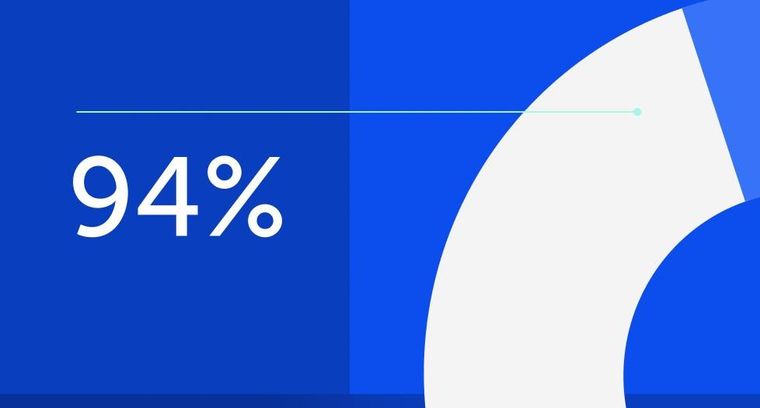
94% of researchers rate our articles as excellent or good
Learn more about the work of our research integrity team to safeguard the quality of each article we publish.
Find out more
REVIEW article
Front. Genet., 15 February 2022
Sec. Evolutionary and Genomic Microbiology
Volume 13 - 2022 | https://doi.org/10.3389/fgene.2022.787665
This article is part of the Research TopicUnconventional Model Systems in Genomic and Biological ResearchView all 5 articles
The evolution of multicellularity is a major evolutionary transition that underlies the radiation of many species in all domains of life, especially in eukaryotes. The volvocine green algae are an unconventional model system that holds great promise in the field given its genetic tractability, late transition to multicellularity, and phenotypic diversity. Multiple efforts at linking multicellularity-related developmental landmarks to key molecular changes, especially at the genome level, have provided key insights into the molecular innovations or lack thereof that underlie multicellularity. Twelve developmental changes have been proposed to explain the evolution of complex differentiated multicellularity in the volvocine algae. Co-option of key genes, such as cell cycle and developmental regulators has been observed, but with few exceptions, known co-option events do not seem to coincide with most developmental features observed in multicellular volvocines. The apparent lack of “master multicellularity genes” combined with no apparent correlation between gene gains for developmental processes suggest the possibility that many multicellular traits might be the product gene-regulatory and functional innovations; in other words, multicellularity can arise from shared genomic repertoires that undergo regulatory and functional overhauls.
The evolution of multicellular organisms is a major Transition in Evolution where unicells relinquish their individuality to collectively coordinate for the development of a complex, higher organizational level individual (John Maynard Smith, 1995; Grosberg and Strathmann, 2007; Knoll, 2011). Multicellularity appears to be a successful solution for adapting to the environment; it evolved independently multiple times in all domains of life (Bonner, 2000; Grosberg and Strathmann, 2007; Ruiz-Trillo and Nedelcu, 2015) having generated extant descendants that showcase a wealth of morphological and developmental innovation. Unicellular organisms have been shown to readily evolve multicellular lifestyles in response to selection (Grosberg and Strathmann, 2007) suggesting that the genetic requirements for multicellularity are not a major hurdle for the transition itself to occur (Rokas, 2008; Arenas-Mena, 2017).
Indeed, experimental evolution studies demonstrate the rapid ability of unicells such as yeast (Ratcliff et al., 2013), and green algae (Boraas et al., 1998; Sathe and Durand, 2015; Fisher et al., 2016; Herron et al., 2019) to transition to multicellularity. Although the recurring nature of the evolution of multicellularity provides a useful base for comparative studies, determining the mechanisms underlying the evolution of multicellularity remains enigmatic due to technical obstacles such as long divergence times between multicellular models and their unicellular relatives and limited toolkits for experimental work in multicellularity models. Tractable models for understanding the evolution of multicellularity are thus indispensable to define the molecular players underlying multicellular evolution. A curious clade of chlorophytes, the volvocine algae, are an important system for addressing the molecular basis of multicellular evolution.
The volvocines are a well-established model system for the study of multicellularity (Nedelcu and Michod, 2003; Kirk, 2005; Miller and Technau, 2010; Niklas, 2014; Grochau-Wright et al., 2017) that encompasses ∼50 extant multicellular species and their closest unicellular relative, Chlamydomonas reinhardtii (Kirk, 1997; Coleman, 1999; Umen and Olson, 2012) (Figure 1A). Multicellular volvocines are arranged into families Tetrabaenaceae (Basichlamys, Tetrabaena), Goniaceae (Gonium, Astrephomene) and Volvocaceae (Pandorina, Eudorina, Yamagishiella, Volvox) (Hanschen et al., 2018a; Lindsey et al., 2021), and display morphologies ranging from bowl-shaped undifferentiated colonies to differentiated spheroids (Kirk, 1997; Coleman, 2012; Yamashita et al., 2016) (Figure 1A). Despite their phenotypic differences, this diverse group of algae evolved from their Chlamydomonas-like unicellular ancestor relatively recently during the Triassic period (around 250 MYA) (Herron et al., 2009). The late evolution of multicellularity of the volvocines has made them attractive subjects for comparative genomic analyses, which have shown that their genomes are highly similar and share conserved synteny (Prochnik et al., 2010; Hanschen et al., 2016; Featherston et al., 2017; Jiménez-Marín et al., 2021). The remarkable genetic similarity between volvocine species suggests the genetic signals relating to multicellularity might still be traceable within the lineage and makes this algal system suitable for the study of multicellularity at the molecular level. The genetic tractability of the volvocines, coupled to the increasing availability of functional genomic tools in key species (Lerche and Hallmann, 2009; Umen and Olson, 2012; Lerche and Hallmann, 2013), their phenotypic diversity and recent divergence from a Chlamydomonas-like ancestor, provide a unique framework for determining the common principles and functional players behind multicellularity, which impacts the broader fields of evolution and development.
