergence of adults that were paired in oviposition chambers i.e., cell pots wrapped in black paper on all sides and covered with muslin cloth on top. The adults were fed on 5% (v/v) honey solution by hanging honey-soaked cotton swab inside each oviposition chamber. Subsequently, egg laying occurred on the muslin cloth that was shifted to bioassay cup containing semi-synthetic diet for egg hatching, thereafter, neonates were used for bioassay of plant twigs. Ten neonate larvae were released in each bioassay cup and incubated in a growth chamber (Saveer Biotech Limited, India) maintained at 25 ± 2 C, 14 h light: 10 h dark period and >65 ± 5 percent relative humidity. The bioassay was replicated thrice and performed in the Pulses Entomology Laboratory, Department of Plant Breeding & Genetics, PAU, Ludhiana. The assayed plants were visually scored for the damage caused by neonate larvae after 96 h of release on a scale of 1–9 (1 = < 10% leaf area damaged, and 9 = > 80% leaf area damaged) given by Sharma et al. (2005a) for detached leaf assay. The larval mortality rate was compared among plants of backcross populations and donor and recipient parents to monitor the relationship between percent larval mortality and Cry1Ac protein concentration. The data were analyzed for mean ± standard deviation.
Whole plant bioassay: The assay was carried out under net house conditions on plants grown in plots with row to row distance of 40 cm and plant to plant spacing of 10 cm, according to the method given by Sharma et al. (2005b) for screening chickpea against H. armigera under greenhouse conditions with modifications. The healthy plants at the flowering stage from BC1F3 and BC2F3 populations and donor and recipient parents were covered with cages sized 25 × 25 × 75 cm3. The cages made of galvanized iron wire (2 mm in diameter) were supported by four vertical bars and covered with a muslin cloth bag. The experiment was performed in triplicate by caging three plants of each population, parent individually and releasing 10 H. armigera neonatal larvae on each plant, and terminated after 120 h when significant leaf area was damaged in recipient parents. The plants were scored for leaf-feeding visually on a 1-9 scale (where 1 = ˂ 10%, 2 = 11–20%, 3 = 21–30%, 4 = 31–40%, 5 = 41–50%, 6 = 51–60%, 7 = 61–70%, 8 = 71–80% and 9 = > 80% leaf area and/or pods damaged). The number of surviving larvae was recorded and individually placed in 25 ml plastic cups to express the data as percent larval mortality that were analyzed using Microsoft Excel 2007 software. No insecticide was applied in the experiment.
Statistical Analysis
Data on Cry1Ac protein concentration, leaf-feeding, and larval mortality in backcross populations are presented as mean ± SD of three replicates. Statistical significance for the segregation data was determined using Chi-square analysis; calculated Chi-square value > table value was considered statistically significant at 5 percent level of significance.
Results
Analysis on F1 Plants for Determining Toxicity to H. armigera
F1 plants developed from Cross A, Cross B, Cross C, and transgenic donor parent and non-transgenic recipient parents were analyzed for toxicity to H. armigera. F1 plants obtained from Cross A (seven in number), Cross B (seven), Cross C (three), and donor parent displayed 100% H. armigera neonatal larval mortality and negligible (<10–20%) leaf-feeding damage, whereas recipient parents exhibited 23.33–30% larval mortality on an average with significant (51 to 70%) leaf-feeding damage (Table 1). F1 plants toxic to H. armigera were backcrossed to generate BC1F1 populations.
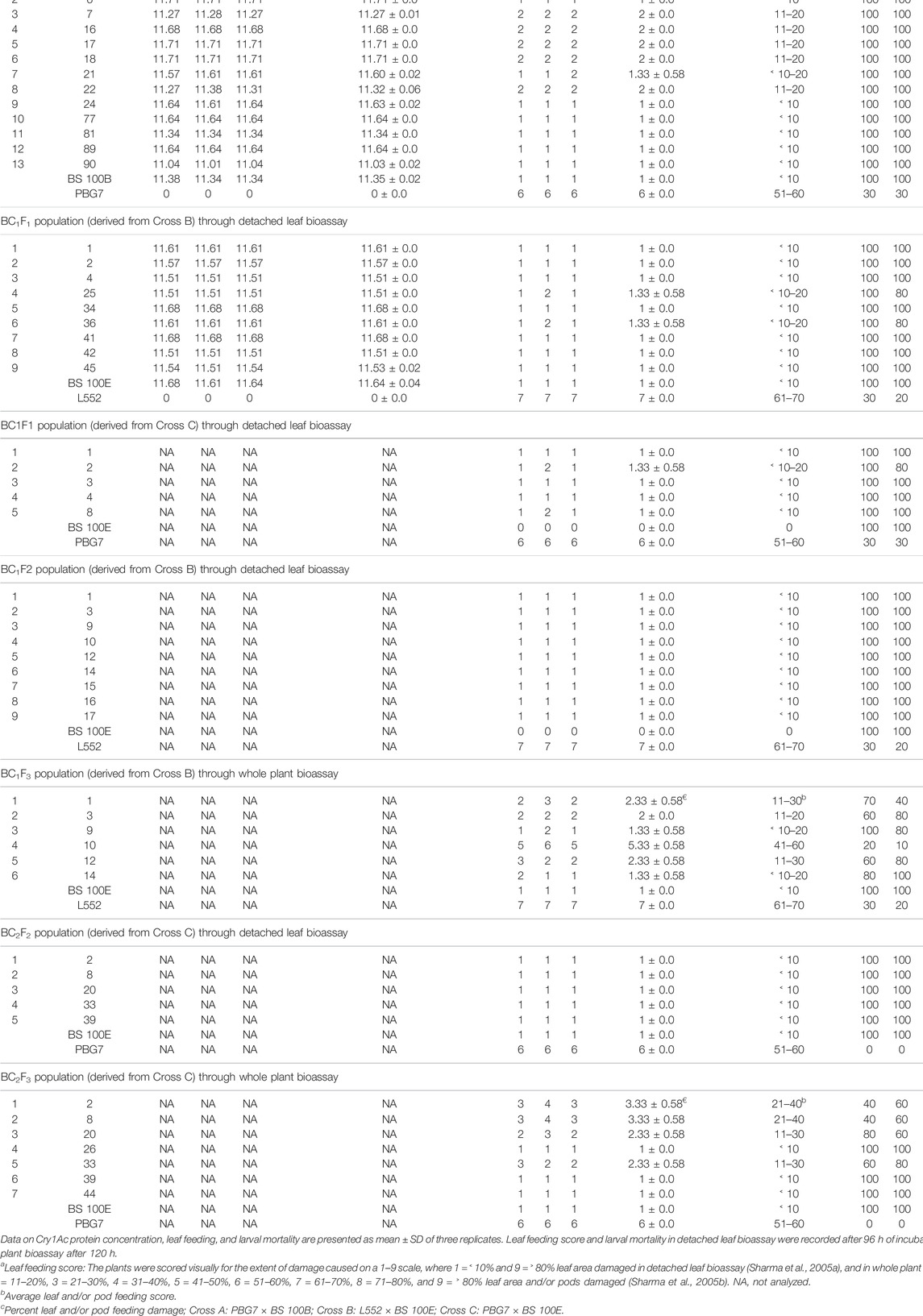
TABLE 1. Bioassay on F1 plants and backcross populations raised by crossing commercial chickpea cultivars with cry1Ac transgenic lines for toxicity to H. armigera neonatal larvae.
