- 1Clinical Immunology Translational Medicine Key Laboratory of Sichuan Province, Sichuan Provincial People’s Hospital, University of Electronic Science and Technology of China, Chengdu, China
- 2Department of Rheumatology and Immunology, Sichuan Academy of Medical Science and Sichuan Provincial People’s Hospital, University of Electronic Science and Technology of China, Chengdu, China
- 3Department of Rheumatology, Wenjiang District People’s Hospital, Chengdu, China
- 4School of Medicine, University of Electronic Science and Technology of China, Chengdu, China
- 5Department of Critical Care Medicine, Sichuan Academy of Medical Science and Sichuan Provincial People’s Hospital, University of Electronic Science and Technology of China, Chengdu, China
Unlike apoptosis, necroptosis, autophagy, and pyroptosis, ferroptosis represents a new type of cell death, which is characterized by iron-dependent lipid peroxidation. This process relies largely on the metabolite reactive oxygen species (ROS), phospholipids containing polyunsaturated fatty acids (PUFA-PL), transition metal iron, intra-, and intercellular signaling events, and environmental stress that regulate cellular metabolism and ROS levels. Recent studies show that ferroptosis plays an important role in tumorigenesis, tumor development, and the treatment of hematological malignancies, including lymphoma. Despite the constant emergence of new drugs, the differences in morphological features, immunophenotypes, biological patterns, rates of onset, and response to treatment in lymphoma pose major therapeutic challenges. Since lymphoma is associated with ferroptosis and shows sensitivity towards it, targeting the potential regulatory factors may regulate lymphoma progression. This has emerged as a research hotspot. This review summarizes the current knowledge on ferroptosis induction and resistance mechanisms, their roles and mechanistic details of ferroptosis in lymphoma suppression and immunity, and finally the treatment strategies for lymphoma by targeting ferroptosis.
1 Introduction
Cell death, including apoptosis (Nagata, 2018), NETosis (Segura et al., 2020), necroptosis (Guo et al., 2019), autophagy (Bonam et al., 2018), pyroptosis (Fu et al., 2017), and cuproptosis (Tsvetkov et al., 2022), is an important physiological process that maintains the integrity by regulating organismal metabolism and removing excess or damaged cells.
Ferroptosis, unlike other forms, is a new type of programmed cell death that shows iron-dependence (Li et al., 2020). Several tumor suppressors, including p53 and BAP1, promote ferroptosis by inhibiting cystine uptake, confirming that ferroptosis is a natural barrier against tumor development (Toyokuni et al., 2017; Zhang et al., 2018). Moreover, tumor cells are more prone to ferroptosis due to their unique metabolic program and high load of reactive oxygen species (ROS) (Chen et al., 2020a), rendering ferroptosis inducers as potential targets of cancer therapy, especially against some of the most drug-resistant and aggressive tumors (Hangauer et al., 2017; Viswanathan et al., 2017). Non-Hodgkin’s lymphomas are clinically heterogeneous, and different subtypes show varying prognoses; even in the same type, some cases are curable with combination therapy, while for the remaining, treatment is difficult and patients exhibit poor prognosis (Chapuy et al., 2018). Diffuse large B cell lymphoma (DLBCL), adult T-cell leukemia/lymphoma (ATLL), and Burkitt’s lymphoma (BL) are particularly sensitive to cell death by ferroptosis as shown in recent studies (Yang et al., 2014; Mancuso et al., 2021; Chen et al., 2022). Thus, treatment with ferroptosis inducers may be a promising therapeutic strategy for lymphoma in the foreseeable future.
2 Mechanisms underlying ferroptosis
Ferroptosis is driven by increased cellular iron load and is characterized by the loss in membrane integrity and changes in mitochondrial ultrastructure, including reduced mitochondrial volume, increased mitochondrial bilayer membrane density, decreased mitochondrial cristae, and ruptured mitochondrial membrane (Doll et al., 2017; Du et al., 2022; Zhou et al., 2022). Ferroptosis can be induced through the interaction of iron regulation, lipid metabolism, and ROS biology, and research in these fields is expected to deepen the mechanistic understanding, biological significance, and clinical therapeutic relevance of ferroptosis (Table 1). However, to date, our understanding of ferroptosis remains incomplete, and the molecular mechanisms that trigger ferroptosis and its selective regulation under certain circumstances, are largely unclear (Davidson and Wood, 2020).
2.1 Role of iron metabolism in ferroptosis
Fe2+ first binds to transferrin (TF) in the intestine and is subsequently absorbed by intestinal mucosal cells, wherein Fe2+ is oxidized to Fe3+ by ceruloplasmin and other ferroxidases (Cronin et al., 2019). Fe3+ enters the capillaries and then binds to TF, which in turn binds to the TF receptor 1 (TfR1) and is endocytosed into cells (Pandrangi et al., 2022). Fe3+ is then reduced to Fe2+ by the metalloreductase, six-transmembrane epithelial antigen of the prostate (STEAP3), and subsequently, Fe2+ is released into the labile iron pool (LIP) via divalent metal transporter one or stored as ferritin, the iron-storage protein (Li et al., 2020). Excessive Fe2+ is oxidized to Fe3+ by the cellular iron exporter, ferroportin (FPN) (Li et al., 2021). There are two major mechanisms by which iron ions regulate ferroptosis. First, Fe2+ can mediate the Fenton reaction to generate excess ROS, which subsequently reacts with polyunsaturated fatty acids (PUFAs) to promote lipid peroxidation, ultimately leading to ferroptosis (Shah et al., 2018). Second, some enzymes that mediate the formation of lipid hydroperoxides for the Fenton reaction require Fe2+ as a cofactor, including arachidonate lipoxygenases (ALOXs) (Li et al., 2021). The regulation of the iron-storage protein, ferritin, via ferritinophagy (Stockwell, 2022) and the serine/threonine kinase mutated in Ataxia-Telangiectasia (ATM) (Chen et al., 2020a) control the size of the LIP, which promotes labile iron accumulation and regulates cellular sensitivity to ferroptosis (Chen et al., 2020a; Stockwell, 2022). Ferroptosis-inducer-1,2-dioxolane (FINO2) induces ferroptosis by oxidizing Fe2+ to Fe3+ through the Fenton reaction to generate alkoxyl radical which initiates lipid peroxidation. Moreover, FINO2 can indirectly inactivate GPX4 by depleting glutathione (GSH) (Abrams et al., 2016). Fe2+ from the LIP binds to GSH(Patel et al., 2019) and its subsequent depletion promotes the availability of labile iron, which further mobilizes Fe2+ for the Fenton reaction, thereby promoting lipid peroxidation and ferroptosis (Patel et al., 2019). Heme oxygenase-1 (HO-1) can induce ferroptosis by promoting the accumulation of intracellular ferrous ions (Jing et al., 2022). Ferroportin and prominin-2-mediated ferritin-containing multivesicular bodies can deplete the LIP and induce resistance to ferroptosis (Brown et al., 2019). Overexpression of heat shock protein beta-1 can inhibit ferroptosis by downregulating TfR1-mediated iron uptake by stabilizing the F-actin cytoskeleton (Ren et al., 2020). Consequently, silencing the TFRC gene encoding TfR1 protein can inhibit ferroptosis (Song et al., 2021).
