- 1National Center for Drug Research and Evaluation, Istituto Superiore di Sanità, Rome, Italy
- 2Department of Biomedical and Neuromotor Sciences, University of Bologna, Bologna, Italy
- 3Unit of Microscopic and Ultrastructural Anatomy, Department of Medicine, University Campus Bio-Medico, Rome, Italy
- 4Department of Clinical and Experimental Medicine, Center of Research and Innovation of Myeloproliferative neoplasms (CRIMM), AOU Careggi, University of Florence, Florence, Italy
- 5Department of Veterinary Medical Sciences, University of Bologna, Bologna, Italy
- 6Myeloproliferative Neoplasm Research Consortium, New York, NY, United States
- 7Department of Medicine and Surgery, University Campus Bio-Medico, Rome, Italy
The phenotype of mice carrying the Gata1low mutation that decreases expression of Gata1 in erythroid cells and megakaryocytes, includes anemia, thrombocytopenia, hematopoietic failure in bone marrow and development of extramedullary hematopoiesis in spleen. With age, these mice develop myelofibrosis, a disease sustained by alterations in stem/progenitor cells and megakaryocytes. This study analyzed the capacity of hGATA1 driven by a μLCR/β-globin promoter to rescue the phenotype induced by the Gata1low mutation in mice. Double hGATA1/Gata1low/0 mice were viable at birth with hematocrits greater than those of their Gata1low/0 littermates but platelet counts remained lower than normal. hGATA1 mRNA was expressed by progenitor and erythroid cells from double mutant mice but not by megakaryocytes analyzed in parallel. The erythroid cells from hGATA1/Gata1low/0 mice expressed greater levels of GATA1 protein and of α- and β-globin mRNA than cells from Gata1low/0 littermates and a reduced number of them was in apoptosis. By contrast, hGATA1/Gata1low/0 megakaryocytes expressed barely detectable levels of GATA1 and their expression of acetylcholinesterase, Von Willebrand factor and platelet factor 4 as well as their morphology remained altered. In comparison with Gata1+/0 littermates, Gata1low/0 mice contained significantly lower total and progenitor cell numbers in bone marrow while the number of these cells in spleen was greater than normal. The presence of hGATA1 greatly increased the total cell number in the bone marrow of Gata1low/0 mice and, although did not affect the total cell number of the spleen which remained greater than normal, it reduced the frequency of progenitor cells in this organ. The ability of hGATA1 to rescue the hematopoietic functions of the bone marrow of the double mutants was confirmed by the observation that these mice survive well splenectomy and did not develop myelofibrosis with age. These results indicate that hGATA1 under the control of µLCR/β-globin promoter is expressed in adult progenitors and erythroid cells but not in megakaryocytes rescuing the erythroid but not the megakaryocyte defect induced by the Gata1low/0 mutation.
Introduction
GATA1 is a member of the GATA family of transcription factors that ensures appropriate differentiation in hematopoietic cells of multiple lineages including erythroid (Tsai et al., 1989), megakaryocytic (Martin et al., 1990; Romeo et al., 1990), eosinophil (Yu et al., 2002a) and mast cells (Migliaccio et al., 2003). The lineages most stringently controlled by GATA1 are the erythroid and megakaryocytic ones (Orkin and Zon, 2008). In fact, genetically engineered alterations in mice and spontaneous mutations in humans are often associated with X-linked inherited disorders with erythroid and/or megakaryocytic phenotypes (Crispino and Weiss, 2004; Crispino and Horwitz, 2017). In mice, loss of Gata1 blocks the maturation of erythroid cells at the pro-erythroblast stage inducing the cells into apoptosis (Fujiwara et al., 2002) while selective loss of Gata1 expression in megakaryocytes (MK) arrests their terminal maturation and retains the cells in proliferation (Shivdasani et al., 1997; Vyas et al., 1999). Therefore, Gata1 deficient mice die of severe anemia between day 10.5 and 11.5 of gestation with sign of intraembryonic hemorrhage (McDevitt et al., 1997). In human, both inherited and acquired GATA1 mutations have been described. Point mutations suppressing the binding of the amino-terminal zinc-finger domain of the protein with either the DNA or the nuclear protein FOG1 are found in inherited disorders with erythroid or megakaryocyte deficiency (Nichols et al., 2000; Freson et al., 2001; Mehaffey et al., 2001; Yu et al., 2002b; Di Pierro et al., 2015; Iolascon et al., 2020). The good agreement between the phenotype of the patients and that of mice carrying the corresponding mutations (Campbell et al., 2013; Russo et al., 2017), indicates that these mutations are indeed responsible for sustaining the clinical traits. Based on extensive information indicating that in mice the ratio between the GATA1 and GATA2 content (Gata2/Gata1 switch) controls the proliferation of murine stem/progenitor cells (Bresnick et al., 2010), it is not surprising that acquired frame shift and point mutations impairing GATA1 functions induce leukemia in humans. Mutations resulting in the expression of a shorter amino-truncated protein (GATA1s) are associated with human Acute Megakaryocytic Leukaemia in Down syndrome (DS-AMKL) (Wechsler et al., 2002), transient myeloproliferative syndromes in newborns (Ahmed et al., 2004) and, in rare cases, with adult megakaryocytic leukemia (Harigae et al., 2004). In addition, ribosomopathies affecting the efficiency of translation of GATA1 mRNA are associated, in addition to Diamond Blackfan Anemia (Ludwig et al., 2014), with primary myelofibrosis, the most severe of the Philadelphia-negative myeloproliferative disorders (Vannucchi et al., 2005; Gilles et al., 2017).
The GATA family genes encode proteins very similar in structure and extremely conserved across species (Trainor et al., 1990). The specificity of their functions is therefore not dictated by the protein structure but rather by their accurate spatial-temporal expression during development and cytogenesis ensured by specific regulatory sequences (Ferreira et al., 2007). As an example, in mice loss of Gata1 function is rescued by gain of function of either Gata2 or Gata3, providing that their expression is driven by the regulatory sequence of Gata1 (Ferreira et al., 2005). The regulatory sequences of Gata1 include three hypersensitive sites which represent putative enhancers (Trainor et al., 1990; Ferreira et al., 2007). Their deletions are defined hypomorphic mutations because they reduce expression and protein content of Gata1 in a lineage-specific fashion. The most studied of these deletions are the Gata10.5 developed by the Yamamoto laboratory, that induce a transmissible erythroid leukemia in mice (Shimizu and Yamamoto, 2016), and the Gata1low mutation developed by the Orkin laboratory, that induce a lethal anemia and thrombocytopenia at birth in the C57BL6 strain (McDevitt et al., 1997; Vyas et al., 1999) and thrombocytopenia with myelofibrosis in CD1 mice (Vannucchi et al., 2002; Martelli et al., 2005). The phenotype induced by the Gata1low mutation in the CD1 background is particularly intriguing. Indeed, these mice are viable at birth because their anemia is rescued within 1 month by recruiting the spleen as extramedullary hematopoietic site (Migliaccio et al., 2009). In this strain, bone marrow hematopoiesis is ineffective as demonstrated by low hematopoietic stem cell content and by the fact that, when splenectomised, these mice die of profound anemia within 15 days (Migliaccio et al., 2009; Spangrude et al., 2016). However, the mice remain thrombocytopenic in spite of the high number of megakaryocytes present in their bone marrow and spleen, and with age develop a syndrome similar to human primary myelofibrosis characterized by fibrosis and hematopoietic failure in bone marrow, and development of hematopoiesis in extramedullary sites (Vannucchi et al., 2002).
To define the function of human GATA1 (hGATA1), two transgenic mice lines have been created carrying either the entire hGATA1 gene or its cDNA under the control of a μLCR coupled with the promoter of the β-globin gene (Peterson et al., 1993; Li et al., 2002). The line carrying the genomic hGATA1 dies between 10.5 and 12.5 days of gestation and, in spite of high expression of the transgene in erythroid cells, is anemic. By contrast, the line containing the μLCR/β-globin promoter coupled with hGATA1 cDNA (hereinafter referred to as hGATA1) is viable at birth (Li et al., 2002). This observation allowed the generation of hGATA1/β-YAC doubly hemizygous transgenic mice that were instrumental to demonstrate that hGATA1 is a specific repressor of the human ε gene in vivo but is dispensable for the expression of the human γ and β globin genes (Peterson et al., 1993). However, this demonstration was confounded by the fact that in these mice the hGATA1 cDNA was expressed at high levels in embryonic and fetal hematopoietic tissues but not in adult tissues (Li et al., 2002). The fact that a following study was capable to detect expression of a LacZ reporter driven by the μLCR/β-globin promoter in erythroid cells from adult transgenic mice (Papayannopoulou et al., 2000), raises the possibility that high levels of GATA1 protein, as a consequence of expression of both the hGATA1 transgene and the endogenous gene, may induce erythroid cells into apoptosis, providing a negative pressure which selects against cells expressing hGATA1. This possibility suggested us the hypothesis that the spectrum of cells in which hGATA1 is expressed is best identified in a genetic contest in which the expression of the endogenous gene is reduced. To test this hypothesis, we analyzed whether hGATA1 is expressed in hematopoietic cells from adult mice hypomorphic at the Gata1low locus, which contain low levels of the endogenous protein in the progenitor, erythroid and megakaryocyte cell compartments. The results obtained indicate that in the context of low GATA1 content, hGATA1 is expressed in adult erythroid progenitor and precursor cells at levels sufficient to rescue their defective phenotype. By contrast, hGATA1 was not expressed in cells of the closely related megakaryocyte lineage of these mutants, which remained abnormal, providing evidence that even in the contest of reduced expression of the endogenous gene, the μLCR/β-globin promoter is erythroid specific.
Materials and Methods
Generation of Double hGATA/Gata1low/0 Mutants
Transgenic hGATA1 (hGATA1/Gata1+/0) males generated as previously described (Li et al., 2002) were crossed with either CD1 (wild type at the Gata1 locus) or Gata1low/low females and their progeny genotyped by polymerase chain reaction (PCR) at birth, as previously described (Li et al., 2002; Vannucchi et al., 2002) (Table 1). Since Gata1 is on the X chromosome while μLCR/hGATA1 is autosomal, the offspring of these matings are all heterozygous for hGATA1 and heterozygous for Gata1low when females or hemizygous Gata1low/0 (wild type) when males. All the experiments were conducted on Gata1low/0 and Gata1+/0 males containing or not the hGATA1 transgene at 3–4 months of age, unless otherwise indicated. Mice were housed under good animal care practice conditions in the animal facilities of Istituto Superiore Sanità and the experiments were performed according to the protocol n. 419/2015-PR approved by the Italian Ministery of Health and according to the directive 2010/63/EU of the European Parliament and of the Council of September 22, 2010 on the protection of animals used for scientific purposes.
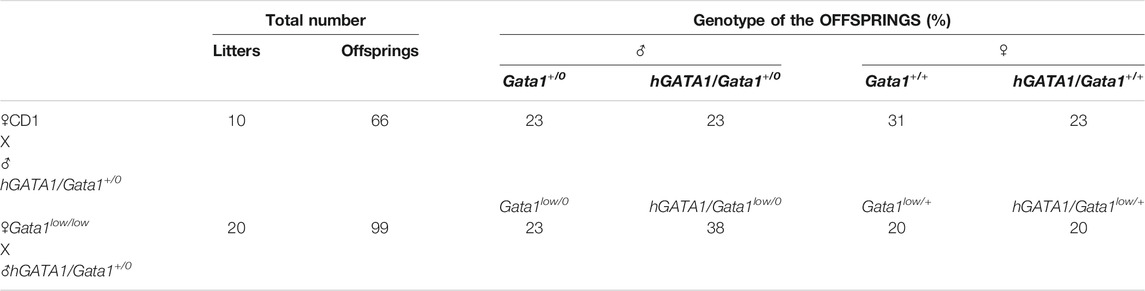
TABLE 1. Summary of the offsprings of the mating of CD1 or Gata1low/low mutants with the hGATA1 transgenic mice. hGATA1/Gata1+/0 males were crossed with either CD1 (Gata1+/0) or Gata1low/low females and their progeny genotyped. All the experiments described in the paper were carried out on Gata1low/0 and Gata1+/0 males, containing or not the hGATA1 transgene, highlighted in pink.
Splenectomy
The spleen was removed from mice anaesthetized with xylazine (10 mg/kg; Bayer) and ketamine (200 mg/kg; Gellini Pharmaceutics) by double ligation of the splenic artery and vein, as described in the animal protocol n. 419/2015-PR. See reference (Migliaccio et al., 2009) for further details.
Hematological Parameters
Mice were topically anesthetized with lidocaine (one drop/eye) and then blood was collected from the retro-orbital plexus into ethylen-diamino-tetracetic acid-coated microcapillary tubes (20–40 µL/sampling). Hematocrit (Hct) and platelet counts (Ptl) were determined manually.