Analysis on BC1F1 Populations for Foreground Selection and Determining Toxicity to H. armigera
The foreground selection of two BC1F1 populations derived from Cross A and Cross B, comprising 130 and 50 plants, respectively, was carried out through PCR using cry1Ac-specific primers. An amplicon corresponding to cry1Ac was detected in 46 (35.38%) BC1F1 plants obtained from Cross A (Supplementary Figure S3; Supplementary Table S1). The transgene segregation in a ratio of 1:1.8 deviated significantly from the 1:1 ratio expected if transgene was inserted at a single locus (Table 2). BC1F1 raised from Cross B segregated for the transgene in an expected Mendelian ratio of 1:1, as 25 (50.0%) plants were found to be cry1Ac positive (Supplementary Figure S4).
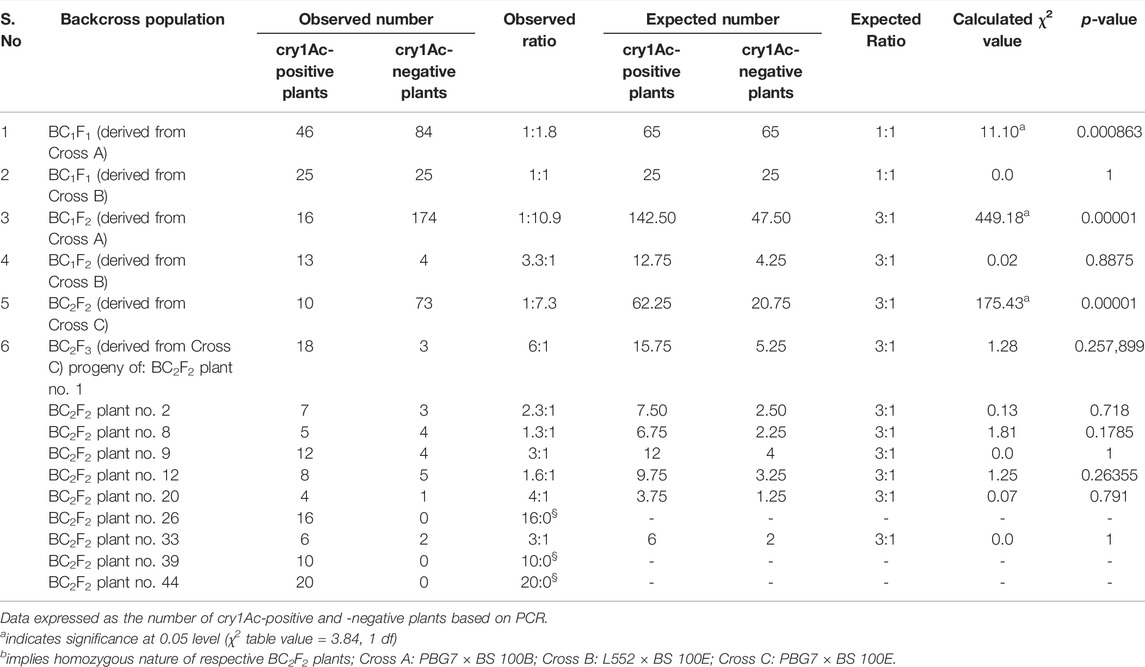
TABLE 2. Segregation analyses of backcross populations developed by crossing commercial chickpea cultivars with cry1Ac transgenic lines.
The recombinant protein concentration was estimated in 13 healthy BC1F1 plants derived from Cross A showing amplification of cry1Ac, nine Cross B plants along with transgenic donor parents BS 100B and BS 100E, and non-transgenic recipient parents PBG7 and L552 through ELISA (Supplementary Table S2). The average Cry1Ac protein concentration in both populations (11.03 to 11.71 μg g−1 leaf tissue) was at par with donor parents (11.35 to 11.64 μg g−1), whereas recipient parents did not exhibit any Cry1Ac concentration (Table 1). The BC1F1 plants (13 obtained from Cross A, nine from Cross B, and five from Cross C) had a phenotype similar to the recurrent parent, their bioassay for toxicity to H. armigera revealed that 13, 7, 4 plants from respective crosses, donor parents showed 100% H. armigera mortality and minor (<10–20%) leaf-feeding damage; in contrast, recipient parents exhibited 23.33–30% larval mortality with significant (51 to 70%) leaf-feeding damage (Table 1; Supplementary Figure S5). BC1F1 plants displaying toxicity to H. armigera were advanced for raising BC1F2 populations.
Analysis on BC1F2 Populations for Foreground Selection and Determining Toxicity to H. armigera
The foreground selection was carried out on two BC1F2 populations: the first comprising of 190 plants derived from Cross A, and the second consisting of 17 plants obtained from Cross B; cry1Ac amplification was detected in 16 (8.4%) and 13 (76.5%) plants (Supplementary Figures S6, S7; Supplementary Table S1), exhibiting non-Mendelian (1:10.9) and Mendelian (3.3:1) segregation ratios in the two populations, respectively (Table 2).
