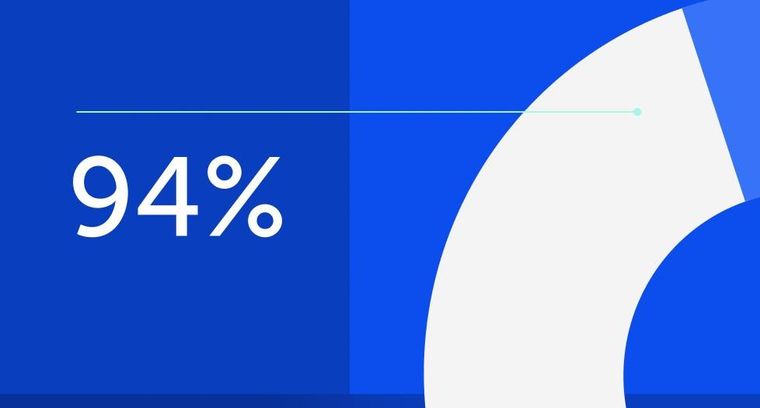
94% of researchers rate our articles as excellent or good
Learn more about the work of our research integrity team to safeguard the quality of each article we publish.
Find out more
ORIGINAL RESEARCH article
Front. Genet., 13 December 2021
Sec. Genomics of Plants and the Phytoecosystem
Volume 12 - 2021 | https://doi.org/10.3389/fgene.2021.784545
This article is part of the Research TopicInheritance and Improvement of Disease Resistance or Stress Tolerance for Triticeae CropsView all 11 articles
Wheat is one of the most important food crops in the world, with development of the grains directly determining yield and quality. Understanding grain development and the underlying regulatory mechanisms is therefore essential in improving the yield and quality of wheat. In this study, the developmental characteristics of the pericarp was examined in developing wheat grains of the new variety Jimai 70. As a result, pericarp thickness was found to be thinnest in grains at the top of the spike, followed by those in the middle and thickest at the bottom. Moreover, this difference corresponded to the number of cell layers in the pericarp, which decreased as a result of programmed cell death (PCD). A number of autophagy-related genes (ATGs) are involved in the process of PCD in the pericarp, and in this study, an increase in ATG8-PE expression was observed followed by the appearance of autophagy structures. Meanwhile, following interference of the key autophagy gene ATG8, PCD was inhibited and the thickness of the pericarp increased, resulting in small premature grains. These findings suggest that autophagy and PCD coexist in the pericarp during early development of wheat grains, with both processes increasing from the bottom to the top of the spike. Moreover, PCD was also found to rely on ATG8-mediated autophagy. The results of this study therefore provide a theoretical basis for in-depth studies of the regulatory mechanisms of wheat grain development.
Wheat, one of the most important cereal crops worldwide, is characterized by its process of grain development. Although grains in the middle spikelet are first to bloom, the upper grains mature first, followed by the middle, and then the lower grains. The wheat grain is a type of caryopsis, whereby the pericarp and episperm develop from the integument and are tightly integrated (Zhou et al., 2009). Development of the pericarp is closely related to grain yield and overall wheat quality. Developing from the ovary wall, it can be divided into the exocarp, mesocarp and endocarp (Xiong et al., 2013). The pericarp covers the seed tegument, and endosperm and embryo tissues of the grain (Brinton et al., 2017) and controls the water transport into the endosperm cavity (Wang and Fisher, 1994), the synthesis of organic compounds (Fujita and Taira, 1998; Foxon et al., 1990), and the temporal storage of starch (Yu et al., 2015).
It was previously suggested that development of the pericarp is a typical process of programmed cell death (PCD) (Pennell and Lamb, 1997; Zhou et al., 2009), a genetically-regulated process of cell suicide that results in the remobilization of cellular contents, nourishing new filial tissues, such as the embryo and endosperm, and providing space for grain filling (Radchuk et al., 2011; Dominguez and Cejudo, 2014). Meanwhile, autophagy is responsible for the delivery of cellular components to the lysosome/vacuole for subsequent degradation, especially under nutrient limitations and other stress conditions, thereby supporting cellular proteostasis and longevity (Ghosh et al., 2015). Autophagic processes mainly serve survival functions during cellular homeostasis, stress adaptation and immune responses, but have also been found to possess cell death-promoting activities (Bozhkov, 2018). Genetic suppression of autophagy in plants is correlated with an overall decrease in plant fitness, including reduced vegetative growth and fecundity, accelerated senescence and enhanced susceptibility to diverse types of stress (Bozhkov, 2018). However, the role of autophagy in regulating PCD in plants remains unknown and a subject of debate (Ustun et al., 2017).
In wheat, autophagy is involved in the regulation of various biotic and abiotic stresses. Under hypoxia, wheat roots can remove reactive oxygen species by autophagy, thus maintaining cell survival (Lin et al., 2021). Under salt stress, interfering of autophagy-related genes ATG2 or ATG7 causes PCD in leaves (Yue et al., 2021). Inhibition of autophagy can accelerate PCD of seedlings caused by drought (Li et al., 2019). Short-term waterlogging and cold stress promote autophagy of wheat root cells (Valitova et al., 2019; Zhou et al., 2021). Autophagy-related genes ATG4, ATG6, and ATG8 of wheat participate in the regulation of basic resistance to powdery mildew (Pei et al., 2014). ATG8 contributes to wheat resistance to stripe rust fungus by regulating cell death (Ma et al., 2012). However, it is unclear whether autophagy is involved in the regulation of wheat grain development.
In this study, we used the new wheat variety Jimai 70 to examine the regulation of autophagy on grain development and PCD in pericarp. Our data suggested that autophagy and PCD coexist in the development of the pericarp; and both processes increasing from the bottom to the top of the spike, which determines the thickness of the pericarp at the corresponding position.