FIGURE 1. The evolution of volvocine developmental complexity is marked by gains and losses of traits. Adapted from (Ruiz-Trillo and Nedelcu, 2015; Lindsey et al., 2021). (A) Phylogeny of the volvocine algae. Multicellular volvocine families Tetrabaenaceae, Goniaceae, and Volvocaceae are demarcated in blue, yellow, and pink respectively. Kirk’s lineage explanation for volvocine multicellularity is highlighted in blue. Traits associated to Kirk’s twelve steps are marked by circles; numbers within circles detail what step is described. Teal circles represent evolution of traits and orange circles represent loss of traits. (B) Organismal morphology for depicted species is not to scale. (B) Kirk’s twelve steps do not always have a known genetic origin, and when they do, it is not always co-opted via duplication and divergence.
The seeming stepwise acquisition of phenotypes that the volvocines display (from unicellular to undifferentiated and differentiated multicellular) have been used as the basis for a hypothesis of sequential acquisition of developmental complexity through genetic co-option (Kirk, 2005; Olson and Nedelcu, 2016). David Kirk’s seminal work on this subject suggested that twelve developmental changes could explain the evolution of differentiated multicellularity in Volvox carteri (Kirk, 2005) (Figure 1A, highlighted in blue). At least four of Kirk’s developmental changes are required for undifferentiated multicellularity as exhibited by Tetrabaena and Basichlamys (Figure 1). The best described undifferentiated multicellular species, Gonium pectorale, covers six of Kirk’s twelve steps (Umen and Olson, 2012) (Figure 1), suggesting that within the volvocines, the evolution of undifferentiated multicellularity requires the most developmental changes.
The lineage hypothesis of co-option proposes that volvocine multicellularity resulted from modification of cellular structures and developmental cycles under an otherwise conserved phenotypic background (Kirk, 1997; Bonner, 2000). This appears to be true for volvocine morphology; except for species with differentiated germ cells, the cells that compose volvocine colonies strongly resemble their closest unicellular relative, Chlamydomonas. Chlamydomonas cells are ovoid shaped and asymmetrical (Holmes and Dutcher, 1989) (Figure 1A). At their anterior end, each cell has two flagella that serve chemosensory and motility functions. At their posterior end, cells have a distinctive large cup-shaped chloroplast that occupies the bulk of the intracellular space (Umen and Olson, 2012). Multicellular volvocine cells are enveloped in a proteinaceous extracellular matrix (ECM) that resembles the ECM of animal cells rather than cellulosic cell walls of plants (Sumper and Hallmann, 1998). Interestingly, the multicellular volvocine ECM is biochemically and genetically conserved with Chlamydomonas’ cell wall (Adair et al., 1987; Prochnik et al., 2010; Hanschen et al., 2016), suggesting the ancestral genes were repurposed for new multicellularity functions. Different species secrete various amounts of ECM, with the most extreme example being Volvox. Cells in Volvox are enveloped in ECM layers such that it comprises roughly 99% of the spheroid volume (Kirk, 1997).
Likewise, the multicellular volvocine life cycle is very similar to that of Chlamydomonas. All volvocines are haploid and capable of both vegetative and sexual reproduction (Hoops et al., 2006). Vegetative reproduction occurs in individual cells that undergo a modified cell cycle called “multiple fission” (Cross and Umen, 2015). Except for germ cells in Volvox, every volvocine cell is flagellated and hence provides motility to the whole organism until cell division occurs, at which point the cells retract their flagella, undergo division, daughter cells regrow their flagella and begin swimming again. Given that the volvocine cells are not symmetrical, in multicellular species the organism as a whole must retain defined cell orientations in the context of their bowl (e.g., Gonium) or spheroid (e.g., Pandorina, Eudorina, Pleodorina and Volvox) morphologies and the number of cells in the colony for the organism to successfully swim (Hoops, 1993).