The insect bioassay was performed on nine BC1F2 plants raised from Cross B displaying phenotypic growth similar to the recurrent parent, along with transgenic donor and non-transgenic recipient parents. In the BC1F2 plants, donor parent displayed 100% H. armigera mortality and negligible (<10%) leaf-feeding damage; however, recipient parent showed 23.33% larval mortality with significant (61 to 70%) leaf-feeding damage (Table 1). BC1F2 plants showing toxicity to H. armigera were used to raise BC1F3 population.
Analysis on BC1F3 Population for Determining Toxicity to H. armigera
Six out of 26 BC1F3 plants having comparable phenotype to the recurrent parent developed from Cross B, transgenic donor parent, and non-transgenic recipient parent were analyzed for toxicity to H. armigera. The plants revealed 16.67–93.33% larval mortality and variable (˂ 10–60%) leaf and pod feeding damage; donor parent exhibited 100% H. armigera mortality with negligible (<10%) leaf and pod feeding damage, whereas recipient parent showed 23.33% larval mortality and significant (61 to 70%) damage to leaves and pods (Table 1). Two BC1F3 plants were observed to display 93.33% insect mortality.
Analysis on BC2F2 Population for Foreground and Background Selection and Determining Toxicity to H. armigera.
The foreground selection of BC2F2 population derived from Cross C and comprising of 83 plants led to the identification of 10 (12.05%) plants showing amplification of cry1Ac (Supplementary Table S1). The population deviated significantly for transgene segregation (1:7.3) from the Mendelian ratio (3:1) for a single insertion site (Table 2).
The donor and recipient parents were assessed for polymorphism using 210 SSR markers leading to the identification of 25 (11.9%) polymorphic markers (Supplementary Figure S8; Supplementary Table S3). The background selection using reproducible polymorphic markers on cry1Ac-positive BC2F2 plants demonstrated amplification pattern in ten BC2F2 plants (designated as 1, 2, 8, 9, 12, 20, 26, 33, 39, and 44) to be similar to recurrent parent “PBG7” profile (Figure 2), and the average recurrent parent genome recovery in these plants after two backcrosses was calculated to be 91.3% (Supplementary Table S4). The comparison of agronomic traits in BC2F2 plants with PBG7 revealed an average recurrent parent phenome recovery of 90.94% in BC2F2 plants (Table 3; Supplementary Table S5).
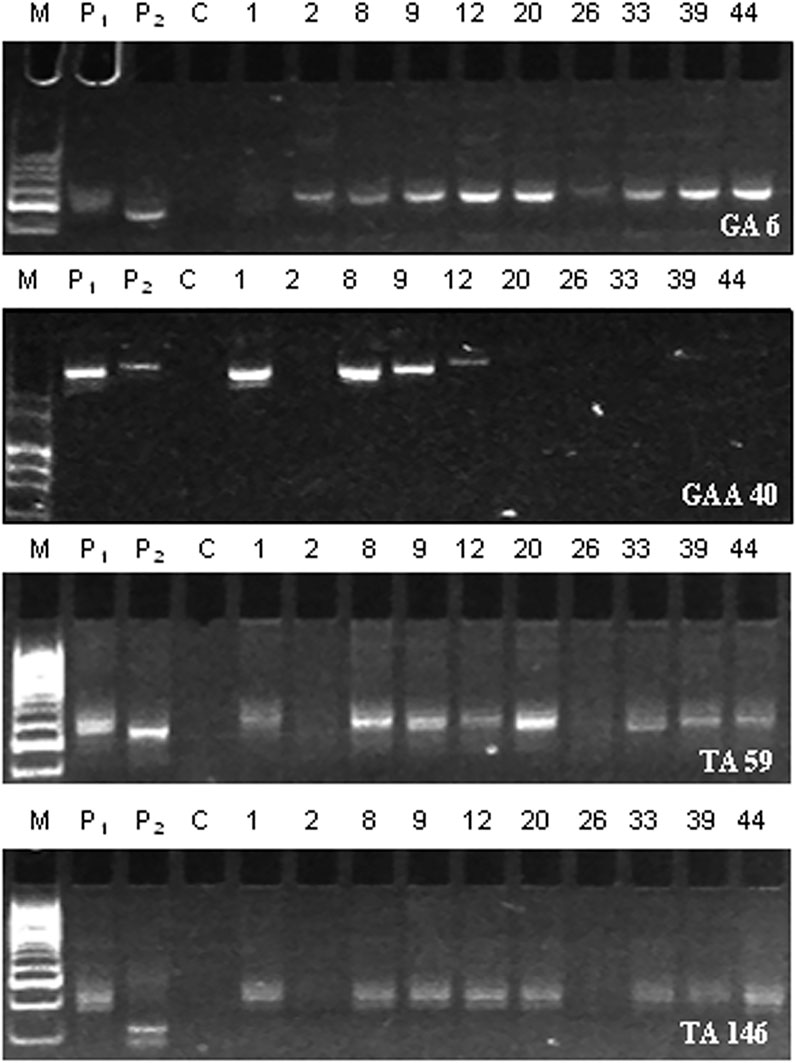
FIGURE 2. SSR amplification profiles of BC2F2 plants using polymorphic markers, namely, GA 6, GAA 40, TA 59, and TA 146. P1 indicates non-transgenic recipient parent PBG7; P2 represents transgenic donor parent BS 100E; C refers to control PCR reaction without template DNA; the numbers 1, 2, 8, 9, 12, 20, 26, 33, 39, and 44 denote BC2F2 plants; and M represents 50 bp DNA ladder (Cat. No. DM1100, SMOBIO Technology, Inc., Taiwan).
The randomly selected BC2F2 plants (designated as 2, 8, 20, 33, and 39) were bioassayed for toxicity to H. armigera. The results revealed that the selected plants and transgenic donor parent exhibited 100% larval mortality and negligible (<10%) leaf-feeding damage, whereas non-transgenic recipient parent was vulnerable to H. armigera with no larval mortality and significant (51 to 60%) leaf-feeding damage (Table 1). Subsequently, seeds of all ten BC2F2 plants were sown to obtain BC2F3 population.
Analysis on BC2F3 Population for Foreground Selection, Agronomic Traits, and Determining Toxicity to H. armigera.
