- 1Department of Cardiology, Shengjing Hospital of China Medical University, Shenyang, China
- 2Department of Neurology, Shengjing Hospital of China Medical University, Shenyang, China
Non-coding RNAs (ncRNAs) are involved in various cellular processes. There are several ncRNA classes, including microRNAs (miRNAs), long non-coding RNAs (lncRNAs), and circular RNAs (circRNAs). The detailed roles of these molecules in pulmonary hypertension (PH) remain unclear. We systematically collected and reviewed reports describing the functions of ncRNAs (miRNAs, lncRNAs, and circRNAs) in PH through database retrieval and manual literature reading. The characteristics of identified articles, especially the experimental methods, were carefully reviewed. Furthermore, regulatory networks were constructed using ncRNAs and their interacting RNAs or genes. These data were extracted from studies on pulmonary arterial smooth muscle cells, pulmonary artery endothelial cells, and pulmonary artery fibroblasts. We included 14 lncRNAs, 1 circRNA, 74 miRNAs, and 110 mRNAs in the constructed networks. Using these networks, herein, we describe the current knowledge on the role of ncRNAs in PH. Moreover, these networks actively provide an improved understanding of the roles of ncRNAs in PH. The results of this study are crucial for the clinical application of ncRNAs.
1 Introduction
Pulmonary hypertension (PH) is a serious disease characterized by progressively increased pulmonary vascular resistance and pulmonary artery pressure; the diagnostic criterion is mean pulmonary artery pressure ≥25 mmHg (Galiè et al., 2016; Weber et al., 2018). The increased pulmonary artery pressure in PH results from changes in the structure and function of the vessel wall, which is induced by abnormal pulmonary cell proliferation, apoptosis, and migration (Bourgeois et al., 2018a). Patients with PH may experience dyspnea, fatigue, syncope, chest pain, and/or edema of the legs and ankles. The causes of PH can be broadly classified as primary and secondary causes. To date, ion channels, vasoactive substances, immune factors, and genetic factors are known to be involved in the pathogenesis of PH (Chelladurai et al., 2016; Veith et al., 2016; Bourgeois et al., 2018b).
Recently, many non-coding RNAs (ncRNAs) have been recognized as important regulators in the development of PH. Most human genes (>95%) do not produce proteins but ncRNA molecules. Among them, microRNAs (miRNAs), long non-coding RNAs (lncRNAs), and circular RNAs (circRNAs) are the most widely studied. MiRNAs are small ncRNAs containing 21–22 nucleotides, which post-transcriptionally regulate gene expression (Wakiyama and Yokoyama. 2014). LncRNAs, which have more than 200 nucleotides, are transcribed from intergenic or intragenic regions. They can bind to proteins, RNA, or DNA to execute regulatory roles (Botti et al., 2017). CircRNAs are a novel class of ncRNAs with a closed loop structure, making them highly stable and capable of interacting with proteins or RNA (Di et al., 2019). NcRNAs have been identified to regulate multiple steps of gene expression. However, because of the large quantity and diverse mechanisms, it is difficult to comprehensively understand the roles of ncRNAs.
NcRNA-based therapeutics have emerged for several diseases, including PH. An effective ncRNA-based strategy demands a thorough understanding of the diverse and context-dependent regulatory relationships of ncRNAs. The regulation of gene expression by ncRNAs is frequently cell specific, suggesting that not only expression level, but also activity or bioavailability contribute to the biofunction of ncRNAs (Correia de Sousa et al., 2019). Thus, in this article, we reviewed the published literature to search for functional miRNAs, lncRNAs, and circRNAs in PH. Next, we constructed networks of validated ncRNAs and their interacting RNAs or genes to investigate the role of ncRNAs in PH.