Optical, Immunofluorescence and Electron Microscopy Observations
Histological (Hematoxylin and eosin, and Gomori) and Transmission Electron Microscopy (TEM) observations were performed as previously described (Vannucchi et al., 2002; Centurione et al., 2004). For immunofluorescence analysis, spleens were fixed in 10% (v/v) phosphate-buffered formalin, paraffin embedded and cut into 2.5–3 µM sections. Sections were incubated first with an anti-GATA1 monoclonal antibody (M-20, sc-1234, Santa Cruz Biotechnology, Santa Cruz, CA) and then with a fluorescein isothiocyanate (FITC)-conjugated anti-rat IgG antibody (Santa Cruz Biotechnology). After several washes, slides were mounted in glycerol-DABCO containing 4-6-diamidine-2-phenylindole (DAPI, 5 µg/ml) (Sigma-Aldrich, Saint Louis, MO) to counterstain the nuclei. Cells not incubated with the primary antibody served as negative controls. In selected experiments, GATA1 content was evaluated by immune-histochemistry with the same GATA1 antibody followed by peroxidase staining with the DAB CHROMOGEN kit (Bio Optica, Milan, Italy) followed by Hematoxylin staining. Consecutive sections, were instead processed for the terminal deoxy transferase uridine triphosphate nick end-labelling (TUNEL) reaction with the “In Situ Cell Death Detection Kit” (Boehringer Mannheim, Mannheim, Germany), as described by the manufacturer. At the end, slides were counter-stained with propidium iodide (Sigma-Aldrich). Samples were analyzed with a light microscope (Leica) equipped with a Coolsnap video camera for computerized images (RS Photometrics, Tucson, AZ, United States).
Flow Cytometry and Cell Sorting
Mononuclear cells obtained from liver and marrow and light density (ρ< 1.080) spleen cells separated over standard Ficoll gradient (Sigma-Aldrich) were suspended in Ca++ Mg++-free phosphate buffered saline supplemented with 1% (v/v) bovine serum albumin, 2 mM EDTA, 0.1% NaN3 and incubated for 30 min on ice with 1 µg/106 cells of phycoerythrin (PE)-conjugated CD117 (anti-cKit), CD71 and CD61, and fluorescein isothiocyanate (FITC)-conjugated anti-CD34, TER119 and CD41 (all from PharMingen, San Diego, CA). Apoptotic cells were detected by double staining with FITC-Annexin V (PharMingen) and propidium iodide (5 μg/ml). In selected experiments, erythroid cells (TER119pos/CD71pos), megakaryocytes (CD41pos/CD61pos), hematopoietic stem cell (LSK, lineage negative/CD117pos/Sca1pos) and erythroid-megakaryocyte (MEP, CD117pos/CD34neg) and myeloid (CMP/GMP, CD117pos/CD34pos) restricted progenitor cells were enumerated and eventually prospectively isolated as previously described (Akashi et al., 2000; Ghinassi et al., 2007). The sorted populations were 80–90% pure, upon re-analyses. Cell analysis and sorting were performed using the FACSAria cell sorter (Becton Dickinson, Franklin Lakes, NJ) with three lasers (488-nm argon laser, 599-nm dye laser and ultraviolet laser). Cells labeled with fluorophore-conjugated isotype antibodies (PharMingen) were used to gate non-specific fluorescence signals, while dead cells were excluded on the basis of propidium iodide (5 µg/ml, Sigma) staining.
Liquid Cultures Under Conditions of Limiting Dilution of Prospectively Isolated Progenitor Cells
MEP cells were cultured under conditions of limiting dilution (3 cells/mL) in 96-well plates containing Iscove modified Dulbecco medium (IMDM; Gibco, Invitrogen, Carlsband, CA) supplemented with fetal calf serum (FCS, 10% v/v; Sigma-Aldrich), 7.5 × 10−5 M β-mercaptoethanol (Sigma-Aldrich), penicillin, and streptomycin sulfate (50 U/mL, Gibco), and glutamine (2 mM, Gibco). Cultures were stimulated with a cocktail of growth factors contained rat SCF (100 ng/ml; Amgen, Thousand Oaks, CA), murine IL-3 (10 ng/ml, PeproTech, London, United Kingdom) and GM-CSF (10 ng/ml; PeproTech), human FLT3-ligand (10 ng/ml; PeproTech), IL-11 (10 ng/ml; PeproTech) in combination with either thrombopoietin (TPO, 50 ng/ml; PeproTech) (megakaryocyte-permissive conditions) or erythropoietin (EPO, 3 U/mL; Hoffman-La Roche, Basel, Switzerland) (erythroid-permissive conditions), as previously described (Ghinassi et al., 2007). The cultures were incubated at 37°C in a humidified incubator containing 5% CO2 in air. Cell growth and phenotype (erythroid: CD71pos/TER119pos, megakaryocyte: CD117pos/CD34neg, and mast cells: CD117pos/FcεRIpos by flow cytometry) were analyzed 7 days after the beginning of the culture, as described (Ghinassi et al., 2007).
Ribo Nucleic Acid Isolation and Quantitative Reverse Transcriptase-Polymerase Chain Reaction Analysis
Total RNA was prepared using a commercial guanidine thiocyanate/phenol method (TRIzol, Gibco BRL, Paisley, UK) as described by the manufacturer. Glycogen (20 μg, Roche Ltd., Basel, CH) was added to each sample as a carrier. cDNA was synthesized from 1 μg of total RNA using random primers and SuperScript III (Invitrogen, Life Technologies, Bethesda, MD). Quantitative PCR was carried out as recommended by the manufacture with TaqMan PCR kits specific for the human or the murine Gata1 gene, or additional erythroid (Hba-a1 and Hbb-b1) or megakaryocytic (Ache, vWF and Pf4) specific genes (Applied Biosystems, Foster City, CA), with the ABI PRISM 7700 Sequence Detection System (Applied Biosystems). For each gene (X) quantitative values were obtained from the threshold cycle number (Ctx), and expressed as 2−ΔCtx, were ΔCtx is the difference between the average Ct of the target X gene from that of the housekeeping gene (Gapdh).
Statistical Analysis
Statistical analysis was performed by one-way analysis of variance Dunnett’s Multiple Comparison and one-way analysis of variance Tukey Multiple Comparison Test, as indicated. The analyses were performed with GraphPad Prism 5 software for Windows (GraphPad Software, San Diego, California United States).
Results
Mendelian Inheritance of the hGATA1 Transgene
Males wild type at the Gata1 locus (Gata1+/0) carrying the hGATA1 transgene (hGATA1/Gata1+/0) were crossed with either CD1 (wild-type at the Gata1 locus) or Gata1low/low females. For each crossing, 10 and 20 separate matings, respectively, were performed. These crossing generated a total of 66 and 99 pups with an average number of pups per mating of 6.6 and 4.9 for CD1 and Gata1low/low mating, respectively (Table 1). The genotype of the offspring was distributed according to the expected Mendelian ratios. The slight prevalences of wild-type females not carrying hGATA1 and of hGATA1/Gata1low/0 males are not statistically significant. The Mendelian inheritance of the transgene was associated with a normal phenotype of the embryos (Figure 1). In fact, at both E11.5 and E13.5, Gata1low embryos were reported to be pale with clear evidence of anemia (McDevitt et al., 1997). Consistently with this observation, by E11.5 Gata1low/0 embryos mice were pale, an indication of insufficient red blood cell production (Figure 1A) while E11.5 hGATA1/Gata1low/0 embryos had a normal morphology, which included a red appearance of the fetal liver (Figure 1B).
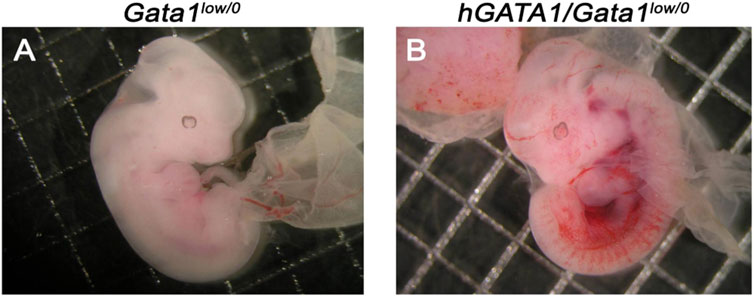
FIGURE 1. Fetuses from GATA1low/0 mice carrying the hGATA1 transgene are not anemic. Representative photograph of 11.5 days fetuses from the same litter. The genotype of the fetuses is indicated on the top. Compared to age-matched Gata1low/0 mice (A), the double mutant hGATA1/Gata1low/0 mice (B) are not anemic. Results are representative of those observed with 5 fetuses per genotype.
The hGATA1 Transgene Rescues the Red Cell Counts of the Blood From Adult Gata1low Mice
The hematocrit (Hct) and platelet counts of the Gata1+/0, Gata1low/0, hGATA1/Gata1+/0 and hGATA/Gata1low/0 mice are compared in Figure 2A. As expected (McDevitt et al., 1997; Vannucchi et al., 2001), Gata1low/0 males expressed levels of Hct and platelet counts significantly lower than normal. The presence of the hGATA1 transgene restored the Hct of the Gata1low/0 mutants up to normal values but had no effects on platelet counts that remained low. This platelet deficiency was confirmed by morphological evaluation that showed reduced number of platelets with size greater than normal (megathrombocytes) in blood smears not only as expected in Gata1low/0 mice (McDevitt et al., 1997) but also in their hGATA/Gata1low/0 littermates (Figures 2B,C).
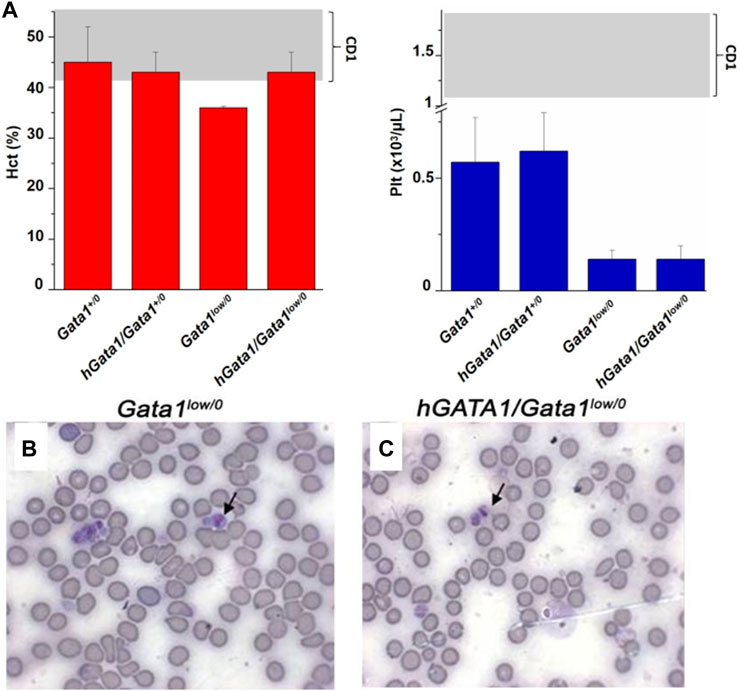
FIGURE 2. The hGATA1 transgene rescues the hematocrit (Hct) but not the platelet counts of GATA1low/0 mice. (A) Hct and platelet counts of 4 months-old wild-type (Gata1+/0) mice and Gata1low/0 mice carrying or not the hGATA1 transgene, as indicated. The presence of the hGATA1 transgene restores the Hct of Gata1low mice to normal values but does not alter their platelet counts, which remain lower than normal. The shaded areas indicate the range of Hct and Ptl observed in CD1 males available from commercial sources (https://www.criver.com/sites/default/files/resources/CD1IGSMouseModelInformationSheet.pdf). Results are expressed as Mean (±SD) of those obseved with 5 mice per experimental group. Statistical analyses was performed by One-way analysis of variance Tukey Multiple Comparison Test. Results of the statistical analyses: Hct = Gata1+/0 vs Gata1low/0 p < 0.05, other values are not statistically different; Ptl = Gata1+/0 vs. Gata1low/0 p < 0.05, Gata1+/0 vs. hGATA1/Gata1low/0 p < 0.05, hGata1/Gata1+/0 vs. Gata1low/0 p < 0.05 and hGata/Gata1+/0 vs. hGata1/Gata1low/0 p < 0.05. (B,C) Representative blood smears of the blood from 4 months old Gata1low/0 (B) and hGATA1/Gata1low/0 (C) littermates showing the presence of megathrombocytes in both.
These results indicate that the presence of hGATA1 rescues the anemia but not the thrombocytopenia induced by the Gata1low mutation.
hGATA1 is Expressed in Adult Hematopoietic Progenitor and Precursor Cells.
By quantitative RT-PCR, we compared the levels of hGATA1 and of the endogenous murine Gata1 (mGata1) gene expressed by adult hematopoietic progenitor and precursors cells prospectively isolated from the bone marrow of adult Gata1+/0, Gata1low/0 and hGATA1/Gata1low/0 mice (Figure 3). Human erythroblasts (CD235pos/CD36pos cells), expanded in vitro as previously described (Federici et al., 2019), were analyzed in parallel as positive control for hGATA1 expression. We measured only the total level of Gata1 mRNA because in humans the ribosomopathy induced by the driver mutations found in myelofibrosis equally reduces the megakaryocyte content of the full-length and short isoform of the GATA1 protein (Vannucchi et al., 2005; Gilles et al., 2017).