Wheat cultivar Jimai 70 was developed by the Crop Research Institute, Shandong Academy of Agricultural Sciences, China. It possesses a number of elite traits, such as high and stable yield, lodging resistance, strong wind resistance, and slow stripe rust resistance.
Total RNA was extracted from the pericarp using RNAprep Pure Plant Kit (DP432, TIANGEN, Beijing, China) according to the manufacturer’s instructions. After determining RNA quality by electrophoresis on 1% agarose gel, 2 μg of RNA was reverse transcribed into cDNA using EasyScript One-Step gRNA Removal and cDNA Synthesis Super Mix (L20602, Transgen, Beijing, China). The resulting cDNA was then used as a template in the PCR reactions. qRT-PCR was performed using TransStart Tip Green qPCR SuperMix (L20803, Transgen) according to the manufacturer’s instructions in a real-time thermal cycler (LightCycler R 480 II, Roche, Basel, Switzerland). α-Tubulin was amplified for internal standardization. The experiments were repeated three times and the experimental data were statistically analyzed using the Student’s t-test. Relative expression data from the qRT-PCR experiments were obtained using the 2−ΔΔCT method (Ustun et al., 2017). Relevant primers were listed in Table 1.
TABLE 1. Primers used in this study. ATG (4, 6, 7, 8, 12) QRT primers were used for quantitative real-time reverse transcription-PCR (qRT-PCR) of autophagy-related genes (ATGs). RNAi primers of ATG8 were used to amplify the interference sequence of autophagy-related ATG8.
Synthetic peptide (5 mg) samples obtained from the ATG8 protein sequence were first coupled to Keyhole Limpet Hemocyanin. The coupling polypeptide was then used as an antigen to produce rabbit polyclonal antibodies (anti-ATG8 antibodies) as described previously (Liu et al., 2014). A partial cDNA sequence containing 90 - 750 bp of α-tubulin was then amplified with the selected primers α-tubulin F/R (Table 1), and inserted into the pGEX4T-AB1 plasmid. The recombinant plasmid was then transformed into competent Escherichia coli (BL21-DE3). The expressed α-tubulin was then obtained as a supernatant and purified before using the target protein as an antigen to produce rabbit polyclonal antibodies (anti-α-tubulin antibodies) as with the ATG8 antibodies.
Total proteins from the pericarp were extracted using Plant Total Protein Lysis Buffer (P1258, Applygen Technologies Inc., Beijing, China). The protein concentration was then measured according to the Bradford method (Bradford, 1976), and equal amounts (30 μg) of each sample were subjected to SDS-PAGE. Proteins were then electrophoretically transferred onto a nitrocellulose membrane and incubated with blocking buffer [2% skim milk powder dissolved in TBS (8.8 g NaCl, 5 ml of 2 M Tris-HCl, pH 7.6, and 995 ml of H2O)] at room temperature for 1 h. Rabbit source polyclonal antibody ATG8 or α-tubulin was then diluted to 1:500 in blocking buffer in TBS and incubated with the membrane at 4°C overnight. After washing, the membrane was incubated with secondary antibody (alkaline phosphatase conjugated goat anti-rabbit IgG diluted 1:10,000 in blocking buffer) (ZB2308, Zhong Shan Jin Qiao, Beijing, China) at room temperature for 2.5 h then the protein signal was visualized using an Alkaline Phosphatase Color Development Kit (C3206, Beyotime, Shanghai, China). Protein bands on the membrane were then analyzed using Image J software.
The barley stripe mosaic virus (BSMV)-based VIGS method was used to create gene knockdown plants (Ma et al., 2012; Dong et al., 2019). Briefly, a 283-bp fragment of wheat ATG8 from the conserved coding sequence was amplified and purified, with a same-sized fragment of GFP used as a control. The γ strand of BSMV was then digested in XmacI and fused with the ATG8 or GFP fragment to form the vectors BSMVγ-ATG8 and BSMVγ-GFP, respectively. BSMV-α was then linearized with MIuI, BSMV-β was linearized with SpeI, and BSMVγ-ATG8 and BSMVγ-GFP were linearized with BssHII then the linearized vectors were transcribed in vitro to produce 5ʹ-capped infectious BSMV RNA molecules using the RiboMAX Large-Scale RNA Production-T7 Kit (Promega, Madison, WI, United States), with a cap analog added to the transcription mixture. They were then mechanically infected with a 1:1:1 mixture of RNAα, RNAβ and RNAγ-ATG6, or RNAγ- GFP in 1 × GKP buffer (50 mM Gly, 30 mM K2HPO3, 1% bentonite and 1% kieselguhr). In the field, inoculation of BSMV was performed at the heading stage by inoculating 50 spikes with 20 µL of BSMV-ATG8 or BSMV-GFP transcript mixture, respectively.
Wheat seeds were removed from the spike then treated with 4% paraformaldehyde at 4°C overnight and gradient-dehydrated. The prepared grain tissues were then embedded in paraffin, cut into 7-µm sections, adhered to gelatin-coated glass slides, and dried at 37°C overnight. The slides were then dewaxed, gradient-dehydrated, and digested with 20 µM proteinase K at 37°C for 10 min before blocking in 2% BSA at 37°C for 30 min. Rabbit anti-ATG8 antibody was then added before incubating the slides at 47°C overnight. They were then washed three times with PBS before adding 1 µL secondary antibody (goat anti-rabbit-Alexa Fluor 555 antibody in 10 ml blocking buffer), and incubating at 37°C for 1 h. The nuclei were then stained with 4′, 6-diamidino-2-phenylindole (DAPI) (AnaSpec Inc., San Jose, CA, United States) at room temperature for 10 min. Fluorescence was observed with a fluorescence microscope (HT7700, Hitachi, Tokyo, Japan).