At the molecular level, one of key genetic innovations for volvocine multicellularity is co-option of the cell cycle regulator and tumor suppressor ortholog Retinoblastoma (RB). RB (encoded by the MAT3 gene) from multicellular Gonium causes unicellular Chlamydomonas to be multicellular (Hanschen et al., 2016). As in plants and animals, all volvocines have an RB ortholog that regulates their cell cycles through cyclin-dependent kinase (CDK) phosphorylation (Ferris et al., 2010; Hanschen et al., 2016). RB from multicellular volvocine species differ in their regulatory domains from their ortholog in unicellular Chlamydomonas. First, RB from multicellular volvocines have shorter linkers between the RB-A and RB-B domains compared to Chlamydomonas (Ferris et al., 2010; Hiraide et al., 2013; Hanschen et al., 2016; Featherston et al., 2017). Second, RB from multicellular volvocines lack conservation in C-terminal domain and CDK phosphorylation sites that are present in their unicellular relative (Hiraide et al., 2013; Hanschen et al., 2016; Featherston et al., 2017). Additionally, Volvox carteri has different sex-based isoforms of RB that correlate with oogamy (Ferris et al., 2010). While cases of gene family expansion in the volvocines are limited, several multicellular volvocines, including Gonium, show expansion of cyclin D1 gene family (Prochnik et al., 2010; Hanschen et al., 2016). Cyclin D genes regulate the cell cycle by dimerizing with CDKs and phosphorylating RB proteins (Cross and Umen, 2015; Hanschen et al., 2016; Featherston et al., 2017; Jiménez-Marín et al., 2021); whether cyclins are involved in the evolution of volvocine multicellularity is yet to be determined. Regardless of the impact of cyclins, it would seem likely that cell cycle dependent regulation of gene expression by RB is different for Chlamydomonas compared to its multicellular volvocine relatives, possibly driving the evolution of multicellular development in the latter.
Aside from RB and cyclin D1s, other known co-option events do not seem to coincide with Kirk’s six steps to undifferentiated multicellularity (Figure 1B, steps 1–6). For instance, “ECM-related” genes, which provide structure and cohesion to volvocine colonies, were likely co-opted for multicellularity. However, Gonium and Chlamydomonas share similar numbers of key ECM-related genes despite the former being multicellular and the latter unicellular (Hanschen et al., 2016; Olson and Nedelcu, 2016; Jiménez-Marín et al., 2021). Instead, expansion and diversification of ECM-related genes (pherophorins, matrix metalloproteinases- MMPs) seems to relate more to changes in organismal size than to the evolution of colonial life (Prochnik et al., 2010; Hanschen et al., 2016; Featherston et al., 2017; Jiménez-Marín et al., 2021). Similarly, genes that became co-opted for key volvocine developmental processes originated much before the appearance of the phenotypes with which they are associated (Figure 1).
Differentiated multicellularity in the volvocines has been long thought to be a consequence of developmental innovations that originate from further modification of structures and co-option of genes present in undifferentiated multicellular ancestors, and likely in extant undifferentiated sister species. Spheroidal and bowl shaped volvocine embryos need to undergo a process akin to gastrulation in which the colony shape must change to ensure a final configuration that allows the organism to swim (Hallmann, 2006). This process, called inversion, is known to occur in the Goniaceae [all but Astrephomene (Yamashita et al., 2016)], and Volvocaceae (Hallmann, 2006). The product of invA, a kinesin, is known to play a key role in inversion in Volvox. Orthologs of invA are present in several volvocine species, regardless of their morphology, and noteworthily, Chlamydomonas also has an ortholog for this gene, iar1. Surprisingly, the Chlamydomonas iar1 can rescue Volvox inversion-defective invA mutants (Nishii et al., 2003). However, whether Gonium’s ortholog of invA has a role in inversion remains to be demonstrated. Likewise, co-chaperone glsA, which is involved in asymmetric cell division in Volvox—a process tied to cell differentiation in this and other volvocines, has orthologs in species that do not undergo cell differentiation (Jiménez-Marín et al., 2021). The Chlamydomonas ortholog for glsA, much like iar1, can rescue gonidialess glsA Volvox mutants (Cheng et al., 2003).
Perhaps the best-known example of co-option for a key volvocine developmental processes preceding the phenotype itself is Volvox’s cell differentiation master regulator regA. Whilst there are regA homologs in Chlamydomonas and Gonium (RLS1), there are no orthologs of it in either species (Hanschen et al., 2014; Hanschen et al., 2016). In the species that have it, regA is part of a gene cluster that likely evolved through tandem duplication shortly after Goniaceae split from Volvocaceae (Hanschen et al., 2014; Grochau-Wright et al., 2017). Indeed, the Pandorina (Volvocaceae) genome encodes for the full reg cluster, despite Pandorina being an undifferentiated species (Grochau-Wright et al., 2017). Ancestral state reconstruction of the reg cluster supports the hypothesis that the genetic basis for somatic cell differentiation in the Volvocaceae preceded cellular differentiation itself and was subject to co-option (Grochau-Wright et al., 2017). A recent transcriptome study of Volvox gonidia and somatic cells demonstrated the different cell types have markedly distinct transcriptional programs, and suggest that co-option of certain gene expression programs for differentiation might have driven cell type specialization (Matt and Umen, 2018). A major open question in the field is whether regA acts as a key regulator of these transcriptional programs during development to establish the germ and somatic cell lineages (Olson and Nedelcu, 2016).