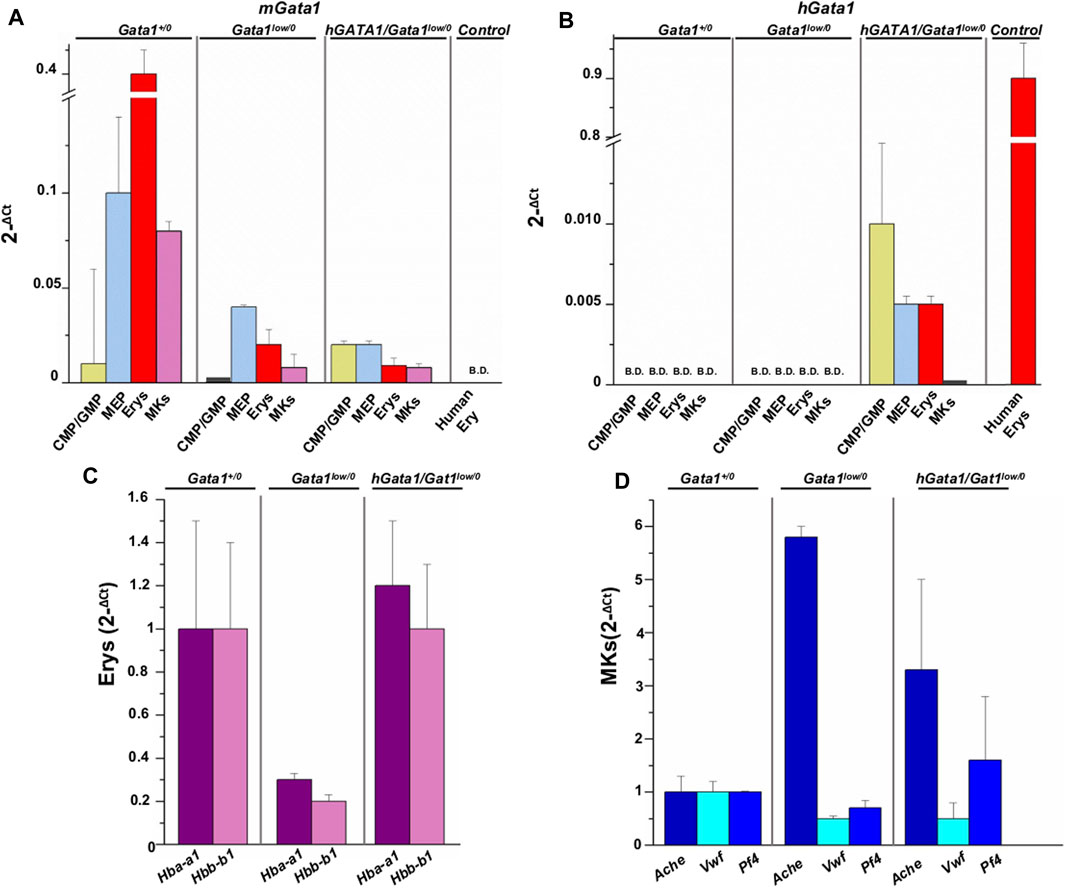
FIGURE 3. hGATA1 is expressed in erythroid but not in megakaryocyte precursors prospectively isolated from hGATA1/Gata1+/0 mice. Quantitative RT-PCR expression analysis of murine Gata1 (mGata1) (A) and hGATA1 (B) mRNA in precursor and progenitor cells prospectively isolated from Gata1+/0, Gata1low/0 and hGATA1/Gata1low/0 mice according to the phenotypes: CD117pos/CD34pos (CMP/GMP), CD117pos/CD34neg (MEP), TER119pos/CD71pos (Erys) and CD41pos/CD61pos (MKs. The levels of the erythroid specific genes Hba-a1 and Hbb-b1 (α- and β-globin) and of the megakaryocyte specific genes Ache, Vwf and Pf4 (acetylcholinesterase, Von Willebrand factor and platelet factor 4) expressed by the Erys and MKs of the four experimental groups are presented in (C,D), respectively. Data are expressed as 2−ΔCt and are presented as Mean (±SD) of those obseved with cells independently isolated in 3 different experiments each with one mouse per group. Ex vivo expanded human erythroblasts (CD235apos/CD36pos cells) served as control for the expression of hGATA1. Statistical analyses were performed by One-way analysis of variance Tukey Multiple Comparison Test. Results of the statistical analyses: in (A,B): CMP/GMP: Statistically non-significant; MEP: Gata1+/0 vs. Gata1low/0 p < 0.05; Gata1+/0 vs. hGATA1/Gata1low/0 p < 0.01; Erys: Statistically non-significant; MKs: Gata1+/0 vs. Gata1low/0 p < 0.001 and Gata1+/0 vs. hGATA1/Gata1low/0 p < 0.001. In (C,D): Erys = Hba-a1: Gata1low/0 vs. hGATA1/Gata1low/0 p < 0.05; Hbb-b1: Gata1+/0 vs. Gata1low/0 and Gata1low/0 vs. hGATA1/Gata1low/0 p < 0.05; MKs = Ache: Gata1+/0 vs. Gata1low/0p < 0.01; Vwf: Gata1+/0 vs. Gata1low/0 p < 0.01; Gata1+/0 vs. hGATA1/Gata1low/0 p < 0.01.
As expected (Ling et al., 2018), CMP (by ∼25-fold), MEP (by ∼2.5-fold), erythroid precursors (by ∼20-fold) and megakaryocytes (by ∼10-fold) from Gata1low/0 mice expressed significantly lower levels of mGata1 mRNA than the corresponding cells from their Gata1+/0 littermates. The presence of hGATA1 did not affect expression of the endogenous gene in these populations purified from hGATA1/Gata1low/0 mice, which remained lower than normal (Figure 3).
Unsurprisingly, hGATA1 was not detected in cells prospectively isolated from Gata1+/0 or Gata1low/0 littermates but it was expressed already at the level of progenitor cells (both CMP and MEP) prospectively isolated from hGATA1/Gata1low/0 mice. In these mice, expression of hGATA1 persisted at the level of the erythroid precursors while it was barely detectable in megakaryocytes (Figure 3).
These results indicate that in the Gata1low/0 genetic contest, the expression of hGATA1 driven by the μLCR coupled with the β-globin gene promoter is restricted to progenitor and erythroid cells and that its expression does not affect the levels of the endogenous gene which remain lower than normal.
hGATA1 Rescues the Defective Phenotype of Erythroid Cells but not That of Megakaryocytes from Gata1low/0 Mice.
In accordance to the Gata1low levels detected by RT-PCR (Figure 3), by immunofluorescence, erythroid cells in the spleen from Gata1low/0 mice contain low levels of GATA1 protein (Figure 4A). By contrast, the erythroid cells in the spleen from mice containing the hGATA1 transgene were more intensively green fluorescent indicating a higher GATA1 content. The GATA1 protein is synthesized in the cytoplasm and translocate in the nucleus as part of the NuRSERY complex formed by HADC5, GATA1, EKLF and ERK in response to factors that phosphorylate ERK (Varricchio et al., 2014). It is not surprising, then, that the GATA1 fluorescent signal was detected both in the cytoplasm and in the nucleus of the cells (see also Supplementary Figure S1). Of note, the fluorescence intensity is particularly evident in the nuclei of the hGATA1/Gata1low/0 mutants. The antibody used to detect GATA1 does not discriminate between the murine and the human protein. Since the levels of the endogenous mGata1 expressed by erythroid cells from Gata1low/0 and hGATA1/Gata1low/0 mice is the same (Figure 3), we believe that the greater levels of GATA1 protein detected in the erythroid cells from the double mutants is the result of hGATA1 expression.
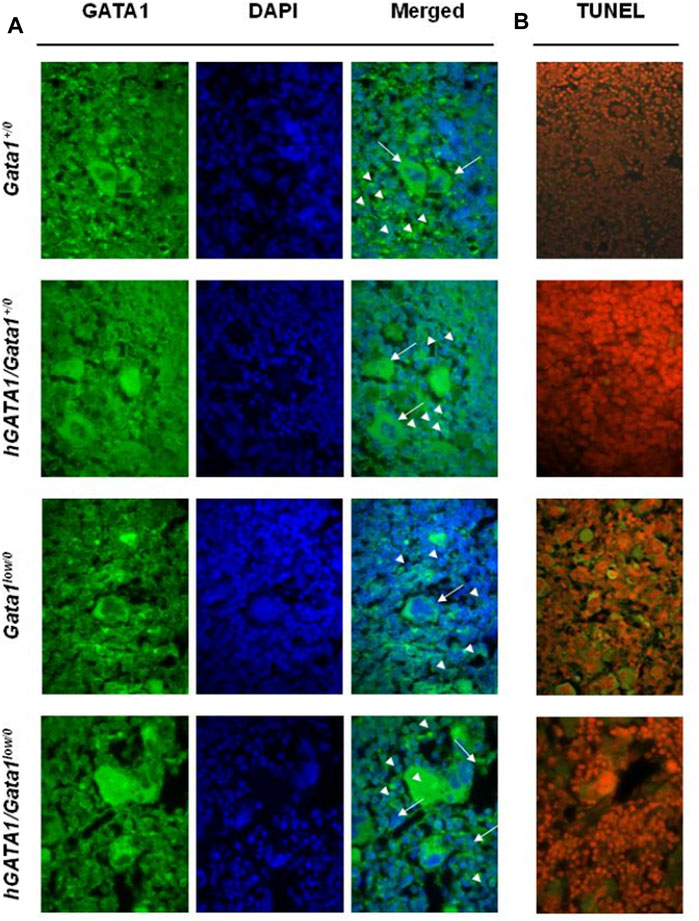
FIGURE 4. Erythroid cells from adult hGATA1/GATA1low/0 double mutants contain high levels of GATA1 protein and express low apoptotic rates. (A) Representative confocal microscopy analysis of GATA1 content of spleen sections from wild-type and Gata1low/0 mice carrying or not the hGATA1 transgene, as indicated. Arrowheads and arrows indicate representative erythroid and megakaryocytes cells, respectively. GATA1 content (green fluorescence) increases in the nuclei of erythroid cells from hGATA1/Gata1low/0 mice whereas remains low in their megakaryocytes. (B) Confocal microscopy analysis by TUNEL assay indicates reduced number of apoptotic cells in the spleen from hGATA1/Gata1low/0 compared to those present in Gata1low/0 mice. Results are representative of those observed with 3 mice per experimental groups. Similar results were observed by immunostaining with 2 additional mice per experimental groups (Supplementary Figure S1A). Original Magnification 64X.
Despite extramedullary hematopoiesis in the spleen corrects erythropoiesis of Gata1low mice (Migliaccio et al., 2009), these animals remain anemic due to the increased apoptotic rates of their erythroid cells, detected by TUNEL staining of this organ (Vannucchi et al., 2001). In order to assess whether the greater GATA1 content of the erythroid cells from the spleen of double mutants rescued their increased apoptosis rates, TUNEL staining was performed. As expected, TUNEL staining was limited in the spleen from wild type mice but it greatly increased in the erythroblasts from the spleen of Gata1low/0 mutants (Figure 4B). Since the localization of the TUNEL staining is expected to be perinuclear (Vannucchi et al., 2001 and Supplementary Figure S1B), it is not surprising that at the magnification presented in Figure 4B the Tunnel signal is observed in the cytoplasm.
By contrast, the presence of the hGATA1 transgene drastically reduced the frequency of TUNEL-positive cells present in spleen sections from the double mutant mice (Figure 3B). These results were confirmed at the bone marrow level by flow cytometry determinations showing that erythroid cells from double mutant mice, by contrast with those from their Gata1low/0 counterpart, are barely stained by Annexin V. In fact, the frequency of Annexinpos Ter119pos cells in the bone marrow was 25.58 ± 13.8 (n = 4) in Gata1low/0 mice vs. 4.17 ± 5.22 in hGATA1Gata1low/0 mice (n = 6) (p = 0.0078 by ANOVA) while the frequency of Annexinpos Ter119pos cells in the bone marrow from two Gata1+/0 mice analysed in parallel was 0.5 and 0.7.
In agreement with the observation that hGATA1 mRNA was similarly undetectable in megakaryocytes from Gata1low/0 and hGATA1/Gata1low/0 littermates (Figure 3), immunofluorescence determinations indicated that the GATA1 protein was barely detectable in the megakaryocytes from the spleen of these experimental groups (Figure 4A and Supplementary Material S1A).
To confirm that hGATA1 rescues erythropoiesis in the bone marrow of Gata1low/0 mice, the expression of specific erythroid and megakaryocytic genes was assessed in erythroid cells and megakaryocytes prospectively isolated from the bone marrow of Gata1+/0, Gata1low/0 and hGATA1/Gata1low/0 mice (Ghinassi et al., 2007). Compared to wild type mice, erythroid cells from Gata1low/0 mice expressed significantly lower levels of α- and β-globins, that were restored up to normal levels in cells from the double mutant (Figure 3C). By contrast, in megakaryocytes the presence of hGATA1 transgene did not affect the expression of acetylcholinesterase, Von Willebrand factor, and platelet factor 4 in hGATA1/Gata1low/0 mice which remained similar to that detected in cells from Gata1low/0 mice and abnormally greater than that of cells from Gata1+/0 littermates (Figure 3D).
In conclusion, the observation that erythroid cells but not the megakaryocytes from the double mutant mice contain levels of GATA1 protein similar to normal provides a mechanistic insight to explain why the presence of hGATA1 rescues the abnormal maturation of erythroid cells but not that of megakaryocytes induced by the Gata1low mutation.
The hGATA1 Transgene Induces Extramedullary Hematopoiesis in Both Spleen and Liver
In Gata1low mice hematopoiesis fails in the bone marrow and is active in the spleen which contains most of the hematopoietic stem cells of these animals (Spangrude et al., 2016). To assess the effect of the hGATA1 transgene on the active site of hematopoiesis, total cell number and frequency of hematopoietic stem/progenitor and precursors cells in bone marrow, spleen and liver from Gata1+/0, hGATA1/Gata1+/0, Gata1low/0 and hGATA1/Gata1low/0 mice were compared (Figure 5). Hematopoietic stem/progenitor cells are defined as Lin−/CD117pos/Sca1pos cells (LSK) and divided by CD34 staining into bipotent erythro-megakaryocytic (MEP, CD34neg) and common myeloid/bipotent granulo-monocytic (CMP/GMP, CD34pos) progenitor cells (Akashi et al., 2000; Ghinassi et al., 2007).