Wheat seeds were removed from the spike then treated with 4% paraformaldehyde at 4°C overnight. A TUNEL assay was then carried out as described previously (Li et al., 2019).
The paraffin-embedded seeds were transected down the middle, placed in xylene for 20 min, anhydrous ethanol for 5 min, and 75% alcohol for 5 min, and then washed with tap water three times for 30 s each time. The sections were then dyed in periodate dye solution for 10 min, rinsed with tap water then distilled water, and dyed in Schaeffer dye solution for 20 min before a final rinse in running water for 5 min. They were then stained in Hematoxylin solution for 3–5 min, rinsed with tap water, and differentiated in 1% HCl alcohol solution for 30 s. Following a final rinse in tap water, they were then placed in blue-back solution for 5 min before rinsing with running water. The prepared slices were examined under a microscope, and images were collected for analysis of cell structure.
It is well known that differences in light, temperature and nutrient supply cause wheat grains to mature at different rates in different positions on the spike. In this study, grains at the top of the spike were premature and small, while those in the middle matured faster and were big and full, and those on the bottom matured late and were also relatively small (Figure 1). The thickness of the pericarp was then examined in grains obtained from the top, middle and bottom of the spike at different development stages. Cross-sections revealed that the thickness was smallest at the top of the spike, followed by the middle, then the bottom, with a gradual decrease in thickness with increasing development (Figures 2A,B). These results indicate that pericarp thickness significantly differs at different positions on the spike and at different developmental stages.
FIGURE 1. Phenotypes of grains taken from the top (T), middle (M) and bottom (B) of the spike 25 days after flowering.
FIGURE 2. Cross-sections of grains from the top (T), middle (M) and bottom (B) of the spike 3, 5, 7 and 9 days after flowering. (A). Scale bars: 500 µm. (B). Statistical analysis of pericarp thickness at each position on the spike. Bars represent the mean ± SD of three independent experiments. Asterisks indicate a significant difference as determined by ANOVA, and different letters represent a significant difference between positions on the spike.
To further explore the differences in pericarp thickness, PAS staining was carried out. The results showed that pericarp samples obtained from the top of the spike had the least number of cell layers, followed by those in the middle, with most numerous layers in those from the bottom (Figure 3A). The number of cell layers also decreased gradually with increasing development (Figure 3B). TUNEL staining is often used to determine PCD in the pericarp (Dominguez et al., 2001), with green fluorescence indicating TUNEL-positive signals in the nuclei indicative of PCD (Zhou et al., 2009). Here, TUNEL signals were extremely intense in pericarp samples from the top of the spike, followed by those in the middle, with weakest signals in those from the bottom, and this trend was consistent for 3 – 9 days after flowering (Figure 4). These data suggest that the reduction in pericarp thickness was the result of a loss in cell layers induced by PCD.
FIGURE 3. (A). PAS staining showing grain morphology and structure. PE: pericarp, AL: aleurone layer, EN: endosperm. Scale bars: 50 µm. (B). Statistical analysis of cell layers in the pericarp of grains taken from the top (T), middle (M) and bottom (B) of the spike. Bars represent the mean ± SD of three independent experiments. Asterisks indicate a significant difference as determined by ANOVA., and different letters represent a significant difference between positions on the spike.
FIGURE 4. The process of programmed cell death (PCD) in the pericarp during early grain development as detected by TUNEL staining. (A). Images of grains sampled from the top (T), middle (M) and bottom (B) of the spike 3 days after flowering. PE: pericarp, AL: aleurone layer, EN: endosperm. TUNEL images indicate nuclei following PCD, while DAPI images indicate all nuclei. Scale bars: 200 µm. (B). Grains taken from the middle of the spike 3, 5, 7 and 9 days after flowering. Scale bars: 200 µm.
In order to determine the regulatory mechanism underlying PCD, we examined autophagy in the pericarp of developing grains obtained from different positions of the spike and different development stages. QRT-PCR showed that key autophagy-related genes (ATG4, ATG6, ATG7, ATG8, and ATG12) were highly expressed in samples from the top of the spike, followed by those in the middle, with weakest expression in those from the bottom (Figure 5). Moreover, highest peaks appeared 5 and 7 days after flowering (Figure 5). Western blotting also showed that the band of ATG8-PE was greatest in the top grains followed by those in the middle, with weakest signal in the bottom grains (Figures 6A–D). Moreover, expression of ATG8-PE was increasing for 3 - 7 days after flowering (Figures 6E,F). In addition, immunohistochemistry showed that most autophagy structures were observed in samples obtained from the top of the spike, followed by those in the middle, with least structures in those from the bottom (Figure 7). These results indicate that autophagy increases from the bottom to the top of the spoke, and is involved in the regulation of PCD in the pericarp during early stages of grain development.
FIGURE 5. The relative expression of autophagy-related genes in the pericarp of grains taken from the top (T), middle (M) and bottom (B) of the spike 3, 5, 7 and 9 days after flowering. α-Tubulin was used as an internal reference, and the relative expression was calculated using the 2−ΔΔT method. Asterisks indicate significant differences as determined by ANOVA, and different letters represent significant differences between columns.
FIGURE 6. Relative expression of ATG8-PE as detected by Western blotting. (A). Specific detection of ATG8 polyclonal antibody on 15% SDS gel. (B). Specific detection of α-tubulin polyclonal antibody on 12.5% SDS gel. (C). Relative expression of ATG8-PE in grains taken from the top (T), middle (M), and bottom (B) of the spike 3 days after flowering. (D). Statistical analysis of ATG8-PE expression at each position on the spike. (E). Relative expression of ATG8-PE at 3, 5, 7 and 9 days (D) after flowering. (F). Statistical analysis of ATG8-PE expression at each development stage. β-actin was used as a standard. Data represent the mean ± SD of three independent experiments. Asterisks indicate a significant difference as determined by ANOVA. Different letters represent significant differences between columns.
