- Department of Obstetrics and Gynecology, Second Hospital of Jilin University, Changchun, China
Despite advances in cervical cancer screening and human papilloma virus (HPV) vaccines, cervical cancer remains a global health burden. The standard treatment of cervical cancer includes surgery, radiation therapy, and chemotherapy. Radiotherapy (RT) is the primary treatment for advanced-stage disease. However, due to radioresistance, most patients in the advanced stage have an adverse outcome. Recent studies have shown that long noncoding RNAs (lncRNAs) participate in the regulation of cancer radiosensitivity by regulating DNA damage repair, apoptosis, cancer stem cells (CSCs), and epithelial–mesenchymal transition (EMT). In this review, we summarize the molecular mechanisms of long noncoding RNAs in cervical cancer and radiosensitivity, hoping to provide a theoretical basis and a new molecular target for the cervical cancer RT in the clinic.
Introduction
Cervical cancer is the fourth most common female lower genital tract malignancy, and over 570000 women are diagnosed with cervical cancer each year (Ferlay et al., 2019). The staging system of the International Federation of Gynecology and Obstetrics (FIGO) determines the clinical decision of cervical cancer. Patients with cervical cancer in its early stages (stages I, II) usually undergo surgical treatment, and those who have risk factors will receive adjuvant radiotherapy (RT). Patients with advanced cervical cancer (stage III, IV) or patients with severe complications or surgical contraindications will receive RT as the main treatment (Meng et al., 2018). Approximately three-fifths of all cervical cancer patients undergo RT (Chung et al., 2005). RT has greatly improved therapeutic effectiveness in cervical cancer. However, in the clinic, some patients experience radioresistance, and those patients often suffer an unfavorable treatment outcome. Hence, enhancing the radiosensitivity of cervical cancer cells is important for patients. Researchers have looked for various strategies to solve this urgent problem. In recent years, researchers have found that long noncoding RNAs (lncRNAs) have a crucial function in regulating cervical cancer radioresistance.
LncRNAs are a class of noncoding RNAs that are longer than 200 nucleotides and have a complex function in regulating biological processes, including cell proliferation, migration, invasion, the cell cycle and apoptosis (Esteller, 2011). Recent studies suggest that lncRNAs interact with DNA, RNA, and protein, thus participating in a variety of cellular processes, including transcription, translation, and posttranscriptional regulation (Huarte et al., 2010). LncRNAs can affect gene expression epigenetically via diverse mechanisms: 1) lncRNAs can regulate histone modification and chromatin status to affect gene transcription. 2) lncRNAs can attract transcription factors or repressors to the promoter of a particular gene (Long et al., 2017), 3) lncRNAs can prevent transcription-related proteins from binding to their DNA targets by acting as decoys. LncRNAs can compete with endogenous RNAs to silence target genes by binding to miRNAs and blocking their function (Gutschner and Diederichs, 2012). Many studies have demonstrated that there is an association between lncRNAs and radiosensitivity of malignant tumors (Yang Q. et al., 2019; Tang T. et al., 2019; Yang Z. et al., 2019; Liu R. et al., 2020; Wang et al., 2020). However, there is no summary of the systematic and comprehensive molecular mechanisms of long noncoding RNAs associated with cervical cancer radiosensitivity. Hence, we performed a systematic literature review on lncRNAs and the detailed mechanisms by which they enhance or weaken the radiosensitivity of cervical cancer, and we discuss these topics in seven sections.
Radiation causes DNA damage, inhibits cell proliferation, and induces cellular apoptosis to treat cancer (Yao et al., 2019). The conventional radiobiological principles proposed by Withers are known as the “4Rs”, repair of sublethal cellular damage, reoxygenation of cells within the cell cycle, redistribution of the surviving cells and repopulation of cells after radiation (Withers, 1975). Radiotherapy induces tumour cell death by causing cell cycle arrest and leading to DNA damage. Repair of sublethal cellular damage and repopulation of cells after radiation make tumor cells resistant to radiation and decrease radiosensitivity; on the other hand, reoxygenation of cells within the cell cycle and redistribution of the surviving cells decrease the radiosensitivity of tumor cells (Brown et al., 2014).
Numerous studies have shown that lncRNAs are functionally involved in various critical cellular processes that regulate RT in cervical cancer. LncRNAs can modulate DNA damage repair, the cell cycle, and apoptosis and can also influence the redistribution of surviving cells by regulating EMT, cancer stem cells (CSCs) and the reoxygenation of cells by regulating aerobic glycolysis after RT in cervical cancer (Figure 1).
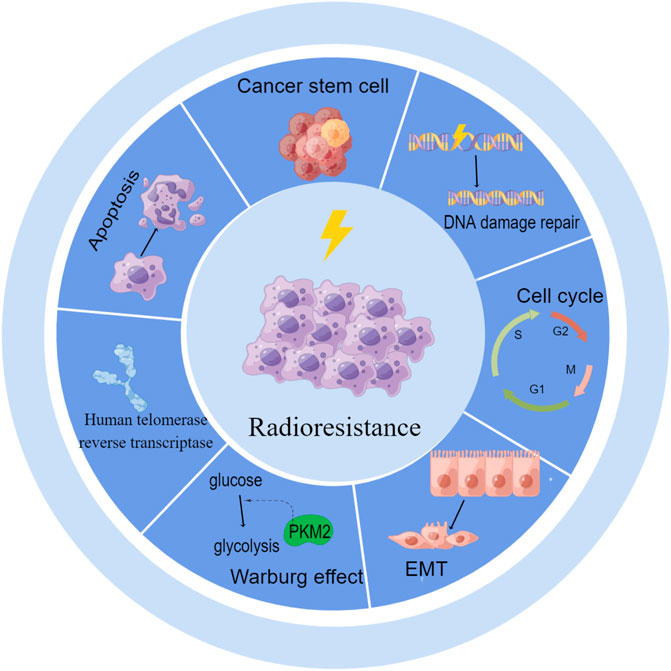
FIGURE 1. LncRNAs regulate radioresistance by DNA damage repair, cell cycle, apoptosis, EMT, CSCs, aerobic glycolysis and human telomerase reverse transcriptase.
LncRNAs modulate DNA damage repair and the cell cycle
Radiation-induced DNA damage includes base damage, single- and double-strand breaks, and DNA crosslinks caused by direct ionization or free oxygen radical effects (Olive, 1998), DNA double-strand breaks (DSBs) are the most critical. When DNA damage occurs, the cell cycle checkpoint is active, causing a delay in the cell cycle, and providing time to repair DNA damage. Finally, damage that cannot be repaired will induce cancer cell apoptosis. Different DNA repair pathways initiate and repair different types of DNA damage. However, there is also functional overlap between different DNA repair pathways (Mao and Wyrick, 2016). Among these repairs are nonhomologous end joining (NHEJ) repair, homologous recombination (HR) nucleotide excision repair, mismatch repair, and base excision repair (BER) (Sancar, 1995; Hoeijmakers, 2001; Schärer, 2003; Chapman et al., 2012; Krokan and Bjørås, 2013; Mao and Wyrick, 2016). In mammalian cells, ataxia telangiectasia-mutated (ATM) and ATM and Rad-3 related (ATR) are two phosphatidylinositol kinases that are involved in these pathways (Bakkenist and Kastan, 2004). DNA damage and DNA repair pathways are crucial determinants of radiosensitivity.
In mammalian cells, ATM and ATR are two phosphatidylinositol kinases that are involved in DNA damage repair (Bakkenist and Kastan, 2004). When DNA damage occurs, the MRE11-RAD50-NBS1 (MRN) complex first detects damaged DNA and recruits ATM to damage sites (Lee and Paull, 2004). Then ATM is activated by autophosphorylation at Ser 1981 (Bakkenist and Kastan, 2003) and acetylation at K3106 (Sun et al., 2005). Activated ATM can also activate p53-dependent and independent pathways to initiate the downstream protein kinase phosphorylation cascade (Shiloh and Ziv, 2013). In p53-independent pathways, activated ATM phosphorylates downstream targets Chk2 at Thr68 and leading to phosphorylation of Cdc25 at Ser 216. Phosphorylated Cdc25 bind to 14-3-3 proteins and sequestrate Cdc25 in cytoplasm inhibiting activation of cyclin B/Cdk1 by Cdc25 and resulting in G2/M arrest. In p53 dependent pathways, the p53 protein activates downstream genes such as p21 which can inhibit CDK2 resulting in G1 arrest, and 14-3-3, which can inhibit cyclin B/Cdk1, resulting in G2/M arrest. Teng et al. (Teng et al., 2015) revealed that after IR-induced DNA damage, ATM activation activates ATR, and then Chk1 is phosphorylated at Ser345. ATR-Chk1 restricts CDK2 activity and finally cause G1 arrest. Through the above steps, Cell cycle arrest provide more time for DNA damage repair. This will cause radioresistance. Therefore, the use of checkpoint inhibitors to improve the therapeutic effects of radiotherapy is a clinical treatment strategy in the future.
ATM has been proved to be connected with therapeutic effects of radiotherapy in cervical cancer. Roossink et al. (2012) recruited 349 advanced stage cervical cancer patients to detect the expression of p-ATM in their pretreatment tissue using immunohistochemical analysis and results revealed that 344 patients (98.6%) had positive nuclear staining and 183 patients (52.4%) had high expression. By analyzing clinical data, they found that high p-ATM expression was related to poor survival. To determine the relationship between p-ATM and the response of cervical cancer to irradiation, they carried out in vitro studies and found that the expression of activated ATM was high in Caski cells even before irradiation while in HeLa and SiHa cells was extremely low. And in comparison to HeLa and SiHa cells, Caski cells were significantly more resistant to irradiation. This result reveals us there is a positive correlation between baseline level of p-ATM and radioresistance. Then they proved ATM inhibition led to the destroy of G2/M arrest and finally sensitized tumour cells to radiation. The same result was also reported by. Teng et al. (2015) They use inhibitors of ATR (ETP-46464) (Hickson et al., 2004; Toledo et al., 2011) and ATM (KU55933) to treat Hela and SiHa cervical cancer cells for 15 min before IR exposure and then assessed clonogenic survival. Both ETP-46464 and KU55933 could enhance the response to IR by affecting the expression levels of p-ATM/P-Chk1 and p-ATM/P-Chk2. A compound named Ro 90-7501 can enhance the adiosensitivity of cervical cancer by inhibiting ATM (Tamari et al., 2019), A Chinese herbal medicine Osthole can also inhibited phosphorylation of ATM and enhances irradiation sensitivity of cervical cancer cells (Che et al., 2018).
