- Department of Anatomy, Physiology and Genetics, School of Medicine, Uniformed Services University of the Health Sciences, Bethesda, MD, United States
Non-coding RNAs (ncRNAs), notably microRNAs (miRNAs) and long noncoding RNAs (lncRNAs), have recently gained increasing consideration because of their versatile role as key regulators of gene expression. They adopt diverse mechanisms to regulate transcription and translation, and thereby, the function of the protein, which is associated with several major biological processes. For example, proliferation, differentiation, apoptosis, and metabolic pathways demand fine-tuning for the precise development of a specific tissue or organ. The deregulation of ncRNA expression is concomitant with multiple diseases, including lung diseases. This review highlights recent advances in the post-transcriptional regulation of miRNAs and lncRNAs in lung diseases such as asthma, chronic obstructive pulmonary disease, cystic fibrosis, and idiopathic pulmonary fibrosis. Further, we also discuss the emerging role of ncRNAs as biomarkers as well as therapeutic targets for lung diseases. However, more investigations are required to explore miRNAs and lncRNAs interaction, and their function in the regulation of mRNA expression. Understanding these mechanisms might lead to early diagnosis and the development of novel therapeutics for lung diseases.
Introduction
Non-coding RNAs (ncRNAs) are non-protein-coding RNA transcripts and were initially believed as “non-functional parts” and/or “junk RNAs” and/or “dark matter” of the human genome. But, the discoveries of the transcribed regions and protein-coding genes, i.e., approximately 85 and 2%, respectively, reveal that only a small portion of the human transcriptome encode for protein and the majority are non-protein-coding (Lander et al., 2001; Djebali et al., 2012; Hangauer et al., 2013; Jensen et al., 2013). This assessment has subverted the aforementioned conception and highlights the significance of ncRNAs, which leads to a paradigm shift and scientific revolution in RNA biology and regulation. Today, there is enormous evidence proving the function of ncRNAs as versatile key regulators of epigenetics, transcription, post-transcription, and translation (Cech and Steitz, 2014; Peschansky and Wahlestedt, 2014; Zhang et al., 2019). The pivotal role of ncRNAs in the regulation of nearly all biological activities, from tissue repair to organ development and immunity, is well-established. Consequently, deregulation in ncRNA networks has been associated with a broad spectrum of pathological conditions and human diseases including lung diseases (Taft et al., 2010; Esteller, 2011; Beermann et al., 2016; Groot et al., 2018; Bao et al., 2019; Bhatti et al., 2021).
Lung diseases are a leading public health concern and cause substantial morbidity and mortality, globally (Schluger and Koppaka, 2014; Glass and Rosenthal, 2018). Undoubtedly, this necessitates in-depth knowledge of the lung disease etiology and pathophysiology, with a focus on inventing more efficacious therapeutic approaches. In recent decades, several reports have established the association of ncRNAs in various lung diseases and their pivotal functions in lung development and homeostasis (Lu et al., 2018; Pattarayan et al., 2018; Wang et al., 2019), expediting a new paradigm for lung disease diagnosis, control, and treatment. Here, we provide a comprehensive overview of the post-transcriptional regulation of ncRNAs, with special emphasis on microRNAs (miRNAs) and long ncRNAs (lncRNAs), in lung diseases such as asthma, chronic obstructive pulmonary disease (COPD), cystic fibrosis (CF), and idiopathic pulmonary fibrosis (IPF). Alterations of miRNA and lncRNA expression level in the disease state compared to the normal state could be exploited to identify biomarkers and targets for drug development. Understanding how post-transcriptional mechanisms regulate lung diseases will lead to the development of candidate therapeutic targets for the early diagnosis and treatment of lung diseases.
MicroRNAs and Long ncRNAs
The ncRNA repertoire encompasses myriads of RNA species and according to their regulatory roles are broadly classified into two categories, housekeeping and regulatory ncRNAs. The regulatory ncRNAs further consist of diverse groups of ncRNAs with the two-utmost noteworthy, microRNAs (miRNAs, transcripts between 19 and 25 nucleotides) and long ncRNAs (lncRNAs, transcripts >200 nucleotides). MiRNAs generally negatively regulate gene expression in a sequence-specific way at the post-transcriptional stage either through the target messenger RNA (mRNA) cleavage and degradation, and/or by inhibition of translation. On the other hand, lncRNAs are divided into different types and regulate either negatively or positively each stage of gene expression via the interactions with DNA, RNA, or protein and through various mechanisms (Fatica and Bozzoni, 2014; Chew et al., 2018). The biogenesis, characteristics, types, and mechanism of action of miRNAs and lncRNAs have been described in multiple articles (Denli et al., 2004; Han et al., 2004; Du and Zamore, 2005; Kim, 2005; Bartel, 2009; Kugel and Goodrich, 2012; Fatica and Bozzoni, 2014; Quinn and Chang, 2016; Kopp and Mendell, 2018; Zhang et al., 2019; Statello et al., 2021). Collectively, both of these ncRNA species have an imperative role in development and homeostasis as well as in diseases.
Non-Coding RNAs in Lung Disease
Emerging evidence suggests that in the respiratory system, ncRNAs are accountable for normal lung development and maintenance of lung homeostasis. Thus, deregulation of miRNAs and lncRNAs causes pathophysiological alteration of the respiratory system leading to the initiation, progression, and development of various types of lung diseases. In the following sections, we have described the emerging roles and mechanistic functions of miRNA and lncRNA in various lung diseases such as asthma, chronic obstructive pulmonary disease (COPD), cystic fibrosis (CF), and idiopathic pulmonary fibrosis (IPF).
Asthma
Asthma is a multifaceted heterogeneous disease, primarily characterized by chronic inflammation, hyperresponsiveness, and transient airflow obstruction of the airways. The global increase in the incidence of asthma has been reported in all age groups, and approximately 300 million people are affected by asthma (Cevhertas et al., 2020; Stern et al., 2020). Therefore, the management of asthma as well as developing novel therapies is vital.
Several ncRNAs regulate airway inflammation and are associated with the pathophysiology of asthma. For example, upregulation of miR-221 and miR-485-5p are reported in the blood sample of asthmatic children (Liu et al., 2012). In a murine model of asthma, miR-221 and miR-485-5p regulate interleukin-5 (IL-5) by targeting sprouty-related protein with an EVH1 domain-2 (Spred-2), which negatively regulates the Ras/ERK pathway involved in a variety of cellular processes, including airway inflammation and hypersensitivity (Liu et al., 2012). Elevated level of miR-1248 has been reported in the serum of asthmatic patients and it induces increased expression of IL-5 and upregulation of Th2 cytokine through the direct interaction with IL-5 (Panganiban et al., 2012). The imbalance of Th1/Th2 cytokines has been found as a predominant factor associated with asthma, where increased expression of Th2 cytokines, mainly IL-4, IL-5, IL-9, and IL-13 promote the serum immunoglobulin E (IgE) and eosinophilia that stimulate a variety of cellular processes, including mucus hypersecretion, airway inflammation, and hypersensitivity (Ngoc et al., 2005; Zhu et al., 2016). On the other hand, Th1 cytokines, such as IFN-γ and IL-12, play an antagonist role in IgE synthesis as well as other Th2 responses. Thus, restoration of Th1/Th2 balance via inhibition of Th2 cytokines and activation of Th1 cytokines is one of the critical aspects in the treatment of asthma. One of the most studied miRNAs in asthma is miR-21. Several studies with asthmatic mice model and in asthmatic children indicate the upregulation of miR-21 negatively regulates IL-12p35, signal transducer and activator of transcription 4 (STAT4), phosphatase and tensin homolog deleted on chromosome 10 (PTEN), and histone deacetylase 2 (HDAC2), and positively regulates phosphoinositide 3-kinase (PI3K), which may promote increased expression of Th2 cytokines and inhibit Th1 cytokines expression (Lu et al., 2009; Wu S.-Q. et al., 2014; Wu XB. et al., 2014; Liu et al., 2015; Perry et al., 2015; Sawant et al., 2015; Elbehidy et al., 2016; Kim et al., 2017; Hammad Mahmoud Hammad et al., 2018). Thereby, the role of miR-21 in controlling the Th1/Th2 ratio, airway hypersensitivity, and cell proliferation and migration is established in different asthmatic models. In the bronchial epithelium of neutrophilic asthmatic, miR-629-3p is upregulated and induces neutrophil chemoattractant IL-8, which suggests its role in the airway neutrophilia and disease pathogenesis through the regulation of proinflammatory and wound-repair pathways (Maes et al., 2016). In lung tissues from allergic asthma patients and ovalbumin (OVA)-induced mice, upregulation of miR-943-3p and downregulation of its target secreted frizzled-related protein 4 (SFRP4) enhances airway inflammation progression and remodeling via the activation of Wingless/Integrase I (Wnt) signaling pathway (Shen et al., 2019). The importance of WNT signaling has been shown in the development of the organism, context-dependent transcription of targets genes, maintaining equilibrium among proliferation and differentiation of airway smooth muscle (ASM) progenitor cells, and asthma pathogenesis (Sharma et al., 2010; Choy et al., 2011; Wang et al., 2013; Carraro et al., 2014; Barreto-Luis et al., 2017).
The decreased expression of miR-181b-5p is reported in plasma and epithelium of eosinophilic asthmatic and it has been demonstrated that miR-181b-5p negatively regulates proinflammatory cytokines, IL-1β and C-C motif chemokine ligand (CCL)-11 (eotaxin-1) expression by binding to its target secreted phosphoprotein-1 (SPP-1), which is associated with the recruitment of eosinophils into airways (Huo et al., 2016). In the lungs of asthmatic mice, miR-20b promotes the elevation of CCL-2 concentration and accumulation of myeloid-derived suppressor cells, which suppresses the Th2 response and airway inflammation in a transforming growth factor-beta (TGF-β)-dependent manner (Ma et al., 2017a; Ma et al., 2017b). MiR-221-3p is downregulated in the airway epithelium and sputum of asthmatics and its downregulation suppresses inflammatory cytokine, chemokine CCL-24 (eotaxin-2) and CCL-26 (eotaxin-3) expression, which are involved in the migration of eosinophils into the airways, by inducing the expression of its target chemokine C-X-C motif ligand (CXCL)-17, an anti-inflammatory chemokine (Zhang K. et al., 2018). This suggests the protective role of miR-221-3p against airway eosinophilic inflammation. The reduced expression of miR-485 is observed in the mouse model of chronic asthma. Consistently, overexpression of miR-485 leads to reduced proliferation of airway smooth muscle cells (ASMCs) and induces apoptosis by targeting Smad ubiquitin regulatory factor 2 (Smurf2) (Wang et al., 2018). Smurf2 modulates the TGF-β/decapentaplegic homolog (Smads) signaling pathway, which is shown to be associated with the remodeling of the airway in asthma (Qu et al., 2012). The association of miR-142-3p with WNT signaling and maintaining equilibrium among proliferation and migration of ASMCs has been observed in bronchial biopsies of asthmatics (Bartel et al., 2018). In bronchial epithelial cells from asthmatic patients, decreased level of miR-744 induces cell proliferation by targeting TGF-ß1 and regulating the Smad3 pathway (Huang et al., 2019). The downregulation of miR-30a and upregulation of its target autophagy-related 5 (ATG5) is reported in lung tissues from asthmatic children and in mice treated with OVA, and promotes fibrogenesis, autophagic flux, and airway remodeling (Li et al., 2020).
In addition to miRNAs, lncRNAs are also associated with the regulation of airway inflammation and asthma. For example, in the rat model of asthma, upregulation of brain cytoplasmic RNA 1 (BCYRN1) lncRNA targets canonical transient receptor potential 1 (TRPC1), which is implicated in the pro-proliferative and pro-migratory role of BCYRN1 and induces proliferation and migration of ASMCs (Zhang XY. et al., 2016). TRPC1 has been reported as an important molecular counterpart of Ca2+ channels in ASMCs and as a critical component contraction and proliferation of vascular smooth muscle cells (Ong et al., 2003; Dietrich et al., 2006). A similar study, using rat model asthma, demonstrated that miR-150 downregulates BCYRN1 and reduces proliferation and migration of ASMCs (Zhang X.-y. et al., 2017). PVT1 lncRNA is upregulated in severe asthmatic patients who are insensitive to corticosteroids, and induces IL-6 expression and proliferation of ASMCs (Austin et al., 2017). The upregulation of TCF7 lncRNA and TIMMDC1 and the role of TCF7 in the regulation of TIMMDC1 expression and proliferation and migration of ASMCs have been established in asthmatics (Fan et al., 2019). Similarly, TUG1 and MALAT1 lncRNAs induces proliferation and migration of ASMCs via targeting miR-590-5p (TUG1/miR-590-5p/FGF1 axis) and miR-150 (miR-150-eIF4E/Akt signaling), respectively (Lin J. et al., 2019; Lin L. et al., 2019). The upregulation of the lncRNA antisense non-coding RNA in the INK4 locus (ANRIL)/miR-125a axis is found especially in the plasma of bronchial asthmatics at exacerbation (BA-E) compared to bronchial asthmatics at remission (BA-R) and control groups (Ye et al., 2020). Furthermore, there is a positive correlation between this regulatory axis and pro-inflammatory cytokines (TNF-α, IL-1β, IL-6, and IL-17) in bronchial asthmatics. A brief summary of ncRNAs, miRNAs and lncRNAs, associated with asthma are shown in Table 1. From these studies, it is clear that through the various mechanisms including post-transcriptional regulation miRNAs, lncRNAs and associated molecules play a pivotal role in the genesis and development of asthma. Further analyses of the function, and mechanism of action of ncRNAs will lead to therapeutic targets for asthma.
Chronic Obstructive Pulmonary Disease (COPD)
COPD is a heterogenous persistent lung disease, caused by progressive and irreversible airflow obstruction. COPD has a high rate of morbidity and mortality, accounting for 3.2 million deaths globally, and is considered the third leading cause of death (WHO, 2021). Among the environmental factors, the recurrent exposure of noxious particles and gas irritants such as cigarette smoke to the lungs are among the main causes of the development of COPD. However, genetic and epigenetic factors also play an important role in the pathogenesis of COPD, as this disease is reported in only 20% of smokers.