2.2 Lipid metabolism in ferroptosis
Ferroptosis is mainly driven by the peroxidation of specific membrane lipids. PUFAs are highly susceptible to ROS-induced peroxidation and are crucial to ferroptosis (Conrad et al., 2018). Free PUFAs cannot induce ferroptosis. After incorporation into membrane lipids, including phospholipids (PLs), the excessive accumulation of oxidized (PUFA-PLs) can contribute to ferroptosis (Xu et al., 2021). Lysophosphatidylcholine acyltransferase 3 and acyl-coenzyme A synthetase long-chain family member 4 (ACSL4) are involved in activating and incorporating PUFAs into PLs (Brown et al., 2019). Other ACSL enzymes, including ACSL1, are required to exert the pro-ferroptosis activity of conjugated linolenic acids (Beatty et al., 2021). Moreover, monounsaturated fatty acids (MUFAs), including oleic acid and palmitoleic acid, are not susceptible to peroxidation due to a lack of a bisallyl moiety (Magtanong et al., 2019). In turn, they require ACSL3 to displace the PUFA component from PUFA-PLs, thus exerting anti-ferroptosis effects (Magtanong et al., 2019). Activation of the liver kinase B1-AMP-activated protein kinase axis also plays a protective role against ferroptosis by limiting PUFA biosynthesis by inhibiting the phosphorylation of acetyl-CoA carboxylase (ACC) (Lee et al., 2020).
2.3 ROS accumulation in ferroptosis
Generation of ROS is a result of Fenton reactions and its accumulation can induce ferroptosis (Tang et al., 2021). The cytoplasmic and mitochondrial cystine/cysteine/GSH/GPX4 axis is a central regulator to protect cells from ferroptosis (Jiang et al., 2021). System Xc-is a heterodimeric 12-pass transmembrane cystine/glutamate antiporter comprising SLC3A2 and SLC7A11 subunits, which import cystine that is reduced to cysteine in the cell (Sato et al., 2018). Cysteine and glutamate are substrates for the biosynthesis of GSH, and GPX4 is a selenoprotein acting as a GSH-dependent peroxidase against lipid peroxidation by reducing the toxic lipid peroxide PL-PUFA-OOH to non-toxic PUFA-PL-OH (Ursini and Maiorino, 2020).
Substances that induce ferroptosis through the cystine/cysteine/GSH/GPX4 axis can be divided into four categories. Erastin is the prototype ferroptosis inducer that reduces cellular cystine uptake by directly inhibiting system Xc- and promotes the degradation of GPX4 by enhancing chaperone-mediated autophagy (Wu et al., 2019). Rat sarcoma-selective-lethal-3 (RSL3) and 5,6-dihydro-2H-pyrano [3,2-g]indolizine-7 can induce ferroptosis by directly inhibiting the activity of GPX4 (7). Ferroptosis-Inducer-56 (FIN56) can induce ferroptosis either by promoting GPX4 degradation or depleting endogenous antioxidant coenzyme Q10 (COQ10) (Liang et al., 2019). FINO2, as discussed above, can also initiate ferroptosis by indirectly inhibiting GPX4 (42). Other new molecules that induce ferroptosis have been recently discovered. The multidrug resistance gene, MDR1, increases cellular sensitivity to ferroptosis through GSH efflux (Cao et al., 2019), and the iron metalloenzyme, cysteine dioxygenase1, drives cell susceptibility to ferroptosis by depleting cysteine, which in turn reduces GSH (Hao et al., 2017).
GPX4 plays an important role in maintaining intracellular redox homeostasis and is the primary enzyme that inhibits ferroptosis. However, three other GPX4-independent systems also play a role in suppressing ferroptosis. The ferroptosis suppressor protein 1 (FSP1)/CoQ10/nicotinamide adenine dinucleotide phosphate (NADPH) pathway regulates ferroptosis independent of GPX4 and GSH. FSP1 catalyzes the formation of reduced CoQ10 in an NADPH-dependent manner, thereby reducing lipid free radicals and ultimately inhibiting lipid peroxidation and ferroptosis (Bersuker et al., 2019). Dihydroorotate dehydrogenase inhibits mitochondrial lipid peroxidation and ferroptosis by reducing mitochondrial CoQ10 to CoQH2 (Mao et al., 2021). The GTP cyclohydrolase 1/tetrahydrobiopterin axis also inhibits ferroptosis by reducing CoQ10 against lipid peroxidation and depleting PUFA-PLs (Kraft et al., 2020). Furthermore, several negative regulators of ferroptosis are known. The antioxidant transcription factor, NRF2, suppresses ferroptosis by regulating the expression of genes related to GSH biosynthesis, NADPH regeneration, and iron homeostasis (Song and Long, 2020). Trans-sulfuration produces cystine from methionine, providing more substrates for GSH and inhibiting ferroptosis (Zhang et al., 2022). Activation of the recombination activating gene (Rag)- the mechanistic target of rapamycin complex 1 (mTORC1)-eukaryotic initiation factor 4E (eIF4E)-binding proteins (4EBPs) signaling axis induces resistance to ferroptosis by increasing GPX4 synthesis (Zhang et al., 2021) and the phosphatidylinositol 3-kinase-alpha serine/threonine-protein kinase-the mechanistic target of rapamycin pathway can suppress ferroptosis by increasing sterol regulatory element-binding protein-mediated lipogenesis (Yi et al., 2020).
2.4 p53 in ferroptosis
p53 can inhibit the expression of SLC7A11 at the transcriptional level and subsequently reduce the biosynthesis of GSH, thus inducing ferroptosis and suppressing tumor growth (Hu et al., 2022). In 2019, Gu Wei et al. demonstrated that the p53-SLC7A11 axis can also promote ferroptosis in a GSH-independent manner (Liu and Gu, 2022). ALOX12, a lipoxygenase, oxidizes PUFA and induces cellular ferroptosis. p53 induces ALOX12 activity by repressing SLC7A11 expression as the latter can directly bind to the former, thereby limiting its functions (Liu and Gu, 2022). p53 induces SAT1 expression, thus promoting the function of ALOX15 and inducing ferroptosis (Liu and Gu, 2022). Recently, a new target gene of p53, independent phospholipase A2beta, was found to suppress ferroptosis by cleaving peroxidized lipids and releasing PUFAs from membrane PLs (Chen et al., 2021a). However, p53 also inhibits ferroptosis under certain circumstances, a process requiring the involvement of P21, a p53 transcriptional target that can inhibit cell cycle progression, thereby converting part of the raw materials necessary for the synthesis of nucleic acids to those required for NADPH and GSH and inhibiting ferroptosis (Tarangelo et al., 2018). Therefore, p53 may regulate ferroptosis through distinct mechanisms which need further investigation.