2 Screening of Articles
2.1 Criteria for Study Selection
A literature search was performed in PubMed with the query listed in Table 1; we identified 602 articles. In addition, we also reviewed other public databases, including the Human microRNA Disease Database v3.2, miRWalk 2.0, and LncRNADisease v2.0, to identify validated functional ncRNAs in PH. Studies were selected when the following criteria were met: 1) the study reported pathogenic roles of miRNAs, lncRNAs, and/or circRNAs in PH; 2) mechanistic studies were performed in pulmonary arterial smooth muscle cells (PASMCs), pulmonary artery endothelial cells (PAECs), and/or pulmonary artery fibroblasts (PAFs); and 3) the relationships between ncRNAs and their interacting RNAs or genes were experimentally identified via luciferase reporter assay, western blot, and/or qPCR. Using these criteria returned 140 qualified articles (Figure 1).
2.2 General Characteristics of Qualified Articles
When sorted by publication date, we found that the number of eligible articles continuously increased year by year (Figure 2A). The impact factors (IF) of the articles ranged from 0 to 36.13; articles with 3 ≤ IF < 5 accounted for the highest proportion (Figure 2B). Of the 140 qualified articles, 32.14% were studies using human tissues or cells. In studies using experimental animals, rats were the most commonly used, accounting for 26.43% of the total studies (Figure 2C). Moreover, when classified by cell type, 78.42, 15.83, 1.44, and 4.32% of studies were performed in PASMCs, PAECs, PAFs, and both PASMCs and PAECs, respectively (Figure 2D).
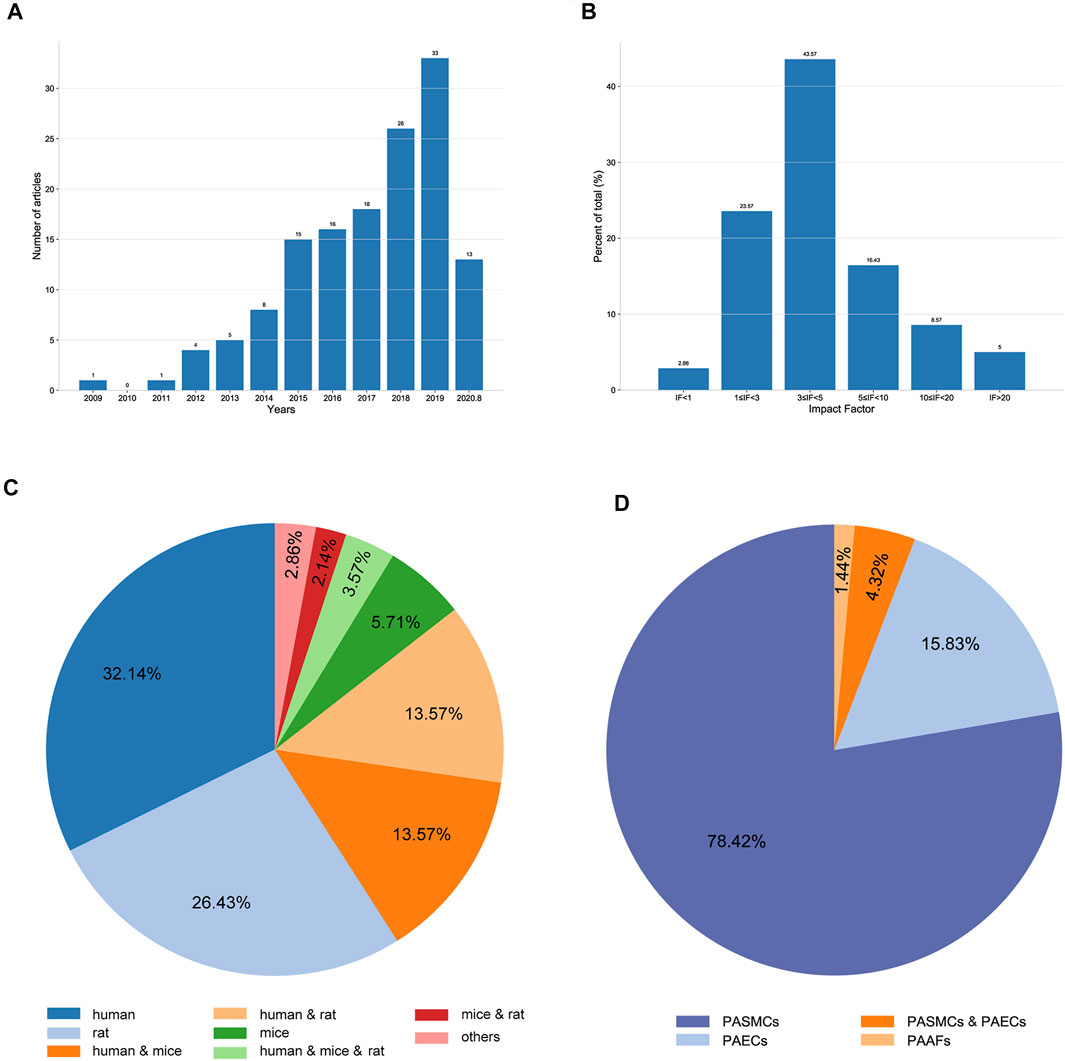
FIGURE 2. Characteristics of the extracted studies. (A) Distribution of the included articles according to the publication year. (B) Distribution of the included articles according to the impact factor. (C) Proportions of different species studied in the included articles. (D) Proportions of different cell types used in the included articles.