FIGURE 7. Immunohistochemical analysis of autophagy structures in grains taken from the top (T), middle (M) and bottom (B) of the spike. Short arrows indicate autophagy structures (brown indicate pericarp autophagy structures, white indicate endosperm autophagy structures). Grains were obtained 3 days after flowering, then the pericarp was stained with DAPI and anti-ATG8 antibody followed by Alexa 555-labeled secondary antibody. PE: pericarp, AL: aleurone layer, EN: endosperm; Scale bars: 200 µm.
In order to determine the effect of autophagy on PCD, we carried out knockdown of the key autophagy gene (ATG8). The results showed that interference of ATG8 (Figures 8C,D) resulted in a significant increase in the thickness of the pericarp (Figures 8A,B) and the number of cell layers (Figures 8E,F), with an obvious delay in the process of PCD (Figure 8G). These findings indicate that PCD in the pericarp cells of developing wheat grains is dependent on ATG8-mediated autophagy.
FIGURE 8. Morphology of the grains after knockdown of ATG8. (A). Cross sections of grains sampled five and 7 days after flowering following ATG8 knockdown. dsGFP was used as a control. PE: pericarp, AL: aleurone layer, EN: endosperm; Scale bars: 500 µm. (B). Statistical analysis of pericarp thickness following ATG8 knockdown. Asterisks indicate a significant difference based on the Student’s t-test, p < 0.05. (C). Knockdown efficiency of ATG8 as determined by Western blotting. α-tubulin was used as the internal reference. (D). Statistical analysis of the knockdown efficiency of ATG8. Asterisks indicate a significant difference based on the Student’s t-test, p < 0.05. (E). PAS staining 7 days after flowering showing the morphology and structure of the grains following ATG8 knockdown. Scale bars: 20 μm. (F). Statistical analysis of pericarp cell layers following ATG8 knockdown. Bars represent the mean ± SD of three independent experiments. Asterisks indicate a significant difference according to the Student’s t-test, p < 0.05. (G). TUNEL staining 7 days after flowering showing the morphology and structure of the grains following ATG8 knockdown. Scale bars: 50 μm.
To further examine the effect of autophagy, changes in the wheat phenotype were also examined following knockdown of ATG8. The results showed that interference resulted in earlier maturation by 4 days (Figures 9A,B), with smaller, less full grains (Figure 9C).
FIGURE 9. Phenotype of wheat following ATG8 knockdown. (A). The phenotype of wheat in the field 25 days after flowering following ATG8 knockdown. (B). The phenotype of the spike 25 days after flowering. (C). mature grains following ATG8 knockdown.
PCD is central to the development, homeostasis, and integrity of multi-cellular organisms (Ameisen, 2002). In plant cells, extensive chromatin condensation and degradation of nuclear DNA is one of the most conspicuous features of cells undergoing PCD (Latrasse et al., 2016). For example, during the early development of barley grains, PCD in the pericarp cells provides space and nutrients for subsequent grain filling (Radchuk et al., 2018). Meanwhile, autophagy is responsible for degrading unnecessary components and redistributing nutrients, thereby ensuring normal grain development (Li et al., 2015; Masclaux-Daubresse et al., 2017; Di Berardino et al., 2018). The process of autophagy begins with the formation of a phagophore with a double-membrane cup-shaped structure, which expands to form a double-membrane vesicle called an autophagosome. Upon completion, the autophagosome docks and fuses with the vacuole for cargo degradation (Feng et al., 2014) then the resulting breakdown products are released back into the cytosol to maintain nutrient and energy homeostasis (Yin et al., 2016). However, whether or not autophagy is involved in regulating PCD in the pericarp of developing wheat grains, and the underlying regulatory mechanism, remains unknown. Our data suggest that autophagy is indeed involved, with repression of ATG8-mediated autophagy seeming to delay PCD, resulting in an increased thickness and number of cell layers within the pericarp.
High temperatures after anthesis can significantly reduce the grain weight in wheat, thereby causing a reduction in yield (Zahedi et al., 2003; Djanaguiraman et al., 2020). However, grains in different positions of the spike are affected by environmental stress to a differing degree (Yu et al., 2014; Li et al., 2016). Light and temperature stress have the most serious effect on grains at the top of the spike, followed by those in the middle, and lastly, those on the bottom (Steinmeyer et al., 2013). Top grains therefore reach maturity earlier and are smaller in size, while bottom grains mature later, but are also relatively small due to the lack of light and insufficient supply of nutrients. It was previously reported that stress promotes autophagy and PCD in plants (Bassham et al., 2006; Kabbage et al., 2017; Chua et al., 2019). Pericarp and endosperm PCD are necessary for grain maturation (Dominguez and Cejudo, 2014). In this study, both processes occurred most strongly in the pericarp of grains at the top of the spike, followed by those in the middle, and lastly, to a weaker degree, those at the bottom. The top-to-bottom maturation sequence of wheat grain and its peel is actually a process of abiotic stress that causes wheat autophagy and PCD.