3 Potential role of ferroptosis in lymphoma
DLBCL is the most common type of adult non-Hodgkin’s lymphoma (NHL), accounting for nearly 30% of the cases of adult NHL wordwide (Beham-Schmid, 2017). DLBCL cell lines are susceptible to ferroptosis induced by system Xc-inhibitors because of their inability to use the trans-sulfuration pathway to convert methionine to cysteine (Gout et al., 2001; Sun et al., 2014). Ferroptosis slows down tumor growth in a DLBCL xenograft model (Deng et al., 2019). Yuko Kinowaki et al. (2018) assessed the expression of GPX4 by immunohistochemistry and found that the GPX4-positive group showed poor overall survival relative to the GPX4-negative group, indicating that GPX4 overexpression is an independent prognostic predictor of adverse prognosis in DLBCL. Moreover, the activation of ferroptosis participates in p53-mediated cell cycle arrest and cell death in B-cell lymphomas (Li et al., 2016). Hydroxyacyl-CoA dehydrogenase (HADHA) is involved in fatty acid beta-oxidation (FAO) and is overexpressed in high-grade lymphoma. Its overexpression indicates a poor prognosis in DLBCL(62). Recently, some studies have attempted to identify ferroptosis-related genes in DLBCL and elucidate a method to predict the prognosis of these patients, along with novel treatment strategies. Huan Chen et al. (2021b) performed a systematic study and identified a ferroptosis-related gene signature comprising 8 genes that could be used to divide DLBCL patients into high- and low-risk groups. Moreover, Junmei Weng et al. built a risk score model related to ferroptosis with 11 genes and revealed that the high-risk score group showed resistance to ibrutinib treatment, while ferroptosis inducer, acetaminophen, inhibited the expression of the high-risk genes in the DLBCL cell lines (Weng et al., 2022). Julie Devin et al. (2022) built the iron score-related model to identify patients with DLBCL showing poor prognosis who might benefit from treatment targeted to maintaining iron homeostasis. All these studies suggest that therapeutic drugs targeting ferroptosis might contribute to alleviating DLBCL.
Despite limited information, other types of lymphoma that have been studied in the light of ferroptosis, include adult T-cell leukemia/lymphoma, BL, and natural killer/T cell lymphoma (NKTCL) (Du et al., 2022). These lymphomas are highly invasive and treatment options are limited especially in patients older than 60 years. Ferroptosis inducers, especially artesunate, alleviate these types of lymphoma through different mechanisms (Wang et al., 2019; Ishikawa et al., 2020; He et al., 2022), thus providing perspectives for the development of novel therapeutic strategies. However, unlike DLBCL, basic research for elucidating the mechanism of ferroptosis on tumorigenesis of these lymphomas is lacking.
4 Therapeutic applications
Several ferroptosis inducers against lymphoma have shown good outcomes in animal models. A summary of ferroptosis inducers against lymphomas is shown in Figure 1.
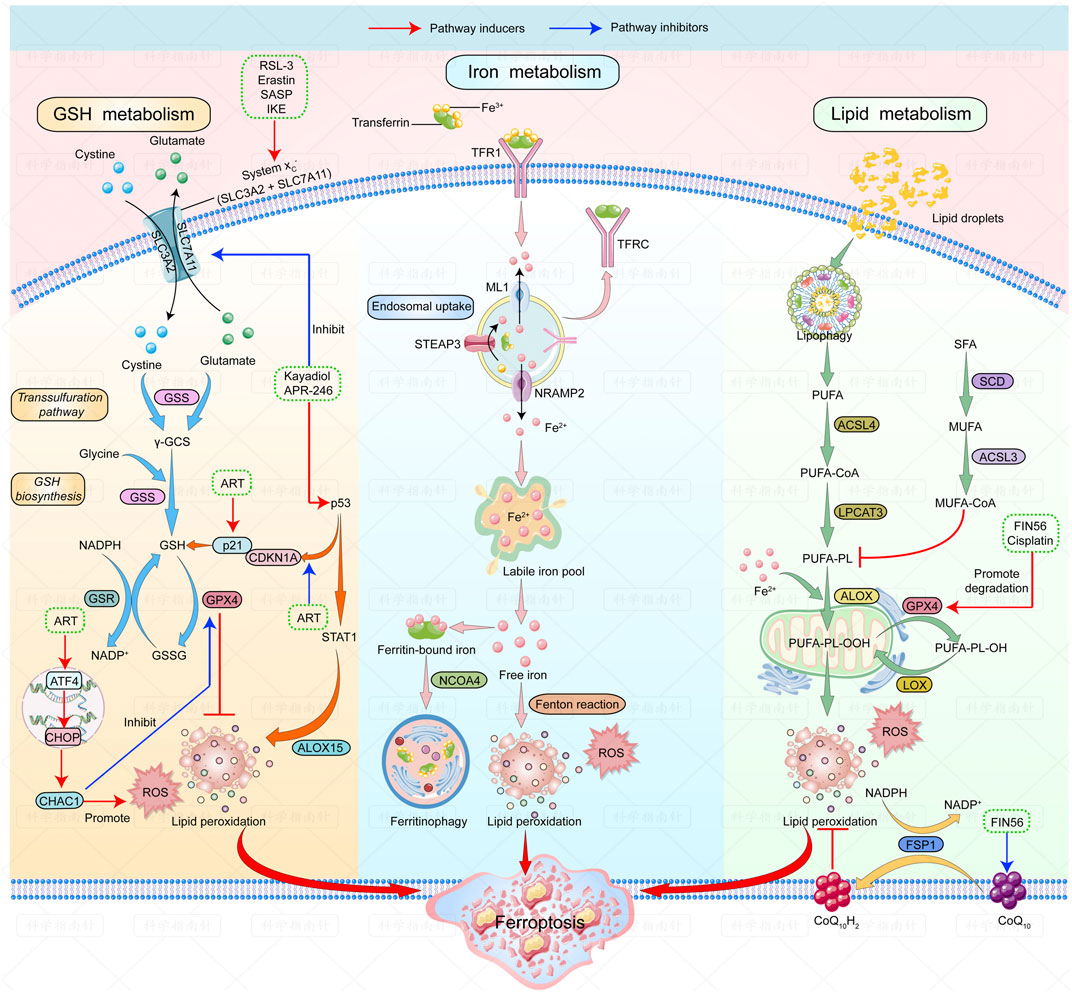
FIGURE 1. | Mechanisms of ferroptosis and ferroptosis inducers in lymphoma. The figure shows the regulatory pathways of ferroptosis can be divided into three categories. The first one is regulated by GSH/GPX4 pathway. The second one is the iron metabolism pathway that regulates the labile iron pool. The third category is the lipid metabolism pathways. Ferroptosis inducers that have been studied in lymphoma are listed: RSL-3, erastin, SASP and IKE can induce ferroptosis by inhibiting system Xc-; Kayadiol and APR-246 induce ferroptosis by both inhibiting system Xc- and promoting P53-P21 pathway; ART could induce ferroptosis by activating the ATF4-CHOP-CHAC1 pathway or by increasing p21 expression. Fin56 induces ferroptosis by promoting degradation of GPX4 and depleting endogenous antioxidant coenzyme Q10. Abbreviations: RSL-3, RAS-selective-lethal-3; SASP, safasalazine; IKE, Imidazole-ketone-erastin; ART, Artesunate.