Traits related to sexual reproduction are oftentimes highly dynamic evolutionarily. In the case of the volvocines, there are members whose reproductive morphology range from isogamous (Chlamydomonas, Gonium, Pandorina) to anisogamous (Eudorina) and oogamous (Volvox). However, the evolution of anisogamy does not appear to be correlated to the evolution of cellular differentiation, though it does correlate with increased complexity (Hanschen et al., 2018a). The mating-type (MT) loci of different volvocines experience frequent turnover, and their distribution (as well as that of MT linked genes) does not seem to relate to the evolution of anisogamy within the clade. Thus, it is hypothesized that the evolution of volvocine males might be the consequence of altering the function of MID, a sex determining putative transcription factor, or of its target genes, as opposed to the acquisition of novel genes controlling gamete size (Hamaji et al., 2018). Empirical, phylogenetics-based evidence supports predictions that multicellularity is a driver of derived sexual traits, starting with anisogamy and then with sexual dimorphism (Hanschen et al., 2018a).
While the co-option of RB, glsA, regA and other examples here given demonstrates that shared genetic elements can be repurposed for a wide array of biological roles, surprisingly few ‘multicellularity genes’ are found in the volvocines (Figure 1B). Unlike the initial shift to undifferentiated multicellularity that was likely boosted by co-option of RB, it would seem like subsequent genetic changes related to ECM maintenance, inversion, and cell differentiation have roles in stabilizing colonial life and controlling subsequent developmental processes rather than impacting multicellularity itself (Figure 1B). Indeed, Kirk’s streamlined framework has undergone revisions in light of the discovery of a dynamic history of gains and losses of morphological and developmental traits (Nanjundiah et al., 2018; Lindsey et al., 2021) (Figure 1A). Hence, the volvocine phylogenetic tree is under constant revision (Nozaki et al., 2003; Hanschen et al., 2018b; Lindsey et al., 2021). Genera Eudorina, Pleodorina and Volvox are not monophyletic (Figure 1A), and family Goniaceae likely is not either (Lindsey et al., 2021). Ancestral state reconstruction shows that there have been repeated instances of evolution of cell differentiation and loss of cell differentiation within the volvocines (Grochau-Wright et al., 2017; Lindsey et al., 2021) (Figure 1A). Other landmark traits, including the evolution of spheroidal morphologies, inversion, and expansion/contraction of the ECM, seem to have occurred independently more than once as well (Herron et al., 2009; Ruiz-Trillo and Nedelcu, 2015) (Figure 1A). The most extreme example of independent evolution is Astrephomene, a proposed (but debated) sister genus of Gonium, which evolved a spheroidal shape, has sterile somatic cells without the reg cluster, and rearranges itself without undergoing inversion (Yamashita et al., 2016). Upon inspecting gains and losses in morphology, it would seem that the transition to undifferentiated multicellularity is not as readily gained as lost, again supporting the idea that this step is more developmentally complex to evolve. The frequent shifts between simplification and complexification of traits along multicellular volvocine history paint a picture that does not support widespread genetic innovation as a driver of developmental complexity.
One of the most striking features of multicellular evolution in the volvocine algae is how few new genes seem to be required to drive complex developmental changes. Moreover, many such genes are largely conserved in 1:1 orthology across the entire lineage (e.g., invA, glsA, RB/MAT3) and relatively few examples of gene expansion (e.g., cyclin Ds, regA, MMPs). Surprisingly, comparative analyses of multicellular volvocine genomes to unicellular Chlamydomonas (Jiménez-Marín et al., 2021) combined with experimental approaches support the impression that few key genes were co-opted for volvocine developmental traits, and that other processes such as de novo gene evolution are much less important (Hanschen et al., 2016; Olson and Nedelcu, 2016; Jiménez-Marín et al., 2021) to multicellularity in this system. Thus, alternative mechanisms must be behind the dynamic developmental and evolutionary picture the volvocine algae have painted.
Inferences on the minimal role of genetic innovation made through phylogenetic approaches have been confirmed through comparative genomics. Orthologous groups, protein family (Pfam) domains, regulatory protein families, and even histones except for H1 are less numerous in Volvox than they are in less developmentally complex volvocines. Contraction of shared groups among the genomes of Gonium, Pandorina, Yamagishiella, Eudorina and Volvox relative to Chlamydomonas is more frequent than is expansion of other shared elements (Jiménez-Marín et al., 2021). Interestingly, the highly conserved synteny between volvocine genomes facilitates mechanistic analysis of gene loss (that is, analysis of what genes have been lost and the manner of their loss). This analysis shows that losses mainly occur through progressive decay (Jiménez-Marín et al., 2021). What is more, a significant proportion of analyzed gene losses are common to Gonium, Pandorina, Yamagishiella, Eudorina and Volvox but not to Chlamydomonas, strongly suggesting that bursts of gene loss at the last common ancestor (LCA) of the multicellular volvocines might have played a significant role in the transition to multicellularity of this lineage (Jiménez-Marín et al., 2021).
The high sequence similarity shared among volvocine genomes has allowed for detailed advances in our understanding of how small changes have ‘primed’ this algal lineage for multicellularity. However, the small-scale innovations observable in the genome cannot account for the variety of phenotypes that the clade itself possesses. For instance, there are similar abundances of transcription factors (TFs) between Chlamydomonas and Gonium (Hanschen et al., 2016), though there is evidence that certain TF families as well as other regulatory elements are contracting as developmental complexity increases in the multicellular volvocines (Jiménez-Marín et al., 2021). Making sense of these seemingly contradictory data is as challenging as it is promising, and some interesting insight is already becoming available.