The involvement of ncRNAs in the pathogenesis and development of COPD is established by several studies. For example, upregulation of miR-15b and downregulation of its target SMAD7, which is an inhibitory SMAD in TGF-β signaling, is reported in lung tissues of COPD patients compared with smokers without obstruction, and thereby regulates TGF-β signaling pathway and pathogenesis of COPD (Ezzie et al., 2012). TGF-β is a profibrogenic cytokine and the impairment in TGF-β signaling in COPD patients induces fibrotic airway remodeling that could promote a decline in lung function (Morty et al., 2009). MiR-135b is upregulated in lung tissues of mice exposed to cigarette smoke, and regulates the IL-1 pathway by targeting IL-1R1 (Halappanavar et al., 2013). Several studies have shown the involvement of IL-1 signaling in chronic inflammation, remodeling of airways, and pathogenesis of COPD (Osei et al., 2020). The upregulation of miR-223 in lung tissues of COPD patients and in mice exposed to cigarette smoke is inversely correlated to the expression of its target HDAC2 and leads to the upregulation of CX3CL1 (Leuenberger et al., 2016). Declined HDAC activity permits the acetylated chromatin to be unbound to histones and this step allows chromatin access for transcription factors and transcription of various inflammatory cytokines and chemokines (Barnes et al., 2005). Elevated expression of miR-195 is observed in lung tissues of COPD patients and mice exposed to cigarette smoke, which causes downregulation of its target PH domain and leucine-rich repeat protein phosphatase 2 (PHLPP2) and increases Akt phosphorylation, leading to increased expression of IL-6 and TNF-α (Gu et al., 2018). Earlier studies suggest the role of PHLPP2 in direct dephosphorylation and inactivation of Akt, which has multifunctional activities and is a potential regulator of various cellular processes involved in the pathogenesis of COPD (Bozinovski et al., 2006; Gao et al., 2009; Nowak et al., 2015). The upregulation of miR-664a-3p and downregulation of its target four and a half LIM domains 1 (FHL1), which acts as a transcription factor and implicated in various cellular mechanisms, in lung tissue and peripheral blood mononuclear cells (PBMCs) of COPD patients positively correlated with forced expiratory volume in one second (FEV1)/forced vital capacity (FVC)% and has a role in cigarette smoke-induced COPD (Zhong et al., 2019). A recent report demonstrates that the upregulation of miR-130 in BEAS-2B cells treated with cigarette smoke extract (CSE) and in mice exposed to CSE, negatively regulates Wnt/β-catenin signaling by targeting Wnt1 and modulating β-Catenin, and lymphoid enhancer-binding factor (LEF) (Wu et al., 2020). Earlier, the role of β-Catenin is shown in cell proliferation and injury repair (Zemans et al., 2011; Tanjore et al., 2013). Further, it has been demonstrated that activation of Wnt/β-catenin signaling may potentially attenuate COPD pathogenesis (Kneidinger et al., 2011; Uhl et al., 2015).
Reduced expression of miR-34c in lung tissues of COPD patients modulates the expression of SERPINE1, which is a protease and fibrinolysis inhibitor (Savarimuthu Francis et al., 2014). The authors suggest that SERPINE1 has other functions apart from antiproteases in the lung that may play important role in emphysema progression. Nuclear factor-kappaB (NF-κB) is a crucial transcription factor and persistent stimulation of the NF-κB signaling pathway provokes the exaggerated synthesis of pro-inflammatory mediators such as IL-8 and TNF-α, which leads to airway impairment in COPD patients (Edwards et al., 2009). The downregulation of miR-149-3p in the blood of smokers with COPD activates TLR-4/NF-κB signaling and upregulates IL-1β and TNF-α by targeting TLR-4 (Shen et al., 2017). Moreover, miR-145-5p expression is reduced in lung tissues of smokers without or with COPD and regulates p53-mediated apoptotic signaling, NF-κB signaling, TNF-α, IL-6, and IL-8 by targeting kruppel like factor 5 (KLF5) (Dang et al., 2019). Consistently, overexpression of miR-145-5p attenuates CSE-stimulated apoptosis and inflammation in human bronchial epithelial cells (HBECs) (Dang et al., 2019). The role of p53-mediated signaling pathways has been shown in CSE-induced cell apoptosis (Lee and Choi, 2018). KLF5 belongs to a family of zinc-finger (ZF) containing transcription factors and is implicated in the regulation of a wide range of cellular processes such as cell proliferation, apoptosis, inflammation, migration, and differentiation (Dong and Chen, 2009). The downregulation of miR-29b is found in lung tissues and plasma from COPD patients, which regulates CSE-induced IL-8 expression by targeting bromodomain protein 4 (BRD4) (Tang et al., 2019). The role of BRD4 has been shown in direct or indirect regulation of gene transcription (Devaiah et al., 2016a; Devaiah et al., 2016b). Further, studies also demonstrated that inhibition of BRD4 significantly decreases the level of proinflammatory cytokines, which suggests its important role in the inflammatory process (Nicodeme et al., 2010; Tian et al., 2017). Thus, signify the vital function of the miR-29b-BRD4 axis in airway inflammation and pathogenesis of COPD.
In addition to miRNAs, the association of lncRNA is also shown in the pathogenesis and development of COPD. For example, in the lung tissues of COPD patients, TUG1 is upregulated and its silencing reduces α-SMA and fibronectin expression and stimulates the proliferation of TGF-β induced- BEAS-2B and HFL1 cells (Tang W. et al., 2016). The upregulation of lncRNA-ENST00000502883.1 is found in B cells and CD4+ T cells from COPD patients and it is shown that it affects PBMC recruitment via regulation of CXCL16 (Qu et al., 2018). CXCL16 functions as a chemoattractant for Th1 cells and it is considered as a systemic inflammatory marker for COPD (Shashkin et al., 2003; Donnelly and Barnes, 2006; Eagan et al., 2010). The nuclear enriched abundant transcript 1 (NEAT1) is upregulated in plasma from COPD patients and negatively correlates with miR-193a and positively correlates with GOLD stage and the expressions of TNF-α, IL-1β, IL-6, and IL-17 (Ming et al., 2019). NEAT1-induced inflammatory cascades and oxidative stress lead to severe lung injury, which establishes NEAT1 is positively correlated with COPD severity and inflammation and its potential in the prediction of disease susceptibility and acute exacerbation risk. The metastasis-associated lung adenocarcinoma transcript 1 (MALAT1) is upregulated in lung tissues of COPD patients (Hu et al., 2020). In the same study, in vitro experiments with TGF-β-treated human lung fibroblasts showed that MALAT1 downregulation stimulates cellular viability and inhibits mesenchymal protein expression by regulating the mTOR pathway, which is involved in lung cell senescence in COPD. The downregulation of HOXA cluster antisense RNA 2 (HOXA-AS2) is found in lung tissues from COPD patients and further studies in CSE-treated human pulmonary microvascular endothelial cells (HPMECs) demonstrated that the downregulation of HOXA-AS2 suppresses cell proliferation via Notch1 signaling (Zhou et al., 2020). This implies that upregulation Notch1, which is implicated in various cellular processes such as cell proliferation, differentiation, and apoptosis, stimulates HOXA-AS2-dependent cell proliferation and mitigates the cell viability injury. The lung cancer-associated transcript 1 (LUCAT1) is elevated in the serum of COPD patients (Zhao et al., 2021). Further studies in CSE-treated 16HBE cells show that LUCAT1 downregulates its target, miR-181a-5p, upregulates inflammatory cytokines (IL-1β, IL-6, and TNF-α), and regulates cell proliferation and apoptosis via the Wnt/β-catenin pathway (Zhao et al., 2021). The role of activated Wnt/β-catenin pathway in induction of inflammatory cytokines (IL-1β, IL-6, and TNF-α) and cell proliferation and apoptosis are well characterized (Masckauchan et al., 2005; Aumiller et al., 2013; Jang et al., 2017). This suggests LUCAT1 plays an important role in the regulation of inflammatory cytokines and the Wnt/β-catenin pathway, thus have a crucial function in the pathogenesis of COPD. Table 2 summarizes the list of ncRNAs, miRNAs and lncRNAs with their targets and functions in COPD. Collectively, these studies suggest that miRNAs, lncRNAs, and their interaction and regulation have a significant role in the pathogenesis and development of COPD. Understanding these mechanisms will lead to novel therapeutic interventions and approaches for better management of COPD.
Cystic Fibrosis (CF)
CF is the most common genetic autosomal recessive lethal disease. It is caused by loss-of-function mutations in the cystic fibrosis transmembrane conductance regulator (CFTR) gene leading to aberrant translation, protein mis-folding, and/or trafficking (Riordan et al., 1989; Cutting, 2015). Impairment of CFTR, a crucial chloride ion channel, results in ionic disequilibria and concurrently, airway dehydration and mucus accumulation. This further leads to chronic airway infections and inflammation and eventually, fatal deterioration in lung function.
Growing evidence supports the role of miRNAs in the direct or indirect regulation of CFTR and/or CFTR-related genes/proteins (Table 3). For example, several miRNAs including miR-101, miR-145, miR-384, miR-494, miR-600 are directly concomitant with CFTR dysregulation in airway epithelial cells like A549, Beas-2B, bronchial brushings, Caco-2, Calu-3, CFBE41o-, differentiated primary cell cultures, 16HBE14o-, HBEpiC, HEK293, PANC-1 (Gillen et al., 2011; Megiorni et al., 2011; Hassan et al., 2012; Oglesby et al., 2013; Ramachandran et al., 2013; Viart et al., 2015; Fabbri et al., 2017; Lutful Kabir et al., 2018). These studies suggest that the regulation of CFTR expression by miRNAs in different cell types is diverse, tissue-specific, and time-dependent. Antisense targeting of miR-145-5p through peptide nucleic acid (PNA) upregulates CFTR expression (Fabbri et al., 2017; Finotti et al., 2019). Consistently, suppression of miR-145 has been shown to restore F508del CFTR expression (Lutful Kabir et al., 2018; Dutta et al., 2019). Further, a recent study shows PNA masking of the miR-145-5p binding site of CFTR mRNA upregulates CFTR at both mRNA and protein levels (Sultan et al., 2020). Similar PNA targeting of miR-101-3p also upregulates CFTR (Fabbri et al., 2021). The indirect association is also determined between miRNA and CFTR. For example, miRNA-138 interacts with its target switch-independent 3 homolog A (SIN3A), a transcriptional regulatory protein, and downregulates CFTR (Ramachandran et al., 2012). Further, the same study showed that controlling miR-138/SIN3A expression restores F508del-CFTR expression. The upregulation of miR-9 in CF cells downregulates its target anoctamin 1 (ANO1) alias calcium-activated chloride channel (transmembrane protein 16A, TMEM16A) and preventing the inhibition of ANO1 in vitro and in vivo CF models via the miR-9 target site blocker (TSB) elevates chloride efflux, mucociliary clearance, and migration rate of cells (Benedetto et al., 2017; Sonneville et al., 2017).
MiRNAs that regulate CF lung disease via the regulation of inflammation, airway obstruction, or infection are listed in Table 4. For example, the elevated expression of miR-155 in the CF lung epithelium leads to downregulation of SH-2 containing inositol 5′ polyphosphatase 1 (SHIP1), an inositol 5-phosphatase, and thereby induces IL-8 expression via regulation of phosphatidylinositol-3 kinase/protein kinase B (PI3K/Akt) signaling (Bhattacharyya et al., 2011). Further, the RNA-binding protein tristetraprolin (TTP), a zinc finger protein also known as ZFP36, suppresses miR-155 expression in CF lung epithelial cells via upregulation of miR-1, while KH-type splicing regulatory protein (KSRP), the KH domain-containing splicing factor, upregulates miR-155 via promoting enhanced biogenesis (Bhattacharyya et al., 2013). Moreover, miR-155 targets the regulatory associated protein of mTOR complex 1 (RPTOR) and activates TGF-β signaling, and upregulates connective tissue growth factor (CTGF) in CF lung epithelial cells, thereby promoting fibrosis. (Tsuchiya et al., 2016). RPTOR is implicated in the modulation of the mammalian target of rapamycin complex 1 (mTORC1) activity that controls cell growth and survival whereas CTGF is a fibrotic factor that stimulates amplified fibrogenesis and airway remodeling. Furthermore, miR-16 rescues the F508del-CFTR trafficking defects probably through downregulation of heat shock protein 90 (HSP90) (Kumar et al., 2015).
In endobronchial brushings from CF patients, reduced expression of miR-126 upregulates the Target of Myb1 (TOM1) and regulates NF-κB-mediated IL-8 secretion (Oglesby et al., 2010). TOM1 belongs to a family of proteins containing an N-terminal VHS (Vps27p/Hrs/STAM) domain, and it has been demonstrated that TOM1 negatively regulates IL-1β- and TNF-α–induced signaling pathways while its upregulation leads to suppression of NF-κB (Yamakami and Yokosawa, 2004). Moreover, TOM1 through the interaction with Toll-interacting protein (Tollip) regulates intracellular trafficking (Yamakami et al., 2003; Katoh et al., 2004). These findings suggest miR-126, which directly targets TOM1, represents a crucial role in the regulation of innate immune responses and endosomal trafficking of ubiquitinated proteins in the CF lung. miR-146a negatively regulates Mucin 5AC (MUC5AC) expression, which is one of the foremost constituents of airway mucus, probably through the c-Jun N-terminal kinase (JNK) and NF-κB signaling in the neutrophil elastase (NE)-induced 16HBE14o-cells (Zhong et al., 2011). These results indicate the manipulation in miR-146a expression could regulate the excessive synthesis of mucus and thereby, CF pathogenesis. A recent study demonstrated that the inhibition of miR-146a induces increased expression of IL-6 in lipopolysaccharide (LPS)-stimulated CF macrophages (Luly et al., 2019). This study indicates that miR-146a dysregulation leads to dysfunctional CF macrophages, which results in impaired host defense and overproduction of inflammatory responses, and contributes to the progression and severity of CF. Several miRNAs including miR-509-3p, miR-494, and miR-126 regulate NF-kB, which in turn regulate CFTR expression and function (McKiernan et al., 2013; Ramachandran et al., 2013). Mir-31 downregulation increases cathepsin S, an inhibitor of antimicrobial proteins, through targeting the transcription factor IRF-1 in CF pulmonary epithelial cells (Weldon et al., 2014). This results in the excessive accumulation of cathepsin S, which leads to the protease burden of the CF lung, and thereby the miR-31/IRF-1/CTSS pathway contributes to pulmonary inflammation in the CF airways (Weldon et al., 2014). The exogenous overexpression of miR-17 and miR-1343 downregulates IL-8 and TGF-β, respectively, in CF airway epithelial cells (Oglesby et al., 2015; Stolzenburg et al., 2016). MiR-199a-3p negatively regulates the NF-kB signaling pathway and IL-8 via its target inhibitor of nuclear factor kappa-B kinase subunit beta (IKKβ), which is implicated in the NF-κB pathway, and downregulation of miR-199a-3p contributes to pulmonary inflammation in the CF airways (Bardin et al., 2018).