4.1 Drugs targeting system Xc-
GSH metabolic pathways are classical targets of ferroptosis inducers. Erastin and its analog, RSL3, and sulfasalazine (SASP), have been widely applied as classical ferroptosis-inducing agents by targeting system Xc- and GPX4 (Xiao et al., 2015; Zhang et al., 2020). Imidazole-ketone-erastin (IKE), an improved erastin analog, can inhibit system Xc-at low concentrations (Ye et al., 2020). Zhang et al. (2019) demonstrated that IKE prevented tumor progression by inhibiting system Xc-in a DLBCL xenograft model through GSH depletion and lipid peroxidation. Erastin and RSL3-termed ferroptosis inducers can inhibit tumor growth in two DLBCL cell lines (Yang et al., 2014). In rats treated with sulfasalazine (SASP), a system Xc-inhibitor, the transplanted lymphoma growth shows marked reduction (Gout et al., 2003). As lymphoid cells cannot synthesize cysteine and since their growth is dependent on cysteine uptake from the microenvironment, SASP exerts inhibitory effects of cysteine secretion by somatic cells, causing cysteine starvation in lymphoma cells and inducing ferroptosis in vivo (Gout et al., 2001).
Since GSH metabolism is well understood, it is easy to speculate that the above drugs should be among the most promising ferroptosis inducers but more in vivo experiments are needed to validate their efficacy and safety in the future.
4.2 Drugs targeting p53-mediated ferroptosis
Drugs targeting the p53-mediated ferroptosis pathway, including eprenetapopt (or APR-246) and kayadiol, are new research hotspots. APR-246 reactivates the transcriptional activity of the mutant p53 by promoting its binding to target genes, thereby showing efficacy in p53-mutated tumors (Cluzeau et al., 2021; Mishra et al., 2022). APR-246 reactivates the transcriptional activity of mutant p53 by promoting their binding to its target genes and is effective in p53-mutated tumors (Cluzeau et al., 2021; Mishra et al., 2022). APR-246 can induce tumor protein p53 TP53-mutation-mediated cell death in DLBCL through ferroptosis by p53-dependent ferritinophagy (Hong et al., 2022). APR-246 can also induce ferroptosis in a p53-independent manner by binding to GSH or inhibiting antioxidant enzymes in acute myeloid leukemia but this has not been validated in lymphomas yet (Birsen et al., 2022). Thus, APR-246 may be a promising new therapeutic drug for DLBCL patients.
Kayadiol, a diterpenoid, shows a strong inhibitory effect on extranodal NKTCL cells. Kayadiol treatment triggers significant ferroptosis events through the p53-mediated pathway, including ROS accumulation and GSH depletion and reducing the expressions of SLC7A11 and GPX4. Moreover, Kayadiol also promotes the phosphorylation of p53, thus upregulating its protein expression. Hence, Kayadiol can be used as an alternative for NK/T cell lymphoma treatment, especially in cases of failure of initial therapeutic strategies (He et al., 2022).
Ferroptosis inducers involved in the p53-mediated pathway have been recently developed, and APR-246 has been studied in different types of hematologic malignancies, showing good results in clinical trials (Gout et al., 2003). It is expected that drugs targeting the p53 pathway may soon enter clinical trials for lymphoma.
4.3 Other ferroptosis inducers
Other types of drugs, including artesunate (ART), the FAO inhibitor, and dimethyl fumarate, can promote ferroptosis through different mechanisms. ART, a widely used antimalarial compound exerting cytotoxicity, induces ferroptosis in different types of lymphomas (Wang et al., 2019; Ishikawa et al., 2020; Chen et al., 2021c). By inducing lysosomal degradation of ferritin, ART increases intracellular labile iron and ROS levels, rendering cells sensitive to ferroptosis (Li, 2012; Chen et al., 2020b). Moreover, ART can inhibit the activation of signal transducer and activator of transcription 3 (STAT3), thereby downregulating the expression of GPX4, finally leading to ferroptosis (Chen et al., 2021c). Ning Wang et al. (2019) showed that ART could induce ferroptosis by activating the transcription factor 4-CEBP-homologous protein-cation transport regulator-like protein 1 (ATF4-CHOP-CHAC1) pathway, thus inhibiting GPX4 expression in BL cell lines. As mentioned above, the upregulation of fatty acid beta-oxidation is observed in high-grade lymphoma (Yamamoto et al., 2020). Inhibition of HADHA may disrupt the balance between saturated and unsaturated fatty acids that are subsequently supplied to PLs, in turn promoting the accumulation of polyunsaturated fatty acids in the cell membrane along with ferroptosis (Zhou et al., 2012a; Zhou et al., 2012b). Treatment with the FAO inhibitor, ranolazine, increases cell death in DLBCL (Sekine et al., 2022). Finally, dimethyl fumarate (DMF) potently and rapidly depletes GSH by inducing the succination of cysteine residues and induces lipid peroxidation, subsequently inducing ferroptosis, particularly in DLBCL (Schmitt et al., 2021).
With the continued and improved understanding of the mechanisms underlying ferroptosis, targeting different pathways to induce ferroptosis in lymphomas is emerging as a promising new option that has been validated in animal and in vitro experiments. Follow-up studies should focus on the effects and safety of these drugs in humans.
5 Conclusion and perspectives
Ferroptosis is an iron-dependent form of programmed cell death, a new direction for future research. It is regulated by several cellular metabolic pathways such as redox homeostasis, iron metabolism, mitochondrial activity, lipid, and amino acid metabolism, and ROS accumulation, as described above. Novel pathways such as p53-mediated signaling also play a complicated role in regulating of ferroptosis. Presumably, the p53-p21 pathway is activated to protect cells from damage when the external stimuli are small. On the contrary, other p53-mediated pathways are initiated to destroy cells via ferroptosis as stimuli exceed the threshold. Several non-classical regulatory pathways associated with ferroptosis are emerging such as mitochondrial VDACs (Skonieczna et al., 2017) and FSP1(45)-induced ferroptosis. Moreover, other kinds of programmed cell death such as autophagy can cause changes in ferritin levels, subsequently increasing the labile iron pool and inducing ferroptosis (Hou et al., 2016). Therefore, crosstalk between ferroptosis and other cell death modes exists but the exact underlying mechanisms remain elusive.
Ferroptosis was proposed for the first time in 2012, and since, it has emerged as an attractive target in tumor biology and cancer therapy. Certain types of tumors are more prone to ferroptosis due to their unique metabolic characteristics and high load of ROS, making ferroptosis a promising candidate for targeted therapy. The sensitivity of lymphoma to ferroptosis can be increased by drugs that regulate intracellular ROS production, iron metabolism, GPX4 levels, and other molecules. As most evidence has been obtained from animal models, there remains a long way toward the clinical application of ferroptosis inducers in lymphoma treatment. More research is needed in the future to uncover all the underlying mechanisms of ferroptosis in lymphoma.
The figure shows the regulatory pathways involved in ferroptosis. There are three main types of mechanisms involved in regulating ferroptosis: first, the regulation by the GSH/GPX4 pathway. The second is the iron metabolism pathway that regulates the labile iron pool. The third includes pathways related to lipid metabolism that affect lipid regulation. Ferroptosis inducers studied in lymphoma are as follows: RSL-3, Erastin, SASP, and IKE can induce ferroptosis by inhibiting system Xc-; Kayadiol and APR-246 induce ferroptosis by both inhibiting system Xc- and promoting the p53-p21 pathway; ART can induce ferroptosis by activating the ATF4-CHOP-CHAC1 pathway or by increasing the expression of P21. FIN56 induces ferroptosis by accelerating GPX4 degradation and depleting the endogenous antioxidant coenzyme, Q10.
Author contributions
YW and XH contributed to the conception and design of the work. QZ and TL drafted the manuscript. QQ prepared the figure. YW and XH substantively revised the manuscript. All authors read and approved the final manuscript.