Many studies showing that lncRNAs can directly act on DNA damage repair-related protein to regulate DNA damage response. We retrieved related literature and found that lncRNAs can regulate the expression of ATM in various tumors, including cervical cancer (Sharma et al., 2020), esophageal squamous cell cancer (Chen et al., 2018; Zhang et al., 2019), pancreatic cancer (Chen et al., 2017), and thyroid cancer (Yuan et al., 2020). Zhao et al. (2020) identified an lncRNA named HITT that maps to 14q32, and contains three exons. LncRNA HITT inhibits ATM activity by affecting MRN. ATM inhibitors may serve as an attractive target to overcome radioresistance in cervical cancer (Figure 2).
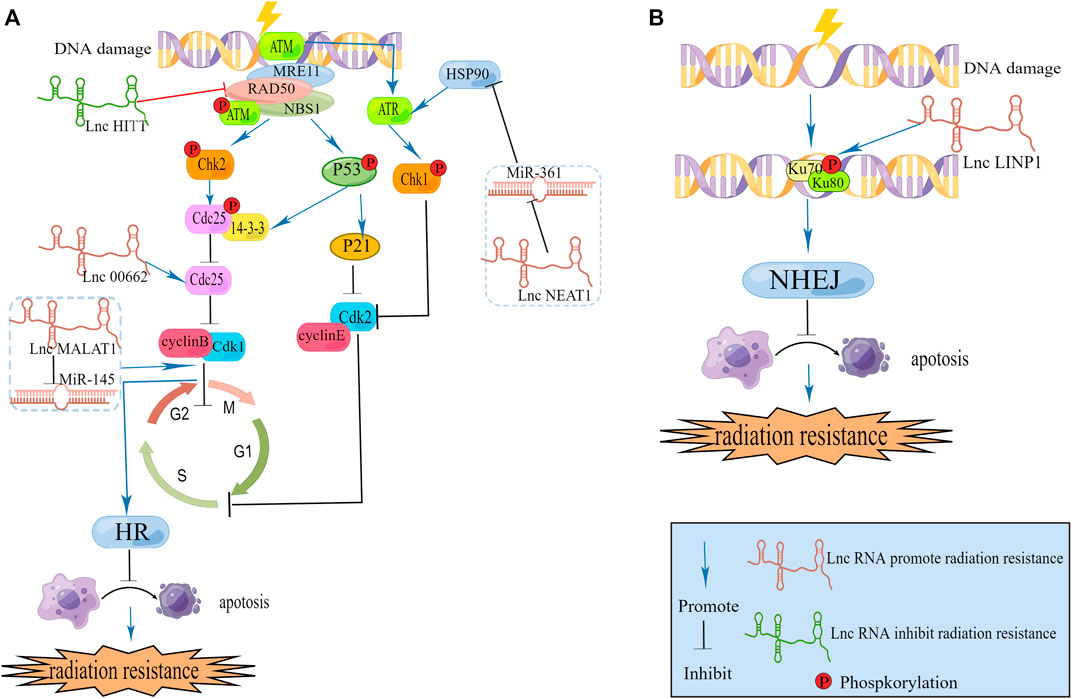
FIGURE 2. (A) LncRNAs modulate DNA damage repair caused by HR and cell cycle. (B) LncRNA modulate DNA damage repair caused by NHEJ.
Kyungsoo Ha c (Ha et al., 2011) found in cervical cancer that ATR, which is a bona fide heat shock protein (hsp) 90 client protein, is downregulated when hsp90 is inhibited. Additionally, they found that treatment with hsp90 inhibitors decreased both ATR and CHK1 levels, and the hsp90 inhibitor increased radiation-induced DNA damage as well as apoptosis of transformed cells by blocking ATR-CHK1-mediated DNA damage repair signaling. Xu et al. (2020) carried out a luciferase reporter assay and demonstrated that in cervical cancer, miR-361 reduces the expression of hsp90 directly, and lncRNA NEAT1. Directly downregulates the expression of miR-361. From the two above studies, we can establish a link between the lncRNAs NEAT1, hsp90, ATR and radiation-mediated DNA damage repair. We infer that lncRNA NEAT1 could increase the expression of hsp90 and ATR, DNA damage repair, causing radioresistance. Han et al. (2018) have already revealed that NEAT1 can facilitate radioresistance in cervical cancer. They use the luciferase reporter analysis and RNA immunoprecipitation (RIP) assay to prove lncRNA NEAT1 can spong miR-193b-3p as a ceRNA to regulate cyclin D1. More experiments are needed to investigate this hypothesis.
As we mentioned above CDC25 is required for DNA damage repair. LINC00662 is reported to be connected with CDC25A in cervical cancer. Wei et al. (2020) collected 39 samples from patients (cancer samples and adjacent cervical samples) with cervical cancer and found that the expression of LINC00662 was markedly elevated in cervical cancer tissues in comparison with adjacent tissues. They subsequently used two CC cell lines (C33A and Caski) to investigate the function of LINC00662. According to their findings, LINC00662 facilitated cervical cancer progression and radioresistance via miR-497-5p adsorption and upregulation of CDC25A. However they did not compare LINC00662 expression between different patients’ tumor tissues and collected clinical information to assess the therapeutic effects of radiotherapy in different expression level. Follow-up researchers can supplement the above points if they carry out experiments in this area, which can significantly improve the quality of the article.
LncRNA in nonhomologous end joining pathway 1 (LINP1) is located on chromosome 10p14 and is associated with the NHEJ repair pathway (Thapar et al., 2020). The NHEJ repair pathway is a major DNA damage repair pathway involved in the repair of DSBs in DNA that are damaged after radiotherapy (Lord and Ashworth, 2012). Wu et al. (2020) collected twenty paired cervical cancer tissues and adjacent normal tissues from Guizhou Provincial People’s Hospital (Guizhou, China) to examine each tissue’s LINP1 expression level and revealed that CC tissues exhibit higher expression of LINP1 than adjacent noncarcinoma tissues and that high expression of LINP1 can suppress KLF2 and PRSS8 to aggravatecervical cancer development. Similarly, Wang et al. (2018) isolated total RNA from five tissues of multiple patients and then carried out qRT‒PCR experiments. In comparison to adjacent tissues, tumor tissues from cervical cancer patients contained higher levels of LINP1. They found that within 30 min of RT, LINP1 translocates into the nucleus, and the protein was upregulated later. We know that RT can cause DNA DSBs and then Ku70–Ku80 heterodimers are recruited and activated by NHEJ. Additionally, by promoting NHEJ protein recruitment to DSBs, Ku80 can bind breaks in DNA. One of the Ku-interacting proteins called DNA-PKCs is a serine/threonine-protein kinase required for NHEJ in human cells; Wang et al. (2018) revealed that LINP1 associates with Ku80 and DNA-PKCs in cervical cancer cell lines. Moreover, LINP1-deficient cells were more prone to radiation-induced cell death and DNA DSBs after RT than control cells. Together, these data indicated that in cervical cancer, the lncRNA LINP1 contributes to radiation resistance by increasing the efficiency of DNA damage repair through the NHEJ pathway (Figure 2).
DNA damage induced by radiation induces DNA repair pathways and alters the expression of cell cycle checkpoint molecules. Affected cells are able to arrest cell cycle progression and repair damaged DNA or undergo apoptosis if the damage cannot be repaired. Radiosensitivity is closely associated with cell cycle arrest, since different phases of the cell cycle exhibit different levels of radiosensitivity, and the G2/M phase is the most radiosensitive phase of the cell cycle (Wilson, 2004). LncRNAs regulate radiosensitivity in cervical cancer by arresting cell cycle growth to interfere with DNA damage repair. G0/G1 phase Han et al. (2018) found that in cervical cancer, the absence of NEAT1 leads to cell cycle arrest in G0/G1, and this change allows cells to undergo apoptosis. This is because NEAT1 binds to miR-193b in a competitive manner to regulate the expression of CCND1, enhancing radioresistance in cervical cancer. In another study, Jiang et al. (2014) investigated lncRNA MALAT1 in cervical cancer and found that MALAT1 downregulation causes cells to enter the G1 phase and that cyclin D1, cyclin E, and CDK6 levels are significantly altered. Other researchers Lu et al. (2016) reported that lncRNA MALAT1 can regulate the cell cycle to influence radiosensitivity. They revealed that lncRNA MALAT1 was negatively correlated with miR-145, which can enhance G2/M phase block. These two studies provide evidence that the function of MALAT1 in cervical cancer is related to the cell cycle. In the future, we can investigate more lncRNAs that are connected with the cell cycle to learn their roles in regulating radiosensitivity in cervical cancer.
LncRNAs modulate apoptosis
Among the mechanisms of irradiation-induced toxicity, apoptosis plays a significant role. Tumor cells that undergo apoptosis display a number of morphological features, including condensed chromatin, shrinkage and fragmentation, blebbing of the plasma membrane, and the formation of apoptotic bodies (Wyllie, 2010). Extrinsic death receptor signals and intrinsic mitochondrial signals are the two main inducers of apoptosis.
To determine the changes in signaling pathways in cervical cancer cells after radiation, (Khalilia et al., 2018) exposed cervical cancer cells to different doses of radiation and revealed that apoptosis, RAS, TGF-β, WNT, the oxidative stress response, and p53 were significant downstream signals. Among these pathways, the p53 pathway is considered to be the most crucial regulator. Accumulating evidence indicates that the p53 pathway participates in proliferation and apoptosis to regulate radiosensitivity in various cancers (Concin et al., 2000; Oei et al., 2015; Granados-López et al., 2021). In p53 dependent apoptosis, p53 promotes apoptosis by activating the transcription of apoptotic factors including Bax, PUMA, Noxa at the same time it can inhibit the transcription of anti-apoptotic factors such as Bcl-2, Bcl-xL, Survivin. On the other hand, p53 can accumulate in the cytoplasm and directly connect to the mitochondria, leading to changes in the permeability of the mitochondrial membrane.