Besides miRNAs, lncRNAs also regulate CF lung disease. For example, a number of lncRNAs including TLR8-AS1, HOTAIR, XIST, and MALAT are differentially expressed in bronchial brushings of CF patients (McKiernan et al., 2014). Pseudomonas aeruginosa infected CF bronchial epithelial cells exhibit dysregulation of several lncRNAs including MEG9 (maternally expressed 9) and BLACAT1 (bladder cancer-associated transcript 1) (Balloy et al., 2017). However, more investigations are required to understand the role and molecular mechanism of these lncRNAs in CF. Further, a recent study has illustrated the differential expression of lncRNAs in CF lung airway and parenchyma tissues, that affect multiple signaling pathways and cell membrane functions (Kumar et al., 2019). Interestingly, the suppression of lncRNA BGas rescues CFTR expression and function through the interaction with HMGB DNA-distorting proteins, members of the high mobility group (HMG) superfamily that lead to modifications of local chromatin and DNA structure of intron 11 of the CFTR gene (Saayman et al., 2016). Collectively, these observations underscore the promising associations of certain ncRNAs, both miRNAs and lncRNAs, in the direct or indirect regulation of CFTR expression and function, as well other aspects of CF disease phenotypes such as inflammation, airway obstruction, or infection as summarized in Tables 3, 4. The comprehensive knowledge of their roles and mechanisms in the pathogenesis and regulation of CF disease may represent a novel therapeutic approach for cystic fibrosis.
Idiopathic Pulmonary Fibrosis (IPF)
IPF is a lethal progressive fibrotic disease of the lung interstitium, and is mainly characterized by persistent epithelial injury, scar tissue accumulation, increased fibroblast proliferation, amplified production of extracellular matrix (ECM), and excessive inflammation (Martinez et al., 2017; Mora et al., 2017). However, the exact etiology and pathogenesis of this disease is still not very well-defined.
In recent decades, the association between the pathogenesis of IPF and ncRNAs is recognized by an increasing number of studies. For example, miR-199a-5p is upregulated in lungs and lung myofibroblasts from IPF patients and bleomycin (BLM)-induced mouse models and activates lung fibroblast and fibrosis through targeting caveolin-1 (CAV-1), a major mediator of pulmonary fibrosis, and modulation of TGF-β signaling, which is involved in activation of fibroblasts proliferation and induction of EMT in alveolar epithelial cells (Lino Cardenas et al., 2013). MiR-21 is upregulated in peripheral blood from IPF patients, and inhibition of miR-21 in rat models upregulates its target a disintegrin-like and metalloproteinase with thrombospondin type 1 motif (ADAMTS-1), which downregulates pulmonary collagen type 1 (Col1) and collagen type 3 (Col3) contents and reduces the progression of IPF (Liu et al., 2016). The increased expression of miR-142-5p and reduced expression of miR-130a-3p are observed in macrophages from IPF patients and BLM-induced mouse models (Su et al., 2015). Thus, inhibition of miR-142-5p and overexpression of miR-130a-3p suppress lung fibrosis through stimulation of the STAT6 pathway by targeting peroxisome proliferator-activated receptor γ (PPARγ, a STAT6 coordinator) and suppressor of cytokine signaling 1 (SOCS1, a STAT6 inhibitor), respectively, which facilitates macrophage activation and contribute to extensive tissue fibrosis.
MiR-26a is downregulated in A549 cells and BLM-induced mouse models, and its overexpression diminishes epithelial-mesenchymal transition (EMT) through targeting high mobility group AT-hook 2 (HMGA2), a main positive regulatory factor in EMT (Liang et al., 2014a). The downregulation of miR-326 is reported in the lungs of IPF patients and BLM-induced mouse models (Das et al., 2014). Consistently, the overexpression of miR-326 suppresses TGF-β1 expression and diminishes the fibrotic response by downregulation of profibrotic genes (Ets1, Smad3, and matrix metalloproteinase 9 (MM9) and upregulation of antifibrotic genes (Smad7) (Das et al., 2014). The downregulation of miR-486-5p is found in lung tissues of IPF patients, and its overexpression in mouse models reduces lung fibrosis through targeting SMAD2, a crucial mediator of pulmonary fibrosis and implicated in TGF-β1 signaling (Ji et al., 2015). The downregulation of miR-323a-3p is found in lung epithelium from IPF patients, and its overexpression in IPF mouse models reduces fibroproliferation via directly targeting its targets TGFA and SMAD2 and modulation of various profibrotic signaling such as TGF-α, TGF-β, and apoptosis (Ge et al., 2016). In addition, miR-323a-3p downregulates CASP3, which prevents programmed cell death. MiR-221 is downregulated in tissues from human IPF, and in adenocarcinoma A549 and human bronchial epithelium (HBE) cell lines (Wang et al., 2016). Consistently, overexpression of miR-221 in these cell lines suppresses HMGA2 as well as phosphorylated-Smad3, which modulate TGF-β1 signaling, and leads to attenuation of EMT and lung fibrosis. The reduced expression of miR-29c is observed in alveolar epithelial cells from IPF patients, and overexpression of miR-29c in mice model reduces apoptosis, increases epithelial renewal, and thereby reduces lung fibrosis through targeting forkhead box O3a (Foxo3a), which is a transcription factor and play a crucial role in the induction of apoptosis (Xie et al., 2017). MiR-30a is downregulated in IPF patients, and further in vitro analyses indicate that overexpression of miR-30a directly targets ten-eleven translocation 1 (TET1) that modulates dynamin-related protein1 (Drp-1) promoter hydroxymethylation and thereby, show antifibrotic effect and defensive role against pulmonary damage (Zhang S. et al., 2017). The downregulation of miR-18a-5p is reported in pleural mesothelial cells (PMCs) and BLM-induced mouse models, and overexpression of miR-18a-5p downregulates its target TGF-βRII and reduces EMT of PMCs and sub-pleural pulmonary fibrosis (Zhang Q. et al., 2017). MiR-155 is downregulated in lung fibroblasts from IPF patients, and lung macrophages and fibroblasts from BLM-induced mouse models (Kurowska-Stolarska et al., 2017). In the same study, it is shown that overexpression of miR-155 decreases the exacerbated fibrotic response through downregulating its target liver X receptor α (LXRα), an oxysterol-activated transcription factor, and thereby, decreased production of collagen and TGF-β (Kurowska-Stolarska et al., 2017). The downregulation of miR-30a-5p is shown in exosomes from bronchoalveolar lavage fluid (BALF) of IPF elderly patients and A549 cells, its overexpression downregulates α-smooth muscle actin, and fibronectin expression by targeting TGF-β activated kinase 1/MAP3K7 binding protein 3 (TAB3), which is implicated in various cellular processes such as immune and inflammatory responses, altered fibrosis, and tissue repair and remodeling involved in IPF pathogenesis (Liu et al., 2018a). MiR-708-3p is downregulated in plasma and tissues from IPF patients, and overexpression of miR-708-3p attenuates lung fibrogenesis through directly modulating its target a disintegrin and metalloproteinase 17 (ADAM17), which regulates immune responses, fibrosis, and tissue regeneration, and by GATA/STAT3 signal pathway that is implicated in fibroblast-myofibroblast differentiation (Liu et al., 2018b). A recent report shows that miR-186 is downregulated in lung tissues of IPF patients, and delivery of miR-186 by human bone marrow mesenchymal stem cell-derived extracellular vesicles (BMSC-EVs) reduces fibroblast activation by downregulating its target SRY-related HMG box transcription factor 4 (SOX4) and thereby Dickkopf-1 (DKK1) (Zhou et al., 2021). SOX4 acts as a transcription factor and is associated with lung development and cell survival, whereas DKK1 is an inhibitor of the Wnt signaling pathway that have a significant role in lung development and differentiation and IPF pathogenesis and progression (Menezes et al., 2012; Guan and Zhou, 2017; Pan et al., 2017).
Several studies have also demonstrated the involvement of lncRNAs in the pathogenesis of IPF. For example, elevated levels of the lncRNA H19 in BLM-induced mouse models upregulates COL1A1 and Acta2, prominent factors linked with IPF pathogenesis, through direct targeting of miR-29b, and consequently knockdown of H19 attenuates fibrogenesis (Tang Y. et al., 2016). The telomeric repeat-containing RNA (TERRA) is upregulated in the blood of IPF patients, and further in vivo and in vitro studies demonstrate its role in fibrogenesis by the regulation of telomeric and mitochondrial functions (Gao et al., 2017). Dysfunctional telomerase activity and mitochondria under oxidative stress elicit apoptosis of epithelial cells and other processes linked with IPF progression. The increased expression of lncRNA PCF in the lungs of IPF patients induces pulmonary fibrosis by directly targeting miR-344a-5p and regulating map3k11 that elicit the proliferation of activated epithelial cells (Liu et al., 2017). Pulmonary fibrosis-regulatory lncRNA (PFRL) regulates the reciprocal repression of miR-26a and Smad2, which elicits the proliferation of activated epithelial cells, and contributes to the collagen deposition and progression of lung fibrosis (Jiang et al., 2018). In lungs and lung fibroblasts from mice, lncRNA pulmonary fibrosis-associated RNA (PFAR) functions as a competitive endogenous RNA (ceRNA) for miR-15a and in the regulation of yes-associated protein 1 (YAP1)-Twist expression, which is an important transcriptional effector in the Hippo pathway and implicated in the organ fibrosis process (Zhao et al., 2018; Sun et al., 2019). Upregulated lncRNA H19 in tissues from IPF patients downregulates miR-140 and modulates TGF-β/Smad3 signaling, and further in vivo and in vitro experiments show that the knockdown of H19 diminishes pulmonary fibrosis (Wang et al., 2019). The elevated expression of Zinc-finger E-box binding homeobox 1 antisense RNA 1 (ZEB1-AS1) and its positive correlation with the expression of ZEB1, which is a master regulator of EMT, is found in BLM-induced rats and TGF-β1-induced RLE-6TN cells (Qian et al., 2019). Subsequent experiments demonstrated that silencing of lncRNA ZEB1-AS1upregulates its target miR-141-3p and suppresses progression of EMT and fibrogenesis. Therefore, cumulatively these data clearly depict the regulatory functions, particularly the post-transcriptional regulation of ncRNA, miRNAs and lncRNAs, in onset, progression, and development of IPF. Table 5 summarizes the list of ncRNAs, their targets, and functions associated with IPF. Further, additional in-depth studies will lead to therapies for early diagnosis, control, and treatment of IPF.
Discussion
Here, we summarize the emerging roles, post-transcriptional regulations, and mechanistic functions of ncRNAs, with emphasis on miRNAs and lncRNAs, in lung diseases that are a major public health concern. According to a recent report, only in the year 2017, lung diseases globally affected nearly 545 million people and caused 3.9 million deaths with an increase of 39.8 and 18.0%, respectively, since 1990 (Collaborators, 2020). Thus, lung diseases are a predominant cause of substantial morbidity and mortality worldwide and demand an exhaustive understanding of etiology and pathophysiology. Recent studies have established the association and regulatory function of ncRNAs in lung development and maintenance of lung homeostasis. The deregulation of ncRNAs causes pathophysiological alteration and contributes to the onset, progression, and development of various types of lung diseases such as asthma, COPD, CF, and IPF (Figure 1)
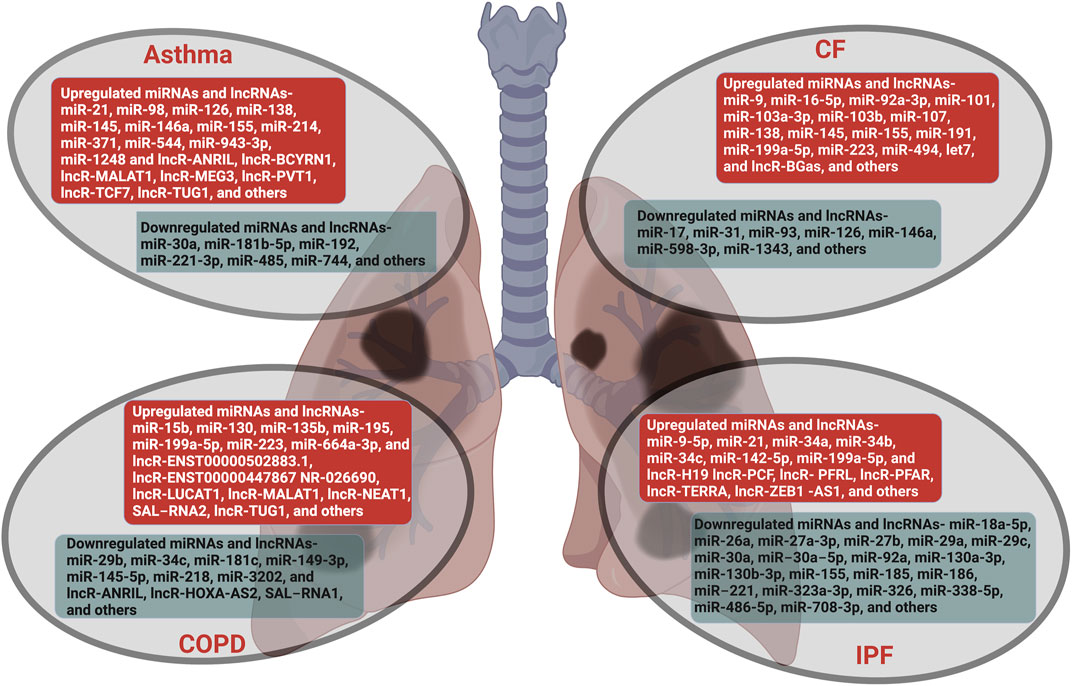
FIGURE 1. Lung diseases regulated by non-coding RNAs. The miRNAs and lncRNAs that are aberrantly expressed in lung diseases such as asthma, COPD, CF and IPF are listed. The ncRNAs that are upregulated are in red boxes and those that are downregulated are in green boxes.