Funding
This research was supported by the National Natural Science Foundation of China (81802504, 81872207), Sichuan Science and Technology Bureau (2019YFS0439, 2020JDJQ0067, 2020JDRC0118, 2021YJ0564, and 2022YFH0005), the Science and Technology Innovation Project of Chengdu, China (No. 2021-YF05-00225-SN), a Sichuan Medical Association grant (No. Q19037).
Conflict of interest
The authors declare that the research was conducted in the absence of any commercial or financial relationships that could be construed as a potential conflict of interest.
Publisher’s note
All claims expressed in this article are solely those of the authors and do not necessarily represent those of their affiliated organizations, or those of the publisher, the editors and the reviewers. Any product that may be evaluated in this article, or claim that may be made by its manufacturer, is not guaranteed or endorsed by the publisher.
References
Abrams, R. P., Carroll, W. L., and Woerpel, K. A. (2016). Five-membered ring peroxide selectively initiates ferroptosis in cancer cells. ACS Chem. Biol. 11 (5), 1305–1312. doi:10.1021/acschembio.5b00900
Beatty, A., Singh, T., Tyurina, Y. Y., Tyurin, V. A., Samovich, S., Nicolas, E., et al. (2021). Ferroptotic cell death triggered by conjugated linolenic acids is mediated by ACSL1. Nat. Commun. 12 (1), 2244. doi:10.1038/s41467-021-22471-y
Beham-Schmid, C. (2017). Aggressive lymphoma 2016: Revision of the WHO classification. Memo 10 (4), 248–254. doi:10.1007/s12254-017-0367-8
Bersuker, K., Hendricks, J. M., Li, Z., Magtanong, L., Ford, B., Tang, P. H., et al. (2019). The CoQ oxidoreductase FSP1 acts parallel to GPX4 to inhibit ferroptosis. Nature 575 (7784), 688–692. doi:10.1038/s41586-019-1705-2
Birsen, R., Larrue, C., Decroocq, J., Johnson, N., Guiraud, N., Gotanegre, M., et al. (2022). APR-246 induces early cell death by ferroptosis in acute myeloid leukemia. Haematologica 107 (2), 403–416. doi:10.3324/haematol.2020.259531
Bonam, S. R., Wang, F., and Muller, S. (2018). Autophagy: A new concept in autoimmunity regulation and a novel therapeutic option. J. Autoimmun. 94, 16–32. doi:10.1016/j.jaut.2018.08.009
Brown, C. W., Amante, J. J., Chhoy, P., Elaimy, A. L., Liu, H., Zhu, L. J., et al. (2019). Prominin2 drives ferroptosis resistance by stimulating iron export. Dev. Cell 51 (5), 575–586. e4. doi:10.1016/j.devcel.2019.10.007
Cao, J. Y., Poddar, A., Magtanong, L., Lumb, J. H., Mileur, T. R., Reid, M. A., et al. (2019). A genome-wide haploid genetic screen identifies regulators of glutathione abundance and ferroptosis sensitivity. Cell Rep. 26 (6), 1544–1556. e8. doi:10.1016/j.celrep.2019.01.043
Chapuy, B., Stewart, C., Dunford, A. J., Kim, J., Kamburov, A., Redd, R. A., et al. (2018). Molecular subtypes of diffuse large B cell lymphoma are associated with distinct pathogenic mechanisms and outcomes. Nat. Med. 24 (5), 679–690. doi:10.1038/s41591-018-0016-8
Chen, D., Chu, B., Yang, X., Liu, Z., Jin, Y., Kon, N., et al. (2021). iPLA2β-mediated lipid detoxification controls p53-driven ferroptosis independent of GPX4. Nat. Commun. 12 (1), 3644. doi:10.1038/s41467-021-23902-6
Chen, G. Q., Benthani, F. A., Wu, J., Liang, D., Bian, Z. X., and Jiang, X. (2020). Artemisinin compounds sensitize cancer cells to ferroptosis by regulating iron homeostasis. Cell Death Differ. 27 (1), 242–254. doi:10.1038/s41418-019-0352-3
Chen, H., He, Y., Pan, T., Zeng, R., Li, Y., Chen, S., et al. (2021). Ferroptosis-related gene signature: A new method for personalized risk assessment in patients with diffuse large B-cell lymphoma. Pharmgenomics. Pers. Med. 14, 609–619. doi:10.2147/PGPM.S309846
Chen, P. H., Wu, J., Ding, C. C., Lin, C. C., Pan, S., Bossa, N., et al. (2020). Kinome screen of ferroptosis reveals a novel role of ATM in regulating iron metabolism. Cell Death Differ. 27 (3), 1008–1022. doi:10.1038/s41418-019-0393-7
Chen, Y., Wang, F., Wu, P., Gong, S., Gao, J., Tao, H., et al. (2021). Artesunate induces apoptosis, autophagy and ferroptosis in diffuse large B cell lymphoma cells by impairing STAT3 signaling. Cell. Signal. 88, 110167. doi:10.1016/j.cellsig.2021.110167
Chen, Z., Jiang, J., Fu, N., and Chen, L. (2022). Targetting ferroptosis for blood cell-related diseases. J. Drug Target. 30 (3), 244–258. doi:10.1080/1061186X.2021.1971237
Cluzeau, T., Sebert, M., Rahmé, R., Cuzzubbo, S., Lehmann-Che, J., Madelaine, I., et al. (2021). Eprenetapopt Plus Azacitidine in TP53-Mutated Myelodysplastic Syndromes and Acute Myeloid Leukemia: A Phase II Study by the Groupe Francophone des Myélodysplasies (GFM). J. Clin. Oncol. 39 (14), 1575–1583. doi:10.1200/JCO.20.02342
Conrad, M., Kagan, V. E., Bayir, H., Pagnussat, G. C., Head, B., Traber, M. G., et al. (2018). Regulation of lipid peroxidation and ferroptosis in diverse species. Genes Dev. 32 (9-10), 602–619. doi:10.1101/gad.314674.118
Cronin, S. J. F., Woolf, C. J., Weiss, G., and Penninger, J. M. (2019). The role of iron regulation in immunometabolism and immune-related disease. Front. Mol. Biosci. 6, 116. doi:10.3389/fmolb.2019.00116
Davidson, A. J., and Wood, W. (2020). Igniting the spread of ferroptotic cell death. Nat. Cell Biol. 22 (9), 1027–1029. doi:10.1038/s41556-020-0570-4
Deng, R., Zhang, J., and Chen, J. (2019). lncRNA SNHG1 negatively regulates miRNA-101-3p to enhance the expression of ROCK1 and promote cell proliferation, migration and invasion in osteosarcoma. Int. J. Mol. Med. 43 (3), 1157–1166. doi:10.3892/ijmm.2018.4039
Devin, J., Cañeque, T., Lin, Y. L., Mondoulet, L., Veyrune, J. L., Abouladze, M., et al. (2022). Targeting cellular iron homeostasis with ironomycin in diffuse large B-cell lymphoma. Cancer Res. 82 (6), 998–1012. doi:10.1158/0008-5472.CAN-21-0218
Doll, S., Proneth, B., Tyurina, Y. Y., Panzilius, E., Kobayashi, S., Ingold, I., et al. (2017). ACSL4 dictates ferroptosis sensitivity by shaping cellular lipid composition. Nat. Chem. Biol. 13 (1), 91–98. doi:10.1038/nchembio.2239
Du, G., Zhang, Q., Huang, X., and Wang, Y. (2022). Molecular mechanism of ferroptosis and its role in the occurrence and treatment of diabetes. Front. Genet. 13, 1018829. doi:10.3389/fgene.2022.1018829