By interacting with p53 pathway factors, LncRNAs regulate radiosensitivity. Under hypoxic conditions, p53 participates in the degradation of HIF-1α (Ravi et al., 2000). Fu et al. (2015) found that HIF-1α increased radiation resistance in cervical cancer cells by inhibiting the expression of p53 and increasing the expression of VEGF. Li N. et al. (2018) found that radiation reduced lncRNA levels and HIF-1α levels in mice bearing HeLa cells as well as in HeLa cells and C33A cells, and they carried out a series of experiments and finally revealed that overexpression of lncRNA HOTAIR induces radiation resistance by increasing the expression of HIF-1α in cervical cancer cells. The lncRNA DINO is a p53 transcriptional target and functional modulator (Sharma et al., 2020). LncRNA DINO can bind to TP53 to stabilize and upregulate transcriptional target genes of TP53. The lncRNA DINO has been found to be a potent tumor suppressor in specific subsets of human and mouse tissues (Marney et al., 2022). However, the relationship between DINO and radiation sensitivity has not been revealed. From previous research, we infer that DINO may have a positive effect on radiosensitivity.
LncRNA GAS5 is reported to connected with cancer cell apoptotic to affect radiosensitivity. Gao et al. (2019) collected twenty cervical cancer patient biopsy tumor samples before RT. These patients had never received any chemotherapy before radiation therapy. The twenty patients were divided into two groups: 1) radioresistant (9 cases) and 2) radiosensitive (11 cases). Researchers compared the expression of related molecules between the two groups. And found lncRNA GAS5 and miR-106b expression was significantly different between radioresistant tissues and radiosensitive tissues. Then, they used SiHa and ME180 cervical cancer cells to investigate the biological role of GAS5 in the radiosensitivity of cervical cancer. They found that upregulation of GAS5 enhanced radiosensitivity in SiHa cells, while downregulation of GAS5 decreased radiosensitivity in ME180 cells. GAS5 acted as a miR-106b sponge, inhibiting miR-106b and promote IER3 expression increasing cervical cancer cell radiosensitivity both in vitro and in vivo. IER3 is necessary for cervical cancer cell apoptotic activity by inhibiting the expression of BCL-2 and BCL-xL (Yoon et al., 2009). Fang et al. (2020) illustrated the relationship between GAS5 expression and the overall survival (OS) of cervical cancer patients using Kaplan–Meier analysis. They confirmed that poor OS was associated with low GAS5 expression. Univariate analysis indicated that GAS5 could be an independent prognostic factor for patients with CC. These above studies indicate that lncRNA GAS5 may become an important topic for cervical cancer clinical research, and researchers could detect these lncRNAs to infer the effect of RT in cervical cancer patients.
A majority of miRNAs play key roles in regulating radiation-induced apoptosis. In various cancers, lncRNAs function as miRNA sponges to regulate target gene expression. In recent studies, lncRNAs were reported to regulate radiosensitivity in cervical cancer cells by working as miRNA sponges. For example, in cervical cancer tissues and cells, the lncRNA SNHG6, which is a small nucleolar RNA host gene (SNHG family), was upregulated, knockdown of SNHG6 can improve the radiosensitivity of cervical cancer cells by promoting radiation-induced apoptosis. SNHG6 can directly sponge miR-485-3p.
Researchers have mentioned that in cervical cancer, miR-21 could facilitate cell proliferation by downregulating the expression of programmed cell death 4 (PDCD4) (Yao et al., 2009) as well as in breast cancer. MiR-21 inhibits the expression of p53-regulated genes. In various tumors, including non-small cell lung cancer (Song et al., 2017), and esophageal squamous cell carcinoma (ESCC) (Li F. et al., 2018; Lin et al., 2020), miR-21 has been shown to be related to radiation sensitivity. In cervical cancer, Zhang L et al. (2020) found that lncRNA MEG3 was negatively related to miR-21 to affect cell proliferation and apoptosis. These studies revealed that lncRNA MEG3 may also sensitize cervical cancer cells to radiation treatment by affecting the expression of miR-21, and this hypothesis needs to be proven. There are also many miRNAs that can interact with lncRNAs to affect the radiation effect in cervical cancer.
Above all, lncRNAs are believed to affect cervical cancer cell radiosensitivity through their ability to induce apoptosis. Several mechanisms can be employed to achieve this effect, including affecting signaling pathways such as p53 or miRNA sponges that manipulate the expression of target genes (Figure 3). Hence, we can identify the difference between apoptotic and nonapoptotic cervical cancer cells by detecting noncoding RNAs in the future. Their relationship with RT sensitivity was studied, and a corresponding database was created for further exploration of the relationship between these noncoding RNAs and apoptotic signaling and to identify genes that can regulate the expression of these noncoding RNAs and therefore achieve RT sensitivity regulation.
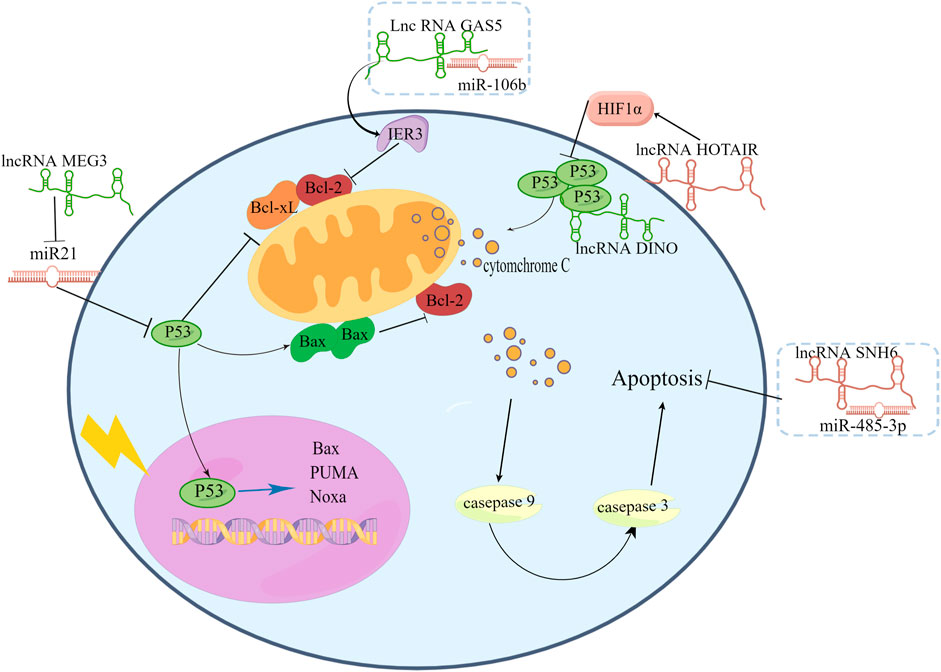
FIGURE 3. LncRNAs affect signaling pathways such as p53 or miRNA sponges to affect cervical cancer cell radiosensitivity.
LncRNAs modulate EMT and CSCs
EMT plays an important role during tumor development. Tumor cells acquire an invasive phenotype when EMT programs aberrant activation. When tumor cells accept radiation, they may acquire stemness and oncogenic metabolism by inducing EMT (Lee et al., 2017). In tumors, there are cells that can self-renewing called CSCs. CSCs are similar to multipotent embryonic stem cells and possess self-renew capabilities (Reya et al., 2001). . Under specific conditions, CSCs and non-CSCs can convert to each other. CSCs play a crucial role in tumorigenesis and metastasis (Ponti et al., 2005). Researchers have shown that CSCs are generated by EMT (Mani et al., 2008). CSCs existence in cervical cancer with CD44+CK17+/sphere-forming and express stemness-related genes (Oct-4, Sox2 and so on) (Feng et al., 2009). In cervical cancer CSCs, EMT marker vimentin is high expression and perform a higher level of radioresistance than normal cervical cancers (López et al., 2012). And in HeLa, high expression of EMT marker Twist can induce EMT and elevate the expression of CD44 which is cancer stem marker of CSCs (Li and Zhou, 2011). These data indicate that there is a tight link between EMT and CSCs.
In cervical cancer, lncRNAs can regulate EMT-related gene and transcription factors (Figure 4). For example, lncRNA NEAT1 can downregulate miR-361, which can inhibit EMT process in cervical cancer cells by targeting on EMT activator HSP90 (Xu et al., 2020). LncRNA ZEB1-AS1, SPRY4-IT1, MALAT1 and TUG1 are high-expression in cervical cancer cells and associated with poor prognosis. Overexpression of ZEB1-AS1 can increase the level of N-cadherin and vimentin and increase EMT promoter ZEB1 to facilitate EMT process (Cheng et al., 2018). Lnc RNA SPRY4-IT1 and HOTAIR can also target on ZEB1 to promote EMT process. SPRY4-IT1 can bind to miR-101-3p to down regulate its target gene ZEB1 (Fan et al., 2019). HOTAIR can inhibit the expression of miR-203 which is negatively correlated with ZEB1. LncRNA MALAT1 has a positive influence on EMT transcription factor snail and promote the EMT process (Sun et al., 2016). Knockdown the expression of lncRNA TUG1 can down regulate EMT-related markers (fibronectin, vimentin, and cytokeratin) (Hu et al., 2017).
LncRNAs are involved in the EMT process of cervical cancer cells by different signaling pathways (Figure 4). LncRNA tyrosine protein kinase transmembrane receptor one antisense RNA 1 (ROR1-AS1) is high-expression in cervical cancer cells and tissues. Downregulate ROR1-AS1 in cervical cancer can inhibit EMT-related markers N-cadherin and vimentin by influencing Wnt/β-catenin signaling pathway (Zhang J et al., 2020). LncRNA-CTS is high-expression in cervical cancer tissues and can bind to miR-505 to enhance EMT process by activating the TGF/SMAD pathway (Feng S. et al., 2019). TNF-α can upregulated lncRNA LOC105374902 by promoting it binding to STAT3. LncRNA LOC105374902 promote EMT process in cervical cancer by sponging miR-1285-3p (Feng Y. et al., 2019).