BC2F3 population obtained from Cross C, consisting of 128 plants was subjected to foreground selection for identifying BC2F2 plants homozygous for cry1Ac through recognition of BC2F3 plants carrying cry1Ac gene. The results revealed that on an average, 82.81% BC2F3 plants carried cry1Ac, and three (30%) BC2F2 plants designated as 26, 39, and 44 were homozygous for the transgene as all progeny plants (16 of plant no. 26, 10 of plant no. 39, and 20 of plant no. 44) contained the transgene (Table 2: Figure 3). On the contrary, the remaining seven BC2F2 plants designated as 1, 2, 8, 9, 12, 20, and 33 were hemizygous for cry1Ac with their BC2F3 progeny plants segregating in a ratio of 6:1, 2.3:1, 1.3:1, 3:1, 1.6:1, 4:1, and 3:1, respectively for transgenes that were found to fit in Mendelian 3:1 ratio expected for a selfed population (Table 2).
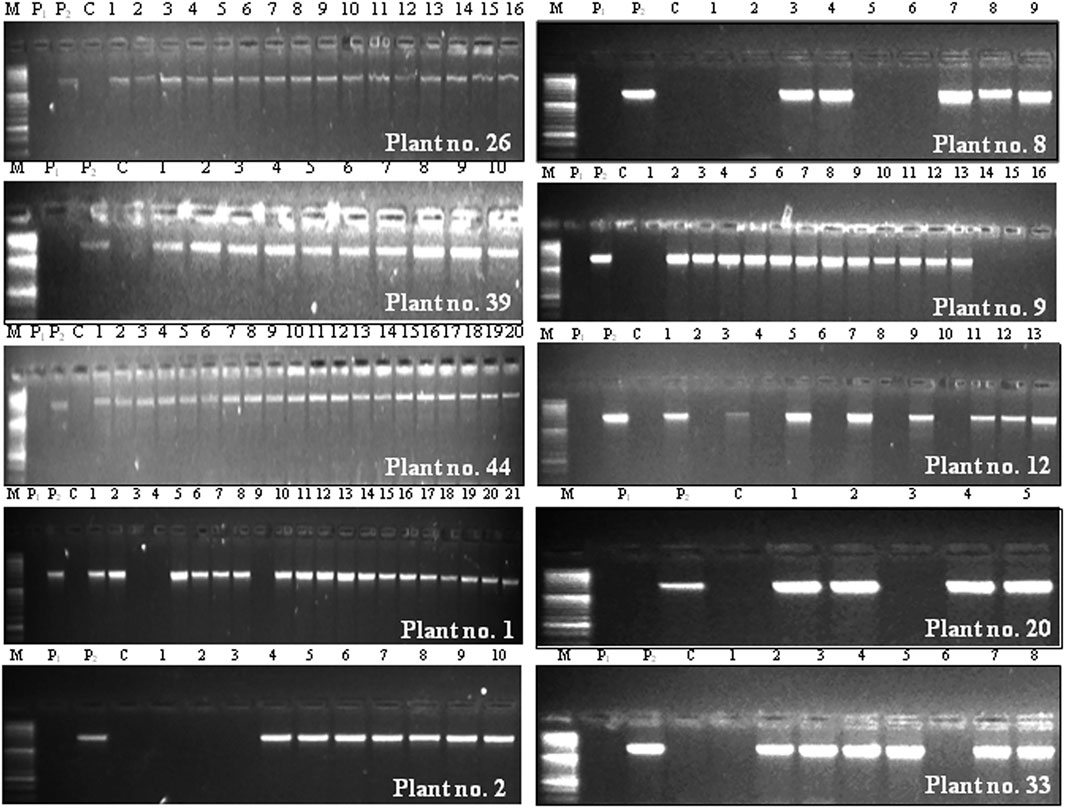
FIGURE 3. Foreground selection of BC2F3 population derived from Cross C (PBG7 × BS 100E) through PCR using cry1Ac-specific primers. P1 indicates non-transgenic recipient parent PBG7; P2 represents transgenic donor parent BS 100E; C refers to control PCR reaction without template DNA; the numbers on top of each gel represent BC2F3 progenies of a specific plant, and its identity is mentioned in the right bottom corner of each gel; the plants designated as 26, 39, and 44 were homozygous for cry1Ac, and those designated as 1, 2, 8, 9, 12, 20, and 33 were hemizygous for the transgene; M represents 50 bp DNA ladder (Cat. No. DM1100).
BC2F3 progeny plants belonging to seven BC2F2 plants, namely 2, 8, 20, 26, 33, 39, and 44, were assessed for agronomic performance. The results showed that the mean number of pods and seed yield of BC2F3 progeny plants derived from BC2F2 plant no. 20 and homozygous BC2F2 plants, namely 26, 39, and 44 were 53.67 ± 1.53, 54.00 ± 3.00, 55.00 ± 4.00, 53.67 ± 3.78, and 15.64 ± 0.51 g, 15.33 ± 0.98 g, 15.74 ± 0.62 g, 14.84 ± 0.53 g, respectively were statistically similar to mean number of pods (54.67 ± 3.05) and seed yield (15.90 ± 0.56 g) of recurrent parent (PBG 7) (Table 3; Supplementary Table S5).
The bioassay of BC2F3 progeny plants revealed 53.33–100% H. armigera larval mortality and variable (<10–40%) leaf and pod feeding damage; amongst these, the progeny of homozygous BC2F2 plants displayed 100% mortality with negligible (<10%) leaf and pod feeding damage (Table 1; Figure 4A,B). The larval mortality in transgenic donor parent and BC2F3 progeny plants was similar, whereas the non-transgenic recipient parent displayed no larval mortality and significant (51 to 60%) leaf, pod feeding damage (Figure 4C).