Fu, R., Guo, C., Wang, S., Huang, Y., Jin, O., Hu, H., et al. (2017). Podocyte activation of NLRP3 inflammasomes contributes to the development of proteinuria in lupus nephritis. Arthritis Rheumatol. 69 (8), 1636–1646. doi:10.1002/art.40155
Gout, P. W., Buckley, A. R., Simms, C. R., and Bruchovsky, N. (2001). Sulfasalazine, a potent suppressor of lymphoma growth by inhibition of the x(c)- cystine transporter: A new action for an old drug. Leukemia 15 (10), 1633–1640. doi:10.1038/sj.leu.2402238
Gout, P. W., Simms, C. R., and Robertson, M. C. (2003). In vitro studies on the lymphoma growth-inhibitory activity of sulfasalazine. Anticancer. Drugs 14 (1), 21–29. doi:10.1097/00001813-200301000-00004
Guo, C., Fu, R., Zhou, M., Wang, S., Huang, Y., Hu, H., et al. (2019). Pathogenesis of lupus nephritis: RIP3 dependent necroptosis and NLRP3 inflammasome activation. J. Autoimmun. 103, 102286. doi:10.1016/j.jaut.2019.05.014
Hangauer, M. J., Viswanathan, V. S., Ryan, M. J., Bole, D., Eaton, J. K., Matov, A., et al. (2017). Drug-tolerant persister cancer cells are vulnerable to GPX4 inhibition. Nature 551 (7679), 247–250. doi:10.1038/nature24297
Hao, S., Yu, J., He, W., Huang, Q., Zhao, Y., Liang, B., et al. (2017). Cysteine dioxygenase 1 mediates erastin-induced ferroptosis in human gastric cancer cells. Neoplasia (New York, NY) 19 (12), 1022–1032. doi:10.1016/j.neo.2017.10.005
He, C., Wang, C., Liu, H., and Shan, B. (2022). Kayadiol exerted anticancer effects through p53-mediated ferroptosis in NKTCL cells. BMC cancer 22 (1), 724. doi:10.1186/s12885-022-09825-5
Hong, Y., Ren, T., Wang, X., Liu, X., Fei, Y., Meng, S., et al. (2022). APR-246 triggers ferritinophagy and ferroptosis of diffuse large B-cell lymphoma cells with distinct TP53 mutations. Leukemia 36, 2269–2280. doi:10.1038/s41375-022-01634-w
Hou, W., Xie, Y., Song, X., Sun, X., Lotze, M. T., Zeh, H. J., et al. (2016). Autophagy promotes ferroptosis by degradation of ferritin. Autophagy 12 (8), 1425–1428. doi:10.1080/15548627.2016.1187366
Hu, J., Zhang, R., Chang, Q., Ji, M., Zhang, H., Geng, R., et al. (2022). p53: A regulator of ferroptosis induced by galectin-1 derived peptide 3 in MH7A cells. Front. Genet. 13, 920273. doi:10.3389/fgene.2022.920273
Ishikawa, C., Senba, M., and Mori, N. (2020). Evaluation of artesunate for the treatment of adult T-cell leukemia/lymphoma. Eur. J. Pharmacol. 872, 172953. doi:10.1016/j.ejphar.2020.172953
Jiang, X., Stockwell, B. R., and Conrad, M. (2021). Ferroptosis: Mechanisms, biology and role in disease. Nat. Rev. Mol. Cell Biol. 22 (4), 266–282. doi:10.1038/s41580-020-00324-8
Jing, S., Lu, Y., Zhang, J., Ren, Y., Mo, Y., Liu, D., et al. (2022). Levistilide a induces ferroptosis by activating the Nrf2/HO-1 signaling pathway in breast cancer cells. Drug Des. devel. Ther. 16, 2981–2993. doi:10.2147/DDDT.S374328
Kinowaki, Y., Kurata, M., Ishibashi, S., Ikeda, M., Tatsuzawa, A., Yamamoto, M., et al. (2018). Glutathione peroxidase 4 overexpression inhibits ROS-induced cell death in diffuse large B-cell lymphoma. Lab. Invest. 98 (5), 609–619. doi:10.1038/s41374-017-0008-1
Kraft, V. A. N., Bezjian, C. T., Pfeiffer, S., Ringelstetter, L., Müller, C., Zandkarimi, F., et al. (2020). GTP cyclohydrolase 1/tetrahydrobiopterin counteract ferroptosis through lipid remodeling. ACS Cent. Sci. 6 (1), 41–53. doi:10.1021/acscentsci.9b01063
Lee, H., Zandkarimi, F., Zhang, Y., Meena, J. K., Kim, J., Zhuang, L., et al. (2020). Energy-stress-mediated AMPK activation inhibits ferroptosis. Nat. Cell Biol. 22 (2), 225–234. doi:10.1038/s41556-020-0461-8
Li, J., Cao, F., Yin, H. L., Huang, Z. J., Lin, Z. T., Mao, N., et al. (2020). Ferroptosis: Past, present and future. Cell Death Dis. 11 (2), 88. doi:10.1038/s41419-020-2298-2
Li, T., Liu, X., Jiang, L., Manfredi, J., Zha, S., and Gu, W. (2016). Loss of p53-mediated cell-cycle arrest, senescence and apoptosis promotes genomic instability and premature aging. Oncotarget 7 (11), 11838–11849. doi:10.18632/oncotarget.7864
Li, Y. (2012). Qinghaosu (artemisinin): Chemistry and pharmacology. Acta Pharmacol. Sin. 33 (9), 1141–1146. doi:10.1038/aps.2012.104
Li, Y., Zeng, X., Lu, D., Yin, M., Shan, M., and Gao, Y. (2021). Erastin induces ferroptosis via ferroportin-mediated iron accumulation in endometriosis. Hum. Reprod. 36 (4), 951–964. doi:10.1093/humrep/deaa363
Liang, C., Zhang, X., Yang, M., and Dong, X. (2019). Recent progress in ferroptosis inducers for cancer therapy. Adv. Mat. 31 (51), e1904197. doi:10.1002/adma.201904197
Liu, Y., and Gu, W. (2022). p53 in ferroptosis regulation: the new weapon for the old guardian. Cell Death Differ. 29 (5), 895–910. doi:10.1038/s41418-022-00943-y
Magtanong, L., Ko, P. J., To, M., Cao, J. Y., Forcina, G. C., Tarangelo, A., et al. (2019). Exogenous monounsaturated fatty acids promote a ferroptosis-resistant cell state. Cell Chem. Biol. 26 (3), 420–432. e9. doi:10.1016/j.chembiol.2018.11.016
Mancuso, R. I., Foglio, M. A., and Olalla Saad, S. T. (2021). Artemisinin-type drugs for the treatment of hematological malignancies. Cancer Chemother. Pharmacol. 87 (1), 1–22. doi:10.1007/s00280-020-04170-5
Mao, C., Liu, X., Zhang, Y., Lei, G., Yan, Y., Lee, H., et al. (2021). DHODH-mediated ferroptosis defence is a targetable vulnerability in cancer. Nature 593 (7860), 586–590. doi:10.1038/s41586-021-03539-7
Mishra, A., Tamari, R., DeZern, A. E., Byrne, M. T., Gooptu, M., Chen, Y. B., et al. (2022). Eprenetapopt plus azacitidine after allogeneic hematopoietic stem-cell transplantation for TP53-mutant acute myeloid leukemia and myelodysplastic syndromes. J. Clin. Oncol., Jco2200181. doi:10.1200/JCO.22.00181
Nagata, S. (2018). Apoptosis and clearance of apoptotic cells. Annu. Rev. Immunol. 36, 489–517. doi:10.1146/annurev-immunol-042617-053010
Pandrangi, S. L., Chittineedi, P., Chikati, R., Lingareddy, J. R., Nagoor, M., and Ponnada, S. K. (2022). Role of dietary iron revisited: In metabolism, ferroptosis and pathophysiology of cancer. Am. J. Cancer Res. 12 (3), 974–985.