Alterations of miRNA and lncRNA expression level in the disease state compared to the normal state expedite a new paradigm for the diagnosis and appraisal of drug action. As presented in this review, disease-specific dysregulated miRNAs/lncRNAs are identified in various types of lung cells and tissues, which together with the higher stability of miRNAs mark them as clinical diagnostic biomarkers. However, a major challenge is the invasive procedures used for obtaining lung biopsies. Recent reports indicate detection of miRNAs/lncRNAs in body fluids such as blood, serum, plasma, BAL fluid, saliva, sputum, and urine, which have tremendous potential for relatively non-invasive diagnosis and prognosis of lung disease as well as appraisal of drug action. However, the utility of these biospecimen as a clinical diagnostic biomarker is yet to be examined and established with a larger patient cohort in various lung diseases.
Notably, ncRNA-based therapeutics have great potential in the treatment of lung diseases. Collectively, the studies summarized here show that enormous efforts have been made to deliver mimic or antisense oligonucleotide (ASO, including inhibitor, miRNA sponge, and target site blocker (TSB)) to overexpress or suppress specific genes that are downregulated or upregulated, respectively, in the diseased state and contribute to the pathogenesis and pathophysiology of lung diseases. However, in order to translate this treatment strategy from lab to clinical settings, some challenges including cell/tissue-specific delivery, stability and binding affinity, and off-target effects need to be addressed. Recent progress in generating modified derivatives of nucleic acid as potential drugs include numerous chemical strategies, such as the addition of 2′-O-methyl (2-O′-Me) or phosphorothioate-like groups, locked nucleic acids (LNA), miRNA sponges, nanoparticles, morpholinos, or peptide nucleic acids (PNA) as well as strategies for efficient delivery, such as viral vectors, polymers-, peptides-, and lipid-based delivery systems. Despite these efforts, there is still a need for more extensive studies to evaluate the effect of chemical modifications in in vivo systems and develop more consistent cell/tissue-specific delivery strategies.
Our current knowledge suggests that the identification of disease-specific miRNAs/lncRNAs and comprehensive knowledge of post-transcriptional regulation mechanisms will help understand their role and mode of functioning in the pathogenesis of lung diseases. Concurrently, the development of safe and cell/tissue-specific delivery systems will help to translate ncRNAs-based therapeutics from lab to clinical settings. Hence, we are optimistic that the continued elucidation of the function of ncRNAs encompasses the great potential to uncover diagnostic and prognostic tools and candidate therapeutic targets for lung diseases in the near future.
Author Contributions
DS and RB prepared the manuscript.
Conflict of Interest
The authors declare that the research was conducted in the absence of any commercial or financial relationships that could be construed as a potential conflict of interest.
Publisher’s Note
All claims expressed in this article are solely those of the authors and do not necessarily represent those of their affiliated organizations, or those of the publisher, the editors and the reviewers. Any product that may be evaluated in this article, or claim that may be made by its manufacturer, is not guaranteed or endorsed by the publisher.
Abbreviations
CF, Cystic Fibrosis; COPD, Chronic obstructive pulmonary disease; IPF, Idiopathic pulmonary fibrosis; LncRNA, Long non-coding RNA; miRNA, miR, MicroRNA.
References
Aumiller, V., Balsara, N., Wilhelm, J., Günther, A., and Königshoff, M. (2013). WNT/β-Catenin Signaling Induces IL-1β Expression by Alveolar Epithelial Cells in Pulmonary Fibrosis. Am. J. Respir. Cel Mol Biol 49 (1), 96–104. doi:10.1165/rcmb.2012-0524OC
Austin, P. J., Tsitsiou, E., Boardman, C., Jones, S. W., Lindsay, M. A., Adcock, I. M., et al. (2017). Transcriptional Profiling Identifies the Long Noncoding RNA Plasmacytoma Variant Translocation ( PVT1 ) as a Novel Regulator of the Asthmatic Phenotype in Human Airway Smooth Muscle. J. Allergy Clin. Immunol. 139 (3), 780–789. doi:10.1016/j.jaci.2016.06.014
Balloy, V., Koshy, R., Perra, L., Corvol, H., Chignard, M., Guillot, L., et al. (2017). Bronchial Epithelial Cells from Cystic Fibrosis Patients Express a Specific Long Non-coding RNA Signature Upon Pseudomonas aeruginosa Infection. Front. Cel. Infect. Microbiol. 7, 218. doi:10.3389/fcimb.2017.00218
Bao, Z., Yang, Z., Huang, Z., Zhou, Y., Cui, Q., and Dong, D. (2019). LncRNADisease 2.0: An Updated Database of Long Non-coding RNA-Associated Diseases. Nucleic Acids Res. 47 (D1), D1034–D1037. doi:10.1093/nar/gky905
Bardin, P., Marchal-Duval, E., Sonneville, F., Blouquit-Laye, S., Rousselet, N., Le Rouzic, P., et al. (2018). Small RNA and Transcriptome Sequencing Reveal the Role of miR-199a-3p in Inflammatory Processes in Cystic Fibrosis Airways. J. Pathol. 245 (4), 410–420. doi:10.1002/path.5095
Barnes, P. J., Adcock, I. M., and Ito, K. (2005). Histone Acetylation and Deacetylation: Importance in Inflammatory Lung Diseases. Eur. Respir. J. 25 (3), 552–563. doi:10.1183/09031936.05.00117504
Barreto-Luis, A., Corrales, A., Acosta-Herrera, M., Gonzalez-Colino, C., Cumplido, J., Martinez-Tadeo, J., et al. (2017). A Pathway-Based Association Study Reveals Variants From Wnt Signalling Genes Contributing to Asthma Susceptibility. Clin. Exp. Allergy 47 (5), 618–626. doi:10.1111/cea.12883
Bartel, D. P. (2009). MicroRNAs: Target Recognition and Regulatory Functions. Cell 136 (2), 215–233. doi:10.1016/j.cell.2009.01.002
Bartel, S., Carraro, G., Alessandrini, F., Krauss-Etschmann, S., Ricciardolo, F. L. M., and Bellusci, S. (2018). miR-142-3p Is Associated with Aberrant WNT Signaling During Airway Remodeling in Asthma. Am. J. Physiology-Lung Cell Mol. Physiol. 315 (2), L328–L333. doi:10.1152/ajplung.00113.2018
Beermann, J., Piccoli, M.-T., Viereck, J., and Thum, T. (2016). Non-coding RNAs in Development and Disease: Background, Mechanisms, and Therapeutic Approaches. Physiol. Rev. 96 (4), 1297–1325. doi:10.1152/physrev.00041.2015
Benedetto, R., Ousingsawat, J., Wanitchakool, P., Zhang, Y., Holtzman, M. J., Amaral, M., et al. (2017). Epithelial Chloride Transport by CFTR Requires TMEM16A. Sci. Rep. 7 (1), 12397. doi:10.1038/s41598-017-10910-0
Berschneider, B., Ellwanger, D. C., Baarsma, H. A., Thiel, C., Shimbori, C., White, E. S., et al. (2014). miR-92a Regulates TGF-Β1-Induced WISP1 Expression in Pulmonary Fibrosis. Int. J. Biochem. Cel Biol. 53, 432–441. doi:10.1016/j.biocel.2014.06.011
Bhattacharyya, S., Balakathiresan, N. S., Dalgard, C., Gutti, U., Armistead, D., Jozwik, C., et al. (2011). Elevated miR-155 Promotes Inflammation in Cystic Fibrosis by Driving Hyperexpression of Interleukin-8. J. Biol. Chem. 286 (13), 11604–11615. doi:10.1074/jbc.M110.198390
Bhattacharyya, S., Kumar, P., Tsuchiya, M., Bhattacharyya, A., and Biswas, R. (2013). Regulation of miR-155 Biogenesis in Cystic Fibrosis Lung Epithelial Cells: Antagonistic Role of Two mRNA-Destabilizing Proteins, KSRP and TTP. Biochem. Biophysical Res. Commun. 433 (4), 484–488. doi:10.1016/j.bbrc.2013.03.025
Bhatti, G. K., Khullar, N., Sidhu, I. S., Navik, U. S., Reddy, A. P., Reddy, P. H., et al. (2021). Emerging Role of Non‐coding RNA in Health and Disease. Metab. Brain Dis. 36 (6), 1119–1134. doi:10.1007/s11011-021-00739-y
Bozinovski, S., Vlahos, R., Hansen, M., Liu, K., and Anderson, G. P. (2006). Akt in the Pathogenesis of COPD. Int. J. COPD 1 (1), 31–38. doi:10.2147/copd.2006.1.1.31
Carraro, G., Shrestha, A., Rostkovius, J., Contreras, A., Chao, C.-M., El Agha, E., et al. (2014). miR-142-3p Balances Proliferation and Differentiation of Mesenchymal Cells During Lung Development. Development 141 (6), 1272–1281. doi:10.1242/dev.105908
Cech, T. R., and Steitz, J. A. (2014). The Noncoding RNA Revolution-Trashing Old Rules to Forge New Ones. Cell 157 (1), 77–94. doi:10.1016/j.cell.2014.03.008
Cevhertas, L., Ogulur, I., Maurer, D. J., Burla, D., Ding, M., Jansen, K., et al. (2020). Advances and Recent Developments in Asthma in 2020. Allergy 75 (12), 3124–3146. doi:10.1111/all.14607
Chen, L., Xu, J., Chu, X., and Ju, C. (2017). MicroRNA-98 Interferes with Thrombospondin 1 Expression in Peripheral B Cells of Patients with Asthma. Biosci. Rep. 37 (4), BSR20170149. doi:10.1042/BSR20170149
Chew, C. L., Conos, S. A., Unal, B., and Tergaonkar, V. (2018). Noncoding RNAs: Master Regulators of Inflammatory Signaling. Trends Mol. Med. 24 (1), 66–84. doi:10.1016/j.molmed.2017.11.003
Choy, D. F., Modrek, B., Abbas, A. R., Kummerfeld, S., Clark, H. F., Wu, L. C., et al. (2011). Gene Expression Patterns of Th2 Inflammation and Intercellular Communication in Asthmatic Airways. J.I. 186 (3), 1861–1869. doi:10.4049/jimmunol.1002568
Collaborators, G. B. D. C. R. D. (2020). Prevalence and Attributable Health Burden of Chronic Respiratory Diseases, 1990-2017: A Systematic Analysis for the Global Burden of Disease Study 2017. Lancet Respir. Med. 8 (6), 585–596. doi:10.1016/S2213-2600(20)30105-3
Comer, B. S., Camoretti-Mercado, B., Kogut, P. C., Halayko, A. J., Solway, J., and Gerthoffer, W. T. (2015). Cyclooxygenase-2 and microRNA-155 Expression Are Elevated in Asthmatic Airway Smooth Muscle Cells. Am. J. Respir. Cel Mol Biol 52 (4), 438–447. doi:10.1165/rcmb.2014-0129OC
Cryan, S.-A., McKiernan, P., Cunningham, C. M., and Greene, C. (2013). Targeting miRNA-Based Medicines to Cystic Fibrosis Airway Epithelial Cells Using Nanotechnology. Ijn 8, 3907–3915. doi:10.2147/IJN.S47551
Cui, H., Banerjee, S., Xie, N., Ge, J., Liu, R.-M., Matalon, S., et al. (2016). MicroRNA-27a-3p Is a Negative Regulator of Lung Fibrosis by Targeting Myofibroblast Differentiation. Am. J. Respir. Cel Mol Biol 54 (6), 843–852. doi:10.1165/rcmb.2015-0205OC
Cui, H., Ge, J., Xie, N., Banerjee, S., Zhou, Y., Antony, V. B., et al. (2017). miR-34a Inhibits Lung Fibrosis by Inducing Lung Fibroblast Senescence. Am. J. Respir. Cel Mol Biol 56 (2), 168–178. doi:10.1165/rcmb.2016-0163OC
Cutting, G. R. (2015). Cystic Fibrosis Genetics: From Molecular Understanding to Clinical Application. Nat. Rev. Genet. 16 (1), 45–56. doi:10.1038/nrg3849
Dang, X., Yang, L., Guo, J., Hu, H., Li, F., Liu, Y., et al. (2019). miR-145-5p Is Associated with Smoke-Related Chronic Obstructive Pulmonary Disease via Targeting KLF5. Chemico-Biological Interactions 300, 82–90. doi:10.1016/j.cbi.2019.01.011
Das, S., Kumar, M., Negi, V., Pattnaik, B., Prakash, Y. S., Agrawal, A., et al. (2014). MicroRNA-326 Regulates Profibrotic Functions of Transforming Growth Factor-β in Pulmonary Fibrosis. Am. J. Respir. Cel Mol Biol 50 (5), 882–892. doi:10.1165/rcmb.2013-0195OC