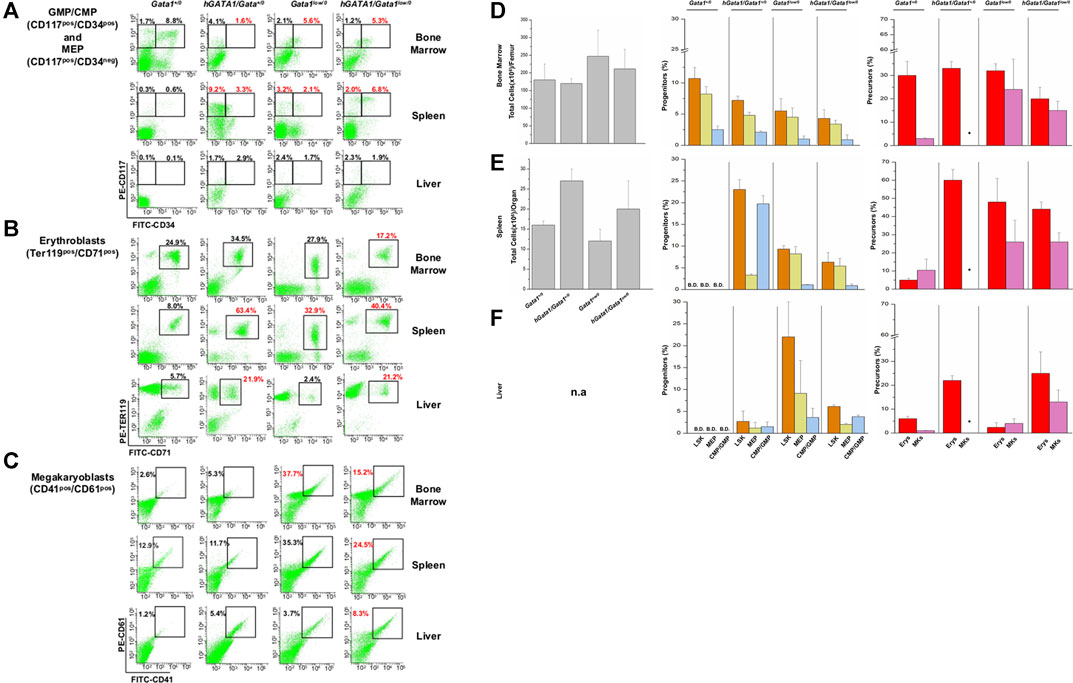
FIGURE 5. The presence of hGATA1 increases the frequency of hematopoietic cells in the bone marrow and liver of Gata1low/0 mice and in the liver of wild type (Gata1+/0) mice. (A–C) Gates used to identify GMP/CMP and MEP, erythroblasts and megakaryocytes in organs from representative Gata1+/0, hGATA1/Gata1+/0, Gata1low/0 and hGATA1/Gata1low/0 mice are indicated in panel (A–C), respectively. The numbers on the top of the gates indicate the frequency of the cell population in that particular experiment. (D–F) Total cell numbers (left panels) and frequency of stem/progenitors cells (LSK, Lin−/CD117pos/Sca1pos, CMP/GMP, CD117pos/CD34pos, MEP, CD117pos/CD34neg) (middle panels) and of erythroid (Erys, TER119pos/CD71pos) and megakaryocyte (MKs, CD41pos/CD61pos) precursors (right panels) in the femur (D), spleen (E) and liver (F) from Gata1+/0, hGATA1/Gata1+/0, Gata1low/0 and hGATA1/Gata1low/0 mice. Results are expressed as Mean (±SD) of those obseved with 3 mice per experimental group. Statistical analyses was performed by One-way analysis of variance Tukey Multiple Comparison Test. Results of the statistical analyses: Bone marrow = total cell number: Gata1+/0 vs hGATA1/Gata1+/0 p < 0.05 and hGATA1/Gata1+/0 vs. Gata1low/0 p < 0.01; LSK: Gata1+/0 vs. Gata1low/0 p < 0.05 and Gata1+/0 vs. hGATA1/Gata1+/0 p < 0.01; CMP/GMP: Gata1+/0 vs. hGATA1/Gata1+/0 p < 0.05 and Gata1+/0 vs. Gata1low/0 p < 0.05 and Gata1+/0 vs. hGATA1/Gata1low/0 p < 0.01; MEP: Gata1+/0 vs. Gata1low/0 p < 0.05 and Gata1+/0 vs. hGATA1/Gata1low/0 p < 0.05; Erys: hGATA1/Gata1+/0 vs. hGATA1/Gata1low/0 p < 0.05 and Gata1low/0 vs. hGATA1/Gata1low/0 p < 0.05, MKs: Gata1+/0 vs. Gata1low/0 p < 0.05. Spleen = total cell number: Statistically non-significant; LSK: Gata1+/0 vs. hGATA1/Gata1+/0 p < 0.001, Gata1+/0 vs. Gata1low/0 p < 0.001, Gata1+/0 vs. hGATA1/Gata1low/0 p < 0.01, hGATA1/Gata1+/0 vs. Gata1low/0 p < 0.001 and hGATA1/Gata1+/0 vs. hGATA1/Gata1low/0 p < 0.001; CMP/GMP: Gata1+/0 vs. hGATA1/Gata1+/0 p < 0.05, Gata1+/0 vs. Gata1low/0 p < 0.001, Gata1+/0 vs. hGATA1/Gata1low/0 p < 0.01 and hGATA1/Gata1+/0 vs. Gata1low/0 p < 0.01; MEP: Gata1+/0 vs. hGATA1/Gata1+/0 p < 0.001, hGATA1/Gata1+/0 vs. Gata1low/0 p < 0.001 and hGATA1/Gata1+/0 vs. hGATA1/Gata1low/0 p < 0.001; Erys: Gata1+/0 vs. hGATA1/Gata1+/0 p < 0.001, Gata1+/0 vs. Gata1low/0 p < 0.001 and Gata1+/0 vs. hGATA1/Gata1low/0 p < 0.001; MKs: Statistically non-significant. Liver = LSK: Gata1+/0 vs. hGATA1/Gata1low/0 p < 0.01, hGATA1/Gata1+/0 vs. hGATA1/Gata1low/0 p < 0.05 and Gata1low/0 vs hGATA1/Gata1low/0 p < 0.05; CMP/GMP: Statistically non-significant; MEP: Statistically non-significant; Erys: Gata1+/0 vs. hGATA1/Gata1+/0 p < 0.05, Gata1+/0 vs. hGATA1/Gata1low/0 p < 0.01, hGATA1/Gata1+/0 vs. Gata1low/0 p < 0.01 and Gata1low/0 vs. hGATA1/Gata1low/0 p < 0.01; MKs: Gata1+/0 vs. hGATA1/Gata1low/0 p < 0.01 and Gata1low/0 vs. hGATA1/Gata1low/0 p < 0.01.
Bone marrow. As previously reported (Vannucchi et al., 2002), the bone marrow from Gata1low/0 mice contained statistically significant lower numbers of cells than that from wild type mice (Figure 5). Significantly lower than normal were also the frequencies of GMP/CMP while the slightly lower frequency of MEP was not statistically significant. At the precursor level, the frequency of erythroid cells was within normal values while that of megakaryocytes, as expected (Vannucchi et al., 2002), was greater than normal. The presence of hGATA1 had a small not significant impact on the cellularity of the femur from Gata1low/0 animals. However, it significantly decreased the frequency of erythroid precursors in this organ (a sign of increased maturation) and had no effect on the frequency of progenitor cells and megakaryocytes that remained, respectively, lower and higher than normal (Figure 5).
Spleen. As reported (Vannucchi et al., 2002), the total cell number of the spleen from Gata1low/0 mice was about 0.4-fold greater than normal (Figure 5). However, due to great variability in this organ size among individual mice, there was insufficient power to demonstrate statistically significant differences with the low number (three) of mice analyzed per group. The spleen from these mice contained greater frequencies of progenitor and precursor cells than that of hemizygote Gata1+/0 mice in which these cell populations were barely detectable. The presence of hGATA1 dramatically increased the frequency of LSK and progenitor cells and that of erythroid precursors in the spleen from Gata1+/0 mice. However, although the frequency of these cell populations in the spleen from hGATA1/Gata1low/0 remained greater than that of wild type mice, it was significantly lower when compared to that of hGATA1/Gata1+/0 mice (Figure 5).
Liver. Although the total number of cells in the liver of the various mice groups was not recorded, the liver from transgenic mice containing hGATA1 appeared pale, an indication of reduced vascularization, and with a gummy consistency, an indication of altered tissue architecture (data not shown). In agreement with these observations, the liver from mice containing the human transgene, hGATA1/Gata1+/0 and hGATA1/Gata1low/0 alike, contained detectable numbers of LSK and hematopoietic progenitors, and significantly higher numbers of erythroid and megakaryocyte precursors compared to wild type mice (Figure 5). Of note, although the liver from Gata1low/0 mice contained the greatest number of progenitor cells, the frequency of the erythroid and megakaryocyte precursors in this organ is very low, confirming that the liver is not an active hematopoietic site in Gata1low/0 mice at least at the age analyzed (4-months of age) (Vannucchi et al., 2002).
These results indicate that the presence of hGATA1 induces hematopoiesis in the bone marrow and reduces that in the spleen in mice carrying the Gata1low mutation.
hGATA1 Rescues the Altered Differentiation Potential Expressed in vitro by Erythroid-Megakaryocyte Carrying the Gata1low Mutation
In a previous publication, we demonstrated that the Gata1low mutation increases the proliferation potential of MEP which acquire at the single cell level the ability to generate in vitro, in addition to erythroid cells and megakaryocytes, also mast cells with the phenotype CD117pos/FcεRIpos (Ghinassi et al., 2007). To clarify whether the expression of the transgene rescues the differentiation potential of Gata1low/0 MEP, the cells were prospectively isolated from bone marrow of Gata1+/0, hGATA1/Gata1+/0, Gata1low/0 and hGATA1/Gata1low/0 mice using gates described in Figure 6A, and cultured under condition of limiting dilutions stimulated with either erythroid- or megakaryocyte-permissive growth factors. The number and phenotype of their progeny were then analyzed after 7 days of culture (Figures 6B,C). MEP from wild type mice generated by day 7 cells with a fold increase of about 100. Approximately 34 and 17% of their progeny were erythroid cells (TER119pos/CD71pos) and megakaryocytes (CD41pos/CD61pos), respectively (Figures 6B,C). Although, MEP from hGATA1/Gata1+/0 mice generated a lower number of cells (fold increase ∼50), the great majority of their progeny was erythroid, with low numbers of megakaryocytes even when the cells were cultured under megakaryocyte-specific conditions, and very few mast cells (CD117pos/FcεRIpos) (Figures 6B,C). As expected, also MEP carrying the Gata1low/0 mutation expressed a fold increase of ∼50%. However, the progeny of these MEP contained not only great numbers of erythroid cells, but also mast cells and megakaryocytes (Figures 6B,C), confirming that this mutation alters the differentiation potential of these cells (Ghinassi et al., 2007). The fact that the MEP were cultured under conditions of limiting dilution indicate that this abnormality is cell autonomous. By contrast, MEP from hGATA1Gata1low/0 mice, generated by day 7 significantly more cells than those isolated from the other groups (fold increase ∼250). By contrast with the progeny of Gata1low MEP, that of double mutant MEP contained mostly erythroid cells, with very few mast cells, in cultures stimulated with erythroid specific conditions while the frequency of megakaryocytes in cultures stimulated with megakaryocyte-specific growth factors remained high (Figures 6B,C).