The study of gene loss as a driver of evolutionary innovation is relatively recent (Go et al., 2005; Kvitek and Sherlock, 2013; Albalat and Canestro, 2016; Fernandez and Gabaldon, 2020; Guijarro-Clarke et al., 2020), but mathematical modeling suggests that gene loss might be related to changes to the molecular network of the impacted species in such a way that novel interactions might arise (Jiménez-Marín et al., 2021). This could translate to the ancestor of the colonial volvocines evolving multicellularity whilst setting up the resulting lineages for a wide array of developmental outcomes that might explain the discordance between the emergence of gene families, their co-option, and the emergence of more complex phenotypes among species.
Despite a general trend of reduction of histone copy numbers, Gonium histones H2B have a greater N-terminal variant diversity than other volvocines do (Jiménez-Marín et al., 2021). This might mean that differential combinations in histone tails between species could fuel differential post-translational change programs between species that could facilitate increases in developmental complexity even in the context of gene loss. Furthermore, protein-protein interaction (PPI) analysis of Chlamydomonas, Gonium and Eudorina strongly suggests that the proteomic makeup of each species is vastly different even for conserved gene products (Jiménez-Marín et al., 2021). These novel findings hint at the possibility that post-genomic programs have a strong role in generating and stabilizing more developmentally complex morphologies (Figure 2). Perhaps that is why it has proven so difficult to find “multicellularity genes”: differential usage of shared functional repertoires is a likely path to varying phenotypes that cannot be readily identified through comparative genomics alone.
FIGURE 2. Gene loss contributes to molecular network rewiring for volvocine multicellularity in concert with limited co-option and expansion of functional units. (A) current understanding of how molecular networks in a unicellular, Chlamydomonas-like ancestor of the volvocines evolved upon the transition to multicellularity (Adami et al., 2000; Trigos et al., 2018). In this model, network elements are co-opted for multicellular function, and expanded to varying degrees for different multicellular lineages (magenta nodes) with minimal changes to the core “ancestral” (unicellular) network. (B) changes in molecular networks from a unicellular, Chlamydomonas-like ancestor of the volvocines by gene loss and limited co-option to evolve new functions for multicellularity. Some network elements were lost (light blue disconnected nodes), which caused a reconfiguration of the network to promote novel interactions (dotted magenta edges), co-option events (cyan nodes), and expansion of units with novel multicellularity related functions (magenta nodes).
The elusive nature of the molecular players behind evolution of multicellularity and other Major Transitions in biological complexity might be due in part because they do not necessarily have specific genetic determinants. Rather, a few key master regulators of gene expression (e.g., RB and regA) undergo extensive co-option that results in a cascade of genome-wide developmental expression rewiring. In addition to this, it is feasible that epigenetic changes add to differential expression patterns between species; differences in histone diversity and abundance between species (Jiménez-Marín et al., 2021) provide some initial support to this possibility. Among the effects of global network rewiring is the formation of novel interactions at the protein level, which yields a combinatorial co-option of function that alters the organism’s functional repertoire without significantly shifting its genetic repertoire. In other words, rather than duplication and divergence to co-opt the function of genes, their combinations during development are altered for biological novelty. Functions that lose their adaptive value or become dispensable are gradually lost, as they are no longer needed.
The consequence of this framework is that, outside of a few key regulators, there may not be such a thing as “multicellularity genes”. Protein-protein interaction network rewiring should be capable of producing divergent phenotypes without the intervention of extensive genetic novelty. While on its face this mode of evolutionary novelty does not fit preconceptions, it is biologically sound. Unicellular ancestors were jack-of-all trades and had to perform many different functions. As they evolved into undifferentiated and differentiated multicellular organisms, these functions were either used as a “parts bin” for novel functions or their functions were no longer needed and lost. While increased sampling of volvocine genomes might increase the resolution on the history of key multicellularity genes, this framework hints at the need for the exploitation of other “omic” approaches (transcriptomics, proteomics) in conjunction with the usage and development of molecular biology tools (transformation, RNAi, CRISPR) that allow for comparisons between species and between WT and experimental strains under diverse conditions.
Upon transitioning to multicellularity, colonial organisms can switch from strictly temporal gene regulation to spatial or spatial temporal regulation patterns (Mikhailov et al., 2009). In the context that multicellularity has occurred numerous times under vastly different genetic backgrounds, it seems feasible that the many efforts to review the genetic underpinnings of this transition have come up short because multicellular evolution is not a process that requires a handful of genes to orchestrate organismal integration; rather, it requires a complete overhaul of how an organism uses whatever genes it has. Our future understanding of multicellularity will likely require a similar scientific overhaul, where the integration of information at different levels (DNA, RNA, phenotypic data) should lead to a more complete picture of the greater and lesser hurdles underlying this fascinating Major Transition.
BJ-M: Conceptualization, investigation, writing—original draft, visualization. BO: Conceptualization, supervision, writing—review and editing, project administration, funding acquisition.
This material is based upon work supported by the National Science Foundation under Grant Nos. MCB-1715894 and MCB-1412738. BJ-M is supported by a fellowship from the Kansas State University Interdepartmental Genetics Program.