Denli, A. M., Tops, B. B. J., Plasterk, R. H. A., Ketting, R. F., and Hannon, G. J. (2004). Processing of Primary MicroRNAs by the Microprocessor Complex. Nature 432 (7014), 231–235. doi:10.1038/nature03049
Devaiah, B. N., Case-Borden, C., Gegonne, A., Hsu, C. H., Chen, Q., Meerzaman, D., et al. (2016a). BRD4 Is a Histone Acetyltransferase That Evicts Nucleosomes From Chromatin. Nat. Struct. Mol. Biol. 23 (6), 540–548. doi:10.1038/nsmb.3228
Devaiah, B. N., Gegonne, A., and Singer, D. S. (2016b). Bromodomain 4: A Cellular Swiss Army Knife. J. Leukoc. Biol. 100 (4), 679–686. doi:10.1189/jlb.2RI0616-250R
Dietrich, A., Chubanov, V., Kalwa, H., Rost, B. R., and Gudermann, T. (2006). Cation Channels of the Transient Receptor Potential Superfamily: Their Role in Physiological and Pathophysiological Processes of Smooth Muscle Cells. Pharmacol. Ther. 112 (3), 744–760. doi:10.1016/j.pharmthera.2006.05.013
Disayabutr, S., Kim, E. K., Cha, S.-I., Green, G., Naikawadi, R. P., Jones, K. D., et al. (2016). miR-34 miRNAs Regulate Cellular Senescence in Type II Alveolar Epithelial Cells of Patients with Idiopathic Pulmonary Fibrosis. PLoS One 11 (6), e0158367. doi:10.1371/journal.pone.0158367
Djebali, S., Davis, C. A., Merkel, A., Dobin, A., Lassmann, T., Mortazavi, A., et al. (2012). Landscape of Transcription in Human Cells. Nature 489 (7414), 101–108. doi:10.1038/nature11233
Dong, J.-T., and Chen, C. (2009). Essential Role of KLF5 Transcription Factor in Cell Proliferation and Differentiation and its Implications for Human Diseases. Cell. Mol. Life Sci. 66 (16), 2691–2706. doi:10.1007/s00018-009-0045-z
Donnelly, L. E., and Barnes, P. J. (2006). Chemokine Receptors as Therapeutic Targets in Chronic Obstructive Pulmonary Disease. Trends Pharmacol. Sci. 27 (10), 546–553. doi:10.1016/j.tips.2006.08.001
Du, T., and Zamore, P. D. (2005). microPrimer: The Biogenesis and Function of MicroRNA. Development 132 (21), 4645–4652. doi:10.1242/dev.02070
Du, Y., Ding, Y., Chen, X., Mei, Z., Ding, H., Wu, Y., et al. (2017). MicroRNA-181c Inhibits Cigarette Smoke-Induced Chronic Obstructive Pulmonary Disease by Regulating CCN1 Expression. Respir. Res. 18 (1), 155. doi:10.1186/s12931-017-0639-1
Dutta, R. K., Chinnapaiyan, S., Rasmussen, L., Raju, S. V., and Unwalla, H. J. (2019). A Neutralizing Aptamer to TGFBR2 and miR-145 Antagonism Rescue Cigarette Smoke- and TGF-β-Mediated CFTR Expression. Mol. Ther. 27 (2), 442–455. doi:10.1016/j.ymthe.2018.11.017
Eagan, T. M. L., Ueland, T., Wagner, P. D., Hardie, J. A., Mollnes, T. E., Damas, J. K., et al. (2010). Systemic Inflammatory Markers in COPD: Results From the Bergen COPD Cohort Study. Eur. Respir. J. 35 (3), 540–548. doi:10.1183/09031936.00088209
Edwards, M. R., Bartlett, N. W., Clarke, D., Birrell, M., Belvisi, M., and Johnston, S. L. (2009). Targeting the NF-Κb Pathway in Asthma and Chronic Obstructive Pulmonary Disease. Pharmacol. Ther. 121 (1), 1–13. doi:10.1016/j.pharmthera.2008.09.003
Elbehidy, R. M., Youssef, D. M., El-Shal, A. S., Shalaby, S. M., Sherbiny, H. S., Sherief, L. M., et al. (2016). MicroRNA-21 as a Novel Biomarker in Diagnosis and Response to Therapy in Asthmatic Children. Mol. Immunol. 71, 107–114. doi:10.1016/j.molimm.2015.12.015
Esteller, M. (2011). Non-coding RNAs in Human Disease. Nat. Rev. Genet. 12 (12), 861–874. doi:10.1038/nrg3074
Ezzie, M. E., Crawford, M., Cho, J.-H., Orellana, R., Zhang, S., Gelinas, R., et al. (2012). Gene Expression Networks in COPD: MicroRNA and mRNA Regulation. Thorax 67 (2), 122–131. doi:10.1136/thoraxjnl-2011-200089
Fabbri, E., Borgatti, M., Montagner, G., Bianchi, N., Finotti, A., Lampronti, I., et al. (2014). Expression of microRNA-93 and Interleukin-8 During Pseudomonas Aeruginosa-Mediated Induction of Proinflammatory Responses. Am. J. Respir. Cel Mol Biol 50 (6), 1144–1155. doi:10.1165/rcmb.2013-0160OC
Fabbri, E., Tamanini, A., Jakova, T., Gasparello, J., Manicardi, A., Corradini, R., et al. (2021). Treatment of Human Airway Epithelial Calu-3 Cells with a Peptide-Nucleic Acid (PNA) Targeting the MicroRNA miR-101-3p Is Associated with Increased Expression of the Cystic Fibrosis Transmembrane Conductance Regulator () Gene. Eur. J. Med. Chem. 209, 112876. doi:10.1016/j.ejmech.2020.112876
Fabbri, E., Tamanini, A., Jakova, T., Gasparello, J., Manicardi, A., Corradini, R., et al. (2017). A Peptide Nucleic Acid Against MicroRNA miR-145-5p Enhances the Expression of the Cystic Fibrosis Transmembrane Conductance Regulator (CFTR) in Calu-3 Cells. Molecules 23 (1), 71. doi:10.3390/molecules23010071
Fan, M., Xu, J., Xiao, Q., Chen, F., and Han, X. (2019). Long Non-coding RNA TCF7 Contributes to the Growth and Migration of Airway Smooth Muscle Cells in Asthma Through Targeting TIMMDC1/Akt Axis. Biochem. Biophysical Res. Commun. 508 (3), 749–755. doi:10.1016/j.bbrc.2018.11.187
Fatica, A., and Bozzoni, I. (2014). Long Non-coding RNAs: New Players in Cell Differentiation and Development. Nat. Rev. Genet. 15 (1), 7–21. doi:10.1038/nrg3606
Fierro‐Fernández, M., Busnadiego, Ó., Sandoval, P., Espinosa‐Díez, C., Blanco‐Ruiz, E., Rodríguez, M., et al. (2015). miR‐9‐5p Suppresses Pro‐fibrogenic Transformation of Fibroblasts and Prevents Organ Fibrosis by Targeting NOX 4 and TGFBR 2. EMBO Rep. 16 (10), 1358–1377. doi:10.15252/embr.201540750
Finotti, A., Gasparello, J., Fabbri, E., Tamanini, A., Corradini, R., Dechecchi, M. C., et al. (2019). Enhancing the Expression of CFTR Using Antisense Molecules Against MicroRNA miR-145-5p. Am. J. Respir. Crit. Care Med. 199 (11), 1443–1444. doi:10.1164/rccm.201901-0019LE
Gao, M. H., Miyanohara, A., Feramisco, J. R., and Tang, T. (2009). Activation of PH-Domain Leucine-Rich Protein Phosphatase 2 (PHLPP2) by Agonist Stimulation in Cardiac Myocytes Expressing Adenylyl Cyclase Type 6. Biochem. Biophysical Res. Commun. 384 (2), 193–198. doi:10.1016/j.bbrc.2009.04.110
Gao, Y., Zhang, J., Liu, Y., Zhang, S., Wang, Y., Liu, B., et al. (2017). Regulation of TERRA on Telomeric and Mitochondrial Functions in IPF Pathogenesis. BMC Pulm. Med. 17 (1), 163. doi:10.1186/s12890-017-0516-1
Ge, J., Geng, S., and Jiang, H. (2019). Long Noncoding RNAs Antisense Noncoding RNA in the INK4 Locus (ANRIL) Correlates with Lower Acute Exacerbation Risk, Decreased Inflammatory Cytokines, and Mild GOLD Stage in Patients with Chronic Obstructive Pulmonary Disease. J. Clin. Lab. Anal. 33 (2), e22678. doi:10.1002/jcla.22678
Ge, L., Habiel, D. M., Hansbro, P. M., Kim, R. Y., Gharib, S. A., Edelman, J. D., et al. (2016). miR-323a-3p Regulates Lung Fibrosis by Targeting Multiple Profibrotic Pathways. JCI Insight 1 (20), e90301. doi:10.1172/jci.insight.90301
Gillen, A. E., Gosalia, N., Leir, S.-H., and Harris, A. (2011). MicroRNA Regulation of Expression of the Cystic Fibrosis Transmembrane Conductance Regulator Gene. Biochem. J. 438 (1), 25–32. doi:10.1042/BJ20110672
Glass, R. I., and Rosenthal, J. P. (2018). International Approach to Environmental and Lung Health. A Perspective From the Fogarty International Center. Ann. ATS 15 (Suppl. 2), S109–S113. doi:10.1513/AnnalsATS.201708-685MG
Groot, M., Zhang, D., and Jin, Y. (2018). Long Non-coding RNA Review and Implications in Lung Diseases. JSM Bioinform Genom Proteom 3 (2), 1033.
Gu, C., Li, Y., Liu, J., Ying, X., Liu, Y., Yan, J., et al. (2017). LncRNA-mediated SIRT1/FoxO3a and SIRT1/p53 Signaling Pathways Regulate Type II Alveolar Epithelial Cell Senescence in Patients with Chronic Obstructive Pulmonary Disease. Mol. Med. Rep. 15 (5), 3129–3134. doi:10.3892/mmr.2017.6367
Gu, W., Yuan, Y., Yang, H., Wu, H., Wang, L., Tang, Z., et al. (2018). Role of miR-195 in Cigarette Smoke-Induced Chronic Obstructive Pulmonary Disease. Int. Immunopharmacology 55, 49–54. doi:10.1016/j.intimp.2017.11.030
Guan, S., and Zhou, J. (2017). Frizzled-7 Mediates TGF-β-Induced Pulmonary Fibrosis by Transmitting Non-canonical Wnt Signaling. Exp. Cel Res. 359 (1), 226–234. doi:10.1016/j.yexcr.2017.07.025
Halappanavar, S., Nikota, J., Wu, D., Williams, A., Yauk, C. L., and Stampfli, M. (2013). IL-1 Receptor Regulates MicroRNA-135b Expression in a Negative Feedback Mechanism During Cigarette Smoke-Induced Inflammation. J.I. 190 (7), 3679–3686. doi:10.4049/jimmunol.1202456
Hammad Mahmoud Hammad, R., Hamed, D. H. E. D., Eldosoky, M. A. E. R., Ahmad, A. A. E. S., Osman, H. M., Abd Elgalil, H. M., et al. (2018). Plasma microRNA-21, microRNA-146a and IL-13 Expression in Asthmatic Children. Innate Immun. 24 (3), 171–179. doi:10.1177/1753425918763521
Han, J., Lee, Y., Yeom, K. H., Kim, Y. K., Jin, H., and Kim, V. N. (2004). The Drosha-DGCR8 Complex in Primary MicroRNA Processing. Genes Development 18 (24), 3016–3027. doi:10.1101/gad.1262504
Hangauer, M. J., Vaughn, I. W., and McManus, M. T. (2013). Pervasive Transcription of the Human Genome Produces Thousands of Previously Unidentified Long Intergenic Noncoding RNAs. Plos Genet. 9 (6), e1003569. doi:10.1371/journal.pgen.1003569
Hassan, F., Nuovo, G. J., Crawford, M., Boyaka, P. N., Kirkby, S., Nana-Sinkam, S. P., et al. (2012). MiR-101 and miR-144 Regulate the Expression of the CFTR Chloride Channel in the Lung. PLoS One 7 (11), e50837. doi:10.1371/journal.pone.0050837
Hu, T. J., Huang, H. B., Shen, H. B., Chen, W., and Yang, Z. H. (2020). Role of Long Non-coding RNA MALAT1 in C-hronic O-bstructive P-ulmonary D-isease. Exp. Ther. Med. 20 (3), 2691–2697. doi:10.3892/etm.2020.8996
Huang, H., Lu, H., Liang, L., Zhi, Y., Huo, B., Wu, L., et al. (2019). MicroRNA-744 Inhibits Proliferation of Bronchial Epithelial Cells by Regulating Smad3 Pathway via Targeting Transforming Growth Factor-Β1 (TGF-Β1) in Severe Asthma. Med. Sci. Monit. 25, 2159–2168. doi:10.12659/MSM.912412
Huo, X., Zhang, K., Yi, L., Mo, Y., Liang, Y., Zhao, J., et al. (2016). Decreased Epithelial and Plasma miR-181b-5p Expression Associates with Airway Eosinophilic Inflammation in Asthma. Clin. Exp. Allergy 46 (10), 1281–1290. doi:10.1111/cea.12754
Jang, J., Jung, Y., Chae, S., Chung, S.-I., Kim, S.-M., and Yoon, Y. (2017). WNT/β-catenin Pathway Modulates the TNF-α-Induced Inflammatory Response in Bronchial Epithelial Cells. Biochem. Biophysical Res. Commun. 484 (2), 442–449. doi:10.1016/j.bbrc.2017.01.156
Jensen, T. H., Jacquier, A., and Libri, D. (2013). Dealing with Pervasive Transcription. Mol. Cel 52 (4), 473–484. doi:10.1016/j.molcel.2013.10.032
Ji, X., Wu, B., Fan, J., Han, R., Luo, C., Wang, T., et al. (2015). The Anti-fibrotic Effects and Mechanisms of MicroRNA-486-5p in Pulmonary Fibrosis. Sci. Rep. 5, 14131. doi:10.1038/srep14131
Jiang, H., Chen, Y., Yu, T., Zhao, X., Shan, H., Sun, J., et al. (2018). Inhibition of lncRNA PFRL Prevents Pulmonary Fibrosis by Disrupting the miR-26a/smad2 Loop. Am. J. Physiology-Lung Cell Mol. Physiol. 315 (4), L563–L575. doi:10.1152/ajplung.00434.2017