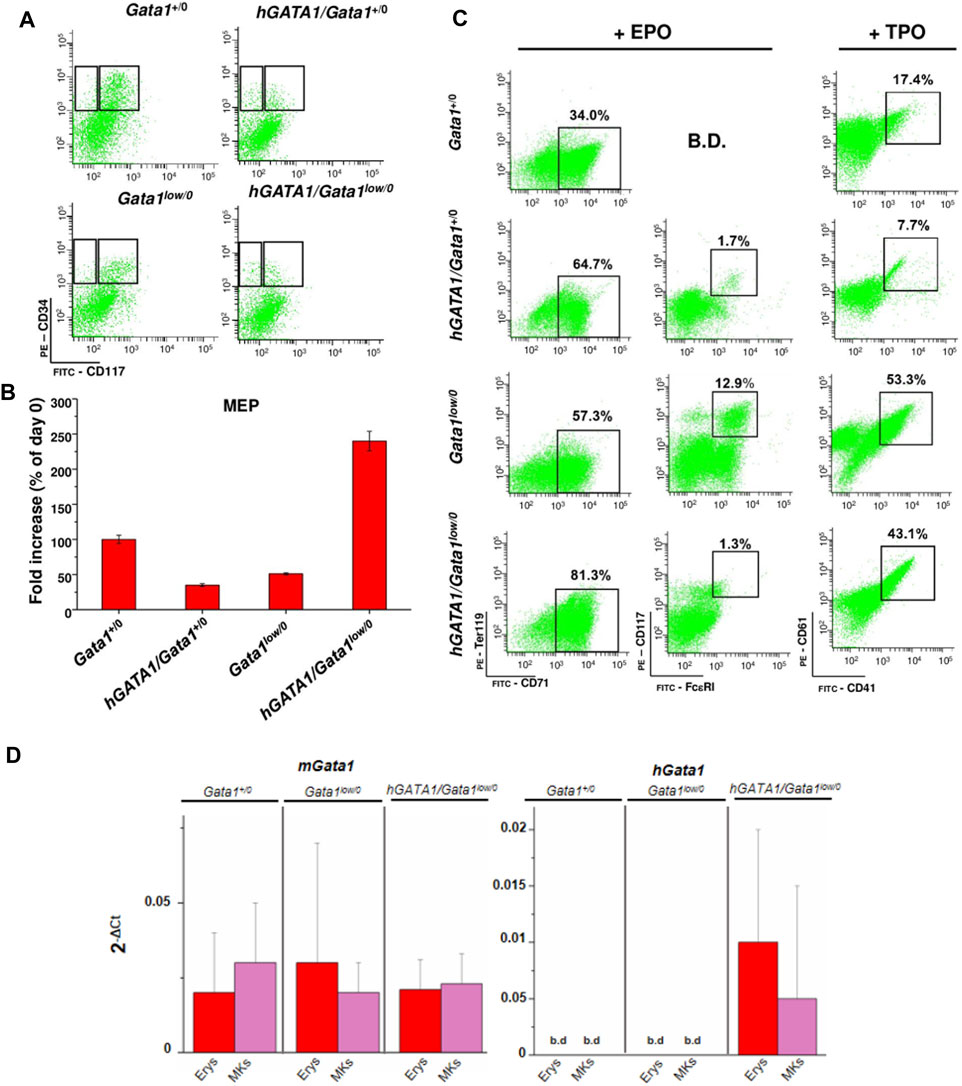
FIGURE 6. The presence of hGATA1 reduced number of mast cells generated by MEP carrying the Gata1low mutation in vitro, rescuing their abnormal maturation potential. (A) Representative gates used for the prospective purification of MEP (right gate) and CMP/GMP (left gate) progenitor cells from Gata1+/0, hGATA1/Gata1+/0, Gata1low/0 and hGATA1/Gata1low/0 mice, as indicated. (B) Fold increase by day 7 in cultures of MEP prospectively isolated from the different animal groups. Since the fold increases observed under erythroid and megakaryocytes culture conditions were the same, results obtained under the two conditions were pooled. (C) Analysis of the phenotype expressed by the progeny generated by MEP after 7 days of liquid culture under erythroid- or megakaryocyte-permissive conditions, as indicated. Erythroid, mast cells and megakaryocytes were identified according to the TER119pos/CD71pos, CD117pos/FcεRIpos and CD41pos/CD61pos phenotype, respectively, as described (Ghinassi et al., 2007). B.D.: below detection. (D) Quantitative RT-PCR expression analysis of hGATA1 and murine Gata1 (mGata1) mRNA in the erythroid (Erys) and megakaryocyte (MKs) progeny of MEP prospectively isolated from Gata1+/0, Gata1low/0 and hGATA1/Gata1low/0 mice. Results are presented as Mean (±SD) of at least three experiments, each one with one mouse per experimental group. Statistical analyses were performed by One-way analysis of variance Dunnett’s Multiple Comparison Test. Results of the statistical analyses: in B and D there are no statistically significant differences among groups.
Mechanistic insights for these results were provided by assessment of the expression of mGata1 and hGATA1 mRNA in erythroid precursors and megakaryocytes generated in vitro from MEP cells prospectively isolated from Gata1+/0, Gata1low/0 and hGATA1/Gata1low/0 mice (Figure 6D). Once again, hGATA1 was detected only in the progeny of the double mutant mice and the expression of this gene was greater in erythroid that in megakaryocyte precursors. The fact that, by contrast with the primary megakaryocytes, megakaryocytes expanded in vitro expressed detectable levels of hGATA1 may reflect functional differences between the two populations (Abbonate et al., 2016; Malara et al., 2014).
These results indicate that hGATA1 rescued the abnormal differentiation potential of Gata1low MEP making them unable to generate mast cells in culture.
The hGATA1 Transgene Rescues Erythroid Failure in Bone Marrow of Gata1low/0 Mice
In a previous publication, we had shown that Gata1low mice die within 15 days from removal of the spleen, an indication that in these mice erythropoiesis fails in the bone marrow and is sustained mainly by the spleen (Migliaccio et al., 2009). To assess whether the greater levels of GATA1 content in the erythroid cells from Gata1low/0 mice carrying the hGATA1 transgene rescue the erythropoietic functions of the marrow of these animals, we compared survival rates of untreated hGATA1/Gata1low/0 littermates with those subjected to splenectomy. To take into account that the functions of the bone marrow from Gata1low/0 mice are particularly reduced after 8-months of age when the mutant develop myelofibrosis (Vannucchi et al., 2002), experiments were repeated with 8- and 14-months old mice. Splenectomized Gata1low/0 mice were analyzed as positive controls.
As expected (Migliaccio et al., 2009), all Gata1low/0 mice die within 15–20 days from splenectomy (Figure 7A), confirming the fundamental role of this organ in sustaining their erythropoiesis. By contrast, splenectomy did not affect survival and Hct values of hGATA1/Gata1low/0 mice (Figures 7A,B). In addition, no significant differences were detected in the cellularity and progenitor/precursor cell frequency in the bone marrow from hGATA1/Gata1low/0 splenectomized mice in comparison to that of untreated littermates (Figure 7C).
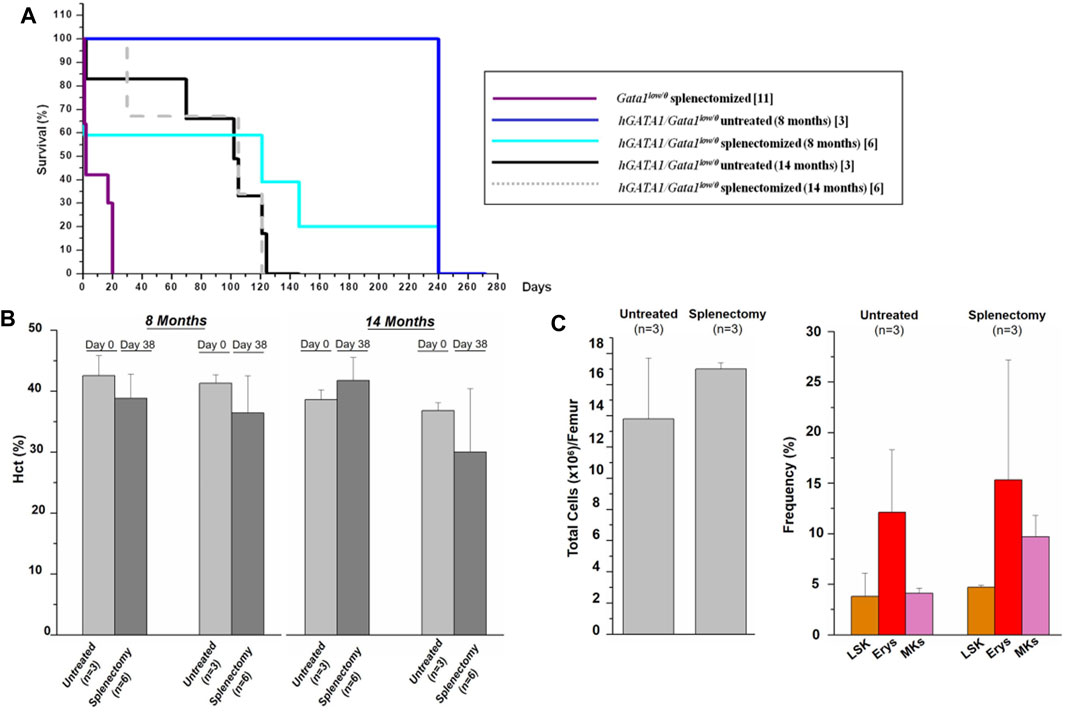
FIGURE 7. The presence of hGATA1 rescues erythropoiesis in the bone marrow of Gata1low/0 mice. Survival (A) and hematocrit (B) of untreated hGATA1/Gata1low/0 mice and of their littermates subjected to splenectomy. At the time of splenectomy, mice were either 8- or 14-month old, as indicated. In comparison to Gata1low/0 mice, hGATA1/Gata1low/0 survive well splenectomy, demonstrating that the bone marrow in these mice is a functional erythroid organ. Furthermore, at day 38, untreated and splenectomized mice have similar Hct values and these values are not different from those observed at day 0, demonstrating that the spleen has a marginal role in the erythropoiesis of Gata1low/0 mice carrying the hGATA1 transgene. (C) Total cell number (left panel) and frequency of LSK (Lin−/CD117pos/Sca1pos), and erythroid (TER119pos/CD71pos) and megakaryocyte (CD41pos/CD61pos) precursors (right panel) from untreated hGATA1/Gata1low/0 mice and from their littermates subjected to splenectomy. The mice were splenectomized at 8-months of age and analyzed 240 days after the surgery. (n) indicates the number of mice included in each experimental group. Statistical analyses was performed by One-way analysis of variance Dunnett’s Multiple Comparison Test. Results of the statistical analyses: in (B,C) there are no statistically significant differences among groups.
Collectively, these data provide functional support for the conclusion that hGATA1 rescues the erythroid failure of the bone marrow of Gata1low/0 mice.
Although hGATA1 Does not Rescue the Abnormal Maturation of Gata1low Megakaryocytes, it Reduces the Bone Marrow Fibrosis Developed by the Gata1low Animal Model
Several lines of evidence in patients (Vannucchi et al., 2005; Gilles et al., 2017) and in mouse models, including the Gata1low model (Vannucchi et al., 2002; Malara et al., 2018) suggest that myelofibrosis, a disease associated with hematopoietic failure in the bone marrow and development of hematopoiesis in extramedullary sites, is sustained by proinflammatory cytokines secreted at high levels by immature megakaryocytes, a cellular hallmark of this disorder. However, both in patients (Vainchenker et al., 2019) and in Gata1low mice (Ghinassi et al., 2007), the disease is also associated with increased proliferation of the stem/progenitor cells sustained by the driver mutations. The observation that the presence of hGATA1 rescued erythropoiesis in the bone marrow (Figure 5) and normalized the proliferation potential of the MEP (Figure 6) but did not restore the maturation of the megakaryocytes (Figures 2, 3) suggested to us to assess the relative importance of these two abnormalities for the development of the disease by analyzing whether hGATA1/Gata1low/0 mice develop fibrosis in the bone marrow.
In a first set of experiments we confirmed that hGATA1 does not rescues the megakaryocyte defects induced by the mutation by comparing the frequency of the clusters of megakaryocytes and the ultrastructural morphology of these cells in the spleen and bone marrow from Gata1+/0, Gata1low/0 and hGATA1/Gata1low/0 mice (Figure 8 and data not shown). For reasons linked to technical challenges in performing TEM studies on femurs, ultrastructural studies were performed on spleen only. Previous publications (Vyas et al., 1999; Centurione et al., 2004) had shown that the Gata1low mutation blocks megakaryocytic maturation between stage I and II, resulting in accumulation of megakaryocytes arranged in clusters and with a cytoplasm partitioned by a rudimental demarcation membrane system and containing mostly granules of light electron-density instead of the heavy electron-density granules that characterize the cytoplasm of normal megakaryocytes. Electron and light microscopy observations indicated that the hGATA1 transgene did not rescue the abnormal morphology of the megakaryocytes from the spleen and marrow of Gata1low mice. In fact, morphological analysis of spleen from Gata1+/0, Gata1low/0 and hGATA1/Gata1low/0 adult mice showed that, in contrast with wild type mice, in both groups megakaryocytes were organized in clusters of small cells with poorly developed platelet territories partitioned by a rudimental demarcation membrane system and light-electron dense granules (Figure 8A). Histological observations of the femur of these mice groups confirmed the presence of megakaryocytes clusters also in the medulla from both hGATA1/Gata1low/0 and Gata1low/0 mice (Figure 8B) while these clusters are absent from the medulla of femurs from wild type animals [(Vannucchi et al., 2002) and data not shown]. The conclusion that the megakaryocytes from hGATA1/Gata1low/0 and Gata1low/0 mice are similarly immature is supported also by the observation that the trabecular and the compact bone in both animal groups is thicker than normal (Figure 8B). Increased osteogenesis, in fact, is one of the traits expressed by Gata1low mice that has been more strongly mechanistically linked with the megakaryocyte abnormalities induced by the mutation (Kacena et al., 2004).