The authors declare that the research was conducted in the absence of any commercial or financial relationships that could be construed as a potential conflict of interest.
All claims expressed in this article are solely those of the authors and do not necessarily represent those of their affiliated organizations, or those of the publisher, the editors and the reviewers. Any product that may be evaluated in this article, or claim that may be made by its manufacturer, is not guaranteed or endorsed by the publisher.
Adair, W. S., Steinmetz, S. A., Mattson, D. M., Goodenough, U. W., and Heuser, J. E. (1987). Nucleated Assembly of Chlamydomonas and Volvox Cell walls. J. Cel Biol 105, 2373–2382. doi:10.1083/jcb.105.5.2373
Adami, C., Ofria, C., and Collier, T. C. (2000). Evolution of Biological Complexity. Proc. Natl. Acad. Sci. 97, 4463–4468. doi:10.1073/pnas.97.9.4463
Albalat, R., and Cañestro, C. (2016). Evolution by Gene Loss. Nat. Rev. Genet. 17, 379–391. doi:10.1038/nrg.2016.39
Arenas-Mena, C. (2017). The Origins of Developmental Gene Regulation. Evol. Develop. 19, 96–107. doi:10.1111/ede.12217
Bonner, J. T. (2000). First Signals: The Evolution of Multicellular Development. Princeton, NJ: Princeton University Press.
Boraas, M. E., Seale, D. B., and Boxhorn, J. E. (1998). Phagotrophy by a Flagellate Selects for Colonial Prey: A Possible Origin of Multicellularity. Evol. Ecol. 12, 153–164. doi:10.1023/a:1006527528063
Cheng, Q., Fowler, R., Tam, L.-w., Edwards, L., and Miller, S. M. (2003). The Role of GlsA in the Evolution of Asymmetric Cell Division in the green Alga Volvox Carteri. Develop. Genes Evol. 213, 328–335. doi:10.1007/s00427-003-0332-x
Coleman, A. W. (2012). A Comparative Analysis of the Volvocaceae (Chlorophyta)1. J. Phycol 48, 491–513. doi:10.1111/j.1529-8817.2012.01168.x
Coleman, A. W. (1999). Phylogenetic Analysis of "Volvocacae" for Comparative Genetic Studies. Proc. Natl. Acad. Sci. 96, 13892–13897. doi:10.1073/pnas.96.24.13892
Cross, F. R., and Umen, J. G. (2015). The Chlamydomonas Cell Cycle. Plant J. 82, 370–392. doi:10.1111/tpj.12795
Featherston, J., Arakaki, Y., Hanschen, E. R., Ferris, P. J., Michod, R. E., Olson, B. J. S. C., et al. (2017). The 4-celled Tetrabaena Socialis Nuclear Genome Reveals the Essential Components for Genetic Control of Cell Number at the Origin of Multicellularity in the Volvocine Lineage. Mol. Biol. Evol. 35 (4), 855–870. doi:10.1093/molbev/msx332
Fernández, R., and Gabaldón, T. (2020). Gene Gain and Loss across the Metazoan Tree of Life. Nat. Ecol. Evol. 4, 524–533. doi:10.1038/s41559-019-1069-x
Ferris, P., Olson, B. J. S. C., De Hoff, P. L., Douglass, S., Casero, D., Prochnik, S., et al. (2010). Evolution of an Expanded Sex-Determining Locus in Volvox. Science 328, 351–354. doi:10.1126/science.1186222
Fisher, R. M., Bell, T., and West, S. A. (2016). Multicellular Group Formation in Response to Predators in the Alga Chlorella Vulgaris. J. Evol. Biol. 29, 551–559. doi:10.1111/jeb.12804
Go, Y., Satta, Y., Takenaka, O., and Takahata, N. (2005). Lineage-Specific Loss of Function of Bitter Taste Receptor Genes in Humans and Nonhuman PrimatesSequence Data from This Article Have Been Deposited with the EMBL/GenBank Data Libraries under Accession Nos. AB198983, AB199308. Genetics 170, 313–326. doi:10.1534/genetics.104.037523
Grochau-Wright, Z. I., Hanschen, E. R., Ferris, P. J., Hamaji, T., Nozaki, H., Olson, B. J. S. C., et al. (2017). Genetic Basis for Soma Is Present in Undifferentiated Volvocine green Algae. J. Evol. Biol. 30, 1205–1218. doi:10.1111/jeb.13100
Grosberg, R. K., and Strathmann, R. R. (2007). The Evolution of Multicellularity: A Minor Major Transition? Annu. Rev. Ecol. Evol. Syst. 38, 621–654. doi:10.1146/annurev.ecolsys.36.102403.114735
Guijarro-Clarke, C., Holland, P. W. H., and Paps, J. (2020). Widespread Patterns of Gene Loss in the Evolution of the Animal Kingdom. Nat. Ecol. Evol. 4, 519–523. doi:10.1038/s41559-020-1129-2
Hallmann, A. (2006). Morphogenesis in the Family Volvocaceae: Different Tactics for Turning an Embryo Right-Side Out. Protist 157, 445–461. doi:10.1016/j.protis.2006.05.010
Hamaji, T., Kawai-Toyooka, H., Uchimura, H., Suzuki, M., Noguchi, H., Minakuchi, Y., et al. (2018). Anisogamy Evolved with a Reduced Sex-Determining Region in Volvocine green Algae. Commun. Biol. 1, 17. doi:10.1038/s42003-018-0019-5
Hanschen, E. R., Ferris, P. J., and Michod, R. E. (2014). Early Evolution of the Genetic Basis for Soma in the Volvocaceae. Evolution 68, 2014–2025. doi:10.1111/evo.12416