Johansson, K., Malmhäll, C., Ramos-Ramírez, P., and Rådinger, M. (2017). MicroRNA-155 Is a Critical Regulator of Type 2 Innate Lymphoid Cells and IL-33 Signaling in Experimental Models of Allergic Airway Inflammation. J. Allergy Clin. Immunol. 139 (3), 1007–1016. doi:10.1016/j.jaci.2016.06.035
Kamikawaji, K., Seki, N., Watanabe, M., Mataki, H., Kumamoto, T., Takagi, K., et al. (2016). Regulation of LOXL2 and SERPINH1 by Antitumor microRNA-29a in Lung Cancer with Idiopathic Pulmonary Fibrosis. J. Hum. Genet. 61 (12), 985–993. doi:10.1038/jhg.2016.99
Katoh, Y., Shiba, Y., Mitsuhashi, H., Yanagida, Y., Takatsu, H., and Nakayama, K. (2004). Tollip and Tom1 Form a Complex and Recruit Ubiquitin-Conjugated Proteins onto Early Endosomes. J. Biol. Chem. 279 (23), 24435–24443. doi:10.1074/jbc.M400059200
Khalil, W., Xia, H., Bodempudi, V., Kahm, J., Hergert, P., Smith, K., et al. (2015). Pathologic Regulation of Collagen I by an Aberrant Protein Phosphatase 2A/Histone Deacetylase C4/MicroRNA-29 Signal Axis in Idiopathic Pulmonary Fibrosis Fibroblasts. Am. J. Respir. Cel Mol Biol 53 (3), 391–399. doi:10.1165/rcmb.2014-0150OC
Kim, R. Y., Horvat, J. C., Pinkerton, J. W., Starkey, M. R., Essilfie, A. T., Mayall, J. R., et al. (2017). MicroRNA-21 Drives Severe, Steroid-Insensitive Experimental Asthma by Amplifying Phosphoinositide 3-Kinase-Mediated Suppression of Histone Deacetylase 2. J. Allergy Clin. Immunol. 139 (2), 519–532. doi:10.1016/j.jaci.2016.04.038
Kim, V. N. (2005). MicroRNA Biogenesis: Coordinated Cropping and Dicing. Nat. Rev. Mol. Cel Biol 6 (5), 376–385. doi:10.1038/nrm1644
Kneidinger, N., Yildirim, A. Ö., Callegari, J., Takenaka, S., Stein, M. M., Dumitrascu, R., et al. (2011). Activation of the WNT/β-Catenin Pathway Attenuates Experimental Emphysema. Am. J. Respir. Crit. Care Med. 183 (6), 723–733. doi:10.1164/rccm.200910-1560OC
Kopp, F., and Mendell, J. T. (2018). Functional Classification and Experimental Dissection of Long Noncoding RNAs. Cell 172 (3), 393–407. doi:10.1016/j.cell.2018.01.011
Kugel, J. F., and Goodrich, J. A. (2012). Non-coding RNAs: Key Regulators of Mammalian Transcription. Trends Biochem. Sci. 37 (4), 144–151. doi:10.1016/j.tibs.2011.12.003
Kumar, P., Bhattacharyya, S., Peters, K. W., Glover, M. L., Sen, A., Cox, R. T., et al. (2015). miR-16 Rescues F508del-CFTR Function in Native Cystic Fibrosis Epithelial Cells. Gene Ther. 22 (11), 908–916. doi:10.1038/gt.2015.56
Kumar, P., Sen, C., Peters, K., Frizzell, R. A., and Biswas, R. (2019). Comparative Analyses of Long Non-coding RNA Profiles In Vivo in Cystic Fibrosis Lung Airway and Parenchyma Tissues. Respir. Res. 20 (1), 284. doi:10.1186/s12931-019-1259-8
Kurowska-Stolarska, M., Hasoo, M. K., Welsh, D. J., Stewart, L., McIntyre, D., Morton, B. E., et al. (2017). The Role of microRNA-155/liver X Receptor Pathway in Experimental and Idiopathic Pulmonary Fibrosis. J. Allergy Clin. Immunol. 139 (6), 1946–1956. doi:10.1016/j.jaci.2016.09.021
Lander, E. S., Linton, L. M., Birren, B., Nusbaum, C., Zody, M. C., Baldwin, J., et al. (2001). Initial Sequencing and Analysis of the Human Genome. Nature 409 (6822), 860–921. doi:10.1038/35057062
Lee, H.-M., and Choi, K.-C. (2018). Cigarette Smoke Extract and Isoprene Resulted in the Induction of Apoptosis and Autophagy in Human Placenta Choriocarcinoma JEG-3 Cells. Environ. Toxicol. 33 (2), 178–190. doi:10.1002/tox.22506
Lei, G.-S., Kline, H. L., Lee, C.-H., Wilkes, D. S., and Zhang, C. (2016). Regulation of Collagen V Expression and Epithelial-Mesenchymal Transition by miR-185 and miR-186 during Idiopathic Pulmonary Fibrosis. Am. J. Pathol. 186 (9), 2310–2316. doi:10.1016/j.ajpath.2016.04.015
Leuenberger, C., Schuoler, C., Bye, H., Mignan, C., Rechsteiner, T., Hillinger, S., et al. (2016). MicroRNA-223 Controls the Expression of Histone Deacetylase 2: A Novel axis in COPD. J. Mol. Med. 94 (6), 725–734. doi:10.1007/s00109-016-1388-1
Li, B. B., Chen, Y. l., and Pang, F. (2020). MicroRNA-30a Targets ATG5 and Attenuates Airway Fibrosis in Asthma by Suppressing Autophagy. Inflammation 43 (1), 44–53. doi:10.1007/s10753-019-01076-0
Li, S., Geng, J., Xu, X., Huang, X., Leng, D., Jiang, D., et al. (2016). miR-130b-3p Modulates Epithelial-Mesenchymal Crosstalk in Lung Fibrosis by Targeting IGF-1. PLoS One 11 (3), e0150418. doi:10.1371/journal.pone.0150418
Liang, H., Gu, Y., Li, T., Zhang, Y., Huangfu, L., Hu, M., et al. (2014a). Integrated Analyses Identify the Involvement of microRNA-26a in Epithelial-Mesenchymal Transition During Idiopathic Pulmonary Fibrosis. Cell Death Dis 5, e1238. doi:10.1038/cddis.2014.207
Liang, H., Liu, S., Chen, Y., Bai, X., Liu, L., Dong, Y., et al. (2016). miR-26a Suppresses EMT by Disrupting the Lin28B/let-7d Axis: Potential Cross-Talks Among miRNAs in IPF. J. Mol. Med. 94 (6), 655–665. doi:10.1007/s00109-016-1381-8
Liang, H., Xu, C., Pan, Z., Zhang, Y., Xu, Z., Chen, Y., et al. (2014b). The Antifibrotic Effects and Mechanisms of microRNA-26a Action in Idiopathic Pulmonary Fibrosis. Mol. Ther. 22 (6), 1122–1133. doi:10.1038/mt.2014.42
Lin, J., Feng, X., Zhang, J., and Tong, Z. (2019a). Long Noncoding RNA TUG1 Promotes Airway Smooth Muscle Cells Proliferation and Migration via Sponging miR-590-5p/FGF1 in Asthma. Am. J. Transl Res. 11 (5), 3159–3166.
Lin, L., Li, Q., Hao, W., Zhang, Y., Zhao, L., and Han, W. (2019b). Upregulation of LncRNA Malat1 Induced Proliferation and Migration of Airway Smooth Muscle Cells via miR-150-eIF4E/Akt Signaling. Front. Physiol. 10, 1337. doi:10.3389/fphys.2019.01337
Lino Cardenas, C. L., Henaoui, I. S., Courcot, E., Roderburg, C., Cauffiez, C., Aubert, S., et al. (2013). miR-199a-5p Is Upregulated During Fibrogenic Response to Tissue Injury and Mediates TGFbeta-Induced Lung Fibroblast Activation by Targeting Caveolin-1. Plos Genet. 9 (2), e1003291. doi:10.1371/journal.pgen.1003291
Liu, B., Jiang, T., Hu, X., Liu, Z., Zhao, L., Liu, H., et al. (2018a). Downregulation of microRNA-30a in B-ronchoalveolar L-avage F-luid from I-diopathic P-ulmonary F-ibrosis P-atients. Mol. Med. Rep. 18 (6), 5799–5806. doi:10.3892/mmr.2018.9565
Liu, B., Li, R., Zhang, J., Meng, C., Zhang, J., Song, X., et al. (2018b). MicroRNA-708-3p as a Potential Therapeutic Target via the ADAM17-Gata/stat3 Axis in Idiopathic Pulmonary Fibrosis. Exp. Mol. Med. 50 (3), e465. doi:10.1038/emm.2017.311
Liu, F., Qin, H.-B., Xu, B., Zhou, H., and Zhao, D.-Y. (2012). Profiling of miRNAs in Pediatric Asthma: Upregulation of miRNA-221 and miRNA-485-3p. Mol. Med. Rep. 6 (5), 1178–1182. doi:10.3892/mmr.2012.1030
Liu, H., Wang, B., Zhang, J., Zhang, S., Wang, Y., Zhang, J., et al. (2017). A Novel Lnc-PCF Promotes the Proliferation of TGF-Β1-Activated Epithelial Cells by Targeting miR-344a-5p to Regulate Map3k11 in Pulmonary Fibrosis. Cel Death Dis 8 (10), e3137. doi:10.1038/cddis.2017.500
Liu, L., Yin, H., Huang, M., He, J., Yi, G., Wang, Z., et al. (2016). miR-21 Promotes Pulmonary Fibrosis in Rats via Down-Regulating the Expression of ADAMTS-1. Xi Bao Yu Fen Zi Mian Yi Xue Za Zhi 32 (12), 1636–1640.
Liu, Y., Yang, K., Shi, H., Xu, J., Zhang, D., Wu, Y., et al. (2015). MiR-21 Modulates Human Airway Smooth Muscle Cell Proliferation and Migration in Asthma Through Regulation of PTEN Expression. Exp. Lung Res. 41 (10), 535–545. doi:10.3109/01902148.2015.1090501
Lu, Q., Guo, Z., Xie, W., Jin, W., Zhu, D., Chen, S., et al. (2018). The lncRNA H19 Mediates Pulmonary Fibrosis by Regulating the miR-196a/COL1A1 Axis. Inflammation 41 (3), 896–903. doi:10.1007/s10753-018-0744-4
Lu, T. X., Munitz, A., and Rothenberg, M. E. (2009). MicroRNA-21 Is Up-Regulated in Allergic Airway Inflammation and Regulates IL-12p35 Expression. J. Immunol. 182 (8), 4994–5002. doi:10.4049/jimmunol.0803560
Luly, F. R., Lévêque, M., Licursi, V., Cimino, G., Martin-Chouly, C., Théret, N., et al. (2019). MiR-146a Is Over-expressed and Controls IL-6 Production in Cystic Fibrosis Macrophages. Sci. Rep. 9 (1), 16259. doi:10.1038/s41598-019-52770-w
Lutful Kabir, F., Ambalavanan, N., Liu, G., Li, P., Solomon, G. M., Lal, C. V., et al. (2018). MicroRNA-145 Antagonism Reverses TGF-β Inhibition of F508del CFTR Correction in Airway Epithelia. Am. J. Respir. Crit. Care Med. 197 (5), 632–643. doi:10.1164/rccm.201704-0732OC
Ma, H., Wang, H., Luo, Y., Guo, S., and Song, C. (2017b). Mir-20b-Induced Increase in Myeloid-Derived Suppressor Cells in the Lungs of Mice with Chronic Asthma. Ann. Clin. Lab. Sci. 47 (1), 76–82.
Ma, H., Guo, S., Luo, Y., Wang, Y., Wang, H., He, J., et al. (2017a). MicroRNA-20b Promotes the Accumulation of CD11b+Ly6G+Ly6C Low Myeloid-Derived Suppressor Cells in Asthmatic Mice. cejoi 1 (1), 30–38. doi:10.5114/ceji.2017.67316
Maes, T., Cobos, F. A., Schleich, F., Sorbello, V., Henket, M., De Preter, K., et al. (2016). Asthma Inflammatory Phenotypes Show Differential microRNA Expression in Sputum. J. Allergy Clin. Immunol. 137 (5), 1433–1446. doi:10.1016/j.jaci.2016.02.018
Martinez, F. J., Collard, H. R., Pardo, A., Raghu, G., Richeldi, L., Selman, M., et al. (2017). Idiopathic Pulmonary Fibrosis. Nat. Rev. Dis. Primers 3, 17074. doi:10.1038/nrdp.2017.74
Masckauchán, T. N. H., Shawber, C. J., Funahashi, Y., Li, C.-M., and Kitajewski, J. (2005). Wnt/β-Catenin Signaling Induces Proliferation, Survival and Interleukin-8 in Human Endothelial Cells. Angiogenesis 8 (1), 43–51. doi:10.1007/s10456-005-5612-9
Matsushima, S., and Ishiyama, J. (2016). MicroRNA-29c Regulates Apoptosis Sensitivity via Modulation of the Cell-Surface Death Receptor, Fas, in Lung Fibroblasts. Am. J. Physiology-Lung Cell Mol. Physiol. 311 (6), L1050–L1061. doi:10.1152/ajplung.00252.2016
McKiernan, P. J., Molloy, K., Cryan, S. A., McElvaney, N. G., and Greene, C. M. (2014). Long Noncoding RNA Are Aberrantly Expressed In Vivo in the Cystic Fibrosis Bronchial Epithelium. Int. J. Biochem. Cel Biol. 52, 184–191. doi:10.1016/j.biocel.2014.02.022
Megiorni, F., Cialfi, S., Cimino, G., De Biase, R. V., Dominici, C., Quattrucci, S., et al. (2013). Elevated Levels of miR-145 Correlate with SMAD3 Down-Regulation in Cystic Fibrosis Patients. J. Cystic Fibrosis 12 (6), 797–802. doi:10.1016/j.jcf.2013.03.007
Megiorni, F., Cialfi, S., Dominici, C., Quattrucci, S., and Pizzuti, A. (2011). Synergistic Post-transcriptional Regulation of the Cystic Fibrosis Transmembrane Conductance Regulator (CFTR) by miR-101 and miR-494 Specific Binding. PLoS One 6 (10), e26601. doi:10.1371/journal.pone.0026601
Menezes, M. E., Mitra, A., Shevde, L. A., and Samant, R. S. (2012). DNAJB6 Governs a Novel Regulatory Loop Determining Wnt/β-Catenin Signalling Activity. Biochem. J. 444 (3), 573–580. doi:10.1042/BJ20120205
Ming, X., Duan, W., and Yi, W. (2019). Long Non-coding RNA NEAT1 Predicts Elevated Chronic Obstructive Pulmonary Disease (COPD) Susceptibility and Acute Exacerbation Risk, and Correlates with Higher Disease Severity, Inflammation, and Lower miR-193a in COPD Patients. Int. J. Clin. Exp. Pathol. 12 (8), 2837–2848.