Increasing evidence indicates that lncRNAs may regulate CSCs in various cancers, including liver cancer (Wang et al., 2015; Ding et al., 2016), colorectal cancer (Tang D. et al., 2019), non-small cell lung cancer (Wu and Wang, 2017). In cervical cancer lncRNA HOXA11-AS has an association of EMT/CSCs. Compared with normal tissues, LncRNA HOXA11-AS is highly expressed in cervical cancer cells and has a negative effect on overall survival. Overexpression of LncRNA HOXA11-AS promotes sphere formation, CSC markers CD133+/CD44+ and self-renewal of cervical cancer cells. Knockdown of HOXA11-AS can downregulate the EMT-related genes (β-catenin, vimentin, snail) and stemness genes (SOX2, Oct-4, Nanog). At the same time, sphere-forming capacity of cervical cancer cells is also decreased. In vivo, knockdown of HOXA11-AS can decrease tumor growth. From this study, we predict that LncRNA HOXA11-AS plays an important role in the establishment of EMT/CSCs in cervical cancer cells (Kim et al., 2016). And in gastric cancer cells overexpression of LncRNA HOXA11 can also promote the expression of cancer stem markers including CD44, CD90, CD133, and Bmi1 as well as pluripotency markers Nanog and Sox2 (Wang C et al., 2019). These data indicate LncRNA HOXA11 may be a promising therapeutic target for the treatment of cancers.
According to the cancer stem cell theory, the resistance of cancer to chemotherapy and RT is due to resident CSCs (Wang et al., 2013). Radiation treatment can directly kill the majority of tumor cells by inducing apoptosis. However, a small portion of tumor cells can endure treatment, exhibit radioresistant properties and dedifferentiate. These tumor cells transform into CSCs via EMT (Chi et al., 2017). This shift leads to radioresistance in tumor cells, and CSCs can lead to cancer recurrence and metastasis. In cervical cancer, high levels of CSCs predict poor outcomes after radiotherapy (Fu et al., 2018). Blocking irradiation-induced CSCs activation may increase radiosensitivity in cervical cancer (Prabakaran et al., 2019). LncRNA HOTAIR has been proved to be necessary for EMT and stemness maintenance of cancer cell lines (Pádua Alves et al., 2013; Łuczak et al., 2016). In breast cancer, overexpression of HOTAIR can downregulate miR-34a expression and at the same time, can promote p53 binding to the p21 promoter and enhance CSC-MCF7(enrich the CSCs subpopulation from MCF7) proliferation, colony formation and migration. Overexpression of HOTAIR can upregulate Sox2, which is the key transcription factor regulate that regulates self-renewal capacity, and epigenetic expression of miR-34a can disturb this regulation (Deng et al., 2017). In cervical cancer, the lncRNA HOTAIR is also connected with stemness acquisition. Knockdown of the expression of HOTAIR can downregulate the expression of six stem cell markers, including NANOG, Oct4, CD44, ALDH1, CD133, and vice versa (Zhang et al., 2021). Compared with normal cervical epithelial cells (NCECs), the expression level of HOTAIR was significantly upregulated in cervical cancer cells such as HeLa and C33A cells (Li N. et al., 2018). High HOTAIR expression was correlated with shorter overall survival (Kim et al., 2015). The same outcome has been reported by other researchers. Initially, they used serum samples to detect the different levels of HOTAIR between cervical cancer patients and normal women. They found that circulating HOTAIR levels were significantly higher in cervical cancer patients and connected with a poor prognosis. Then, they performed further research to investigate the biological functions of HOTAIR in cervical cancer. They used four cervical cancer cell lines to detect HOTAIR expression and carried out a series of experiments, such as MTT assays and migration and invasion assays. They finally concluded that HOTAIR acts as an oncogene in cervical cancer and then cultured primary cervical cancer cells from 25 fresh cervical cancer tissues to investigate the relationship between HOTAIR and the response to RT. They demonstrated that the expression level of HOTAIR was negatively correlated with that of P21. By hindering p21 expression in HeLa cells, HOTAIR can induce radioresistance (Jing et al., 2015). This result is consistent with the results of the above breast cancer studies, HOTAIR is also reported to be connected with radiosensitivity in colorectal cancer (Yang et al., 2016; Liu Y. et al., 2020), pancreatic cancer (Wu et al., 2018), and liver cancer (Zhai et al., 2020). Therefore, HOTAIR may be a potential target against cervical cancer.
However, very few studies have investigated the link between lncRNAs and CSCs in cancer RT, and more in vitro experimental data are needed. With suitable experimental methods, in vivo experimental data would be more convincing. It may be possible to develop new methods to improve therapeutic effects of radiotherapy in cervical cancer by gaining a deeper understanding of lncRNAs, CSCs and EMT.
LncRNAs regulate aerobic glycolysis
Malignant tumors have a high aerobic glycolysis rate, leading to high lactic acid production, and this phenomenon is called the Warburg effect. Aerobic glycolysis is necessary for cancer cell proliferation (Vander Heiden et al., 2009). Lactate is the product of aerobic glycolysis and is necessary for cancer cell migration and metastasis (San-Millán and Brooks, 2017). In previous studies, it has been found that glycolysis increases radiosensitivity (Quennet et al., 2006; Shimura et al., 2014). The enzyme pyruvate kinase isozyme type M2 (PKM2), which produces ATP, is a rate-limiting enzyme at the end of glycolysis (Wang H et al., 2019). To investigate the role of PKM2 in the RT of cervical cancer, Lin et al. (2019) collected human tissue samples from 94 patients who accepted radiation therapy. According to the response after the completion of RT, 94 patients were divided into two groups: a complete response (CR) and a noncomplete response (nCR) group. They carried out IHC staining to detect the expression of PKM2 in these two groups and found that the expression of PKM2 in the nCR group was higher than that in the CR group, and PKM2 expression was enhanced in cervical cancer cells after radiation. Then, they used HeLa and SiHa cell lines with stable low PKM2 expression to demonstrate that knocking down PKM2 can enhance the radiosensitivity of cervical cancer cells. Luan and Wang (2018) found that in cervical cancer cells, SiHa and Caski, lncRNA XLOC_006390 were related to PKM2. Puckett et al. (2021) summarized the relationship between noncoding RNAs and PKM2. All these studies revealed that lncRNAs may affect the efficiency of RT by influencing the expression of PKM2.
Like PKM2, hexokinase 2 (HK2) is another rate-limiting enzyme during aerobic glycolysis. LncRNA urothelial cancer associated 1 (UCA1) was reported to be connected with HK2 in cervical cancer. LncRNA UCA1 first discovered and researched in bladder cancer as an oncogene (Wang et al., 2008). Fan et al. (2018) explored the role of the lncRNA UCA1 in cervical cancer radioresistance. They establish irradiation-resistant (IRR) cervical cancer cell lines, SiHa–IRR and HeLa–IRR. The expression of UCA1 was significantly increased in SiHa–IRR and HeLa–IRR compared with SiHa and HeLa. To explore the function of lncRNA UCA1 in regulating the therapeutic effects of radiotherapy in cervical cancer, they carried out siRNA knockdown and plasmid overexpression assays. And they revealed overexpression of LncRNA UCA1 lead to radioresistance in cervical cancer. And in SiHa–IRR and HeLa–IRR glycolysis related proteins (HK2 and PKM, HIF-1α) were increased. Then the glycolysis inhibitor 2-dG which compete with glucose for HK2 is used in SiHa–IRR and HeLa–RR to detect the radiosensitivity. 2-dG improves the radiosensitivity of SiHa–IRR and HeLa–IRR. These findings suggest that LncRNA UCA1 affect the effects of radiotherapy by the HK2/glycolytic pathway in cervical cancer cells. The abnormal activation of glycolysis may have a role in cervical cancer radioresistance. Inhibition of glycolysis restored the radiosensitivity of irradiation-resistant cervical cancer cells. Although further research regarding the mechanism underlying the regulatory effects of lncRNAs regulating aerobic glycolysis to affect radiation is needed, existing studies have expanded the understanding of the role of aerobic glycolysis in radioresistance and provided potential novel targets to enhance radiosensitivity in cervical cancer. Targeting glycolysis may provide an improved radiotherapy for cervical cancer.
Other factors regulating RT sensitivity
Human telomerase reverse transcriptase (hTERT) is a subunit of telomerase capable of preserving telomere integrity. Cancer cells contain a highly active promoter of hTERT, whereas most normal cells contain a weak promoter (Shay and Wright, 2011). Barczak et al. (2018) noted that in head and neck cancer cell lines, inhibiting hTERT expression can induce apoptosis and result in G1/S/G2 cell cycle arrest, thus enhancing the response to RT. This study reminds researchers that hTERT may be an important target to improve the efficacy of RT in malignancy. In 2020, Li et al. (2020) reported that in cervical cancer, binding of HMGB3 to the hTERT promoter promotes the transcriptional upregulation of hTERT and promotes cell growth and radioresistance. HMGB3 is the most abundant nonhistone protein in eukaryotes (Nemeth et al., 2006). Researchers have used bioinformatics and experimental analyses of several cell lines and identified an antisense transcript upstream in the human telomerase reverse transcriptase (hTERT) promoter region named hTERT antisense promoter-associated (hTAPAS) RNA. By analyzing The Cancer Genome Atlas (TCGA), they found that the expression of hTERT was negatively correlated with that of hTAPAS (Malhotra et al., 2018). Hu et al. (2015) reported that in esophageal squamous cell carcinoma, lncRNA CDKN2B-AS1 has a positive correlation with hTERT. Therefore, we suggest that in tumor cells, special lncRNAs may affect the efficacy of RT by regulating hTERT.
Conclusion and future perspectives
Radiotherapy is a key treatment modality for cervical cancer, but the efficacy is limited by radioresistance. Emerging research has demonstrated that lncRNAs are associated with radioresistance, and the relationship between them has gradually become a research hotspot. Studies have shown that lncRNAs have potential for use as biomarkers of the response to RT and can modulate the RT response of cervical cancer.