Hanschen, E. R., Herron, M. D., Wiens, J. J., Nozaki, H., and Michod, R. E. (2018a). Multicellularity Drives the Evolution of Sexual Traits. The Am. Naturalist 192, E93–E105. doi:10.1086/698301
Hanschen, E. R., Herron, M. D., Wiens, J. J., Nozaki, H., and Michod, R. E. (2018b). Repeated Evolution and Reversibility of Self‐fertilization in the Volvocine green Algae*. Evolution 72, 386–398. doi:10.1111/evo.13394
Hanschen, E. R., Marriage, T. N., Ferris, P. J., Hamaji, T., Toyoda, A., Fujiyama, A., et al. (2016). The Gonium Pectorale Genome Demonstrates Co-option of Cell Cycle Regulation during the Evolution of Multicellularity. Nat. Commun. 7, 11370. doi:10.1038/ncomms11370
Herron, M. D., Borin, J. M., Boswell, J. C., Walker, J., Chen, I.-C. K., Knox, C. A., et al. (2019). De Novo origins of Multicellularity in Response to Predation. Sci. Rep. 9, 2328. doi:10.1038/s41598-019-39558-8
Herron, M. D., Hackett, J. D., Aylward, F. O., and Michod, R. E. (2009). Triassic Origin and Early Radiation of Multicellular Volvocine Algae. Proc. Natl. Acad. Sci. 106, 3254–3258. doi:10.1073/pnas.0811205106
Hiraide, R., Kawai-Toyooka, H., Hamaji, T., Matsuzaki, R., Kawafune, K., Abe, J., et al. (2013). The Evolution of Male-Female Sexual Dimorphism Predates the Gender-Based Divergence of the Mating Locus Gene MAT3/RB. Mol. Biol. Evol. 30, 1038–1040. doi:10.1093/molbev/mst018
Holmes, J. A., and Dutcher, S. K. (1989). Cellular Asymmetry in Chlamydomonas Reinhardtii. J. Cel Sci 94 (Pt 2), 273–285. doi:10.1242/jcs.94.2.273
Hoops, H. J. (1993). Flagellar, Cellular and Organismal Polarity in Volvox Carteri. J. Cel Sci. 104, 105–117. doi:10.1242/jcs.104.1.105
Hoops, H. J., Nishii, I., and Kirk, D. L. (2006). “Cytoplasmic Bridges in Volvox and its Relatives,” in Cell-Cell Channels (New York, NYNew York: Springer), 65–84.
I. Ruiz-Trillo, and A.M. Nedelcu (Editors) (2015). Evolutionary Transitions to Multicellular Life (Dordrecht: Springer Netherlands).
Jiménez-Marín, B., Rakijas, J. B., Tyagi, A., Pandey, A., Hanschen, E. R., Anderson, J., et al. (2021). Gene Loss during the Transition to Multicellularity. Biorxiv 2021.02.16.431445. doi:10.1101/2021.02.16.431445
John Maynard Smith, E. S. (1995). The Major Transitions in Evolution. New York: Oxford University Press.
Kirk, D. L. (2005). A Twelve-step Program for Evolving Multicellularity and a Division of Labor. Bioessays 27, 299–310. doi:10.1002/bies.20197
Knoll, A. H. (2011). The Multiple Origins of Complex Multicellularity. Annu. Rev. Earth Planet. Sci. 39, 217–239. doi:10.1146/annurev.earth.031208.100209
Kvitek, D. J., and Sherlock, G. (2013). Whole Genome, Whole Population Sequencing Reveals that Loss of Signaling Networks Is the Major Adaptive Strategy in a Constant Environment. Plos Genet. 9, e1003972. doi:10.1371/journal.pgen.1003972
Lerche, K., and Hallmann, A. (2013). Stable Nuclear Transformation of Eudorina Elegans. BMC Biotechnol. 13, 11. doi:10.1186/1472-6750-13-11
Lerche, K., and Hallmann, A. (2009). Stable Nuclear Transformation of Gonium Pectorale. BMC Biotechnol. 9, 64. doi:10.1186/1472-6750-9-64
Lindsey, C. R., Rosenzweig, F., and Herron, M. D. (2021). Phylotranscriptomics Points to Multiple Independent Origins of Multicellularity and Cellular Differentiation in the Volvocine Algae. BMC Biol. 19, 182. doi:10.1186/s12915-021-01087-0
Matt, G. Y., and Umen, J. G. (2018). Cell-Type Transcriptomes of the Multicellular Green Alga Volvox Carteri Yield Insights into the Evolutionary Origins of Germ and Somatic Differentiation Programs. G3 (Bethesda) 8, 531–550. doi:10.1534/g3.117.300253
Mikhailov, K. V., Konstantinova, A. V., Nikitin, M. A., Troshin, P. V., Rusin, L. Y., Lyubetsky, V. A., et al. (2009). The Origin of Metazoa: a Transition from Temporal to Spatial Cell Differentiation. Bioessays 31, 758–768. doi:10.1002/bies.200800214
Miller, D. J., and Technau, U. (2010). Understanding the Evolution of Multicellularity: Insights from Basal Metazoans. BioEssays 32 (2), 175–178. doi:10.1002/bies.200900168
Nanjundiah, V., Ruiz-Trillo, I., and Kirk, D. (2018). “Protists and Multiple Routes to the Evolution of Multicellularity,” in Cells in Evolutionary Biology. Editors B. Hall, and S. Moody (London: CRC Press). doi:10.1201/9781315155968-4
Nedelcu, A. M., and Michod, R. E. (2003). "Cooperation and Conflict during the Unicellular-Multicellular and Prokaryotic-Eukaryotic Transitions," in Evolution: From Molecules to Ecosystems. Oxford: Oxford Univ. Press, 195–208.