Patel, S. J., Frey, A. G., Palenchar, D. J., Achar, S., Bullough, K. Z., Vashisht, A., et al. (2019). A PCBP1-BolA2 chaperone complex delivers iron for cytosolic [2Fe-2S] cluster assembly. Nat. Chem. Biol. 15 (9), 872–881. doi:10.1038/s41589-019-0330-6
Ren, W., Zhao, W., Cao, L., and Huang, J. (2020). Involvement of the actin machinery in programmed cell death. Front. Cell Dev. Biol. 8, 634849. doi:10.3389/fcell.2020.634849
Sato, M., Kusumi, R., Hamashima, S., Kobayashi, S., Sasaki, S., Komiyama, Y., et al. (2018). The ferroptosis inducer erastin irreversibly inhibits system x(c)- and synergizes with cisplatin to increase cisplatin's cytotoxicity in cancer cells. Sci. Rep. 8 (1), 968. doi:10.1038/s41598-018-19213-4
Schmitt, A., Xu, W., Bucher, P., Grimm, M., Konantz, M., Horn, H., et al. (2021). Dimethyl fumarate induces ferroptosis and impairs NF-κB/STAT3 signaling in DLBCL. Blood 138 (10), 871–884. doi:10.1182/blood.2020009404
Segura, B. T., Bernstein, B. S., McDonnell, T., Wincup, C., Giles, I., Ripoll, V. M., et al. (2020). Damage accrual and mortality over long-term follow-up in 300 patients with systemic lupus erythematosus in a multi-ethnic British cohort. Rheumatol. Oxf. Engl. 59 (3), 524–533. doi:10.1093/rheumatology/kez292
Sekine, Y., Yamamoto, K., Kurata, M., Honda, A., Onishi, I., Kinowaki, Y., et al. (2022). HADHB, a fatty acid beta-oxidation enzyme, is a potential prognostic predictor in malignant lymphoma. Pathology 54 (3), 286–293. doi:10.1016/j.pathol.2021.06.119
Shah, R., Shchepinov, M. S., and Pratt, D. A. (2018). Resolving the role of lipoxygenases in the initiation and execution of ferroptosis. ACS Cent. Sci. 4 (3), 387–396. doi:10.1021/acscentsci.7b00589
Skonieczna, M., Cieslar-Pobuda, A., Saenko, Y., Foksinski, M., Olinski, R., Rzeszowska-Wolny, J., et al. (2017). The impact of DIDS-induced inhibition of voltage-dependent anion channels (VDAC) on cellular response of lymphoblastoid cells to ionizing radiation. Med. Chem. 13 (5), 477–483. doi:10.2174/1573406413666170421102353
Song, J., Liu, T., Yin, Y., Zhao, W., Lin, Z., Yin, Y., et al. (2021). The deubiquitinase OTUD1 enhances iron transport and potentiates host antitumor immunity. EMBO Rep. 22 (2), e51162. doi:10.15252/embr.202051162
Song, X., and Long, D. (2020). Nrf2 and ferroptosis: A new research direction for neurodegenerative diseases. Front. Neurosci. 14, 267. doi:10.3389/fnins.2020.00267
Stockwell, B. R. (2022). Ferroptosis turns 10: Emerging mechanisms, physiological functions, and therapeutic applications. Cell 185 (14), 2401–2421. doi:10.1016/j.cell.2022.06.003
Sun, T., Zhang, Y. S., Pang, B., Hyun, D. C., Yang, M., and Xia, Y. (2014). Engineered nanoparticles for drug delivery in cancer therapy. Angew. Chem. Int. Ed. Engl. 53 (46), 12320–12364. doi:10.1002/anie.201403036
Tang, D., Chen, X., Kang, R., and Kroemer, G. (2021). Ferroptosis: Molecular mechanisms and health implications. Cell Res. 31 (2), 107–125. doi:10.1038/s41422-020-00441-1
Tarangelo, A., Magtanong, L., Bieging-Rolett, K. T., Li, Y., Ye, J., Attardi, L. D., et al. (2018). p53 suppresses metabolic stress-induced ferroptosis in cancer cells. Cell Rep. 22 (3), 569–575. doi:10.1016/j.celrep.2017.12.077
Toyokuni, S., Ito, F., Yamashita, K., Okazaki, Y., and Akatsuka, S. (2017). Iron and thiol redox signaling in cancer: An exquisite balance to escape ferroptosis. Free Radic. Biol. Med. 108, 610–626. doi:10.1016/j.freeradbiomed.2017.04.024
Tsvetkov, P., Coy, S., Petrova, B., Dreishpoon, M., Verma, A., Abdusamad, M., et al. (2022). Copper induces cell death by targeting lipoylated TCA cycle proteins. Sci. (New York, NY) 375 (6586), 1254–1261. doi:10.1126/science.abf0529
Ursini, F., and Maiorino, M. (2020). Lipid peroxidation and ferroptosis: The role of GSH and GPx4. Free Radic. Biol. Med. 152, 175–185. doi:10.1016/j.freeradbiomed.2020.02.027
Viswanathan, V. S., Ryan, M. J., Dhruv, H. D., Gill, S., Eichhoff, O. M., Seashore-Ludlow, B., et al. (2017). Dependency of a therapy-resistant state of cancer cells on a lipid peroxidase pathway. Nature 547 (7664), 453–457. doi:10.1038/nature23007
Wang, N., Zeng, G. Z., Yin, J. L., and Bian, Z. X. (2019). Artesunate activates the ATF4-CHOP-CHAC1 pathway and affects ferroptosis in Burkitt's Lymphoma. Biochem. Biophys. Res. Commun. 519 (3), 533–539. doi:10.1016/j.bbrc.2019.09.023
Weng, J., Chen, L., Liu, H., Yang, X. P., and Huang, L. (2022). Ferroptosis markers predict the survival, immune infiltration, and ibrutinib resistance of diffuse large B cell lymphoma. Inflammation 45 (3), 1146–1161. doi:10.1007/s10753-021-01609-6
Wu, Z., Geng, Y., Lu, X., Shi, Y., Wu, G., Zhang, M., et al. (2019). Chaperone-mediated autophagy is involved in the execution of ferroptosis. Proc. Natl. Acad. Sci. U. S. A. 116 (8), 2996–3005. doi:10.1073/pnas.1819728116
Xiao, Y., Yu, F., Pang, L., Zhao, H., Liu, L., Zhang, G., et al. (2015). MeSiC: A model-based method for estimating 5 mC levels at single-CpG resolution from MeDIP-seq. Sci. Rep. 5, 14699. doi:10.1038/srep14699
Xu, C., Liu, Z., and Xiao, J. (2021). Ferroptosis: A double-edged sword in gastrointestinal disease. Int. J. Mol. Sci. 22 (22), 12403. doi:10.3390/ijms222212403