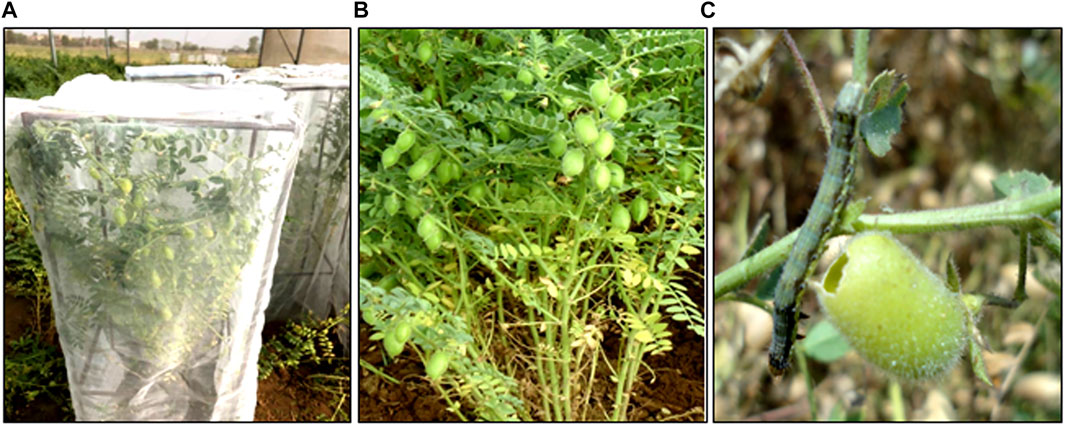
FIGURE 4. Bioassay on BC2F3 plants obtained from Cross C (PBG7 × BS 100E) expressing cry1Ac for toxicity to H. armigera through whole plant screening. (A) Caged plants displaying healthy leaves and pods. (B) Closer view of plant showing healthy leaves and pods. (C) Non-transgenic recipient parent PBG7 exhibiting damaged pod and surviving larva.
Discussion
The elite, commercial chickpea cultivars susceptible to pod borer were converted into resistant by introgressing cry1Ac from transgenic lines through marker-assisted backcross breeding. F1 plants and their backcross populations i.e., BC1F1, BC1F2, BC1F3, BC2F2, and BC2F3, exhibited up to 100% H. armigera neonatal larval mortality with agronomic performance similar to that of the recurrent parent. The high larval mortality was a result of Cry1Ac protein accumulation up to 11.00 μg g−1 in backcross populations; Bt protein concentration as low as 0.9–3.1 μg g−1 is reported to be highly insecticidal to corn earworm, Helicoverpa spp. in backcross populations of Brassica napus lines × wild B. rapa (Halfhill et al., 2001; Zhu et al., 2004). Zhang et al. (2012) and Chen et al. (2018) demonstrated that an even lower Cry1Ac concentration (0.5 to 1.2 μg g−1) in the artificial diet of H. armigera larvae induced distinct histopathological changes in goblet cells of larval midgut epithelial lining, such as breakage of microvilli, endoplasmic reticulum, disorganization of mitochondria and chromatin, 2–36 h after Cry1Ac ingestion that eventually caused mortality.
BC1F1 and BC1F2 populations derived from Cross B segregated in Mendelian ratios of 1:1 and 3:1, respectively, for cry1Ac under contained field conditions; similarly, BC2F3 progenies of hemizygous BC2F2 plants raised from Cross C also segregated in Mendelian ratio of 3:1, pointing toward stable inheritance of cry1Ac as a single dominant gene in plants of different backcross populations. The typical 3:1 segregation ratio in selfed population and 1:1 in backcross population (Peng et al., 1992; Datta et al., 1998) often results from the insertion of one copy of the foreign gene in the host genome. The introgression of cry1Ab transgene following marker-assisted breeding has been reported in BC2F2 and BC1 generations of cotton and rice, respectively (Agbios-Agriculture & Biotechnology Strategies (Canada), Inc., 2007; Kiani et al., 2009). The recurrent parent genome recovery in BC2F2 plants was higher (91.3%) in this study as compared to 87.5% genetic similarity to the recurrent parent obtained after two backcrosses through conventional breeding (Venkatesh et al., 2015). A recurrent parent genome recovery of 95.9% in BC2F2 rice plants was reported using polymorphic SSR markers by Chukwu et al. (2020). The marker-assisted backcross breeding is a dynamic approach for conveniently recognizing plants that have recovered over 98% of the recurrent parent genome in two to three backcross generations depending upon the availability of polymorphic markers (Stojsin 2010). The similarity of BC2F2 plants for agronomic traits with the recurrent parent in our study pointed toward the recurrent parent genome recovery, suggesting that in a situation where a limited number of polymorphic markers is available, the phenotypic characterization for agronomic traits is important. Joseph et al. (2004) reported that phenotypic selection coupled with fewer polymorphic markers between the parental lines maximizes recurrent parent genome recovery. We observed that the agronomic performance of BC2F3 progeny plants (derived from homozygous BC2F2 plants 26, 39 and 44) for main traits i.e., the number of pods and seed yield was statistically similar to the recurrent parent. Likewise, marker-assisted breeding between β-carotene-rich inbred lines UMI1200β+, UMI1230β+ × HKI163 in maize resulted in the development of improved BC2F3 lines exhibiting agronomic traits e.g., cob weight and single plant yield similar to the recurrent parents (Chandran et al., 2019).
The distorted segregation ratios were detected in BC1F1 and BC1F2 populations developed from Cross A. The distorted ratios generally arise due to transgene inactivation (Matzke and Matzke 1995), low viability/fertilization ability of transgenic pollen (Zhang et al., 1996), reduced germination (Sachs et al., 1998), genetic background (Scott et al., 1998; Wu et al., 2002), recessive lethal (Scott et al., 1998) etc. In the present study, segregation distortion in BC1F1 and BC1F2 populations might be a result of reduced germination and not due to 1) transgene inactivation: as cry1Ac amplicon was observed in 46 plants from a total of 130 BC1F1 plants, and 16 out of 190 BC1F2 plants 2) low viability/fertility of transgenic pollen: as the pollen from recipient parent PBG7 (and not from the transgenic line) was used to pollinate F1 plants to obtain BC1F1 plants; and further both BC1F1 and BC1F2 populations had resulted from a cross between desi PBG7 and desi BS 100B. Wu et al. (2002) observed that crosses between japonica and japonica rice had no significant effect on segregation ratios of cry1Ab, whereas japonica × indica resulted in distorted gene segregation in F2 population, 3) genetic background: as both PBG7 and BS 100B are desi chickpeas, or 4) recessive lethal: as BC1F1 plants were hemizygous in nature for cry1Ac. Our assumption of reduced germination responsible for distorted segregation ratios draws support from observations by Sachs et al. (1998) on non-Mendelian segregation of cry1Ac in F2 populations derived from MON 249 × CAMD-E due to failure of a large number of F2 seeds inheriting cry1Ac to germinate. They further suggested that reduced germination associated with the inheritance of cry1Ac in MON 249 plants was a result of direct insertion effect leading to silencing of one or more native genes. In our case, we conclude that reduced germination was possibly associated with the inheritance of cry1Ac gene present in BS 100B plants. cry1Ac integration in the genomes of homozygous BC2F2 plants and BC2F3 progeny plants is probably at the same position as backcross populations obtained from a single transformation event are reported to carry transgene at a constant position in the genomes (Bakó et al., 2013).