Niklas, K. J. (2014). The Evolutionary-Developmental Origins of Multicellularity. Am. J. Bot. 101, 6–25. doi:10.3732/ajb.1300314
Nishii, I., Ogihara, S., and Kirk, D. L. (2003). A Kinesin, invA, Plays an Essential Role in Volvox Morphogenesis. Cell 113, 743–753. doi:10.1016/s0092-8674(03)00431-8
Nozaki, H., Misumi, O., and Kuroiwa, T. (2003). Phylogeny of the Quadriflagellate Volvocales (Chlorophyceae) Based on Chloroplast Multigene Sequences. Mol. Phylogenet. Evol. 29, 58–66. doi:10.1016/s1055-7903(03)00089-7
Olson, B. J., and Nedelcu, A. M. (2016). Co-option during the Evolution of Multicellular and Developmental Complexity in the Volvocine green Algae. Curr. Opin. Genet. Develop. 39, 107–115. doi:10.1016/j.gde.2016.06.003
Prochnik, S. E., Umen, J., Nedelcu, A. M., Hallmann, A., Miller, S. M., Nishii, I., et al. (2010). Genomic Analysis of Organismal Complexity in the Multicellular green Alga Volvox Carteri. Science 329, 223–226. doi:10.1126/science.1188800
Ratcliff, W. C., Herron, M. D., Howell, K., Pentz, J. T., Rosenzweig, F., and Travisano, M. (2013). Experimental Evolution of an Alternating Uni- and Multicellular Life Cycle in Chlamydomonas Reinhardtii. Nat. Commun. 4, 2742. doi:10.1038/ncomms3742
Rokas, A. (2008). The Origins of Multicellularity and the Early History of the Genetic Toolkit for Animal Development. Annu. Rev. Genet. 42, 235–251. doi:10.1146/annurev.genet.42.110807.091513
Sathe, S., and Durand, P. M. (2015). Cellular Aggregation inChlamydomonas(Chlorophyceae) Is Chimaeric and Depends on Traits like Cell Size and Motility. Eur. J. Phycology 51, 129–138. doi:10.1080/09670262.2015.1107759
Sumper, M., and Hallmann, A. (1998). Biochemistry of the Extracellular Matrix of Volvox. Int. Rev. Cytol. 180, 51–85. doi:10.1016/s0074-7696(08)61770-2
Trigos, A. S., Pearson, R. B., Papenfuss, A. T., and Goode, D. L. (2018). How the Evolution of Multicellularity Set the Stage for Cancer. Br. J. Cancer 118, 145–152. doi:10.1038/bjc.2017.398
Umen, J. G., and Olson, B. J. S. C. (2012). Genomics of Volvocine Algae. Adv. Bot. Res. 64, 185–243. doi:10.1016/b978-0-12-391499-6.00006-2
Keywords: multicellularity, green algae, volvocine algae, co-option, gene loss, developmental complexity, multicellular evolution
Citation: Jiménez-Marín B and Olson BJSC (2022) The Curious Case of Multicellularity in the Volvocine Algae. Front. Genet. 13:787665. doi: 10.3389/fgene.2022.787665
Received: 01 October 2021; Accepted: 20 January 2022;
Published: 15 February 2022.
Edited by:
Krishnaveni Mishra, University of Hyderabad, IndiaReviewed by:
Vidyanand Nanjundiah, Centre for Human Genetics (CHG), IndiaCopyright © 2022 Jiménez-Marín and Olson. This is an open-access article distributed under the terms of the Creative Commons Attribution License (CC BY). The use, distribution or reproduction in other forums is permitted, provided the original author(s) and the copyright owner(s) are credited and that the original publication in this journal is cited, in accordance with accepted academic practice. No use, distribution or reproduction is permitted which does not comply with these terms.
*Correspondence: Bradley J. S. C. Olson, YmpzY29Aa3N1LmVkdQ==
Disclaimer: All claims expressed in this article are solely those of the authors and do not necessarily represent those of their affiliated organizations, or those of the publisher, the editors and the reviewers. Any product that may be evaluated in this article or claim that may be made by its manufacturer is not guaranteed or endorsed by the publisher.
Research integrity at Frontiers
Learn more about the work of our research integrity team to safeguard the quality of each article we publish.