Plant development requires specific cells to be eliminated in a predictable and genetically regulated manner referred to as programmed cell death (PCD). However, the target cells do not merely die but they also undergo autophagy to degrade their cellular corpses (Escamez and Tuominen, 2017). In plants, controversy remains over the regulatory effect of autophagy on PCD. One viewpoint is that autophagy negatively regulates PCD; for example, mutation of autophagy was found to result in leaf senescence in Arabidopsis (Hanaoka et al., 2002), and autophagy in plants was found to eliminate reactive oxygen species induced by various abiotic stresses (Avin-Wittenberg, 2019). Meanwhile, the opposing view suggests that autophagy promotes PCD; for example, mutation of autophagy caused degradation of rice pollen tapetum (Kurusu et al., 2014), and autophagic components contributed to hypersensitive cell death in Arabidopsis (Hofius et al., 2009). In this study, however, the characteristics of autophagy and PCD coexisted in the pericarp during early development of the wheat grains, providing an ideal model for studies on the relationship between autophagy and PCD.
Autophagy occurs not only in the pericarp, but also in the embryo and endosperm of grain (Steinmeyer et al., 2013). At the whole-plant level, autophagy is an essential process for nutrient remobilization from leaves to seeds, and is fundamental for seed filling (Avin-Wittenberg, 2019). In ATG8-RNAi lines of rice, autophagic activity was slightly inhibited, grain yield and quality were reduced, and grains matured early (Fan et al., 2020). In our study, knockdown of wheat ATG8 also resulted in early maturity and smaller grains. The main reason is that inhibition of ATG8-mediated autophagy leads to the decrease of nutrient recycling into wheat grains. So, it can be inferred that other spike positions will also show the corresponding phenomena of smaller grains and early maturity after ATG8 interference.
The ATG8 gene is an evolutionarily conserved gene that is expressed in various plant tissues (Boycheva Woltering and Isono, 2020). In rice, ATG8 interference lines exhibited abnormal roots, a reduced number of grains per panicle, and other unfavorable agronomic traits (Fan et al., 2020). It is therefore difficult to rule out the negative effects of ATG8 knockout on other agronomic traits in analysis of grain phenotypes. In this study, we therefore carried our BSMV-mediated transient interference of ATG8 to minimize interference of other agronomic traits. As a result, PCD was weakened, and the thickness of the pericarp increased, suggesting strong dependency on ATG8-mediated autophagy, and a positive regulatory effect. Overall, the findings suggest that positive and negative regulation of PCD by autophagy mainly depends on the types of plant tissue and stress. Autophagy is dependent on a set of autophagy-related (ATG) proteins, of which the ubiquitin-like protein ATG8 plays a central role, functioning in autophagosome formation, and mediating membrane tethering, elongation and fusion (Nakatogawa et al., 2007). Upon autophagy activation, ATG8 undergoes lipidation to generate a membrane-bound ATG8-phosphatidylethanolamine (ATG8-PE) conjugate that localizes on growing phagophores and autophagosomes. ATG8 proteins are therefore often used as reliable markers to assess the induction and progression of autophagy (Yoshimoto et al., 2004). In this study, ATG8 was required for PCD in the pericarp of developing wheat grains. ATG8 lipidation occurs in both the outer and inner membrane of the phagophore, the precursor to autophagosomes, and is involved in autophagosome formation, as well as the recognition of specific cargo specifically targeted for autophagy (Kellner et al., 2017). These cargo receptors then interact with ATG8 proteins via short peptide motifs known as AIMs (ATG8-family interacting motifs) (Fracchiolla et al., 2017). Vacuolar processing enzymes (VPEs), a class of conserved cysteine proteases, are also involved in plant PCD (Hatsugai et al., 2004; Rojo et al., 2004). For example, VPE4 in barley is required for PCD in the pericarp during grain development (Radchuk et al., 2018), while VPE1 in tomato is translocated to the vacuole through the autophagy pathway, co-localizing with ATG8 in the autophagosomes and autolysosomes to induce PCD (Teper-Bamnolker et al., 2020). However, whether VPE1 interacts with ATG8, and is transported to the vacuole to exert its function on PCD requires further clarification.
The findings of this study suggest that autophagy and PCD coexist in the pericarp during the early development of wheat grains. Moreover, autophagy and PCD increased from the bottom to the top of the spike, and PCD was dependent on ATG8-mediated autophagy. Meanwhile, following knockdown of ATG8, the thickness of the pericarp increased, resulting in small premature grains. Overall, this dependence between autophagy and PCD determines the early development of grain pericarp.
The datasets presented in this study can be found in online repositories. The names of the repository/repositories and accession number(s) can be found below: https://www.ncbi.nlm.nih.gov/genbank/, KF294798.1 https://www.ncbi.nlm.nih.gov/genbank/, AM075827.1 https://www.ncbi.nlm.nih.gov/genbank/, KF294804.1 https://www.ncbi.nlm.nih.gov/genbank/, FJ750848.1 https://www.ncbi.nlm.nih.gov/genbank/, KF294819.1 https://www.ncbi.nlm.nih.gov/genbank/, U76558.1.
Y-BL performed most of the experiments. MY, D-ZC, FZ-G, Q-QF, and CH analyzed the data. X-XS and X-SC conceived and coordinated the study and edited the paper. All authors reviewed the results and approved the final version of the manuscript. All co-authors have checked and confirmed their contribution statement.
This work was supported by grants from the National Natural Science Foundation of China (32001542), Shandong Natural Science Foundation (ZR2020QC114), Open Project of State Key Laboratory for Wheat and Maize Engineering (2018LYZWS06) and Innovation Project of Shandong Academy of Agricultural Sciences Key Techniques of Crop Breeding (CXGC 2021A09).
The authors declare that the research was conducted in the absence of any commercial or financial relationships that could be construed as a potential conflict of interest.
All claims expressed in this article are solely those of the authors and do not necessarily represent those of their affiliated organizations, or those of the publisher, the editors and the reviewers. Any product that may be evaluated in this article, orclaim that may be made by its manufacturer, is not guaranteed or endorsed by the publisher.