The genetic background of BC1F1 populations raised from Cross A and Cross B did not affect the transgene expression as Cry1Ac protein concentration in the two BC1F1 populations was similar to each other and transgenic donor line. This observation is consistent with reports on hybrids of Bt maize (Fearing et al., 1997; Bakó et al., 2013) and eggplant (Ripalda et al., 2012) producing a similar amount of Cry protein in backcross populations irrespective of genetic background. However, this might not always be true as Sachs et al. (1998) observed that cryIA gene expression in cotton lines was influenced by the background genotype. The backcross populations of chickpea F1 plants displayed a high degree of resistance to pod borer as compared to PBG7 and L552, implying stable expression of Cry1Ac throughout different generations.
In conclusion, cry1Ac was introgressed from transgenic chickpea lines into commercial cultivars through marker-assisted backcross breeding for imparting pod borer resistance; consequently, the backcross populations exhibited up to 100% H. armigera larval mortality. The BC2F2 plants homozygous for cry1Ac with high recurrent parent phenome recovery were identified; their BC2F3 progeny plants displaying agronomic performance similar to the recurrent parent are a valuable source of H. armigera resistance and can be used in chickpea breeding programs.
Data Availability Statement
The original contributions presented in the study are included in the article/Supplementary Material, further inquiries can be directed to the corresponding author.
Author Contributions
AK analyzed and interpreted data on foreground selection, background selection, insect bioassay, agronomic traits, prepared the original draft, and edited the manuscript. US carried out foreground selection, background selection, and data compilation. SrS conceptualized the project, arranged funding, attempted crosses, monitored backcrosses and backcross populations, and edited the manuscript. RS reared H. armigera neonatal larvae, carried out insect bioassay, and collected data. YV planned and coordinated background selection. StS and KK carried out ELISA and interpreted the results. PM helped in analyzing segregation data. IS and SB attempted backcrosses, raised backcross populations, and collected agronomic data. BS provided seed of transgenic lines. JS planned and coordinated foreground selection and interpreted the data, prepared the original draft, and edited the manuscript. All the authors approved the final version of the manuscript.
Funding
This work was supported by the Department of Biotechnology, Government of India, with grant number BT/ISCB/Chickpea-PAU/2016 dated 18.5.2017 on “Introgression of Bt-genes (cry1Ac) into elite chickpea lines through backcross breeding and evaluation of introgressed lines against Helicoverpa armigera.”
Conflict of Interest
The authors declare that the research was conducted in the absence of any commercial or financial relationships that could be construed as a potential conflict of interest.
The reviewer MST declared a past co-authorship with the author(s) SS to the handling editor.
Acknowledgments
All authors thankfully acknowledge infrastructural support provided by the Pulses Section, Department of Plant Breeding & Genetics, School of Agricultural Biotechnology, PAU, Ludhiana, and Regional Research Station, Faridkot, for carrying out the research work.
Publisher’s Note
All claims expressed in this article are solely those of the authors and do not necessarily represent those of their affiliated organizations, or those of the publisher, the editors, and the reviewers. Any product that may be evaluated in this article, or claim that may be made by its manufacturer, is not guaranteed or endorsed by the publisher.
Supplementary Material
The Supplementary Material for this article can be found online at: https://www.frontiersin.org/articles/10.3389/fgene.2022.847647/full#supplementary-material
References
Acharjee, S., Sarmah, B. K., Kumar, P. A., Olsen, K., Mahon, R., Moar, W. J., et al. (2010). Transgenic Chickpeas (Cicer Arietinum L.) Expressing a Sequence-Modified cry2Aa Gene. Plant Sci. 178, 333–339. doi:10.1016/j.plantsci.2010.02.001
Agbios-Agriculture & Biotechnology Strategies (Canada), Inc. (2007). Pre-market Environmental Risk Assessment of Transgenic Plants: A Case-Study Approach Utilizing Mon 15985 Cotton, 1–81.
Armes, N. J., Bond, G. S., and Cooters, R. J. (1992). The Laboratory Culture and Development of Helicoverpa Armigera. Chatham, United Kingdom: Natural Resources Institute.