The radiation response of cervical cancer differs greatly among different patients; thus, it is important to predict the response to radiation treatment for each patient. In addition, the overall RT treatment time for cervical cancer patients is usually several weeks. Thus, early identification of patients who are not responding to therapy would provide an opportunity to consider alternative treatment options. This would benefit individualized treatment. However, it is difficult to distinguish different lncRNAs that correlate with only the RT response between responders and nonresponders because in clinical studies, patients usually undergo combined chemoradiotherapy. Fortunately, a limited number of recent cervical cancer studies have shown that lncRNAs indeed exhibit differences in the setting of RT alone. At present, there are few studies on the ability to predict the RT effect of cervical cancer based on lncRNAs, but this will be a hot topic that requires large-scale clinical research.
Studies have focused on the effect of the expression level of lncRNAs on the radiosensitivity of cervical cancer via basic experiments. However, the molecular mechanisms underlying radioresistance have not yet been completely elucidated. In this review, we summarized the potential mechanisms of lncRNAs that are associated with radiosensitivity in cervical cancer (Table 1). The role of different lncRNAs in the RT sensitivity of different malignant tumors could be twofold. Some can enhance radiosensitivity, while others may enhance radioresistance. Therefore, it is of great significance to identify abnormally expressed lncRNAs in cervical cancer and to investigate their function and potential related signaling pathways in the response to RT. In this way, researchers can develop more effective radiotherapies and institute individualized treatment.
The study of lncRNAs in RT in cervical cancer remains in the initial stage, and most existing studies have only been carried out in vitro; thus, clinical trials are lacking. Moreover, the particular molecular biological mechanisms by which lncRNAs regulate radiosensitivity have not yet been completely described.
Understanding the molecular pathways regulated by lncRNAs should greatly improve the effectiveness of RT in cervical cancer and reveal better treatment strategies. Through summarizing the existing research articles, it was found that lncRNAs mainly affect RT sensitivity by affecting the DNA damage repair, cell cycle, apoptosis, EMT, CSCs, and glycolysis in cervical cancer RT. Recent studies have reported that human telomerase reverse transcriptase may also be related to the effect of RT, which is a very important finding and needs to be verified by more experiments. After recognizing the role of lncRNAs in RT of cervical cancer, we need to conduct in vivo experiments to verify these conjectures. We believe that research on lncRNAs in the response to RT will provide new RT sensitization strategies for cervical cancer. Studies on lncRNAs that affect the cervical cancer RT response will provide new sensitization strategies for cervical cancer RT and lay the foundation for the clinical application of lncRNAs in the future.
Author contributions
SW wrote the manuscript. YW and CW drew the figures by Figdraw. XD and HZ participated in discussions and commented on the manuscript. TX conceived the idea, and directed and critically reviewed the manuscript.
Funding
This study was supported by grants from the Department of Science and Technology of Jilin Province (grant number 20200602008ZP).
Conflict of interest
The authors declare that the research was conducted in the absence of any commercial or financial relationships that could be construed as a potential conflict of interest.
Publisher’s note
All claims expressed in this article are solely those of the authors and do not necessarily represent those of their affiliated organizations, or those of the publisher, the editors and the reviewers. Any product that may be evaluated in this article, or claim that may be made by its manufacturer, is not guaranteed or endorsed by the publisher.
Abbreviations
RT, radiotherapy; lncRNAs, long noncoding RNAs; CSCs, cancer stem cells; EMT, epithelial–mesenchymal transition; ATM, ataxia telangiectasia-mutated; ATR, ATM and Rad-3 related; NHEJ, nonhomologous end joining; PKM2, pyruvate kinase isozyme type M2; HK2, hexokinase 2; hTERT human telomerase reverse transcriptase.
References
Bakkenist, C. J., and Kastan, M. B. (2003). DNA damage activates ATM through intermolecular autophosphorylation and dimer dissociation. Nature 421 (6922), 499–506. doi:10.1038/nature01368
Bakkenist, C. J., and Kastan, M. B. (2004). Initiating cellular stress responses. Cell 118 (1), 9–17. doi:10.1016/j.cell.2004.06.023
Barczak, W., Sobecka, A., Golusinski, P., Masternak, M. M., Rubis, B., Suchorska, W. M., et al. (2018). hTERT gene knockdown enhances response to radio- and chemotherapy in head and neck cancer cell lines through a DNA damage pathway modification. Sci. Rep. 8 (1), 5949. doi:10.1038/s41598-018-24503-y
Brown, J. M., Carlson, D. J., and Brenner, D. J. (2014). The tumor radiobiology of SRS and SBRT: Are more than the 5 Rs involved? Int. J. Radiat. Oncol. Biol. Phys. 88 (2), 254–262. doi:10.1016/j.ijrobp.2013.07.022
Chapman, J. R., Taylor, M. R., and Boulton, S. J. (2012). Playing the end game: DNA double-strand break repair pathway choice. Mol. Cell 47 (4), 497–510. doi:10.1016/j.molcel.2012.07.029
Che, Y., Li, J., Li, Z., Li, J., Wang, S., Yan, Y., et al. (2018). Osthole enhances antitumor activity and irradiation sensitivity of cervical cancer cells by suppressing ATM/NF-κB signaling. Oncol. Rep. 40 (2), 737–747. doi:10.3892/or.2018.6514
Chen, M., Liu, P., Chen, Y., Chen, Z., Shen, M., Liu, X., et al. (2018). Long noncoding RNA FAM201A mediates the radiosensitivity of esophageal squamous cell cancer by regulating ATM and mTOR expression via miR-101. Front. Genet. 9, 611. doi:10.3389/fgene.2018.00611
Chen, S., Zhang, J., Chen, J., Chen, H., Qiu, F., Yan, M., et al. (2017). The over expression of long non-coding RNA ANRIL promotes epithelial-mesenchymal transition by activating the ATM-E2F1 signaling pathway in pancreatic cancer: An in vivo and in vitro study. Int. J. Biol. Macromol. 102, 718–728. doi:10.1016/j.ijbiomac.2017.03.123
Cheng, R., Li, N., Yang, S., Liu, L., and Han, S. (2018). Long non-coding RNA ZEB1-AS1 promotes cell invasion and epithelial to mesenchymal transition through inducing ZEB1 expression in cervical cancer. Onco Targets Ther. 11, 7245–7253. doi:10.2147/ott.S179937
Chi, H. C., Tsai, C. Y., Tsai, M. M., Yeh, C. T., and Lin, K. H. (2017). Roles of long noncoding RNAs in recurrence and metastasis of radiotherapy-resistant cancer stem cells. Int. J. Mol. Sci. 18 (9), 1903. doi:10.3390/ijms18091903
Chung, Y. M., Kim, B. G., Park, C. S., Huh, S. J., Kim, J., Park, J. K., et al. (2005). Increased expression of ICAM-3 is associated with radiation resistance in cervical cancer. Int. J. Cancer 117 (2), 194–201. doi:10.1002/ijc.21180
Concin, N., Zeillinger, C., Stimpfel, M., Schiebel, I., Tong, D., Wolff, U., et al. (2000). p53-dependent radioresistance in ovarian carcinoma cell lines. Cancer Lett. 150 (2), 191–199. doi:10.1016/s0304-3835(99)00393-6
Deng, J., Yang, M., Jiang, R., An, N., Wang, X., and Liu, B. (2017). Long non-coding RNA HOTAIR regulates the proliferation, self-renewal capacity, tumor formation and migration of the cancer stem-like cell (CSC) subpopulation enriched from breast cancer cells. PLoS One 12 (1), e0170860. doi:10.1371/journal.pone.0170860
Ding, L., Li, Y., Wang, S., Wang, X., Fang, F., Wang, W., et al. (2016). Long noncoding RNA lncCAMTA1 promotes proliferation and cancer stem cell-like properties of liver cancer by inhibiting CAMTA1. Int. J. Mol. Sci. 17 (10), 1617. doi:10.3390/ijms17101617
Esteller, M. (2011). Non-coding RNAs in human disease. Nat. Rev. Genet. 12 (12), 861–874. doi:10.1038/nrg3074
Fan, L., Huang, C., Li, J., Gao, T., Lin, Z., and Yao, T. (2018). Long non-coding RNA urothelial cancer associated 1 regulates radioresistance via the hexokinase 2/glycolytic pathway in cervical cancer. Int. J. Mol. Med. 42 (4), 2247–2259. doi:10.3892/ijmm.2018.3778