3 Non-coding RNA Networks for Pulmonary Hypertension
3.1 Construction of Non-coding RNA Regulatory Networks
Regulatory networks were constructed using ncRNAs and their interacting RNAs or genes in PASMCs, PAECs, and PAFs. Given ncRNA conservation among species, only human ncRNAs or ncRNAs that were conserved between human and experimental animals were included. If there were contradictory results, the results from higher-impact articles were selected. In addition, some crucial regulatory relationships between protein-coding genes and validated transcription factor–miRNA interactions from TransmiR v2.0 were also described in the networks to present an in-depth explanation on the roles of ncRNAs in PH. The nodes represented interacting molecules, and the edges represented the regulatory connections. Each edge indicated a publication supporting the connection. Square and circular nodes represented ncRNAs and coding RNAs or genes, respectively. Node color was based on the type of molecule (lncRNAs and circRNAs are orange, miRNAs are blue, and coding RNAs or genes are empty). Node sizes represented their degrees (number of edges that directly link to the node). Edges represented the regulatory connections: red edges depicted links indicating repressive action (semicircular arrow heads), and black edges indicated activation (traditional arrow heads). The nodes in this network were involved in cell proliferation, apoptosis, migration, metabolism, endothelial–mesenchymal transition, and extracellular matrix remodeling. The steps used in our approach are shown in Figure 1.
3.2 General Characteristics of the Constructed Networks
In total, 140 articles describing 14 lncRNAs, 1 circRNA, 74 miRNAs, and 110 mRNAs, were included in our networks. Considering the unique biological characteristics of different cell types, we constructed networks according to cell type. The network of PASMCs contained 13 lncRNAs, 1 circRNA, 69 miRNAs, and 96 mRNAs. The network of PAECs contained 1 lncRNA, 25 miRNAs, and 29 mRNAs. The network of PAFs contained 6 miRNAs and four mRNAs. The networks are shown in Figures 3–5. Detailed network compositions are listed in Table 2.