Bakó, A., Gell, G., Zámbó, Á., Spitkó, T., Pók, I., Pintér, J., et al. (2013). Monitoring Transgene Expression Levels in Different Genotypes of Field Grown maize (Zea mays L.). South Afr. J. Bot. 84, 6–10. doi:10.1016/j.sajb.2012.09.005
Bravo, A., Gill, S. S., and Soberón, M. (2008). Mode of Action of Bacillus Thuringiensis Cry and Cyt Toxins and Their Potential for Insect Control. Toxicon 49, 423–435. doi:10.1016/j.toxicon.2006.11.022
Chakrabarti, S. K., Mandaokar, A., Kumar, P. A., and Sharma, R. P. (1998). Efficacy of Lepidopteran Specific δ-Endotoxins ofBacillus thuringiensisagainstHelicoverpa Armigera. J. Invertebr. Pathol. 72, 336–337. doi:10.1006/jipa.1998.4786
Chandran, S., Pukalenthy, B., Adhimoolam, K., Manickam, D., Sampathrajan, V., Chocklingam, V., et al. (2019). Marker-Assisted Selection to Pyramid the Opaque-2 (O2) and β-Carotene (crtRB1) Genes in Maize. Front. Genet. 10, 859. doi:10.3389/fgene.2019.00859
Chen, W., Liu, C., Lu, G., Cheng, H., Shen, Z., and Wu, K. (2018). Effects of Vip3AcAa+Cry1Ac Cotton on Midgut Tissue in Helicoverpa Armigera (Lepidoptera: Noctuidae). J. Insect Sci. 18, 1–6. doi:10.1093/jisesa/iey075
Cherry, A. J., Rabindra, R. J., Parnell, M. A., Geetha, N., Kennedy, J. S., and Grzywacz, D. (2000). Field Evaluation of Helicoverpa Armigera Nucleopolyhedrovirus Formulations for Control of the Chickpea Pod-Borer, H. Armigera (Hubn.), on Chickpea (Cicer Arietinum Var. Shoba) in Southern India. Crop Prot. 19, 51–60. doi:10.1016/s0261-2194(99)00089-7
Chukwu, S. C., Rafii, M. Y., Ramlee, S. I., Ismail, S. I., Oladosu, Y., Muhammad, I. i., et al. (2020). Recovery of Recurrent Parent Genome in a Marker-Assisted Backcrossing against rice Blast and Blight Infections Using Functional Markers and SSRs. Plants 9, 1411. doi:10.3390/plants9111411
Datta, K., Vasquez, A., Tu, J., Torrizo, L., Alam, M. F., Oliva, N., et al. (1998). Constitutive and Tissue-specific Differential Expression of the cryIA(b) Gene in Transgenic rice Plants Conferring Resistance to rice Insect Pest. Theor. Appl. Genet. 97, 20–30. doi:10.1007/s001220050862
Fearing, P. L., Brown, D., Vlachos, D., Meghji, M., and Privalle, L. (1997). Quantitative Analysis of CryIA(b) Protein Expression in Bt maize Plants, Tissues, and Silage and Stability of Expression over Successive Generations. Mol. Breed. 3, 169–176. doi:10.1023/a:1009611613475
Fitt, G. P. (1989). The Ecology of Heliothis Species in Relation to Agroecosystems. Annu. Rev. Entomol. 34, 17–53. doi:10.1146/annurev.en.34.010189.000313
Guo, W., Zhang, T., Zhu, X., and Pan, J. (2005). Modified Backcross Pyramiding Breeding with Molecular Marker-Assisted Selection and its Applications in Cotton. Zuo Wu Xue Bao 31, 963–970.
Halfhill, M. D., Richards, H. A., Mabon, S. A., and Stewart, C. N. (2001). Expression of GFP and Bt Transgenes in Brassica Napus and Hybridization with Brassica Rapa. Theor. Appl. Genet. 103, 659–667. doi:10.1007/s001220100613
Hazarika, N., Acharjee, S., Boruah, R. R., Babar, K., Parimi, S., Char, B., et al. (2019). Enhanced Expression of Arabidopsis Rubisco Small Subunit Gene Promoter Regulated Cry1Ac Gene in Chickpea Conferred Complete Resistance to Helicoverpa Armigera. J. Plant Biochem. Biotechnol., 1–11. doi:10.1007/s13562-019-00531-1
Hospital, F., and Charcosset, A. (1997). Marker-assisted Introgression of Quantitative Trait Loci. Genetics 147, 1469–1485. doi:10.1093/genetics/147.3.1469
Jenkins, N. E., and Grzywacz, D. (2000). Quality Control of Fungal and Viral Biocontrol Agents - Assurance of Product Performance. Biocontrol Sci. Tech. 10, 753–777. doi:10.1080/09583150020011717
Joseph, M., Gopalakrishnan, S., Sharma, R. K., Singh, V. P., Singh, A. K., K. Singh, N., et al. (2004). Combining Bacterial Blight Resistance and Basmati Quality Characteristics by Phenotypic and Molecular Marker-Assisted Selection in rice. Mol. Breed. 13, 377–387. doi:10.1023/b:molb.0000034093.63593.4c
Jukanti, A. K., Gaur, P. M., Gowda, C. L. L., and Chibbar, R. N. (2012). Nutritional Quality and Health Benefits of Chickpea (Cicer arietinumL.): a Review. Br. J. Nutr. 108, S11–S26. doi:10.1017/s0007114512000797
Kar, S., Basu, D., Das, S., Ramkrishnan, N. A., Mukherjee, P., Nayak, P., et al. (1997). Expression of cryIA(c) Gene of Bacillus Thuringiensis in Transgenic Chickpea Plants Inhibits Development of Pod-Borer (Heliothis Armigera) Larvae. Transgenic Res. 6, 177–185. doi:10.1023/a:1018433922766
Khatodia, S., Kharb, P., Batra, P., Kumar, P. A., and Chowdhury, V. K. (2014). Molecular Characterization of Bt Chickpea (Cicer Arietinum L.) Plants Carrying cry1Aa3 Gene. Int. J. Curr. Microbiol. App. Sci. 3, 632–642.
Kiani, G., Nematzadeh, G. A., Ghareyazie, B., and Sattari, M. (2009). Genetic Analysis of cry1Ab Gene in Segregating Populations of rice. Afr. J. Biotechnol. 8, 3703–3707. doi:10.5897/AJB09.483
Kumar, M., Yusuf, M. A., Nigam, M., and Kumar, M. (2018). An Update on Genetic Modification of Chickpea for Increased Yield and Stress Tolerance. Mol. Biotechnol. 60, 651–663. doi:10.1007/s12033-018-0096-1
Mallikarjuna, N., Sharma, H. C., and Upadhyaya, H. D. (2007). Exploitation of Wild Relatives of Pigeonpea and Chickpea for Resistance to Helicoverpa Armigera. J. SAT Agric. Res. 3, 4.
Matzke, M. A., and Matzke, A. (1995). How and Why Do Plants Inactivate Homologous (Trans)genes? Plant Physiol. 107, 679–685. doi:10.1104/pp.107.3.679
Mehrotra, M., Singh, A. K., Sanyal, I., Altosaar, I., and Amla, D. V. (2011). Pyramiding of Modified cry1Ab and cry1Ac Genes of Bacillus Thuringiensis in Transgenic Chickpea (Cicer Arietinum L.) for Improved Resistance to Pod Borer Insect Helicoverpa Armigera. Euphytica 182, 87. doi:10.1007/s10681-011-0501-3
Merga, B., and Haji, J. (2019). Economic Importance of Chickpea: Production, Value, and World Trade. Cogent Food Agric. 5, 1615718. doi:10.1080/23311932.2019.1615718
Patil, S. B., Goyal, A., Chitgupekar, S. S., Kumar, S., and El-Bouhssini, M. (2017). Sustainable Management of Chickpea Pod Borer. A Review. Agron. Sustain. Dev. 37, 20. doi:10.1007/s13593-017-0428-8
Peng, J., Kononowicz, H., and Hodges, T. K. (1992). Transgenic Indica rice Plants. Theoret. Appl. Genet. 83-83, 855–863. doi:10.1007/bf00226708
Rao, G. V. R., Rameshwar Rao, V., and Ghaffar, M. A. (2013). Handbook On Chickpea And Pigeonpea Insect Pests Identification And Management, Information Bulletin No. 57. International Crops Research Institute for the Semi-arid Tropics. Patancheru, Andhra Pradesh.