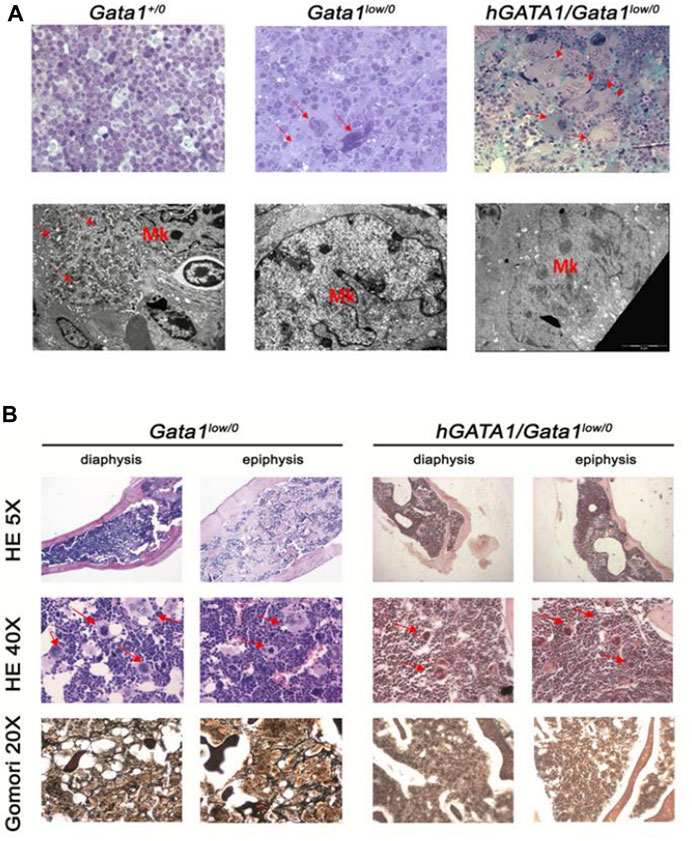
FIGURE 8. The presence of hGATA1 rescues fibrosis in the bone marrow of Gata1low/0 mice without decreasing the great number of abnormally immature megakaryocytes present in their organs. (A) Top panels: semithin sections of spleens from representative Gata1+/0, Gata1low/0 and hGATA1/Gata1low/0 mice, as indicated (magnification 40X). By contrast, with Gata1+/0 mice, megakaryocytes (MK) both from the spleen [arrows in (A)] of both Gata1low/0 and hGATA1/Gata1low/0 mice are organized in clusters of small cells. Lower panels: transmission electron microscopy analyses of representative megakaryocytes from the spleens of Gata1+/0, Gata1low/0 and hGATA1/Gata1low/0 mice (magnification 4,400X). By contrast with the well organized platelet territories and heavily electron dense granules present in the cytoplasm of wild-type megakaryocytes, the cytoplasm of megakaryocytes from both Gata1low/0 and hGATA1/Gata1low/0 mice is poorly partitioned into platelet territories and contains granules with light-electron density, an indication of poor protein content. (B) Histological sections of the diaphysis and epiphysis region of femurs from Gata1low/0 and hGATA1/Gata1low/0 mice, as indicated. The upper and middle panels present hematoxylin-eosin (HE) staining of representative transversal sections from Gata1low/0 (left) and hGATA1/Gata1low/0 (right) mice at 5X (top panels) and 40X (middle panels) magnifications to highlight details of the bone and of the medulla, respectively. The 5X magnification shows that the femur from both Gata1low/0 and hGATA1/Gata1low/0 mice contains numerous bone trabeculae in its diaphysis and thicker compact bone sections in its epiphysis, an indication of active osteogenesis while the space inside the bone available for the medulla is limited. The 40X magnification shows the presence in the medulla from both experimental groups of numerous clusters of megakaryocytes (red arrows) similar to those which were observed in the spleen [panel (A)]. The Gomori staining in the bottom panels (magnification 20X) indicates presence of fibrosis in the medulla of Gata1low/0 mice but not in that from hGATA1/Gata1low/0. littermates. Results are representative of those observed in 3 mice per experimental groups.
Collectively, in agreement with the observation that hGATA1 under the control of μLCR coupled with the β-globin gene promoter is not expressed in megakaryocytes, the presence of this transgene did not rescue the megakaryocyte abnormalities induced by the Gata1low mutation.
To a surprise, however, in spite megakaryocytes of hGATA1/Gata1low/0 remained immature, the double mutants did not develop fibrosis in bone marrow, providing further indication that the transgene rescues the myelofibrotic trait induced by the Gata1low mutation (Figure 8B).
Discussion
The hypomorphic Gata1low mutation impairs expression of the Gata1 gene already at the levels of hematopoietic progenitor cells and continue to exert its effects in erythroid cells and megakaryocytes (Ling et al., 2018). The consequent reduced content of GATA1 protein results in the following cell specific abnormalities: it increases proliferation of MEP altering their differentiation potential making the cells able to generate mast cells under erythroid-specific conditions (Ghinassi et al., 2007), it increases the apoptotic rates of the erythroblasts (McDevitt et al., 1997; Vannucchi et al., 2001) and blocks megakaryocyte maturation retaining these cells in a proliferative state (Vyas et al., 1999; Vannucchi et al., 2002). As a consequence, the mice are born anemic and thrombocytopenic (McDevitt et al., 1997; Vyas et al., 1999). With age, they recover from their anemia by recruiting the spleen as extramedullary site (Migliaccio et al., 2009; Spangrude et al., 2016) but retain the abnormalities at the level of MEP (Ghinassi et al., 2007) and megakaryocytes (Vyas et al., 1999; Vannucchi et al., 2002) for all their life developing myelofibrosis with age (Vannucchi et al., 2002). In fact, overall, the phenotype of Gata1low mice is similar to that of patients with primary myelofibrosis, the most severe of the Philadelphia-negative myeloproliferative neoplasms which is associated with abnormalities in MEP proliferation and megakaryocyte maturation similar to those observed in these mutants (Zahr et al., 2016).
In this manuscript, we used a standard trans-complementation gene approach with the hGATA1 transgene to address two questions: 1) is expression of hGATA1 erythroid restricted? 2) Since hGATA1 is not expected to be expressed by megakaryocytes, do the double mutants develop myelofibrosis, a disease driven by abnormalities sustained by reduced Gata1 expression in these cells?
To address the first question, we investigated whether the hGATA1 gene under the control of a μLCR coupled with the promoter of the β-globin gene is expressed in hematopoietic cells from adult mice carrying the Gata1low mutation, identified the cells in which the transgene is expressed and analyzed whether the levels of expression sustained by these regulatory sequences are sufficient to rescue the phenotypic abnormalities induced by the mutation. Our results confirmed that embryos carrying the Gata1low/0 mutation are anemic and thrombocytopenic while adult mutants express significantly lower levels of Hct and platelet counts compared to the wild type littermates. We confirmed that in Gata1low/0 mice, the levels of Gata1 transcripts are reduced already in progenitor cells (CMP/GMP and MEP) and not only in erythroid and megakaryocyte precursors. We also confirmed that reduced levels of Gata1 mRNA resulted in lower GATA1 protein content in erythroid cells, which displayed greater apoptotic levels, and in megakaryocytes, that remained morphologically immature. The hGATA1 under the control of μLCR/β-globin regulatory sequences was already expressed at the level of progenitor cells (both CMP/GMP and MEP) and of erythroid cells prospectively isolated from hGATA1/Gata1low/0 littermates. These data are consistent with the observation that the μLCR/β-globin regulatory sequences are already active at the levels of the stem/progenitor cells, which express low but clearly detectable levels of β-globin mRNA, and in erythroid cells but not in cells of other hematopoietic lineages such as megakaryocytes (Beru et al., 1990; Ashihara et al., 1997; McKinney-Freeman et al., 2012). The pattern of cell specificity of the expression of hGATA1 was correlated with the pattern of the traits induced by the Gata1low mutation that were rescued by the presence of the transgene. On one hand, the differentiation potential of MEP prospectively isolated from the double mutants became restricted to cells of erythroid and megakaryocytic lineage having lost their ability to generate mast cells. In addition, erythroid cells expressed nearly normal levels of the erythroid specific genes analyzed and displayed low apoptotic rates. On the other hand, megakaryocytes remain detected in great numbers, an indication of increased proliferation, express altered levels of the megakaryocyte specific genes analyzed and displayed an immature morphology. Consistently with the rescue of the MEP alterations, erythropoiesis was effective in the bone marrow of the double mutant mice making their extramedullary hematopoiesis in spleen dispensable for survival. Consistently with the rescue of the defective erythroid maturation, the animals were not anemic and, as predicted by the failure of the transgene to rescue the megakaryocyte defects, the mice remained thrombocytopenic and with increased osteogenesis, a trait driven by the abnormal megakaryocytes (Kacena et al., 2004).
The observation that the expression of hGATA1 driven by the μLCR/β-globin regulatory sequences is restricted to the erythroid cells has important implications for gene therapy. In fact, suitable gene therapy approaches require robust evidence not only for the safety of the retroviral vector used, but also for the specificity of its expression, essential to minimize its off-target effects (Demirci et al., 2018; High and Roncarolo, 2019). Based on the strong evidence for erythroid specificity displayed by the μLCR/β-globin regulatory sequences in transgenic mouse models (Li and Stamatoyannopoulos, 1994; Papayannopoulou et al., 2000; Li et al., 2002), these sequences are used to drive the expression of the human β-globin in most of the retroviral vectors currently under investigation of gene therapy of hemoglobinopathies (Wang et al., 2019; Morgan et al., 2020). Our results confirm that these regulatory sequences are indeed erythroid specific (and therefore likely to be safe) because, with the exception of some expression of the transgene at the level of progenitor cells, in which even under normal circumstances these regulatory sequences are active (McKinney-Freeman et al., 2012), they drove expression of the transgene mainly in erythroid cells and were ineffective in the closely related megakaryocytes.
Erythroid commitment and differentiation is sequentially driven by two genes of the GATA transcription factors family, GATA1 and GATA2. GATA2 is expressed at high levels in multipotential progenitors, affecting their expansion and guiding the early stage of erythroid commitment (Tsai et al., 1994). As erythroid commitment progresses, GATA2 activates the expression of GATA1 which is abundantly expressed in late progenitor cells and erythroblasts (Leonard et al., 1993). The switch from GATA2 to GATA1 expression in the control of erythropoiesis is known as the GATA switch and represents the clock which initiates the translation of the erythroid specific genes (Katsumura and Bresnick, 2017; Bresnick et al., 2020). Once activated by GATA2, the expression of Gata1 is self-sustained. In mice, expression of Gata1 is regulated by three DNase hypersensitive sites (HS), two of which (HSI and HSII) lay within 8 Kb upstream of the coding sequences and the third one (HSIII) within the first intron (Kobayashi and Yamamoto, 2007). The Gata1low mutation specifically deletes HSI. The fact that erythroid cells from Gata1low mice express some level of the endogenous gene reflects the positive effects on its translation exerted by GATA2 likely by binding to HSII and/or HSIII. The observation that in the double mutant cells increases in the content of the GATA1 protein due to the contribution of hGATA1 does not increase the expression of the endogenous gene confirms that HSI is an indispensable element of the self-sustained regulatory loop of Gata1 transcription the expression of which remains at the levels driven by GATA2.
To address the second question, we investigated whether hGATA1/Gata1low/0 mice develop myelofibrosis with age. To a surprise, in spite the megakaryocytes of the double mutants remained GATA1 hypomorphic, these mice did not develop hematopoietic failure and fibrosis in their bone marrow, i.e., the two distinctive traits for myelofibrosis. This observation is counterintuitive given the strong evidence that both in patients and in causative mutation-driven animal models, myelofibrosis is sustained by abnormal GATA1 hypomorphic megakaryocytes (Vannucchi et al., 2005; Gilles et al., 2017). In addition, megakaryocyte-restricted expression of the driver mutations is necessary and sufficient to induce myelofibrosis even if the hematopoietic stem cells are normal (Jeremy Wen et al., 2015; Zhan et al., 2016). This apparent contradiction has been recently clarified by new exciting single cell profiling data indicating that the megakaryocytes in the bone marrow are a mixture of three populations, each one exerting a different function (Psaila et al., 2020; Yeung et al., 2020; Migliaccio and Hoffman, 2021; Sun et al., 2021; Wang et al., 2021). In fact, in addition to megakaryocytes poised to form platelets, the hematopoietic stem cells also generate megakaryocytes poised to exert immune-functions in the lungs (Pariser et al., 2021) or niche-functions locally, by secreting collagen and other extracellular matrix proteins (Malara et al., 2014). This single cell profiling also indicated that while maturation of platelet-poised and immune-poised megakaryocytes requires upregulation of GATA1 expression, niche-poised megakaryocytes are dependent on low levels of GATA1 (Wang et al., 2021). Data from the Balduini laboratory indicate that megakaryocytes expressing collagens, which are supposedly the niche-poised cells, are very rare in the bone marrow from healthy individuals at opposite with myelofibrosis patients where a great proportion of megakaryocytes in the bone marrow express collagen (Abbonante et al., 2016), suggesting that niche poised-megakaryocytes may contribute to deposition of fibrosis in the bone marrow. Overall, these recent results suggest the hypothesis that hypomorphic GATA1 content at the levels of megakaryocytes precursors switches their fate from platelet-poised to niche-poised cells, potentially contributing to fibrosis. Our data are consistent with this refinement of the pathobiological role of megakaryocyte abnormalities in myelofibrosis. In fact, the rescue of the myelofibrotic phenotype of the Gata1low mice is associated with normalization of the abnormal differentiation potential of the stem/progenitor cells (which express hGATA1 and became able to differentiate in the bone marrow) and although expression of hGATA1 is below detection in megakaryocytes prospectively isolated from the bone marrow, it is expressed by megakaryocytes differentiated in culture from the double mutants, the large majority of which has been suggested to represent either niche- or immune-poised MKs (Malara et al., 2014). It is then possible that expression of hGATA1 at the level of the multi-potent megakaryocyte precursors prevented their switch to niche-poised cells, thereby rescuing the myelofibrosis trait. Unfortunately, different populations of megakaryocyte precursors poised to generate megakaryocytes and immune-poised or niche-poised megakaryocytes have all the same morphology (Wang et al., 2021; Migliaccio and Hoffman, 2021), thus they cannot be discriminated by morphological observations. This novel pathobiological model may only be tested when cell surface markers to prospectively identify the various cell populations will became available.