Mizuno, S., Bogaard, H. J., Gomez-Arroyo, J., Alhussaini, A., Kraskauskas, D., Cool, C. D., et al. (2012). MicroRNA-199a-5p Is Associated with Hypoxia-Inducible Factor-1α Expression in Lungs From Patients with COPD. Chest 142 (3), 663–672. doi:10.1378/chest.11-2746
Mora, A. L., Rojas, M., Pardo, A., and Selman, M. (2017). Emerging Therapies for Idiopathic Pulmonary Fibrosis, a Progressive Age-Related Disease. Nat. Rev. Drug Discov. 16 (11), 755–772. doi:10.1038/nrd.2017.170
Morty, R. E., Konigshoff, M., and Eickelberg, O. (2009). Transforming Growth Factor- Signaling Across Ages: From Distorted Lung Development to Chronic Obstructive Pulmonary Disease. Proc. Am. Thorac. Soc. 6 (7), 607–613. doi:10.1513/pats.200908-087RM
Ngoc, L. P., Gold, D. R., Tzianabos, A. O., Weiss, S. T., and Celedón, J. C. (2005). Cytokines, Allergy, and Asthma. Curr. Opin. Allergy Clin. Immunol. 5 (2), 161–166. doi:10.1097/01.all.0000162309.97480.45
Nicodeme, E., Jeffrey, K. L., Schaefer, U., Beinke, S., Dewell, S., Chung, C.-w., et al. (2010). Suppression of Inflammation by a Synthetic Histone Mimic. Nature 468 (7327), 1119–1123. doi:10.1038/nature09589
Nowak, D. G., Cho, H., Herzka, T., Watrud, K., DeMarco, D. V., Wang, V. M. Y., et al. (2015). MYC Drives Pten/Trp53-Deficient Proliferation and Metastasis Due to IL6 Secretion and AKT Suppression via PHLPP2. Cancer Discov. 5 (6), 636–651. doi:10.1158/2159-8290.CD-14-1113
Oglesby, I. K., Bray, I. M., Chotirmall, S. H., Stallings, R. L., O’Neill, S. J., McElvaney, N. G., et al. (2010). miR-126 Is Downregulated in Cystic Fibrosis Airway Epithelial Cells and Regulates TOM1 Expression. J.I. 184 (4), 1702–1709. doi:10.4049/jimmunol.0902669
Oglesby, I. K., Chotirmall, S. H., McElvaney, N. G., and Greene, C. M. (2013). Regulation of Cystic Fibrosis Transmembrane Conductance Regulator by MicroRNA-145, -223, and -494 Is Altered in ΔF508 Cystic Fibrosis Airway Epithelium. J.I. 190 (7), 3354–3362. doi:10.4049/jimmunol.1202960
Oglesby, I. K., Vencken, S. F., Agrawal, R., Gaughan, K., Molloy, K., Higgins, G., et al. (2015). miR-17 Overexpression in Cystic Fibrosis Airway Epithelial Cells Decreases Interleukin-8 Production. Eur. Respir. J. 46 (5), 1350–1360. doi:10.1183/09031936.00163414
Ong, H. L., Brereton, H. M., Harland, M. L., and Barritt, G. J. (2003). Evidence for the Expression of Transient Receptor Potential Proteins in Guinea Pig Airway Smooth Muscle Cells. Respirology 8 (1), 23–32. doi:10.1046/j.1440-1843.2003.00424.x
Osei, E. T., Brandsma, C.-A., Timens, W., Heijink, I. H., and Hackett, T.-L. (2020). Current Perspectives on the Role of Interleukin-1 Signalling in the Pathogenesis of Asthma and COPD. Eur. Respir. J. 55 (2), 1900563. doi:10.1183/13993003.00563-2019
Pan, B., Xue, X., Zhang, D., Li, M., and Fu, J. (2017). SOX4 Arrests Lung Development in Rats with Hyperoxia-induced B-ronchopulmonary D-ysplasia by C-ontrolling EZH2 E-xpression. Int. J. Mol. Med. 40 (6), 1691–1698. doi:10.3892/ijmm.2017.3171
Panganiban, R. P., Pinkerton, M. H., Maru, S. Y., Jefferson, S. J., Roff, A. N., and Ishmael, F. T. (2012). Differential MicroRNA Epression in Asthma and the Role of miR-1248 in Regulation of IL-5. Am. J. Clin. Exp. Immunol. 1 (2), 154–165.
Pattarayan, D., Thimmulappa, R. K., Ravikumar, V., and Rajasekaran, S. (2018). Diagnostic Potential of Extracellular MicroRNA in Respiratory Diseases. Clinic Rev. Allerg Immunol. 54 (3), 480–492. doi:10.1007/s12016-016-8589-9
Perry, M. M., Adcock, I. M., and Chung, K. F. (2015). Role of MicroRNAs in Allergic Asthma. Curr. Opin. Allergy Clin. Immunol. 15 (2), 156–162. doi:10.1097/ACI.0000000000000147
Peschansky, V. J., and Wahlestedt, C. (2014). Non-coding RNAs as Direct and Indirect Modulators of Epigenetic Regulation. Epigenetics 9 (1), 3–12. doi:10.4161/epi.27473
Qi, X., Chen, H., Fu, B., Huang, Z., Mou, Y., Liu, J., et al. (2019). LncRNAs NR-026690 and ENST00000447867 Are Upregulated in CD4+ T Cells in Patients with Acute Exacerbation of COPD. Copd 14, 699–711. doi:10.2147/COPD.S191815
Qian, W., Cai, X., Qian, Q., Peng, W., Yu, J., Zhang, X., et al. (2019). lncRNA ZEB1-AS1 Promotes Pulmonary Fibrosis Through ZEB1-Mediated Epithelial-Mesenchymal Transition by Competitively Binding miR-141-3p. Cel Death Dis 10 (2), 129. doi:10.1038/s41419-019-1339-1
Qiu, Y.-y., Wu, Y., Lin, M.-j., Bian, T., Xiao, Y.-l., and Qin, C. (2019). LncRNA-MEG3 Functions as a Competing Endogenous RNA to Regulate Treg/Th17 Balance in Patients with Asthma by Targeting MicroRNA-17/ RORγt. Biomed. Pharmacother. 111, 386–394. doi:10.1016/j.biopha.2018.12.080
Qiu, Y. Y., Zhang, Y. W., Qian, X. F., and Bian, T. (2017). miR-371, miR-138, miR-544, miR-145, and miR-214 Could Modulate Th1/Th2 Balance in Asthma Through the Combinatorial Regulation of Runx3. Am. J. Transl Res. 9 (7), 3184–3199.
Qu, X., Dang, X., Wang, W., Li, Y., Xu, D., Shang, D., et al. (2018). Long Noncoding RNAs and mRNA Regulation in Peripheral Blood Mononuclear Cells of Patients with Chronic Obstructive Pulmonary Disease. Mediators Inflamm. 2018, 1–14. doi:10.1155/2018/7501851
Qu, Z.-H., Yang, Z.-C., Chen, L., Lv, Z.-D., Yi, M.-J., and Ran, N. (2012). Inhibition Airway Remodeling and Transforming Growth Factor-β1/Smad Signaling Pathway by Astragalus Extract in Asthmatic Mice. Int. J. Mol. Med. 29 (4), 564–568. doi:10.3892/ijmm.2011.868
Quinn, J. J., and Chang, H. Y. (2016). Unique Features of Long Non-coding RNA Biogenesis and Function. Nat. Rev. Genet. 17 (1), 47–62. doi:10.1038/nrg.2015.10
Ramachandran, S., Karp, P. H., Jiang, P., Ostedgaard, L. S., Walz, A. E., Fisher, J. T., et al. (2012). A MicroRNA Network Regulates Expression and Biosynthesis of Wild-type and F508 Mutant Cystic Fibrosis Transmembrane Conductance Regulator. Proc. Natl. Acad. Sci. 109 (33), 13362–13367. doi:10.1073/pnas.1210906109
Ramachandran, S., Karp, P. H., Osterhaus, S. R., Jiang, P., Wohlford-Lenane, C., Lennox, K. A., et al. (2013). Post-transcriptional Regulation of Cystic Fibrosis Transmembrane Conductance Regulator Expression and Function by microRNAs. Am. J. Respir. Cel Mol Biol 49 (4), 544–551. doi:10.1165/rcmb.2012-0430OC
Riordan, J. R., Rommens, J. M., Kerem, B.-S., Alon, N., Rozmahel, R., Grzelczak, Z., et al. (1989). Identification of the Cystic Fibrosis Gene: Cloning and Characterization of Complementary DNA. Science 245 (4922), 1066–1073. doi:10.1126/science.2475911
Saayman, S. M., Ackley, A., Burdach, J., Clemson, M., Gruenert, D. C., Tachikawa, K., et al. (2016). Long Non-coding RNA BGas Regulates the Cystic Fibrosis Transmembrane Conductance Regulator. Mol. Ther. 24 (8), 1351–1357. doi:10.1038/mt.2016.112
Savarimuthu Francis, S. M., Davidson, M. R., Tan, M. E., Wright, C. M., Clarke, B. E., Duhig, E. E., et al. (2014). MicroRNA-34c Is Associated with Emphysema Severity and Modulates SERPINE1 Expression. BMC Genomics 15, 88. doi:10.1186/1471-2164-15-88
Sawant, D., Yao, W., Wright, Z., Sawyers, C., Tepper, R., Gupta, S., et al. (2015). Serum MicroRNA-21 as a Biomarker for Allergic Inflammatory Disease in Children. Mirna 4 (1), 36–40. doi:10.2174/2211536604666150220232507
Schluger, N. W., and Koppaka, R. (2014). Lung Disease in a Global Context. A Call for Public Health Action. Ann. ATS 11 (3), 407–416. doi:10.1513/AnnalsATS.201312-420PS
Sharma, S., Tantisira, K., Carey, V., Murphy, A. J., Lasky-Su, J., Celedón, J. C., et al. (2010). A Role for Wnt Signaling Genes in the Pathogenesis of Impaired Lung Function in Asthma. Am. J. Respir. Crit. Care Med. 181 (4), 328–336. doi:10.1164/rccm.200907-1009OC
Shashkin, P., Simpson, D., Mishin, V., Chesnutt, B., and Ley, K. (2003). Expression of CXCL16 in Human T Cells. Atvb 23 (1), 148–149. doi:10.1161/01.atv.0000043906.61088.4b
Shen, J., Zhao, J., Ye, Q.-y., and Gu, X.-d. (2019). Interference of miR-943-3p with Secreted Frizzled-Related Proteins4 (SFRP4) in an Asthma Mouse Model. Cell Tissue Res 378 (1), 67–80. doi:10.1007/s00441-019-03026-6
Shen, W., Liu, J., Fan, M., Wang, S., Zhang, Y., Wen, L., et al. (2018). MiR-3202 Protects Smokers From Chronic Obstructive Pulmonary Disease Through Inhibiting FAIM2: An In Vivo and In Vitro Study. Exp. Cel Res. 362 (2), 370–377. doi:10.1016/j.yexcr.2017.11.038
Shen, W., Liu, J., Zhao, G., Fan, M., Song, G., Zhang, Y., et al. (2017). Repression of Toll-like Receptor-4 by microRNA-149-3p Is Associated with Smoking-Related COPD. Copd 12, 705–715. doi:10.2147/COPD.S128031
Shetty, S. K., Tiwari, N., Marudamuthu, A. S., Puthusseri, B., Bhandary, Y. P., Fu, J., et al. (2017). p53 and miR-34a Feedback Promotes Lung Epithelial Injury and Pulmonary Fibrosis. Am. J. Pathol. 187 (5), 1016–1034. doi:10.1016/j.ajpath.2016.12.020
Sonneville, F., Ruffin, M., Coraux, C., Rousselet, N., Le Rouzic, P., Blouquit-Laye, S., et al. (2017). MicroRNA-9 Downregulates the ANO1 Chloride Channel and Contributes to Cystic Fibrosis Lung Pathology. Nat. Commun. 8 (1), 710. doi:10.1038/s41467-017-00813-z
Statello, L., Guo, C.-J., Chen, L.-L., and Huarte, M. (2021). Gene Regulation by Long Non-coding RNAs and its Biological Functions. Nat. Rev. Mol. Cel Biol 22 (2), 96–118. doi:10.1038/s41580-020-00315-9
Stern, J., Pier, J., and Litonjua, A. A. (2020). Asthma Epidemiology and Risk Factors. Semin. Immunopathol 42 (1), 5–15. doi:10.1007/s00281-020-00785-1
Stolzenburg, L. R., Wachtel, S., Dang, H., and Harris, A. (2016). miR-1343 Attenuates Pathways of Fibrosis by Targeting the TGF-β Receptors. Biochem. J. 473 (3), 245–256. doi:10.1042/BJ20150821
Su, S., Zhao, Q., He, C., Huang, D., Liu, J., Chen, F., et al. (2015). miR-142-5p and miR-130a-3p Are Regulated by IL-4 and IL-13 and Control Profibrogenic Macrophage Program. Nat. Commun. 6, 8523. doi:10.1038/ncomms9523
Sultan, S., Rozzi, A., Gasparello, J., Manicardi, A., Corradini, R., Papi, C., et al. (2020). A Peptide Nucleic Acid (PNA) Masking the miR-145-5p Binding Site of the 3′UTR of the Cystic Fibrosis Transmembrane Conductance Regulator (CFTR) mRNA Enhances CFTR Expression in Calu-3 Cells. Molecules 25 (7), 1677. doi:10.3390/molecules25071677
Sun, J., Su, W., Zhao, X., Shan, T., Jin, T., Guo, Y., et al. (2019). LncRNA PFAR Contributes to Fibrogenesis in Lung Fibroblasts Through Competitively Binding to miR-15a. Biosci. Rep. 39 (7), BSR20190280. doi:10.1042/BSR20190280
Taft, R. J., Pang, K. C., Mercer, T. R., Dinger, M., and Mattick, J. S. (2010). Non-coding RNAs: Regulators of Disease. J. Pathol. 220 (2), 126–139. doi:10.1002/path.2638
Tang, K., Zhao, J., Xie, J., and Wang, J. (2019). Decreased miR-29b Expression Is Associated with Airway Inflammation in Chronic Obstructive Pulmonary Disease. Am. J. Physiology-Lung Cell Mol. Physiol. 316 (4), L621–L629. doi:10.1152/ajplung.00436.2018
Tang, W., Shen, Z., Guo, J., and Sun, S. (2016a). Screening of Long Non-coding RNA and TUG1 Inhibits Proliferation with TGF-β Induction in Patients with COPD. Copd 11, 2951–2964. doi:10.2147/COPD.S109570
Tang, Y., He, R., An, J., Deng, P., Huang, L., and Yang, W. (2016b). The Effect of H19-miR-29b Interaction on Bleomycin-Induced Mouse Model of Idiopathic Pulmonary Fibrosis. Biochem. Biophysical Res. Commun. 479 (3), 417–423. doi:10.1016/j.bbrc.2016.09.028
Tanjore, H., Degryse, A. L., Crossno, P. F., Xu, X. C., McConaha, M. E., Jones, B. R., et al. (2013). β-Catenin in the Alveolar Epithelium Protects from Lung Fibrosis after Intratracheal Bleomycin. Am. J. Respir. Crit. Care Med. 187 (6), 630–639. doi:10.1164/rccm.201205-0972OC
Tian, B., Yang, J., Zhao, Y., Ivanciuc, T., Sun, H., Garofalo, R. P., et al. (2017). BRD4 Couples NF-κB/RelA with Airway Inflammation and the IRF-RIG-I Amplification Loop in Respiratory Syncytial Virus Infection. J. Virol. 91 (6), e00007. doi:10.1128/JVI.00007-17
Tsuchiya, M., Kalurupalle, S., Kumar, P., Ghoshal, S., Zhang, Y., Lehrmann, E., et al. (2016). RPTOR, A Novel Target of miR-155, Elicits a Fibrotic Phenotype of Cystic Fibrosis Lung Epithelium by Upregulating CTGF. RNA Biol. 13 (9), 837–847. doi:10.1080/15476286.2016.1197484
Uhl, F. E., Vierkotten, S., Wagner, D. E., Burgstaller, G., Costa, R., Koch, I., et al. (2015). Preclinical Validation and Imaging of Wnt-Induced Repair in Human 3D Lung Tissue Cultures. Eur. Respir. J. 46 (4), 1150–1166. doi:10.1183/09031936.00183214
Viart, V., Bergougnoux, A., Bonini, J., Varilh, J., Chiron, R., Tabary, O., et al. (2015). Transcription Factors and miRNAs that Regulate Fetal to Adult CFTR Expression Change Are New Targets for Cystic Fibrosis. Eur. Respir. J. 45 (1), 116–128. doi:10.1183/09031936.00113214
Wang, J., Li, H.-Y., Wang, H.-S., and Su, Z.-B. (2018). MicroRNA-485 Modulates the TGF-β/ Smads Signaling Pathway in Chronic Asthmatic Mice by Targeting Smurf2. Cell Physiol Biochem 51 (2), 692–710. doi:10.1159/000495327
Wang, S.-H., Xu, F., Dang, H.-X., and Yang, L. (2013). Genetic Variations in the Wnt Signaling Pathway Affect Lung Function in Asthma Patients. Genet. Mol. Res. 12 (2), 1829–1833. doi:10.4238/2013.January.4.1
Wang, X., Cheng, Z., Dai, L., Jiang, T., Jia, L., Jing, X., et al. (2019). Knockdown of Long Noncoding RNA H19 Represses the Progress of Pulmonary Fibrosis Through the Transforming Growth Factor β/Smad3 Pathway by Regulating MicroRNA 140. Mol. Cel Biol 39 (12), e00143. doi:10.1128/MCB.00143-19
Wang, Y.-C., Liu, J.-S., Tang, H.-K., Nie, J., Zhu, J.-X., Wen, L.-L., et al. (2016). miR-221 Targets HMGA2 to Inhibit Bleomycin-Induced Pulmonary Fibrosis by Regulating TGF-β1/Smad3-Induced EMT. Int. J. Mol. Med. 38 (4), 1208–1216. doi:10.3892/ijmm.2016.2705
Weldon, S., McNally, P., McAuley, D. F., Oglesby, I. K., Wohlford-Lenane, C. L., Bartlett, J. A., et al. (2014). miR-31 Dysregulation in Cystic Fibrosis Airways Contributes to Increased Pulmonary Cathepsin S Production. Am. J. Respir. Crit. Care Med. 190 (2), 165–174. doi:10.1164/rccm.201311-1986OC
Who, (2021). WHO’s Global Health Estimates [Online]. World Health Organization. Available at: https://www.who.int/news-room/fact-sheets/detail/chronic-obstructive-pulmonary-disease-(copd (Accessed August 9, 2021).