Ameisen, J. C. (2002). On the Origin, Evolution, and Nature of Programmed Cell Death: a Timeline of Four Billion Years. Cell Death Differ 9, 367–393. doi:10.1038/sj.cdd.4400950
Avin-Wittenberg, T. (2019). Autophagy and its Role in Plant Abiotic Stress Management. Plant Cel Environ 42, 1045–1053. doi:10.1111/pce.13404
Bassham, D. C., Laporte, M., Marty, F., Moriyasu, Y., Ohsumi, Y., Olsen, L. J., et al. (2006). Autophagy in Development and Stress Responses of Plants. Autophagy 2, 2–11. doi:10.4161/auto.2092
Boycheva Woltering, S., and Isono, E. (2020). Knowing when to Self-Eat - Fine-Tuning Autophagy through ATG8 Iso-Forms in Plants. Front. Plant Sci. 11, 579875. doi:10.3389/fpls.2020.579875
Bozhkov, P. V. (2018). Plant Autophagy: Mechanisms and Functions. J. Exp. Bot. 69, 1281–1285. doi:10.1093/jxb/ery070
Bradford, M. M. (1976). A Rapid and Sensitive Method for the Quantitation of Microgram Quantities of Protein Utilizing the Principle of Protein-Dye Binding. Anal. Biochem. 72, 248–254. doi:10.1016/0003-2697(76)90527-3
Brinton, J., Simmonds, J., Minter, F., Leverington‐Waite, M., Snape, J., and Uauy, C. (2017). Increased Pericarp Cell Length Underlies a Major Quantitative Trait Locus for Grain Weight in Hexaploid Wheat. New Phytol. 215, 1026–1038. doi:10.1111/nph.14624
Chua, A., Fitzhenry, L., and Daly, C. T. (2019). Sorting the Wheat from the Chaff: Programmed Cell Death as a Marker of Stress Tolerance in Agriculturally Important Cereals. Front. Plant Sci. 10, 1539. doi:10.3389/fpls.2019.01539
Di Berardino, J., Marmagne, A., Berger, A., Yoshimoto, K., Cueff, G., Chardon, F., et al. (2018). Autophagy Controls Resource Allocation and Protein Storage Accumulation in Arabidopsis Seeds. J. Exp. Bot. 69, 1403–1414. doi:10.1093/jxb/ery012
Djanaguiraman, M., Narayanan, S., Erdayani, E., and Prasad, P. V. V. (2020). Effects of High Temperature Stress during Anthesis and Grain Filling Periods on Photosynthesis, Lipids and Grain Yield in Wheat. BMC Plant Biol. 20, 268. doi:10.1186/s12870-020-02479-0
Domínguez, F., and Cejudo, F. J. (2014). Programmed Cell Death (PCD): an Essential Process of Cereal Seed Development and Germination. Front. Plant Sci. 5, 366. doi:10.3389/fpls.2014.00366
Domínguez, F., Moreno, J., and Cejudo, F. J. (2001). The Nucellus Degenerates by a Process of Programmed Cell Death during the Early Stages of Wheat Grain Development. Planta 213, 352–360. doi:10.1007/s004250000517
Dong, J., Zheng, Y., Fu, Y., Wang, J., Yuan, S., Wang, Y., et al. (2019). PDIL1-2 Can Indirectly and Negatively Regulate Expression of the AGPL1 Gene in Bread Wheat. Biol. Res. 52, 56. doi:10.1186/s40659-019-0263-2
Escamez, S., and Tuominen, H. (2017). Contribution of Cellular Autolysis to Tissular Functions during Plant Development. Curr. Opin. Plant Biol. 35, 124–130. doi:10.1016/j.pbi.2016.11.017
Fan, T., Yang, W., Zeng, X., Xu, X., Xu, Y., Fan, X., et al. (2020). A Rice Autophagy Gene OsATG8b Is Involved in Nitrogen Remobilization and Control of Grain Quality. Front. Plant Sci. 11, 588. doi:10.3389/fpls.2020.00588
Feng, Y., He, D., Yao, Z., and Klionsky, D. J. (2014). The Machinery of Macroautophagy. Cell Res 24, 24–41. doi:10.1038/cr.2013.168
Foxon, G. A., Catt, L., and Keeling, P. L. (1990). Starch Synthesis in Developing Wheat Grain : The Effect of Light on Endosperm Starch Synthesis In Vitro and In Vivo. Planta 181, 104–108. doi:10.1007/BF00202331
Fracchiolla, D., Sawa-Makarska, J., and Martens, S. (2017). Beyond Atg8 Binding: The Role of AIM/LIR Motifs in Autophagy. Autophagy 13, 978–979. doi:10.1080/15548627.2016.1277311
Fujita, N., and Taira, T. (1998). A 56-kDa Protein Is a Novel Granule-Bound Starch Synthase Existing in the Pericarps, Aleurone Layers, and Embryos of Immature Seed in Diploid Wheat ( Triticum Monococcum L.). Planta 207, 125–132. doi:10.1007/s004250050464
Ghosh, A., Jana, M., Modi, K., Gonzalez, F. J., Sims, K. B., Berry-Kravis, E., et al. (2015). Activation of Peroxisome Proliferator-Activated Receptor α Induces Lysosomal Biogenesis in Brain Cells. J. Biol. Chem. 290, 10309–10324. doi:10.1074/jbc.m114.610659
Hanaoka, H., Noda, T., Shirano, Y., Kato, T., Hayashi, H., Shibata, D., et al. (2002). Leaf Senescence and Starvation-Induced Chlorosis Are Accelerated by the Disruption of an Arabidopsis Autophagy Gene. Plant Physiol. 129, 1181–1193. doi:10.1104/pp.011024
Hatsugai, N., Kuroyanagi, M., Yamada, K., Meshi, T., Tsuda, S., Kondo, M., et al. (2004). A Plant Vacuolar Protease, VPE, Mediates Virus-Induced Hypersensitive Cell Death. Science 305, 855–858. doi:10.1126/science.1099859