Fan, M. J., Zou, Y. H., He, P. J., Zhang, S., Sun, X. M., and Li, C. Z. (2019). Long non-coding RNA SPRY4-IT1 promotes epithelial-mesenchymal transition of cervical cancer by regulating the miR-101-3p/ZEB1 axis. Biosci. Rep. 39 (6), BSR20181339. doi:10.1042/bsr20181339
Fang, X., Zhong, G., Wang, Y., Lin, Z., Lin, R., and Yao, T. (2020). Low GAS5 expression may predict poor survival and cisplatin resistance in cervical cancer. Cell Death Dis. 11 (7), 531. doi:10.1038/s41419-020-2735-2
Feng, D., Peng, C., Li, C., Zhou, Y., Li, M., Ling, B., et al. (2009). Identification and characterization of cancer stem-like cells from primary carcinoma of the cervix uteri. Oncol. Rep. 22 (5), 1129–1134. doi:10.3892/or_00000545
Feng, S., Liu, W., Bai, X., Pan, W., Jia, Z., Zhang, S., et al. (2019a). LncRNA-CTS promotes metastasis and epithelial-to-mesenchymal transition through regulating miR-505/ZEB2 axis in cervical cancer. Cancer Lett. 465, 105–117. doi:10.1016/j.canlet.2019.09.002
Feng, Y., Ma, J., Fan, H., Liu, M., Zhu, Y., Li, Y., et al. (2019b). TNF-α-induced lncRNA LOC105374902 promotes the malignant behavior of cervical cancer cells by acting as a sponge of miR-1285-3p. Biochem. Biophys. Res. Commun. 513 (1), 56–63. doi:10.1016/j.bbrc.2019.03.079
Ferlay, J., Colombet, M., Soerjomataram, I., Mathers, C., Parkin, D. M., Piñeros, M., et al. (2019). Estimating the global cancer incidence and mortality in 2018: GLOBOCAN sources and methods. Int. J. Cancer 144 (8), 1941–1953. doi:10.1002/ijc.31937
Fu, H.-C., Chuang, I.-C., Yang, Y.-C., Chuang, P.-C., Lin, H., Ou, Y.-C., et al. (2018). Low P16INK4A expression associated with high expression of cancer stem cell markers predicts poor prognosis in cervical cancer after radiotherapy. Int. J. Mol. Sci. 19 (9), 2541. doi:10.3390/ijms19092541
Fu, Z., Chen, D., Cheng, H., and Wang, F. (2015). Hypoxia-inducible factor-1α protects cervical carcinoma cells from apoptosis induced by radiation via modulation of vascular endothelial growth factor and p53 under hypoxia. Med. Sci. Monit. 21, 318–325. doi:10.12659/msm.893265
Gao, J., Liu, L., Li, G., Cai, M., Tan, C., Han, X., et al. (2019). LncRNA GAS5 confers the radio sensitivity of cervical cancer cells via regulating miR-106b/IER3 axis. Int. J. Biol. Macromol. 126, 994–1001. doi:10.1016/j.ijbiomac.2018.12.176
Ge, X., Gu, Y., Li, D., Jiang, M., Zhao, S., Li, Z., et al. (2020). Knockdown of lncRNA PCAT1 enhances radiosensitivity of cervical cancer by regulating miR-128/GOLM1 Axis. Onco Targets Ther. 13, 10373–10385. doi:10.2147/ott.S263728
Granados-López, A., Manzanares-Acuña, E., López-Hernández, Y., Castañeda-Delgado, J., Fraire-Soto, I., Reyes-Estrada, C., et al. (2021). UVB inhibits proliferation, cell cycle and induces apoptosis via p53, E2F1 and microtubules system in cervical cancer cell lines. Int. J. Mol. Sci. 22 (10), 5197. doi:10.3390/ijms22105197
Gutschner, T., and Diederichs, S. (2012). The hallmarks of cancer: A long non-coding RNA point of view. RNA Biol. 9 (6), 703–719. doi:10.4161/rna.20481
Ha, K., Fiskus, W., Rao, R., Balusu, R., Venkannagari, S., Nalabothula, N., et al. (2011). Hsp90 inhibitor-mediated disruption of chaperone association of ATR with hsp90 sensitizes cancer cells to DNA damage. Mol. Cancer Ther. 10 (7), 1194–1206. doi:10.1158/1535-7163.Mct-11-0094
Han, D., Wang, J., and Cheng, G. (2018). LncRNA NEAT1 enhances the radio-resistance of cervical cancer via miR-193b-3p/CCND1 axis. Oncotarget 9 (2), 2395–2409. doi:10.18632/oncotarget.23416
Hickson, I., Zhao, Y., Richardson, C. J., Green, S. J., Martin, N. M., Orr, A. I., et al. (2004). Identification and characterization of a novel and specific inhibitor of the ataxia-telangiectasia mutated kinase ATM. Cancer Res. 64 (24), 9152–9159. doi:10.1158/0008-5472.Can-04-2727
Hoeijmakers, J. H. (2001). Genome maintenance mechanisms for preventing cancer. Nature 411 (6835), 366–374. doi:10.1038/35077232
Hu, Y., Sun, X., Mao, C., Guo, G., Ye, S., Xu, J., et al. (2017). Upregulation of long noncoding RNA TUG1 promotes cervical cancer cell proliferation and migration. Cancer Med. 6 (2), 471–482. doi:10.1002/cam4.994
Hu, Z., Wu, H., Li, Y., Hou, Q., Wang, Y., Li, S., et al. (2015). β-Elemene inhibits the proliferation of esophageal squamous cell carcinoma by regulating long noncoding RNA-mediated inhibition of hTERT expression. Anticancer Drugs 26 (5), 531–539. doi:10.1097/cad.0000000000000216
Huarte, M., Guttman, M., Feldser, D., Garber, M., Koziol, M. J., Kenzelmann-Broz, D., et al. (2010). A large intergenic noncoding RNA induced by p53 mediates global gene repression in the p53 response. Cell 142 (3), 409–419. doi:10.1016/j.cell.2010.06.040
Jiang, Y., Li, Y., Fang, S., Jiang, B., Qin, C., Xie, P., et al. (2014). The role of MALAT1 correlates with HPV in cervical cancer. Oncol. Lett. 7 (6), 2135–2141. doi:10.3892/ol.2014.1996
Jing, L., Yuan, W., Ruofan, D., Jinjin, Y., and Haifeng, Q. (2015). HOTAIR enhanced aggressive biological behaviors and induced radio-resistance via inhibiting p21 in cervical cancer. Tumor Biol. 36 (5), 3611–3619. doi:10.1007/s13277-014-2998-2
Khalilia, W. M., Özcan, G., and Karaçam, S. (2018). Apoptosis signalling pathway in cervical cancer cells treated with gama radiation. Proceedings 2, 1531. doi:10.3390/proceedings2251531
Kim, H. J., Eoh, K. J., Kim, L. K., Nam, E. J., Yoon, S. O., Kim, K. H., et al. (2016). The long noncoding RNA HOXA11 antisense induces tumor progression and stemness maintenance in cervical cancer. Oncotarget 7 (50), 83001–83016. doi:10.18632/oncotarget.12863
Kim, H. J., Lee, D. W., Yim, G. W., Nam, E. J., Kim, S., Kim, S. W., et al. (2015). Long non-coding RNA HOTAIR is associated with human cervical cancer progression. Int. J. Oncol. 46 (2), 521–530. doi:10.3892/ijo.2014.2758
Krokan, H. E., and Bjørås, M. (2013). Base excision repair. Cold Spring Harb. Perspect. Biol. 5 (4), a012583. doi:10.1101/cshperspect.a012583
Lee, J. H., and Paull, T. T. (2004). Direct activation of the ATM protein kinase by the Mre11/Rad50/Nbs1 complex. Science 304 (5667), 93–96. doi:10.1126/science.1091496
Lee, S. Y., Jeong, E. K., Ju, M. K., Jeon, H. M., Kim, M. Y., Kim, C. H., et al. (2017). Induction of metastasis, cancer stem cell phenotype, and oncogenic metabolism in cancer cells by ionizing radiation. Mol. Cancer 16 (1), 10. doi:10.1186/s12943-016-0577-4
Li, F., Lv, J., Liang, L., Wang, J., Li, C., Sun, L., et al. (2018a). Downregulation of microRNA-21 inhibited radiation-resistance of esophageal squamous cell carcinoma. Cancer Cell Int. 18, 39. doi:10.1186/s12935-018-0502-6
Li, J., and Zhou, B. P. (2011). Activation of β-catenin and Akt pathways by Twist are critical for the maintenance of EMT associated cancer stem cell-like characters. BMC Cancer 11, 49. doi:10.1186/1471-2407-11-49
Li, N., Meng, D., Gao, L., Xu, Y., Liu, P., Tian, Y., et al. (2018b). Overexpression of HOTAIR leads to radioresistance of human cervical cancer via promoting HIF-1α expression. Radiat. Oncol. 13 (1), 210. doi:10.1186/s13014-018-1153-4
Li, Z., Zhang, Y., Sui, S., Hua, Y., Zhao, A., Tian, X., et al. (2020). Targeting HMGB3/hTERT axis for radioresistance in cervical cancer. J. Exp. Clin. Cancer Res. 39 (1), 243. doi:10.1186/s13046-020-01737-1
Lin, J., Liu, Z., Liao, S., Li, E., Wu, X., and Zeng, W. J. G. (2020). Elevation of long non-coding RNA GAS5 and knockdown of microRNA-21 up-regulate RECK expression to enhance esophageal squamous cell carcinoma cell radio-sensitivity after radiotherapy. Genomics 112 (3), 2173–2185. doi:10.1016/j.ygeno.2019.12.013
Lin, Y., Zhai, H., Ouyang, Y., Lu, Z., Chu, C., He, Q., et al. (2019). Knockdown of PKM2 enhances radiosensitivity of cervical cancer cells. Cancer Cell Int. 19, 129. doi:10.1186/s12935-019-0845-7
Liu, J., Liu, X., and Li, R. (2020a). LncRNA SNHG6 enhances the radioresistance and promotes the growth of cervical cancer cells by sponging miR-485-3p. Cancer Cell Int. 20 (1), 424. doi:10.1186/s12935-020-01448-9
Liu, R., Zhang, Q., Shen, L., Chen, S., He, J., Wang, D., et al. (2020b). Long noncoding RNA lnc-RI regulates DNA damage repair and radiation sensitivity of CRC cells through NHEJ pathway. Cell Biol. Toxicol. 36 (5), 493–507. doi:10.1007/s10565-020-09524-6
Liu, Y., Chen, X., Chen, X., Liu, J., Gu, H., Fan, R., et al. (2020c). Long non-coding RNA HOTAIR knockdown enhances radiosensitivity through regulating microRNA-93/ATG12 axis in colorectal cancer. Cell Death Dis. 11 (3), 175. doi:10.1038/s41419-020-2268-8