Yamamoto, K., Abe, S., Honda, A., Hashimoto, J., Aizawa, Y., Ishibashi, S., et al. (2020). Fatty acid beta oxidation enzyme HADHA is a novel potential therapeutic target in malignant lymphoma. Lab. Invest. 100 (3), 353–362. doi:10.1038/s41374-019-0318-6
Yang, W. S., SriRamaratnam, R., Welsch, M. E., Shimada, K., Skouta, R., Viswanathan, V. S., et al. (2014). Regulation of ferroptotic cancer cell death by GPX4. Cell 156 (1-2), 317–331. doi:10.1016/j.cell.2013.12.010
Ye, L. F., Chaudhary, K. R., Zandkarimi, F., Harken, A. D., Kinslow, C. J., Upadhyayula, P. S., et al. (2020). Radiation-induced lipid peroxidation triggers ferroptosis and synergizes with ferroptosis inducers. ACS Chem. Biol. 15 (2), 469–484. doi:10.1021/acschembio.9b00939
Yi, J., Zhu, J., Wu, J., Thompson, C. B., and Jiang, X. (2020). Oncogenic activation of PI3K-AKT-mTOR signaling suppresses ferroptosis via SREBP-mediated lipogenesis. Proc. Natl. Acad. Sci. U. S. A. 117 (49), 31189–31197. doi:10.1073/pnas.2017152117
Zhang, H. F., Klein Geltink, R. I., Parker, S. J., and Sorensen, P. H. (2022). Transsulfuration, minor player or crucial for cysteine homeostasis in cancer. Trends Cell Biol. 32 (9), 800–814. doi:10.1016/j.tcb.2022.02.009
Zhang, Y., Shi, J., Liu, X., Feng, L., Gong, Z., Koppula, P., et al. (2018). BAP1 links metabolic regulation of ferroptosis to tumour suppression. Nat. Cell Biol. 20 (10), 1181–1192. doi:10.1038/s41556-018-0178-0
Zhang, Y., Swanda, R. V., Nie, L., Liu, X., Wang, C., Lee, H., et al. (2021). mTORC1 couples cyst(e)ine availability with GPX4 protein synthesis and ferroptosis regulation. Nat. Commun. 12 (1), 1589. doi:10.1038/s41467-021-21841-w
Zhang, Y., Tan, H., Daniels, J. D., Zandkarimi, F., Liu, H., Brown, L. M., et al. (2019). Imidazole ketone erastin induces ferroptosis and slows tumor growth in a mouse lymphoma model. Cell Chem. Biol. 26 (5), 623–633. doi:10.1016/j.chembiol.2019.01.008
Zhang, Y., Zhang, Y., Hu, J., Zhang, J., Guo, F., Zhou, M., et al. (2020). scTPA: a web tool for single-cell transcriptome analysis of pathway activation signatures. Bioinformatics 36 (14), 4217–4219. doi:10.1093/bioinformatics/btaa532
Zhou, Q., Yang, L., Li, T., Wang, K., Huang, X., Shi, J., et al. (2022). Mechanisms and inhibitors of ferroptosis in psoriasis. Front. Mol. Biosci. 9, 1019447. doi:10.3389/fmolb.2022.1019447
Zhou, Z., Zhou, J., and Du, Y. (2012). Estrogen receptor alpha interacts with mitochondrial protein HADHB and affects beta-oxidation activity. Mol. Cell. Proteomics 11 (7), M111.011056. doi:10.1074/mcp.M111.011056
Zhou, Z., Zhou, J., and Du, Y. (2012). Estrogen receptor beta interacts and colocalizes with HADHB in mitochondria. Biochem. Biophys. Res. Commun. 427 (2), 305–308. doi:10.1016/j.bbrc.2012.09.047
Glossary
ART Artesunate
ACSL3 Acyl-coenzyme A synthetase long-chain family member 3
ACSL4 Acyl-coenzyme A synthetase long-chain family member 4
ALOX Arachidonate lipoxygenase
ALOX15 Arachidonate lipoxygenase 15
ATF4-CHOP-CHAC1 Activating transcription factor 4-CEBP-homologous protein-cation transport regulator-like protein 1
CoQ10 Coenzyme Q10
CoQ10H2 Reduced coenzyme Q10
FIN56 Ferroptosis-inducer-56
FSP1 Ferroptosis suppressor protein 1
GPX4 Glutathione peroxidase 4
GSH Glutathione
GSR Glutathione reductase
GSS Glutathione synthetase
GSSG Oxidized glutathione
IKE Imidazole-ketone-erastin
LIP Labile iron pool
LOX Lipoxygenase
LPCAT3 Lysophosphatidylcholine acyltransferase 3
ML1 Mucolipin 1
MUFA Monounsaturated fatty acids
MUFA-COA Monounsaturated fatty acids-coenzyme A
NADPH Nicotinamide adenine dinucleotide phosphate (NADPH)
NCOA4 Nuclear receptor activator 4
NRAMP2 Natural resistance-associated macrophage protein 2
PUFA Polyunsaturated fatty acids
PUFA-PL Polyunsaturated fatty acids of phospholipids
γ-GCS γ-glutamyl-cysteine synthetase
RSL-3 Rat sarcoma-selective-lethal-3
ROS Reactive oxygen species;
STAT1 Signal transducer and activator of transcription 1
SASP Safasalazine
SCD Stearoyl-CoA desaturase
SFA Saturated fatty acids
STEAP3 Six-transmembrane epithelial antigen of prostate 3
TFR1 Transferrin receptor 1
TFRC Transferrin receptor
Keywords: ferroptosis, iron metabolism, lipid Metabolism, reactive oxygen species (ROS), lymphoma, therapeutic applications, ferroptosis inducer cell death
Citation: Zhou Q, Li T, Qin Q, Huang X and Wang Y (2022) Ferroptosis in lymphoma: Emerging mechanisms and a novel therapeutic approach. Front. Genet. 13:1039951. doi: 10.3389/fgene.2022.1039951
Received: 08 September 2022; Accepted: 24 October 2022;
Published: 03 November 2022.
Edited by:
Jing Zhang, Beihang University, ChinaCopyright © 2022 Zhou, Li, Qin, Huang and Wang. This is an open-access article distributed under the terms of the Creative Commons Attribution License (CC BY). The use, distribution or reproduction in other forums is permitted, provided the original author(s) and the copyright owner(s) are credited and that the original publication in this journal is cited, in accordance with accepted academic practice. No use, distribution or reproduction is permitted which does not comply with these terms.
*Correspondence: Xiaobo Huang, ZHJodWFuZ3hiQDE2My5jb20=; Yi Wang, d195aTIwMjJAMTYzLmNvbQ==
†These authors share first authorship