Wu, S.-Q., Wang, G.-L., Li, L.-Y., and Ji, J. (2014a). Effects of microRNA-21 on the Interleukin 12/signal Transducer and Activator of Transcription 4 Signaling Pathway in Asthmatic Mice. cejoi 1 (1), 40–45. doi:10.5114/ceji.2014.42121
Wu, X. B., Wang, M. Y., Zhu, H. Y., Tang, S. Q., You, Y. D., and Xie, Y. Q. (2014b). Overexpression of microRNA-21 and microRNA-126 in the Patients of Bronchial Asthma. Int. J. Clin. Exp. Med. 7 (5), 1307–1312.
Wu, Y., Guan, S., Ge, Y., Yang, Y., Cao, Y., and Zhou, J. (2020). Cigarette Smoke Promotes Chronic Obstructive Pulmonary Disease (COPD) Through the miR-130a/Wnt1 axis. Toxicol. Vitro 65, 104770. doi:10.1016/j.tiv.2020.104770
Xie, T., Liang, J., Geng, Y., Liu, N., Kurkciyan, A., Kulur, V., et al. (2017). MicroRNA-29c Prevents Pulmonary Fibrosis by Regulating Epithelial Cell Renewal and Apoptosis. Am. J. Respir. Cel Mol Biol 57 (6), 721–732. doi:10.1165/rcmb.2017-0133OC
Xu, H., Sun, Q., Lu, L., Luo, F., Zhou, L., Liu, J., et al. (2017). MicroRNA-218 Acts by Repressing TNFR1-Mediated Activation of NF-Κb, Which Is Involved in MUC5AC Hyper-Production and Inflammation in Smoking-Induced Bronchiolitis of COPD. Toxicol. Lett. 280, 171–180. doi:10.1016/j.toxlet.2017.08.079
Yamakami, M., and Yokosawa, H. (2004). Tom1 (Target of Myb 1) Is a Novel Negative Regulator of Interleukin-1- and Tumor Necrosis Factor-Induced Signaling Pathways. Biol. Pharm. Bull. 27 (4), 564–566. doi:10.1248/bpb.27.564
Yamakami, M., Yoshimori, T., and Yokosawa, H. (2003). Tom1, a VHS Domain-Containing Protein, Interacts with Tollip, Ubiquitin, and Clathrin. J. Biol. Chem. 278 (52), 52865–52872. doi:10.1074/jbc.M306740200
Ye, S., Zhu, S., and Feng, L. (2020). LncRNA ANRIL/miR‐125a Axis Exhibits Potential as a Biomarker for Disease Exacerbation, Severity, and Inflammation in Bronchial Asthma. J. Clin. Lab. Anal. 34 (3), e23092. doi:10.1002/jcla.23092
Zemans, R. L., Briones, N., Campbell, M., McClendon, J., Young, S. K., Suzuki, T., et al. (2011). Neutrophil Transmigration Triggers Repair of the Lung Epithelium via -catenin Signaling. Proc. Natl. Acad. Sci. 108 (38), 15990–15995. doi:10.1073/pnas.1110144108
Zeng, X., Huang, C., Senavirathna, L., Wang, P., and Liu, L. (2017). miR-27b Inhibits Fibroblast Activation via Targeting TGFβ Signaling Pathway. BMC Cel Biol 18 (1), 9. doi:10.1186/s12860-016-0123-7
Zhang, D., Wu, Y., and Sun, G. (2018a). miR-192 Suppresses T Follicular Helper Cell Differentiation by Targeting CXCR5 in Childhood Asthma. Scand. J. Clin. Lab. Invest. 78 (3), 236–242. doi:10.1080/00365513.2018.1440628
Zhang, K., Liang, Y., Feng, Y., Wu, W., Zhang, H., He, J., et al. (2018b). Decreased Epithelial and Sputum miR-221-3p Associates with Airway Eosinophilic Inflammation and CXCL17 Expression in Asthma. Am. J. Physiology-Lung Cell Mol. Physiol. 315 (2), L253–L264. doi:10.1152/ajplung.00567.2017
Zhang, P.-x., Cheng, J., Zou, S., D'Souza, A. D., Koff, J. L., Lu, J., et al. (2015). Pharmacological Modulation of the AKT/microRNA-199a-5p/CAV1 Pathway Ameliorates Cystic Fibrosis Lung Hyper-Inflammation. Nat. Commun. 6, 6221. doi:10.1038/ncomms7221
Zhang, P., Wu, W., Chen, Q., and Chen, M. (2019). Non-Coding RNAs and Their Integrated Networks. J. Integr. Bioinform 16 (3), 20190027. doi:10.1515/jib-2019-0027
Zhang, Q., Ye, H., Xiang, F., Song, L.-J., Zhou, L.-L., Cai, P.-C., et al. (2017a). miR-18a-5p Inhibits Sub-pleural Pulmonary Fibrosis by Targeting TGF-β Receptor II. Mol. Ther. 25 (3), 728–738. doi:10.1016/j.ymthe.2016.12.017
Zhang, S., Liu, H., Liu, Y., Zhang, J., Li, H., Liu, W., et al. (2017b). miR-30a as Potential Therapeutics by Targeting TET1 Through Regulation of Drp-1 Promoter Hydroxymethylation in Idiopathic Pulmonary Fibrosis. Ijms 18 (3), 633. doi:10.3390/ijms18030633
Zhang, X.-y., Tang, X.-y., Ma, L.-j., Guo, Y.-l., Li, X.-s., Zhao, L.-m., et al. (2017c). Schisandrin B Down-Regulated lncRNA BCYRN1 Expression of Airway Smooth Muscle Cells by Improving miR-150 Expression to Inhibit the Proliferation and Migration of ASMC in Asthmatic Rats. Cell Prolif 50 (6), e12382. doi:10.1111/cpr.12382
Zhang, X. Y., Zhang, L. X., Tian, C. J., Tang, X. Y., Zhao, L. M., Guo, Y. L., et al. (2016a). LncRNAs BCYRN1 Promoted the Proliferation and Migration of Rat Airway Smooth Muscle Cells in Asthma via Upregulating the Expression of Transient Receptor Potential 1. Am. J. Transl Res. 8 (8), 3409–3418.
Zhang, Y., Xue, Y., Liu, Y., Song, G., Lv, G., Wang, Y., et al. (2016b). MicroRNA-146a Expression Inhibits the Proliferation and Promotes the Apoptosis of Bronchial Smooth Muscle Cells in Asthma by Directly Targeting the Epidermal Growth Factor Receptor. Exp. Ther. Med. 12 (2), 854–858. doi:10.3892/etm.2016.3427
Zhao, S., Lin, C., Yang, T., Qian, X., Lu, J., and Cheng, J. (2021). Expression of Long Non‐coding RNA LUCAT1 in Patients with Chronic Obstructive Pulmonary Disease and its Potential Functions in Regulating Cigarette Smoke Extract‐induced 16HBE Cell Proliferation and Apoptosis. J. Clin. Lab. Anal. 35 (7), e23823. doi:10.1002/jcla.23823
Zhao, X., Sun, J., Chen, Y., Su, W., Shan, H., Li, Y., et al. (2018). lncRNA PFAR Promotes Lung Fibroblast Activation and Fibrosis by Targeting miR-138 to Regulate the YAP1-Twist Axis. Mol. Ther. 26 (9), 2206–2217. doi:10.1016/j.ymthe.2018.06.020
Zhong, S., Chen, C., Liu, N., Yang, L., Hu, Z., Duan, P., et al. (2019). Overexpression of Hsa-miR-664a-3p Is Associated with Cigarette Smoke-Induced Chronic Obstructive Pulmonary Disease via Targeting FHL1. Copd 14, 2319–2329. doi:10.2147/COPD.S224763
Zhong, T., Perelman, J. M., Kolosov, V. P., and Zhou, X.-d. (2011). MiR-146a Negatively Regulates Neutrophil Elastase-Induced MUC5AC Secretion From 16HBE Human Bronchial Epithelial Cells. Mol. Cel Biochem 358 (1-2), 249–255. doi:10.1007/s11010-011-0975-2
Zhou, A.-y., Zhao, Y.-y., Zhou, Z.-j., Duan, J.-x., Zhu, Y.-z., Cai, S., et al. (2020). Microarray Analysis of Long Non-coding RNAs in Lung Tissues of Patients with COPD and HOXA-AS2 Promotes HPMECs Proliferation via Notch1. Copd 15, 2449–2460. doi:10.2147/COPD.S259601
Zhou, J., Lin, Y., Kang, X., Liu, Z., Zhang, W., and Xu, F. (2021). microRNA-186 in Extracellular Vesicles From Bone Marrow Mesenchymal Stem Cells Alleviates Idiopathic Pulmonary Fibrosis via Interaction with SOX4 and DKK1. Stem Cel Res Ther 12 (1), 96. doi:10.1186/s13287-020-02083-x
Zhu, M., Liang, Z., Wang, T., Chen, R., Wang, G., and Ji, Y. (2016). Th1/Th2/Th17 Cells Imbalance in Patients with Asthma With and Without Psychological Symptoms. Allergy Asthma Proc. 37 (2), 148–156. doi:10.2500/aap.2016.37.3928
Zhuang, Y., Dai, J., Wang, Y., Zhang, H., Li, X., Wang, C., et al. (2016a). MiR-338* Suppresses Fibrotic Pathogenesis in Pulmonary Fibrosis Through Targeting LPA1. Am. J. Transl Res. 8 (7), 3197–3205.
Keywords: microRNA, long noncoding RNA, asthma, chronic obstructive pulmonary disease, cystic fibrosis, idiopathic pulmonary fibrosis
Citation: Soni DK and Biswas R (2021) Role of Non-Coding RNAs in Post-Transcriptional Regulation of Lung Diseases. Front. Genet. 12:767348. doi: 10.3389/fgene.2021.767348
Received: 30 August 2021; Accepted: 25 October 2021;
Published: 08 November 2021.
Edited by:
Santosh Kumar, National Institute of Technology Rourkela, IndiaReviewed by:
Gopal Pandi, Madurai Kamaraj University, IndiaGiuseppe Biamonti, National Research Council (CNR), Italy
Copyright © 2021 Soni and Biswas. This is an open-access article distributed under the terms of the Creative Commons Attribution License (CC BY). The use, distribution or reproduction in other forums is permitted, provided the original author(s) and the copyright owner(s) are credited and that the original publication in this journal is cited, in accordance with accepted academic practice. No use, distribution or reproduction is permitted which does not comply with these terms.
*Correspondence: Roopa Biswas, cm9vcGEuYmlzd2FzQHVzdWhzLmVkdQ==