Long, Y., Wang, X., Youmans, D. T., and Cech, T. R. (2017). How do lncRNAs regulate transcription? Sci. Adv. 3 (9), eaao2110. doi:10.1126/sciadv.aao2110
López, J., Poitevin, A., Mendoza-Martínez, V., Pérez-Plasencia, C., and García-Carrancá, A. (2012). Cancer-initiating cells derived from established cervical cell lines exhibit stem-cell markers and increased radioresistance. BMC Cancer 12, 48. doi:10.1186/1471-2407-12-48
Lord, C. J., and Ashworth, A. (2012). The DNA damage response and cancer therapy. Nature 481 (7381), 287–294. doi:10.1038/nature10760
Lu, H., He, Y., Lin, L., Qi, Z., Ma, L., Li, L., et al. (2016). Long non-coding RNA MALAT1 modulates radiosensitivity of HR-HPV+ cervical cancer via sponging miR-145. Tumour Biol. 37 (2), 1683–1691. doi:10.1007/s13277-015-3946-5
Luan, X., and Wang, Y. (2018). LncRNA XLOC_006390 facilitates cervical cancer tumorigenesis and metastasis as a ceRNA against miR-331-3p and miR-338-3p. J. Gynecol. Oncol. 29 (6), e95. doi:10.3802/jgo.2018.29.e95
Łuczak, A., Supernat, A., Łapińska-Szumczyk, S., Jachimowicz, D., Majewska, H., Gulczyński, J., et al. (2016). HOTAIR in relation to epithelial-mesenchymal transition and cancer stem cells in molecular subtypes of endometrial cancer. Int. J. Biol. Markers 31 (3), e245–e251. doi:10.5301/jbm.5000187
Malhotra, S., Freeberg, M. A., Winans, S. J., Taylor, J., and Beemon, K. L. (2018). A novel long non-coding RNA in the hTERT promoter region regulates hTERT expression. Noncoding RNA 4 (1), 1. doi:10.3390/ncrna4010001
Mani, S. A., Guo, W., Liao, M. J., Eaton, E. N., Ayyanan, A., Zhou, A. Y., et al. (2008). The epithelial-mesenchymal transition generates cells with properties of stem cells. Cell 133 (4), 704–715. doi:10.1016/j.cell.2008.03.027
Mao, P., and Wyrick, J. J. (2016). Emerging roles for histone modifications in DNA excision repair. FEMS Yeast Res. 16 (7), fow090. doi:10.1093/femsyr/fow090
Marney, C. B., Anderson, E. S., Baum, R., and Schmitt, A. M. (2022). A unique spectrum of spontaneous tumors in dino knockout mice identifies tissue-specific requirements for tumor suppression. Cells 11 (11), 1818. doi:10.3390/cells11111818
Meng, Q., Wang, W., Liu, X., Hou, X., Lian, X., Sun, S., et al. (2018). Escalated radiation and prophylactic extended field nodal irradiation are beneficial for FIGO IIIB cervical cancer patients' prognosis. Radiat. Oncol. 13 (1), 223. doi:10.1186/s13014-018-1172-1
Nemeth, M. J., Kirby, M. R., and Bodine, D. M. (2006). Hmgb3 regulates the balance between hematopoietic stem cell self-renewal and differentiation. Proc. Natl. Acad. Sci. U. S. A. 103 (37), 13783–13788. doi:10.1073/pnas.0604006103
Oei, A., van Leeuwen, C., ten Cate, R., Rodermond, H., Buist, M., Stalpers, L., et al. (2015). Hyperthermia selectively targets human papillomavirus in cervical tumors via p53-dependent apoptosis. Cancer Res. 75 (23), 5120–5129. doi:10.1158/0008-5472.Can-15-0816
Olive, P. L. (1998). The role of DNA single- and double-strand breaks in cell killing by ionizing radiation. Radiat. Res. 150, S42–S51. doi:10.2307/3579807
Pádua Alves, C., Fonseca, A. S., Muys, B. R., de Barros, E. L. B. R., Bürger, M. C., de Souza, J. E., et al. (2013). Brief report: The lincRNA Hotair is required for epithelial-to-mesenchymal transition and stemness maintenance of cancer cell lines. Stem Cells 31 (12), 2827–2832. doi:10.1002/stem.1547
Ponti, D., Costa, A., Zaffaroni, N., Pratesi, G., Petrangolini, G., Coradini, D., et al. (2005). Isolation and in vitro propagation of tumorigenic breast cancer cells with stem/progenitor cell properties. Cancer Res. 65 (13), 5506–5511. doi:10.1158/0008-5472.CAN-05-0626
Prabakaran, D. S., Muthusami, S., Sivaraman, T., Yu, J.-R., and Park, W.-Y. (2019). Silencing of FTS increases radiosensitivity by blocking radiation-induced Notch1 activation and spheroid formation in cervical cancer cells. Int. J. Biol. Macromol. 126, 1318–1325. doi:10.1016/j.ijbiomac.2018.09.114
Puckett, D. L., Alquraishi, M., Chowanadisai, W., and Bettaieb, A. (2021). The role of PKM2 in metabolic reprogramming: Insights into the regulatory roles of non-coding RNAs. Int. J. Mol. Sci. 22 (3), 1171. doi:10.3390/ijms22031171
Quennet, V., Yaromina, A., Zips, D., Rosner, A., Walenta, S., Baumann, M., et al. (2006). Tumor lactate content predicts for response to fractionated irradiation of human squamous cell carcinomas in nude mice. Radiother. Oncol. 81 (2), 130–135. doi:10.1016/j.radonc.2006.08.012
Ravi, R., Mookerjee, B., Bhujwalla, Z. M., Sutter, C. H., Artemov, D., Zeng, Q., et al. (2000). Regulation of tumor angiogenesis by p53-induced degradation of hypoxia-inducible factor 1α. Genes Dev. 14 (1), 34–44. doi:10.1101/gad.14.1.34
Reya, T., Morrison, S. J., Clarke, M. F., and Weissman, I. L. (2001). Stem cells, cancer, and cancer stem cells. Nature 414 (6859), 105–111. doi:10.1038/35102167
Roossink, F., Wieringa, H. W., Noordhuis, M. G., ten Hoor, K. A., Kok, M., Slagter-Menkema, L., et al. (2012). The role of ATM and 53BP1 as predictive markers in cervical cancer. Int. J. Cancer 131 (9), 2056–2066. doi:10.1002/ijc.27488
San-Millán, I., and Brooks, G. A. (2017). Reexamining cancer metabolism: Lactate production for carcinogenesis could be the purpose and explanation of the Warburg effect. Carcinogenesis 38 (2), 119–133. doi:10.1093/carcin/bgw127
Sancar, A. (1995). DNA repair in humans. Annu. Rev. Genet. 29, 69–105. doi:10.1146/annurev.ge.29.120195.000441
Schärer, O. D. (2003). Chemistry and biology of DNA repair. Angew. Chem. Int. Ed. Engl. 42 (26), 2946–2974. doi:10.1002/anie.200200523
Sharma, S., Munger, K., and Shenk, T. (2020). Expression of the long noncoding RNA DINO in human papillomavirus-positive cervical cancer cells reactivates the dormant TP53 tumor suppressor through ATM/CHK2 signaling. mBio 11 (3), e01190–e01120. doi:10.1128/mBio.01190-20
Shay, J. W., and Wright, W. E. (2011). Role of telomeres and telomerase in cancer. Semin. Cancer Biol. 21 (6), 349–353. doi:10.1016/j.semcancer.2011.10.001
Shiloh, Y., and Ziv, Y. (2013). The ATM protein kinase: Regulating the cellular response to genotoxic stress, and more. Nat. Rev. Mol. Cell Biol. 14 (4), 197–210. doi:10.1038/nrm3546
Shimura, T., Noma, N., Sano, Y., Ochiai, Y., Oikawa, T., Fukumoto, M., et al. (2014). AKT-mediated enhanced aerobic glycolysis causes acquired radioresistance by human tumor cells. Radiother. Oncol. 112 (2), 302–307. doi:10.1016/j.radonc.2014.07.015
Song, Y., Zuo, Y., Qian, X., Chen, Z., Wang, S., Song, L., et al. (2017). Inhibition of MicroRNA-21-5p promotes the radiation sensitivity of non-small cell lung cancer through HMSH2. Cell Physiol. Biochem. 43 (3), 1258–1272. doi:10.1159/000481839
Sun, R., Qin, C., Jiang, B., Fang, S., Pan, X., Peng, L., et al. (2016). Down-regulation of MALAT1 inhibits cervical cancer cell invasion and metastasis by inhibition of epithelial-mesenchymal transition. Mol. Biosyst. 12 (3), 952–962. doi:10.1039/c5mb00685f
Sun, Y., Jiang, X., Chen, S., Fernandes, N., and Price, B. D. (2005). A role for the Tip60 histone acetyltransferase in the acetylation and activation of ATM. Proc. Natl. Acad. Sci. U. S. A. 102 (37), 13182–13187. doi:10.1073/pnas.0504211102
Tamari, K., Sano, K., Li, Z., Seo, Y., Otani, K., Tatekawa, S., et al. (2019). Ro 90-7501 is a novel radiosensitizer for cervical cancer cells that inhibits ATM phosphorylation. Anticancer Res. 39 (9), 4805–4810. doi:10.21873/anticanres.13665
Tang, D., Yang, Z., Long, F., Luo, L., Yang, B., Zhu, R., et al. (2019a). Long noncoding RNA MALAT1 mediates stem cell-like properties in human colorectal cancer cells by regulating miR-20b-5p/Oct4 axis. J. Cell Physiol. 234 (11), 20816–20828. doi:10.1002/jcp.28687
Tang, T., Shan, G., and Zeng, F. J. J. o. c. p. (2019b). Knockdown of DGCR5 enhances the radiosensitivity of human laryngeal carcinoma cells via inducing miR-195. J. Cell Physiol. 234 (8), 12918–12925. doi:10.1002/jcp.27958
Teng, P., Bateman, N., Darcy, K., Hamilton, C., Maxwell, G., Bakkenist, C., et al. (2015). Pharmacologic inhibition of ATR and ATM offers clinically important distinctions to enhancing platinum or radiation response in ovarian, endometrial, and cervical cancer cells. Gynecol. Oncol. 136 (3), 554–561. doi:10.1016/j.ygyno.2014.12.035