Last but not least, our data have implications to design strategies for the cure of primary myelofibrosis, the most severe of the Philadelphia-negative myeloproliferative neoplasms which has presently no cure (Zahr et al., 2016). In fact, drugs targeting the driver mutations displayed by the malignant hematopoietic stem clones, such as the JAK1/2 inhibitor ruxolitinib, are greatly effective in ameliorating the clinical manifestation of the disease but it is still unclear whether they are also effective in halting the progression toward its final stage (Mascarenhas and Hoffman, 2013; Tefferi, 2021). As of today, with exception of bone marrow transplantation which may be offered to a limited number of patients (Tamari et al., 2015), this disease is still an unmet clinical need. Based on the observation that bone marrow transplantation cures the disease by rescuing both the hematopoietic stem cell and the microenvironmental abnormalities displayed by the patient, the consensus has been reached that treatment of the disease requires the use of drug combinations targeting both abnormalities (Eran et al., 2019; Tefferi, 2021). Our data support this consensus and indicate that these combination therapies should not combine drugs targeting the driver mutations (such as ruxolitininb) with those that target the platelet-poised megakaryocytes but rather with those that target the niche-poised cells.
Data Availability Statement
The original contributions presented in the study are included in the article/Supplementary Materials, further inquiries can be directed to the corresponding author.
Ethics Statement
The animal study was reviewed and approved by the protocol n. 419/2015-PR approved by the Italian Ministery of Health and according to the directive 2010/63/EU of the European Parliament and of the Council of September 22, 2010 on the protection of animals used for scientific purposes.
Author Contributions
FM, PV, MZ, MM, and BC performed experiments and analyzed the data. FM, AMV, AL, and GS revised the data and wrote the manuscript. ARM designed the study, interpreted the data and wrote the manuscript. All the authors read the manuscript and concur with its content.
Funding
This study was supported by grants from the National Cancer (No. P01-CA108671), Heart, Lung and Blood (No. 1R01-HL134684) Institute and Associazione Italiana Ricerca Cancro (No. AIRC IG 23252).
Conflict of Interest
The authors declare that the research was conducted in the absence of any commercial or financial relationships that could be construed as a potential conflict of interest.
Publisher’s Note
All claims expressed in this article are solely those of the authors and do not necessarily represent those of their affiliated organizations, or those of the publisher, the editors and the reviewers. Any product that may be evaluated in this article, or claim that may be made by its manufacturer, is not guaranteed or endorsed by the publisher.
Acknowledgments
The authors gratefully acknowledge George Stamatoyannopoulos and Thalia Papayannopoulou for providing the hGATA1 mice and for encouragement, inspiration and critical review of the data and Elena Alfani for quantitative RT-PCR determinations. The paper is dedicated to the memory of Drs. Stamatoyannopoulos and Alfani who are deeply missed.
Supplementary Material
The Supplementary Material for this article can be found online at: https://www.frontiersin.org/articles/10.3389/fgene.2021.720552/full#supplementary-material
References
Abbonante, V., Di Buduo, C. A., Gruppi, C., Malara, A., Gianelli, U., Celesti, G., et al. (2016). Thrombopoietin/TGF-β1 Loop Regulates Megakaryocyte Extracellular Matrix Component Synthesis. Stem Cells 34 (4), 1123–1133. doi:10.1002/stem.2285
Ahmed, M., Sternberg, A., Hall, G., Thomas, A., Smith, O., O’Marcaigh, A., et al. (2004). Natural History of GATA1 Mutations in Down Syndrome. Blood 103 (7), 2480–2489. doi:10.1182/blood-2003-10-3383
Akashi, K., Traver, D., Miyamoto, T., and Weissman, I. L. (2000). A Clonogenic Common Myeloid Progenitor that Gives Rise to All Myeloid Lineages. Nature 404 (6774), 193–197. doi:10.1038/35004599
Ashihara, E., Vannucchi, A. M., Migliaccio, G., and Migliaccio, A. R. (1997). Growth Factor Receptor Expression during In Vitro Differentiation of Partially Purified Populations Containing Murine Stem Cells. J. Cel. Physiol. 171 (3), 343–356. doi:10.1002/(sici)1097-4652(199706)171:3<343:aid-jcp13>3.0.co;2-b
Beru, N., Maples, P. B., Hermine, O., and Goldwasser, E. (1990). Differential Expression of Alpha- and Beta-Globin Genes in Erythroleukemic Cell Lines. Mol. Cel Biol 10 (7), 3591–3595. doi:10.1128/mcb.10.7.3591-3595.1990
Bresnick, E. H., Jung, M. M., and Katsumura, K. R. (2020). Human GATA2 Mutations and Hematologic Disease: How many Paths to Pathogenesis? Blood Adv. 4 (18), 4584–4592. doi:10.1182/bloodadvances.2020002953
Bresnick, E. H., Lee, H.-Y., Fujiwara, T., Johnson, K. D., and Keles, S. (2010). GATA Switches as Developmental Drivers. J. Biol. Chem. 285 (41), 31087–31093. doi:10.1074/jbc.r110.159079
Campbell, A. E., Wilkinson-White, L., Mackay, J. P., Matthews, J. M., and Blobel, G. A. (2013). Analysis of Disease-Causing GATA1 Mutations in Murine Gene Complementation Systems. Blood 121 (26), 5218–5227. doi:10.1182/blood-2013-03-488080
Centurione, L., Di Baldassarre, A., Zingariello, M., Bosco, D., Gatta, V., Rana, R. A., et al. (2004). Increased and Pathologic Emperipolesis of Neutrophils within Megakaryocytes Associated with Marrow Fibrosis in GATA-1low Mice. Blood 104 (12), 3573–3580. doi:10.1182/blood-2004-01-0193
Crispino, J. D., and Weiss, M. J. (2004). Erythro-megakaryocytic Transcription Factors Associated with Hereditary Anemia. Blood 123 (20), 3080–3088. doi:10.1182/blood-2014-01-453167
Crispino, J. D., and Horwitz, M. S. (2017). GATA Factor Mutations in Hematologic Disease. Blood 129 (15), 2103–2110. doi:10.1182/blood-2016-09-687889
Demirci, S., Uchida, N., and Tisdale, J. F. (2018). Gene Therapy for Sickle Cell Disease: An Update. Cytotherapy 20, 899–910. doi:10.1016/j.jcyt.2018.04.003
Di Pierro, E., Russo, R., Karakas, Z., Brancaleoni, V., Gambale, A., Kurt, I., et al. (2015). Congenital Erythropoietic Porphyria Linked to GATA1-R216w Mutation: Challenges for Diagnosis. Eur. J. Haematol. 94 (6), 491–497. doi:10.1111/ejh.12452
Eran, Z., Zingariello, M., Bochicchio, M. T., Bardelli, C., and Migliaccio, A. R. (2019). Novel Strategies for the Treatment of Myelofibrosis Driven by Recent Advances in Understanding the Role of the Microenvironment in its Etiology. F1000Res 8, 1662. doi:10.12688/f1000research.18581.1
Federici, G., Varricchio, L., Martelli, F., Falchi, M., Picconi, O., Francescangeli, F., et al. (2019). Phosphoproteomic Landscaping Identifies Non-canonical cKIT Signaling in Polycythemia Vera Erythroid Progenitors. Front. Oncol. 9, 1245. doi:10.3389/fonc.2019.01245
Ferreira, R., Ohneda, K., Yamamoto, M., and Philipsen, S. (2005). GATA1 Function, a Paradigm for Transcription Factors in Hematopoiesis. Mol. Cel Biol 25 (4), 1215–1227. doi:10.1128/mcb.25.4.1215-1227.2005
Ferreira, R., Wai, A., Shimizu, R., Gillemans, N., Rottier, R., von Lindern, M., et al. (2007). Dynamic Regulation of Gata Factor Levels Is More Important Than Their Identity. Blood 109 (12), 5481–5490. doi:10.1182/blood-2006-11-060491
Freson, K., Devriendt, K., Matthijs, G., Van Hoof, A., De Vos, R., Thys, C., et al. (2001). Platelet Characteristics in Patients with X-Linked Macrothrombocytopenia Because of a Novel GATA1mutation. Blood 98 (1), 85–92. doi:10.1182/blood.v98.1.85
Fujiwara, Y., Browne, C. P., Cunniff, K., Goff, S. C., and Orkin, S. H. (2002). Arrested Development of Embryonic Red Cell Precursors in Mouse Embryos Lacking Transcription Factor GATA-1. Proc. Natl. Acad. Sci. U S A. 93 (22), 12355–12358. doi:10.1073/pnas.93.22.12355
Ghinassi, B., Sanchez, M., Martelli, F., Amabile, G., Vannucchi, A. M., Migliaccio, G., et al. (2007). The Hypomorphic Gata1low Mutation Alters the Proliferation/differentiation Potential of the Common Megakaryocytic-Erythroid Progenitor. Blood 109 (4), 1460–1471. doi:10.1182/blood-2006-07-030726
Gilles, L., Arslan, A. D., Marinaccio, C., Wen, Q. J., Arya, P., McNulty, M., et al. (2017). Downregulation of GATA1 Drives Impaired Hematopoiesis in Primary Myelofibrosis. J. Clin. Invest. 127 (4), 1316–1320. doi:10.1172/jci82905
Harigae, H., Xu, G., Sugawara, T., Ishikawa, I., Toki, T., and Ito, E. (2004). The GATA1 Mutation in an Adult Patient with Acute Megakaryoblastic Leukemia Not Accompanying Down Syndrome. Blood 103 (8), 3242–3243. doi:10.1182/blood-2004-01-0016
High, K. A., and Roncarolo, M. G. (2019). Gene Therapy. N. Engl. J. Med. 381 (5), 455–464. doi:10.1056/nejmra1706910
Iolascon, A., Andolfo, I., and Russo, R. (2020). Congenital Dyserythropoietic Anemias. Blood 136 (11), 1274–1283. doi:10.1182/blood.2019000948
Jeremy Wen, Q., Yang, Q., Goldenson, B., Malinge, S., Lasho, T., Schneider, R. K., et al. (2015). Targeting Megakaryocytic-Induced Fibrosis in Myeloproliferative Neoplasms by AURKA Inhibition. Nat. Med. 21 (12), 1473–1480. doi:10.1038/nm.3995
Kacena, M. A., Shivdasani, R. A., Wilson, K., Xi, Y., Troiano, N., Nazarian, A., et al. (2004). Megakaryocyte-osteoblast Interaction Revealed in Mice Deficient in Transcription Factors GATA-1 and NF-E2. J. Bone Miner Res. 19 (4), 652–660. doi:10.1359/JBMR.0301254
Katsumura, K. R., and Bresnick, E. H. (2017). The GATA Factor Revolution in Hematology. Blood 129 (15), 2092–2102. doi:10.1182/blood-2016-09-687871
Kobayashi, M., and Yamamoto, M. (2007). Regulation of GATA1 Gene Expression. J. Biochem. 142 (1), 1–10. doi:10.1093/jb/mvm122
Leonard, M., Brice, M., Engel, J., and Papayannopoulou, T. (1993). Dynamics of GATA Transcription Factor Expression during Erythroid Differentiation. Blood 82 (4), 1071–1079. doi:10.1182/blood.v82.4.1071.1071
Li, Q., Clegg, C., Peterson, K., Shaw, S., Raich, N., and Stamatoyannopoulos, G. (2002). Binary Transgenic Mouse Model for Studying the Trans Control of Globin Gene Switching: Evidence that GATA-1 Is an In Vivo Repressor of Human Epsilon Gene Expression. Proc. Natl. Acad. Sci. U S A. 94 (6), 2444–2448. doi:10.1073/pnas.94.6.2444
Li, Q., and Stamatoyannopoulos, J. A. (1994). Position independence and Proper Developmental Control of Gamma-Globin Gene Expression Require Both a 5' Locus Control Region and a Downstream Sequence Element. Mol. Cel Biol 14 (9), 6087–6096. doi:10.1128/mcb.14.9.6087-6096.1994
Ling, T., Crispino, J. D., Zingariello, M., Martelli, F., and Migliaccio, A. R. (2018). GATA1 Insufficiencies in Primary Myelofibrosis and Other Hematopoietic Disorders: Consequences for Therapy. Expert Rev. Hematol. 11 (3), 169–184. doi:10.1080/17474086.2018.1436965
Ludwig, L. S., Gazda, H. T., Eng, J. C., Eichhorn, S. W., Thiru, P., Ghazvinian, R., et al. (2014). Altered Translation of GATA1 in Diamond-Blackfan Anemia. Nat. Med. 20 (7), 748–753. doi:10.1038/nm.3557
Malara, A., Abbonante, V., Zingariello, M., Migliaccio, A., and Balduini, A. (2018). Megakaryocyte Contribution to Bone Marrow Fibrosis: Many Arrows in the Quiver. Mediterr. J. Hematol. Infect. Dis. 10 (1), e2018068–18. doi:10.4084/MJHID.2018.068
Malara, A., Currao, M., Gruppi, C., Celesti, G., Viarengo, G., Buracchi, C., et al. (2014). Megakaryocytes Contribute to the Bone Marrow-Matrix Environment by Expressing Fibronectin, Type IV Collagen, and Laminin. Stem Cells 32 (4), 926–937. doi:10.1002/stem.1626
Martelli, F., Ghinassi, B., Panetta, B., Alfani, E., Gatta, V., Pancrazzi, A., et al. (2005). Variegation of the Phenotype Induced by the Gata1low Mutation in Mice of Different Genetic Backgrounds. Blood 106 (13), 4102–4113. doi:10.1182/blood-2005-03-1060
Martin, D. I. K., Zon, L. I., Mutter, G., and Orkin, S. H. (1990). Expression of an Erythroid Transcription Factor in Megakaryocytic and Mast Cell Lineages. Nature 344 (6265), 444–447. doi:10.1038/344444a0
Mascarenhas, J., and Hoffman, R. (2013). A Comprehensive Review and Analysis of the Effect of Ruxolitinib Therapy on the Survival of Patients with Myelofibrosis. Blood 121 (24), 4832–4837. doi:10.1182/blood-2013-02-482232
McDevitt, M. A., Shivdasani, R. A., Fujiwara, Y., Yang, H., and Orkin, S. H. (1997). A "knockdown" Mutation Created by Cis-Element Gene Targeting Reveals the Dependence of Erythroid Cell Maturation on the Level of Transcription Factor GATA-1. Proc. Natl. Acad. Sci. 94 (13), 6781–6785. doi:10.1073/pnas.94.13.6781
McKinney-Freeman, S., Cahan, P., Li, H., Lacadie, S. A., Huang, H.-T., Curran, M., et al. (2012). The Transcriptional Landscape of Hematopoietic Stem Cell Ontogeny. Cell Stem Cell 11 (5), 701–714. doi:10.1016/j.stem.2012.07.018
Mehaffey, M. G., Newton, A. L., Gandhi, M. J., Crossley, M., and Drachman, J. G. (2001). X-linked Thrombocytopenia Caused by a Novel Mutation ofGATA-1. Blood 98 (9), 2681–2688. doi:10.1182/blood.v98.9.2681
Migliaccio, A. R., and Hoffman, R. (2021). An Outline of the Outset of Thrombopoiesis in Human Embryos at Last. Cell Stem Cell 28 (3), 363–365. doi:10.1016/j.stem.2021.02.007
Migliaccio, A. R., Martelli, F., Verrucci, M., Sanchez, M., Valeri, M., Migliaccio, G., et al. (2009). Gata1 Expression Driven by the Alternative HS2 Enhancer in the Spleen Rescues the Hematopoietic Failure Induced by the Hypomorphic Gata1low Mutation. Blood 114 (10), 2107–2120. doi:10.1182/blood-2009-03-211680
Migliaccio, A. R., Rana, R. A., Sanchez, M., Lorenzini, R., Centurione, L., Bianchi, L., et al. (2003). GATA-1 as a Regulator of Mast Cell Differentiation Revealed by the Phenotype of the GATA-1low Mouse Mutant. J. Exp. Med. 197 (3), 281–296. doi:10.1084/jem.20021149
Morgan, R. A., Unti, M. J., Aleshe, B., Brown, D., Osborne, K. S., Koziol, C., et al. (2020). Improved Titer and Gene Transfer by Lentiviral Vectors Using Novel, Small β-Globin Locus Control Region Elements. Mol. Ther. 28 (1), 328–340. doi:10.1016/j.ymthe.2019.09.020
Nichols, K. E., Crispino, J. D., Poncz, M., White, J. G., Orkin, S. H., Maris, J. M., et al. (2000). Familial Dyserythropoietic Anaemia and Thrombocytopenia Due to an Inherited Mutation in GATA1. Nat. Genet. 24 (march), 266–270. doi:10.1038/73480
Orkin, S. H., and Zon, L. I. (2008). SnapShot: Hematopoiesis. Cell 132 (4), 712. doi:10.1016/j.cell.2008.02.013
Papayannopoulou, T., Priestley, G. V. V., Rohde, A., Peterson, K. R. R., and Nakamoto, B. (2000). Hemopoietic Lineage Commitment Decisions: In Vivo Evidence from a Transgenic Mouse Model Harboring Micro LCR-Betapro-LacZ as a Transgene. Blood 95 (4), 1274–1282.