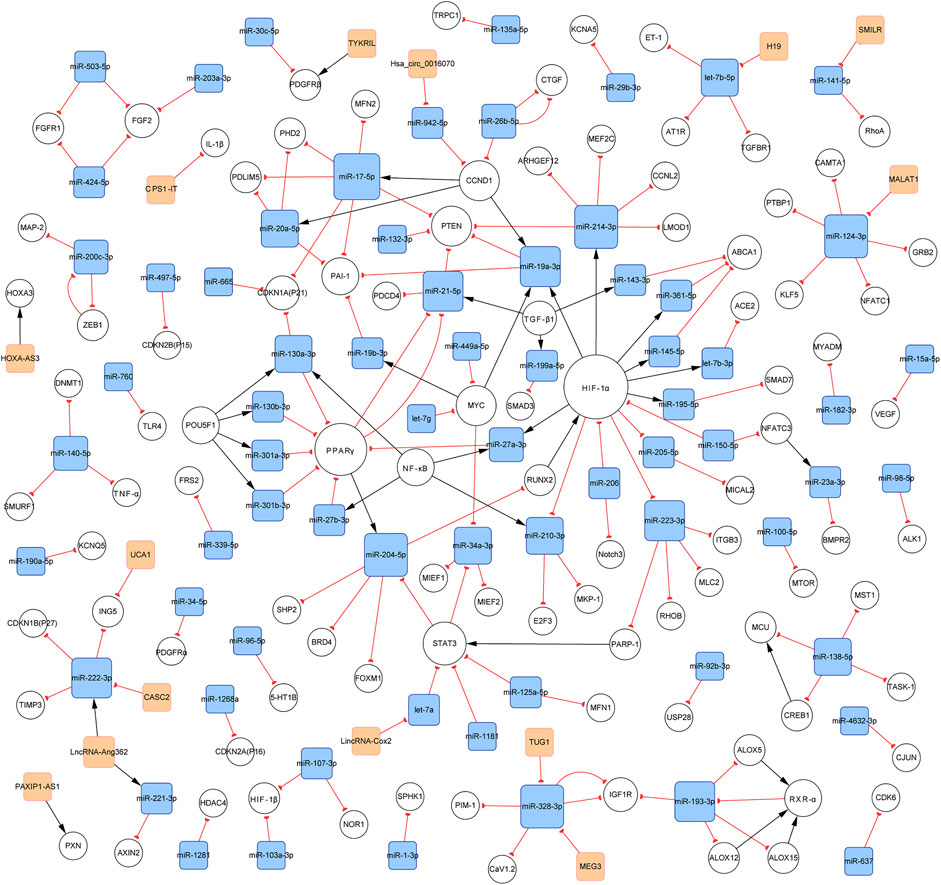
FIGURE 3. PH-associated network of ncRNAs and their interacting RNAs or genes in PASMCs. The square and circular nodes represent ncRNAs and coding RNAs or genes, respectively. Node color is based on the type of molecule (lncRNAs and circRNAs are orange, miRNAs are blue, and coding RNAs or genes are empty). Node sizes represent the degrees (the number of edges that directly link to the node). Edges represent the regulatory connections, and each edge indicates a publication. When multiple publications describe one interaction, multiple edges connect the same two nodes. Red edges depict links indicating repressive action (semicircular arrow heads), and black edges are those indicating activation (with traditional arrow heads). The nodes in this network are involved in cell proliferation, apoptosis, migration, and metabolism. PH: pulmonary hypertension; PASMCs: pulmonary artery smooth muscle cells; lncRNAs: long non-coding RNAs; circRNA: circular RNA; miRNA: microRNA.
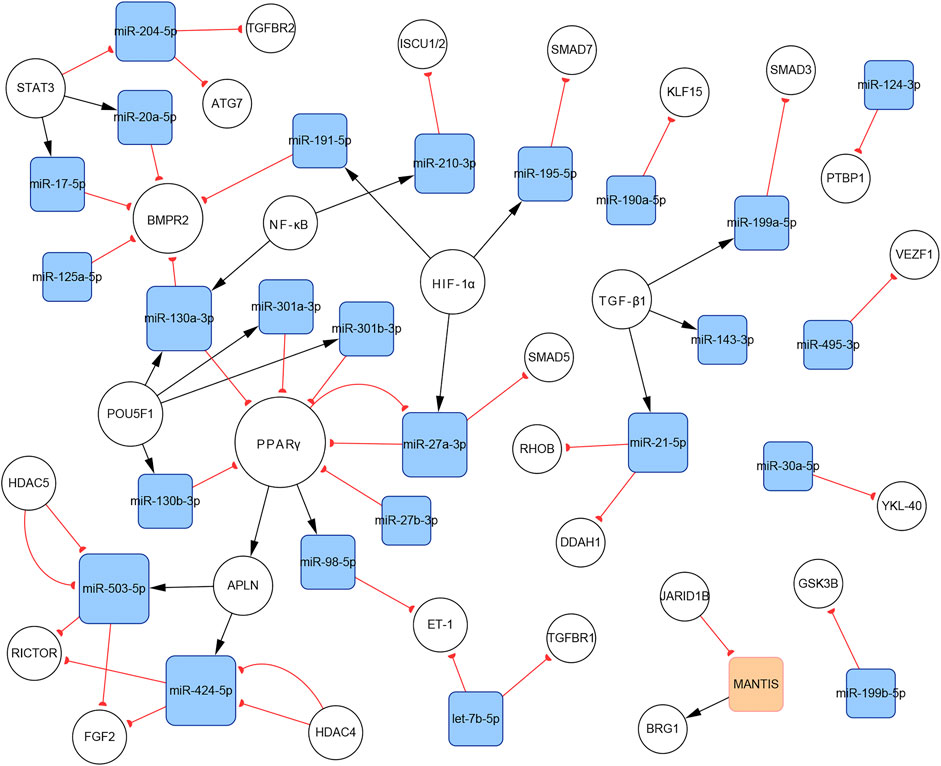
FIGURE 4. The PH-associated network of ncRNAs and their interacting RNAs or genes in PAECs. The square and circular nodes represent ncRNAs and coding RNAs or genes, respectively. Node color is based on the type of molecule (lncRNAs are orange, miRNAs are blue, and coding RNAs or genes are empty). Node sizes represent the degrees (number of edges that directly link to the node). Edges represent regulatory connections. Each edge indicates a publication. When multiple publications describe one interaction, multiple edges connect the same two nodes. Red edges depict links indicating repressive action (semicircular arrow heads), and black edges represent those indicating activation (traditional arrow heads). The nodes in this network were primarily involved in proliferation, apoptosis resistance, migration, and endothelial–mesenchymal transition. PAECs: pulmonary artery endothelial cells.