Thapar, R., Wang, J. L., Hammel, M., Ye, R., Liang, K., Sun, C., et al. (2020). Mechanism of efficient double-strand break repair by a long non-coding RNA. Nucleic Acids Res. 48 (19), 10953–10972. doi:10.1093/nar/gkaa784
Toledo, L. I., Murga, M., Zur, R., Soria, R., Rodriguez, A., Martinez, S., et al. (2011). A cell-based screen identifies ATR inhibitors with synthetic lethal properties for cancer-associated mutations. Nat. Struct. Mol. Biol. 18 (6), 721–727. doi:10.1038/nsmb.2076
Vander Heiden, M. G., Cantley, L. C., and Thompson, C. B. (2009). Understanding the Warburg effect: The metabolic requirements of cell proliferation. Science 324 (5930), 1029–1033. doi:10.1126/science.1160809
Wang, C., Shi, M., Ji, J., Cai, Q., Jiang, J., Zhang, H., et al. (2019). A self-enforcing HOXA11/Stat3 feedback loop promotes stemness properties and peritoneal metastasis in gastric cancer cells. Theranostics 9 (25), 7628–7647. doi:10.7150/thno.36277
Wang, F., Li, X., Xie, X., Zhao, L., and Chen, W. (2008). UCA1, a non-protein-coding RNA up-regulated in bladder carcinoma and embryo, influencing cell growth and promoting invasion. FEBS Lett. 582 (13), 1919–1927. doi:10.1016/j.febslet.2008.05.012
Wang, H. J., Pochampalli, M., Wang, L. Y., Zou, J. X., Li, P. S., Hsu, S. C., et al. (2019). KDM8/JMJD5 as a dual coactivator of AR and PKM2 integrates AR/EZH2 network and tumor metabolism in CRPC. Oncogene 38 (1), 17–32. doi:10.1038/s41388-018-0414-x
Wang, K., Wu, X., Wang, J., and Huang, J. J. I. J. o. N. (2013). Cancer stem cell theory: Therapeutic implications for nanomedicine. Int. J. Nanomedicine 8, 899–908. doi:10.2147/IJN.S38641
Wang, P., Yang, Z., Ye, T., Shao, F., Li, J., Sun, N., et al. (2020). lncTUG1/miR-144-3p affect the radiosensitivity of esophageal squamous cell carcinoma by competitively regulating c-MET. J. Exp. Clin. Cancer Res. 39 (1), 7. doi:10.1186/s13046-019-1519-y
Wang, X., Liu, H., Shi, L., Yu, X., Gu, Y., and Sun, X. J. C. c. (2018). LINP1 facilitates DNA damage repair through non-homologous end joining (NHEJ) pathway and subsequently decreases the sensitivity of cervical cancer cells to ionizing radiation. Cell Cycle 17 (4), 439–447. doi:10.1080/15384101.2018.1442625
Wang, Y., He, L., Du, Y., Zhu, P., Huang, G., Luo, J., et al. (2015). The long noncoding RNA lncTCF7 promotes self-renewal of human liver cancer stem cells through activation of Wnt signaling. Cell Stem Cell 16 (4), 413–425. doi:10.1016/j.stem.2015.03.003
Wei, J., Wang, L., Sun, Y., and Bao, Y. J. C. b. (2020). LINC00662 contributes to the progression and the radioresistance of cervical cancer by regulating miR-497-5p and CDC25A. Cell Biochem. Funct. 38 (8), 1139–1151. doi:10.1002/cbf.3580
Wilson, G. D. (2004). Radiation and the cell cycle, revisited. Cancer Metastasis Rev. 23 (3-4), 209–225. doi:10.1023/B:CANC.0000031762.91306.b4
Withers, H. R. (1975). “The four R's of radiotherapy,” in Advances in radiation biology. Editors J. T. Lett, and H. Adler (Amsterdam, Netherlands: Elsevier), 241–271.
Wu, C., Yang, L., Qi, X., Wang, T., Li, M., and Xu, K. (2018). Inhibition of long non-coding RNA HOTAIR enhances radiosensitivity via regulating autophagy in pancreatic cancer. Cancer Manag. Res. 10, 5261–5271. doi:10.2147/cmar.S174066
Wu, J., and Wang, D. J. H. c. (2017). Long noncoding RNA TCF7 promotes invasiveness and self-renewal of human non-small cell lung cancer cells. Hum. Cell 30 (1), 23–29. doi:10.1007/s13577-016-0147-5
Wu, L., Gong, Y., Yan, T., and Zhang, H. (2020). LINP1 promotes the progression of cervical cancer by scaffolding EZH2, LSD1, and DNMT1 to inhibit the expression of KLF2 and PRSS8. Biochem. Cell Biol. 98 (5), 591–599. doi:10.1139/bcb-2019-0446
Wyllie, A. H. (2010). Where, O death, is thy sting?" A brief review of apoptosis biology. Mol. Neurobiol. 42 (1), 4–9. doi:10.1007/s12035-010-8125-5
Xu, D., Dong, P., Xiong, Y., Yue, J., Konno, Y., Ihira, K., et al. (2020). MicroRNA-361-Mediated inhibition of HSP90 expression and EMT in cervical cancer is counteracted by oncogenic lncRNA NEAT1. Cells 9 (3), 632. doi:10.3390/cells9030632
Yang, Q., Li, B., Xu, G., Yang, S., Wang, P., Tang, H., et al. (2019a). Long noncoding RNA LINC00483/microRNA-144 regulates radiosensitivity and epithelial-mesenchymal transition in lung adenocarcinoma by interacting with HOXA10. J. Cell Physiol. 234 (7), 11805–11821. doi:10.1002/jcp.27886
Yang, X. D., Xu, H. T., Xu, X. H., Ru, G., Liu, W., Zhu, J. J., et al. (2016). Knockdown of long non-coding RNA HOTAIR inhibits proliferation and invasiveness and improves radiosensitivity in colorectal cancer. Oncol. Rep. 35 (1), 479–487. doi:10.3892/or.2015.4397
Yang, Z., Gu, Q., Wang, Y., Liu, B., Zhou, G., Shao, C., et al. (2019b). Heavy-ion carbon radiation regulates long non-coding RNAs in cervical cancer HeLa cells. J. Cancer 10 (21), 5022–5030. doi:10.7150/jca.30846
Yao, Q., Xu, H., Zhang, Q., Zhou, H., Qu, L. J. B., and communications, b. r. (2009). MicroRNA-21 promotes cell proliferation and down-regulates the expression of programmed cell death 4 (PDCD4) in HeLa cervical carcinoma cells. Biochem. Biophys. Res. Commun. 388 (3), 539–542. doi:10.1016/j.bbrc.2009.08.044
Yao, Z., Zhang, Y., Xu, D., Zhou, X., Peng, P., Pan, Z., et al. (2019). Research progress on long non-coding RNA and radiotherapy. Med. Sci. Monit. 25, 5757–5770. doi:10.12659/msm.915647
Yoon, S., Ha, H. J., Kim, Y. H., Won, M., Park, M., Ko, J. J., et al. (2009). IEX-1-induced cell death requires BIM and is modulated by MCL-1. Biochem. Biophys. Res. Commun. 382 (2), 400–404. doi:10.1016/j.bbrc.2009.03.037
Yuan, J., Song, Y., Pan, W., Li, Y., Xu, Y., Xie, M., et al. (2020). LncRNA SLC26A4-AS1 suppresses the MRN complex-mediated DNA repair signaling and thyroid cancer metastasis by destabilizing DDX5. Oncogene 39 (43), 6664–6676. doi:10.1038/s41388-020-01460-3
Zhai, J. J., Du, X. R., and Li, C. X. (2020). [Effect of lncRNA HOTAIR on the radiosensitivity of HCCLM3 cells]. Zhonghua Yi Xue Za Zhi 100 (18), 1419–1425. doi:10.3760/cma.j.cn112137-20190928-02130
Zhang, C., Jiang, F., Su, C., Xie, P., and Xu, L. J. J. o. c. b. (2019). Upregulation of long noncoding RNA SNHG20 promotes cell growth and metastasis in esophageal squamous cell carcinoma via modulating ATM-JAK-PD-L1 pathway. J. Cell Biochem. 120, 11642–11650. doi:10.1002/jcb.28444
Zhang, J., Si, J., Gan, L., Guo, M., Yan, J., Chen, Y., et al. (2020). Inhibition of Wnt signalling pathway by XAV939 enhances radiosensitivity in human cervical cancer HeLa cells. Artif. Cells Nanomed Biotechnol. 48 (1), 479–487. doi:10.1080/21691401.2020.1716779
Zhang, L., Yao, H., Liu, S., and Song, L. J. E. R. M. P. S. (2020). Long noncoding RNA ROR1-AS1 overexpression predicts poor prognosis and promotes metastasis by activating Wnt/β-catenin/EMT signaling cascade in cervical cancer. Eur. Rev. Med. Pharmacol. Sci. 24 (6), 2928–2937. doi:10.26355/eurrev_202003_20656
Zhang, W., Liu, J., Wu, Q., Liu, Y., and Ma, C. (2021). HOTAIR contributes to stemness acquisition of cervical cancer through regulating miR-203 interaction with ZEB1 on epithelial-mesenchymal transition. J. Oncol. 2021, 4190764. doi:10.1155/2021/4190764
Keywords: cervical cancer, long noncoding RNA (lncRNA), radiosensitive, DNA damage repair, apoptosis
Citation: Wu S, Zhu H, Wu Y, Wang C, Duan X and Xu T (2023) Molecular mechanisms of long noncoding RNAs associated with cervical cancer radiosensitivity. Front. Genet. 13:1093549. doi: 10.3389/fgene.2022.1093549
Received: 09 November 2022; Accepted: 16 December 2022;
Published: 04 January 2023.
Edited by:
Yujing Li, Emory University, United StatesReviewed by:
Bibha Choudhary, Institute of Bioinformatics and Applied Biotechnology, IndiaLiang-Ting Lin, Hong Kong Polytechnic University, Hong Kong SAR, China
Copyright © 2023 Wu, Zhu, Wu, Wang, Duan and Xu. This is an open-access article distributed under the terms of the Creative Commons Attribution License (CC BY). The use, distribution or reproduction in other forums is permitted, provided the original author(s) and the copyright owner(s) are credited and that the original publication in this journal is cited, in accordance with accepted academic practice. No use, distribution or reproduction is permitted which does not comply with these terms.
*Correspondence: Tianmin Xu, eHV0aWFubWluQDEyNi5jb20=