Ripalda, R. R., Hautea, D. M., Narciso, J. O., and Canama, A. O. (2012). Inheritance and Expression of cry1Ac Gene in Bt Eggplant Backcross Populations in the Philippines. Philipp. J. Crop Sci. 37, 1–9.
Sachs, E. S., Benedict, J. H., Stelly, D. M., Taylor, J. F., Altman, D. W., Berberich, S. A., et al. (1998). Expression and Segregation of Genes Encoding CrylA Insecticidal Proteins in Cotton. Crop Sci. 38, 1–11. doi:10.2135/cropsci1998.0011183x003800010001x
Sandhu, J. S., Gupta, S. K., Singh, I., and Bains, T. S. (2012). L 552: A New Bold Seeded Variety of Kabuli Gram. J. Res. Punjab Agric. Univ. 49, 292.
Sanyal, I., Singh, A. K., Kaushik, M., and Amla, D. V. (2005). Agrobacterium-mediated Transformation of Chickpea (Cicer Arietinum L.) with Bacillus Thuringiensis cry1Ac Gene for Resistance against Pod Borer Insect Helicoverpa Armigera. Plant Sci. 168, 1135–1146. doi:10.1016/j.plantsci.2004.12.015
Scott, A., Woodfield, D., and White, D. W. R. (1998). Allelic Composition and Genetic Background Effects on Transgene Expression and Inheritance in white clover. Mol. Breed. 4, 479–490. doi:10.1023/a:1009601321343
Sharma, H. C., Pampapathy, G., Dhillon, M. K., and Ridsdill-Smith, J. T. (2005a). Detached Leaf Assay to Screen for Host Plant Resistance to Helicoverpa Armigera. J. Econ. Entomol. 98, 568–576. doi:10.1093/jee/98.2.568
Sharma, H. C., Pampapathy, G., and Kumar, R. (2005b). Standardization of Cage Techniques to Screen Chickpeas for Resistance to Helicoverpa Armigera (Lepidoptera: Noctuidae) in Greenhouse and Field Conditions. J. Econ. Entomol. 98, 210–216. doi:10.1093/jee/98.1.210
Singh, S., Singh, I., Sandhu, J. S., Gupta, S. K., Bains, T. S., Rathore, P., et al. (2015). PBG7: A New High Yielding Variety of Desi Gram (Cicer Arietinum L.). Agric. Res. J. 52, 212–213. doi:10.5958/2395-146x.2015.00024.1
Stojsin, D. (2010). “Transgenes: Plant Breeding,” in Encyclopedia of Biotechnology in Agriculture and Food. Editors D. R. Heldman, D. G. Hoover, and M. B. Wheeler. 1st ed. (Boca Raton: CRC Press). doi:10.1081/E-EBAF
Venkatesh, T. V., Cook, K., Liu, B., Perez, T., Willse, A., Tichich, R., et al. (2015). Compositional Differences between Near-Isogenic GM and Conventional maize Hybrids Are Associated with Backcross Breeding Practices in Conventional Breeding. Plant Biotechnol. J. 13, 200–210. doi:10.1111/pbi.12248
Wang, Z., Yu, C., and Jiang, L. (2012). Segregation and Expression of Transgenes in the Progenies of Bt Transgenic rice Crossed to Conventional rice Varieties. Afr. J. Biotechnol. 11, 7812–7818. doi:10.5897/ajb12.119
Wu, G., Cui, H., Ye, G., Xia, Y., Sardana, R., Cheng, X., et al. (2002). Inheritance and Expression of the cry1Ab Gene in Bt (Bacillus Thuringiensis) Transgenic rice. Theor. Appl. Genet. 104, 727–734. doi:10.1007/s001220100689
Zhang, S., Warkentin, D., Sun, B., Zhong, H., and Sticklen, M. (1996). Variation in the Inheritance of Expression Among Subclones for Unselected (uidA) and Selected (Bar) Transgenes in maize (Zea mays L.). Theor. Appl. Genet. 92, 752–761. doi:10.1007/bf00226098
Zhang, Y., Liang, G., Zhang, L., and Wei, J. (2012). Pathological Changes in Midgut Tissues of Larvae of the Cotton Bollworm, Helicoverpa Armigera (Lepidoptera: Noctuidae), after Feeding Vip3Aa Protein. Acta Entomol. Sinica 55, 869–876.
Keywords: Cicer arietinum, Mendelian inheritance, transgene introgression, Helicoverpa armigera resistance, marker-assisted backcross breeding
Citation: Kaur A, Sharma U, Singh S, Singh R, Vikal Y, Singh S, Malik P, Kaur K, Singh I, Bindra S, Sarmah BK and Sandhu JS (2022) Introgressing cry1Ac for Pod Borer Resistance in Chickpea Through Marker-Assisted Backcross Breeding. Front. Genet. 13:847647. doi: 10.3389/fgene.2022.847647
Received: 03 January 2022; Accepted: 09 March 2022;
Published: 12 April 2022.
Edited by:
Nasya Borisova Tomlekova, Maritsa Vegetable Crops Research Institute (MVCRI), BulgariaReviewed by:
Salej Sood, Indian Council of Agricultural Research (ICAR), IndiaMohar Singh Thakur, Indian Council of Agricultural Research (ICAR), India
Copyright © 2022 Kaur, Sharma, Singh, Singh, Vikal, Singh, Malik, Kaur, Singh, Bindra, Sarmah and Sandhu. This is an open-access article distributed under the terms of the Creative Commons Attribution License (CC BY). The use, distribution or reproduction in other forums is permitted, provided the original author(s) and the copyright owner(s) are credited and that the original publication in this journal is cited, in accordance with accepted academic practice. No use, distribution or reproduction is permitted which does not comply with these terms.
*Correspondence: Jagdeep Singh Sandhu, js_sandhu@pau.edu