Hofius, D., Schultz-Larsen, T., Joensen, J., Tsitsigiannis, D. I., Petersen, N. H. T., Mattsson, O., et al. (2009). Autophagic Components Contribute to Hypersensitive Cell Death in Arabidopsis. Cell 137, 773–783. doi:10.1016/j.cell.2009.02.036
Kabbage, M., Kessens, R., Bartholomay, L. C., and Williams, B. (2017). The Life and Death of a Plant Cell. Annu. Rev. Plant Biol. 68, 375–404. doi:10.1146/annurev-arplant-043015-111655
Kellner, R., De La Concepcion, J. C., Maqbool, A., Kamoun, S., and Dagdas, Y. F. (2017). ATG8 Expansion: A Driver of Selective Autophagy Diversification? Trends Plant Sci. 22, 204–214. doi:10.1016/j.tplants.2016.11.015
Kurusu, T., Koyano, T., Hanamata, S., Kubo, T., Noguchi, Y., Yagi, C., et al. (2014). OsATG7 Is Required for Autophagy-dependent Lipid Metabolism in rice Postmeiotic Anther Development. Autophagy 10, 878–888. doi:10.4161/auto.28279
Latrasse, D., Benhamed, M., Bergounioux, C., Raynaud, C., and Delarue, M. (2016). Plant Programmed Cell Death from a Chromatin point of View. Exbotj 67, 5887–5900. doi:10.1093/jxb/erw329
Li, F., Chung, T., Pennington, J. G., Federico, M. L., Kaeppler, H. F., Kaeppler, S. M., et al. (2015). Autophagic Recycling Plays a central Role in maize Nitrogen Remobilization. Plant Cell 27, 1389–1408. doi:10.1105/tpc.15.00158
Li, Y. B., Cui, D. Z., Sui, X. X., Huang, C., Huang, C. Y., Fan, Q. Q., et al. (2019). Autophagic Survival Precedes Programmed Cell Death in Wheat Seedlings Exposed to Drought Stress. Int. J. Mol. Sci. 20, 5777. doi:10.3390/ijms20225777
Li, Y., Cui, Z., Ni, Y., Zheng, M., Yang, D., Jin, M., et al. (2016). Plant Density Effect on Grain Number and Weight of Two Winter Wheat Cultivars at Different Spikelet and Grain Positions. PLoS One 11, e0155351. doi:10.1371/journal.pone.0155351
Lin, Z., Wang, Y.-L., Cheng, L.-S., Zhou, L.-L., Xu, Q.-T., Liu, D.-C., et al. (2021). Mutual Regulation of ROS Accumulation and Cell Autophagy in Wheat Roots under Hypoxia Stress. Plant Physiol. Biochem. 158, 91–102. doi:10.1016/j.plaphy.2020.11.049
Liu, W., Cai, M.-J., Wang, J.-X., and Zhao, X.-F. (2014). In a Nongenomic Action, Steroid Hormone 20-hydroxyecdysone Induces Phosphorylation of Cyclin-dependent Kinase 10 to Promote Gene Transcription. Endocrinology 155, 1738–1750. doi:10.1210/en.2013-2020
Ma, M., Yan, Y., Huang, L., Chen, M., and Zhao, H. (2012). Virus-induced Gene-Silencing in Wheat Spikes and Grains and its Application in Functional Analysis of HMW-GS-Encoding Genes. BMC Plant Biol. 12, 141. doi:10.1186/1471-2229-12-141
Masclaux-Daubresse, C., Chen, Q., and Havé, M. (2017). Regulation of Nutrient Recycling via Autophagy. Curr. Opin. Plant Biol. 39, 8–17. doi:10.1016/j.pbi.2017.05.001
Nakatogawa, H., Ichimura, Y., and Ohsumi, Y. (2007). Atg8, a Ubiquitin-like Protein Required for Autophagosome Formation, Mediates Membrane Tethering and Hemifusion. Cell 130, 165–178. doi:10.1016/j.cell.2007.05.021
Pei, D., Zhang, W., Sun, H., Wei, X., Yue, J., and Wang, H. (2014). Identification of Autophagy-Related Genes ATG4 and ATG8 from Wheat (Triticum aestivum L.) and Profiling of Their Expression Patterns Responding to Biotic and Abiotic Stresses. Plant Cel Rep 33, 1697–1710. doi:10.1007/s00299-014-1648-x
Pennell, R. I., and Lamb, C. (1997). Programmed Cell Death in Plants. Plant Cell 9, 1157–1168. doi:10.1105/tpc.9.7.1157
Radchuk, V., Tran, V., Radchuk, R., Diaz-Mendoza, M., Weier, D., Fuchs, J., et al. (2018). Vacuolar Processing Enzyme 4 Contributes to Maternal Control of Grain Size in Barley by Executing Programmed Cell Death in the Pericarp. New Phytol. 218, 1127–1142. doi:10.1111/nph.14729
Radchuk, V., Weier, D., Radchuk, R., Weschke, W., and Weber, H. (2011). Development of Maternal Seed Tissue in Barley Is Mediated by Regulated Cell Expansion and Cell Disintegration and Coordinated with Endosperm Growth. J. Exp. Bot. 62, 1217–1227. doi:10.1093/jxb/erq348
Rojo, E., Martı́n, R., Carter, C., Zouhar, J., Pan, S., Plotnikova, J., et al. (2004). VPEγ Exhibits a Caspase-like Activity that Contributes to Defense against Pathogens. Curr. Biol. 14, 1897–1906. doi:10.1016/j.cub.2004.09.056
Steinmeyer, F. T., Lukac, M., Reynolds, M. P., and Jones, H. E. (2013). Quantifying the Relationship between Temperature Regulation in the Ear and Floret Development Stage in Wheat (Triticum aestivum L.) under Heat and Drought Stress. Funct. Plant Biol. 40, 700–707. doi:10.1071/fp12362