Pariser, D. N., Hilt, Z. T., Ture, S. K., Blick-Nitko, S. K., Looney, M. R., Cleary, S. J., et al. (2021). Lung Megakaryocytes Are Immune Modulatory Cells. J. Clin. Invest. 131 (1), e1373776. doi:10.1172/jci137377
Peterson, K. R., Clegg, C. H., Huxley, C., Josephson, B. M., Haugen, H. S., Furukawa, T., et al. (1993). Transgenic Mice Containing a 248-kb Yeast Artificial Chromosome Carrying the Human Beta-Globin Locus Display Proper Developmental Control of Human Globin Genes. Proc. Natl. Acad. Sci. 90 (16), 7593–7597. doi:10.1073/pnas.90.16.7593
Psaila, B., Wang, G., Rodriguez-Meira, A., Li, R., Heuston, E. F., Murphy, L., et al. (2020). Single-Cell Analyses Reveal Megakaryocyte-Biased Hematopoiesis in Myelofibrosis and Identify Mutant Clone-specific Targets. Mol. Cel 78 (3), 477–492.e8. doi:10.1016/j.molcel.2020.04.008
Romeo, P.-H., Prandini, M.-H., Joulin, V., Mignotte, V., Prenant, M., Vainchenker, W., et al. (1990). Megakaryocytic and Erythrocytic Lineages Share Specific Transcription Factors. Nature 344 (6265), 447–449. doi:10.1038/344447a0
Russo, R., Andolfo, I., Gambale, A., De Rosa, G., Manna, F., Arillo, A., et al. (2017). GATA1 Erythroid-specific Regulation of SEC23B Expression and its Implication in the Pathogenesis of Congenital Dyserythropoietic Anemia Type II. Haematologica 102 (9), e371–e374. doi:10.3324/haematol.2016.162966
Shimizu, R., and Yamamoto, M. (2016). GATA-related Hematologic Disorders. Exp. Hematol. 44 (8), 696–705. doi:10.1016/j.exphem.2016.05.010
Shivdasani, R. A., Fujiwara, Y., McDevitt, M. A., and Orkin, S. H. (1997). A Lineage-Selective Knockout Establishes the Critical Role of Transcription Factor GATA-1 in Megakaryocyte Growth and Platelet Development. EMBO J. 16 (13), 3965–3973. doi:10.1093/emboj/16.13.3965
Spangrude, G. J., Lewandowski, D., Martelli, F., Marra, M., Zingariello, M., Sancillo, L., et al. (2016). P-selectin Sustains Extramedullary Hematopoiesis in theGata1lowModel of Myelofibrosis. Stem Cells 34 (1), 67–82. doi:10.1002/stem.2229
Sun, S., Jin, C., Si, J., Lei, Y., Chen, K., Cui, Y., et al. (2021). Single-Cell Analysis of Ploidy and Transcriptome Reveals Functional and Spatial Divergency in Murine Megakaryopoiesis. Blood, 2021010697. [Ahead of print]. doi:10.1182/blood.2021010697
Tamari, R., Mughal, T. I., Rondelli, D., Hasserjian, R., Gupta, V., Odenike, O., et al. (2015). Allo-SCT for Myelofibrosis: Reversing the Chronic Phase in the JAK Inhibitor Era? Bone Marrow Transpl. 50 (5), 628–636. doi:10.1038/bmt.2014.323
Tefferi, A. (2021). Primary Myelofibrosis: 2021 Update on Diagnosis, Risk‐stratification and Management. Am. J. Hematol. 96 (1), 145–162. doi:10.1002/ajh.26050
Trainor, C. D., Evans, T., Felsenfeld, G., and Boguski, M. S. (1990). Structure and Evolution of a Human Erythroid Transcription Factor. Nature 343 (January), 92–96. doi:10.1038/343092a0
Tsai, F.-Y., Keller, G., Kuo, F. C., Weiss, M., Chen, J., Rosenblatt, M., et al. (1994). An Early Haematopoietic Defect in Mice Lacking the Transcription Factor GATA-2. Nature 371 (6494), 221–226. doi:10.1038/371221a0
Tsai, S.-F., Martin, D. I. K., Zon, L. I., D'Andrea, A. D., Wong, G. G., and Orkin, S. H. (1989). Cloning of cDNA for the Major DNA-Binding Protein of the Erythroid Lineage through Expression in Mammalian Cells. Nature 339 (6224), 446–451. doi:10.1038/339446a0
Vainchenker, W., Plo, I., Marty, C., Varghese, L. N., and Constantinescu, S. N. (2019). The Role of the Thrombopoietin Receptor MPL in Myeloproliferative Neoplasms: Recent Findings and Potential Therapeutic Applications. Expert Rev. Hematol. 12 (6), 437–448. doi:10.1080/17474086.2019.1617129
Vannucchi, A. M., Bianchi, L., Cellai, C., Paoletti, F., Carrai, V., Calzolari, A., et al. (2001). Accentuated Response to Phenylhydrazine and Erythropoietin in Mice Genetically Impaired for Their GATA-1 Expression (GATA-1low Mice). Blood 97 (10), 3040–3050. doi:10.1182/blood.v97.10.3040
Vannucchi, A. M., Bianchi, L., Cellai, C., Paoletti, F., Rana, R. A., Lorenzini, R., et al. (2002). Development of Myelofibrosis in Mice Genetically Impaired for GATA-1 Expression (GATA-1low Mice). Blood 100 (4), 1123–1132. doi:10.1182/blood-2002-06-1913
Vannucchi, A. M., Pancrazzi, A., Guglielmelli, P., Di Lollo, S., Bogani, C., Baroni, G., et al. (2005). Abnormalities of GATA-1 in Megakaryocytes from Patients with Idiopathic Myelofibrosis. Am. J. Pathol. 167 (3), 849–858. doi:10.1016/s0002-9440(10)62056-1
Varricchio, L., Dell’Aversana, C., Nebbioso, A., Migliaccio, G., Altucci, L., Mai, A., et al. (2014). Identification of NuRSERY, a New Functional HDAC Complex Composed by HDAC5, GATA1, EKLF and pERK Present in Human Erythroid Cells. Int. J. Biochem. Cel Biol. 50, 112–122. doi:10.1016/j.biocel.2014.02.019
Vyas, P., Ault, K., Jackson, C. W., Orkin, S. H., and Shivdasani, R. A. (1999). Consequences of GATA-1 Deficiency in Megakaryocytes and Platelets. Blood 93 (9), 2867–2875. doi:10.1182/blood.v93.9.2867
Wang, H., Georgakopoulou, A., Psatha, N., Li, C., Capsali, C., Samal, H. B., et al. (2019). In Vivo hematopoietic Stem Cell Gene Therapy Ameliorates Murine Thalassemia Intermedia. J. Clin. Invest. 129 (2), 598–615. doi:10.1172/JCI122836
Wang, H., He, J., Xu, C., Chen, X., Yang, H., Shi, S., et al. (2021). Decoding Human Megakaryocyte Development. Cell Stem Cell 28 (3), 535–549.e8. doi:10.1016/j.stem.2020.11.006
Wechsler, J., Greene, M., McDevitt, M. A., Anastasi, J., Karp, J. E., Le Beau, M. M., et al. (2002). Acquired Mutations in GATA1 in the Megakaryoblastic Leukemia of Down Syndrome. Nat. Genet. 32 (1), 148–152. doi:10.1038/ng955
Yeung, A. K., Villacorta-Martin, C., Hon, S., Rock, J. R., and Murphy, G. J. (2020). Lung Megakaryocytes Display Distinct Transcriptional and Phenotypic Properties. Blood Adv. 4 (24), 6204–6217. doi:10.1182/bloodadvances.2020002843
Yu, C., Cantor, A. B., Yang, H., Browne, C., Wells, R. A., Fujiwara, Y., et al. (2002). Targeted Deletion of a High-Affinity GATA-Binding Site in the GATA-1 Promoter Leads to Selective Loss of the Eosinophil Lineage In Vivo. J. Exp. Med. 195 (11), 1387–1395. doi:10.1084/jem.20020656
Yu, C., Niakan, K. K., Matsushita, M., Stamatoyannopoulos, G., Orkin, S. H., and Raskind, W. H. (2002). X-linked Thrombocytopenia with Thalassemia from a Mutation in the Amino finger of GATA-1 Affecting DNA Binding rather Than FOG-1 Interaction. Blood 100 (6), 2040–2045. doi:10.1182/blood-2002-02-0387
Zahr, A. A., Salama, M. E., Carreau, N., Tremblay, D., Verstovsek, S., Mesa, R., et al. (2016). Bone Marrow Fibrosis in Myelofibrosis: Pathogenesis, Prognosis and Targeted Strategies. Haematologica 101 (6), 660–671. doi:10.3324/haematol.2015.141283
Keywords: GATA1, μLCR, erythroid cells, megakaryocytes, hematopoietic progenitor cells
Citation: Martelli F, Verachi P, Zingariello M, Mazzarini M, Vannucchi AM, Lonetti A, Bacci B, Sarli G and Migliaccio AR (2021) hGATA1 Under the Control of a μLCR/β-Globin Promoter Rescues the Erythroid but Not the Megakaryocytic Phenotype Induced by the Gata1low Mutation in Mice. Front. Genet. 12:720552. doi: 10.3389/fgene.2021.720552
Received: 04 June 2021; Accepted: 24 September 2021;
Published: 11 October 2021.
Edited by:
Emile Van Den Akker, Sanquin Research, NetherlandsReviewed by:
Roberta Russo, University of Naples Federico II, ItalySanalkumar Rajendran, Centre Hospitalier Universitaire Vaudois (CHUV), Switzerland
Copyright © 2021 Martelli, Verachi, Zingariello, Mazzarini, Vannucchi, Lonetti, Bacci, Sarli and Migliaccio. This is an open-access article distributed under the terms of the Creative Commons Attribution License (CC BY). The use, distribution or reproduction in other forums is permitted, provided the original author(s) and the copyright owner(s) are credited and that the original publication in this journal is cited, in accordance with accepted academic practice. No use, distribution or reproduction is permitted which does not comply with these terms.
*Correspondence: Anna Rita Migliaccio, YS5taWdsaWFjY2lvQHVuaWNhbXB1cy5pdA==