Teper-Bamnolker, P., Danieli, R., Peled-Zehavi, H., Belausov, E., Abu-Abied, M., Avin-Wittenberg, T., et al. (2020). Vacuolar Processing Enzyme Translocates to the Vacuole through the Autophagy Pathway to Induce Programmed Cell Death. Autophagy 17, 1–15. doi:10.1080/15548627.2020.1856492
Üstün, S., Hafrén, A., and Hofius, D. (2017). Autophagy as a Mediator of Life and Death in Plants. Curr. Opin. Plant Biol. 40, 122–130. doi:10.1016/j.pbi.2017.08.011
Valitova, J., Renkova, A., Mukhitova, F., Dmitrieva, S., Beckett, R. P., and Minibayeva, F. V. (2019). Membrane Sterols and Genes of Sterol Biosynthesis Are Involved in the Response of Triticum aestivum Seedlings to Cold Stress. Plant Physiol. Biochem. 142, 452–459. doi:10.1016/j.plaphy.2019.07.026
Wang, N., and Fisher, D. B. (1994). The Use of Fluorescent Tracers to Characterize the Post-Phloem Transport Pathway in Maternal Tissues of Developing Wheat Grains. Plant Physiol. 104, 17–27. doi:10.1104/pp.104.1.17
Xiong, F., Yu, X. R., Zhou, L., Wang, F., and Xiong, A. S. (2013). Structural and Physiological Characterization during Wheat Pericarp Development. Plant Cel Rep 32, 1309–1320. doi:10.1007/s00299-013-1445-y
Yin, Z., Pascual, C., and Klionsky, D. (2016). Autophagy: Machinery and Regulation. Microb. Cel 3, 588–596. doi:10.15698/mic2016.12.546
Yoshimoto, K., Hanaoka, H., Sato, S., Kato, T., Tabata, S., Noda, T., et al. (2004). Processing of ATG8s, Ubiquitin-like Proteins, and Their Deconjugation by ATG4s Are Essential for Plant Autophagy. Plant Cell 16, 2967–2983. doi:10.1105/tpc.104.025395
Yu, A., Li, Y., Ni, Y., Yang, W., Yang, D., Cui, Z., et al. (2014). Differences of Starch Granule Distribution in Grains from Different Spikelet Positions in winter Wheat. PLoS One 9, e114342. doi:10.1371/journal.pone.0114342
Yu, X., Li, B., Wang, L., Chen, X., Wang, W., Wang, Z., et al. (2015). Systematic Analysis of Pericarp Starch Accumulation and Degradation during Wheat Caryopsis Development. PLoS One 10, e0138228. doi:10.1371/journal.pone.0138228
Yue, J.-y., Wang, Y.-j., Jiao, J.-l., and Wang, H.-z. (2021). Silencing of ATG2 and ATG7 Promotes Programmed Cell Death in Wheat via Inhibition of Autophagy under Salt Stress. Ecotoxicology Environ. Saf. 225, 112761. doi:10.1016/j.ecoenv.2021.112761
Zahedi, M., Sharma, R., and Jenner, C. F. (2003). Effects of High Temperature on Grain Growth and on the Metabolites and Enzymes in the Starch-Synthesis Pathway in the Grains of Two Wheat Cultivars Differing in Their Responses to Temperature. Funct. Plant Biol. 30, 291–300. doi:10.1071/fp02205
Zhou, L.-L., Gao, K.-Y., Cheng, L.-S., Wang, Y.-L., Cheng, Y.-K., Xu, Q.-T., et al. (2021). Short-term Waterlogging-Induced Autophagy in Root Cells of Wheat Can Inhibit Programmed Cell Death. Protoplasma 258, 891–904. doi:10.1007/s00709-021-01610-8
Keywords: wheat, pericarp, autophagy, programmed cell death, autophagy-related genes
Citation: Li Y-B, Yan M, Cui D-Z, Huang C, Sui X-X, Guo FZ, Fan Q-Q and Chu X-S (2021) Programmed Degradation of Pericarp Cells in Wheat Grains Depends on Autophagy. Front. Genet. 12:784545. doi: 10.3389/fgene.2021.784545
Received: 28 September 2021; Accepted: 19 November 2021;
Published: 13 December 2021.
Edited by:
Pengtao Ma, Yantai University, ChinaReviewed by:
Guohao Han, Institute of Genetics and Developmental Biology (CAS), ChinaCopyright © 2021 Li, Yan, Cui, Huang, Sui, Guo, Fan and Chu. This is an open-access article distributed under the terms of the Creative Commons Attribution License (CC BY). The use, distribution or reproduction in other forums is permitted, provided the original author(s) and the copyright owner(s) are credited and that the original publication in this journal is cited, in accordance with accepted academic practice. No use, distribution or reproduction is permitted which does not comply with these terms.
*Correspondence: Feng Zhi Guo, ZmFucWluZ3FpQDE2My5jb20=; Qing-Qi Fan, bGtsZ2Z6MjAxNEAxNjMuY29t; Xiu-Sheng Chu, eHNjaHUyMDA3QHNpbmEuY29t
Disclaimer: All claims expressed in this article are solely those of the authors and do not necessarily represent those of their affiliated organizations, or those of the publisher, the editors and the reviewers. Any product that may be evaluated in this article or claim that may be made by its manufacturer is not guaranteed or endorsed by the publisher.
Research integrity at Frontiers
Learn more about the work of our research integrity team to safeguard the quality of each article we publish.