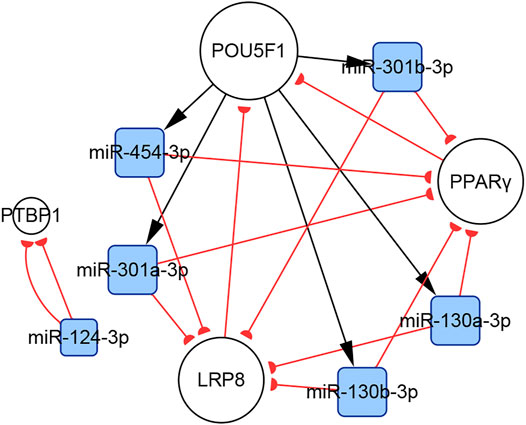
FIGURE 5. The PH-associated network of ncRNAs and their interacting RNAs or genes in PAFs. The square and circular nodes represent ncRNAs and coding RNAs or genes, respectively. Node color is based on the type of molecule (miRNAs are blue, coding RNAs or genes are empty). Node sizes represent the degrees (number of edges that directly link to the node). Edges represent regulatory connections. Each edge indicates a publication. When multiple publications describe one interaction, multiple edges connect the same two nodes. Red edges depict links indicating repressive action (semicircular arrow heads), and black edges are those indicating activation (traditional arrow heads). The nodes in this network were primarily involved in cell proliferation and extracellular matrix remodeling. PAFs: pulmonary artery fibroblasts.
3.3 Functional Enrichment Analysis
We performed gene ontology (GO) biological process term analyses and Kyoto Encyclopedia of Genes and Genomes (KEGG) pathway analyses using the database for Annotation, Visualization, and Integration Discovery (DAVID). The calculation process was dependent on a hypergeometric test, using a statistical significance threshold of p < 0.05 with a false discovery rate (FDR) correction. These analyses provided a general overview of the biological roles of the included ncRNAs. In addition, we performed cell type-specific functional enrichment analyses. However, owing to the lack of sufficient molecules, the enrichment analysis in PAFs could not be conducted. GO analysis and KEGG pathway enrichment in PASMCs and PAECs revealed several PH-associated terms, such as positive regulation of smooth muscle cell proliferation, positive regulation of endothelial cell proliferation, HIF-1 signaling pathway, and MAPK signaling pathway. The top 10 enriched GO biological process terms and KEGG pathways are shown in Figure 6.
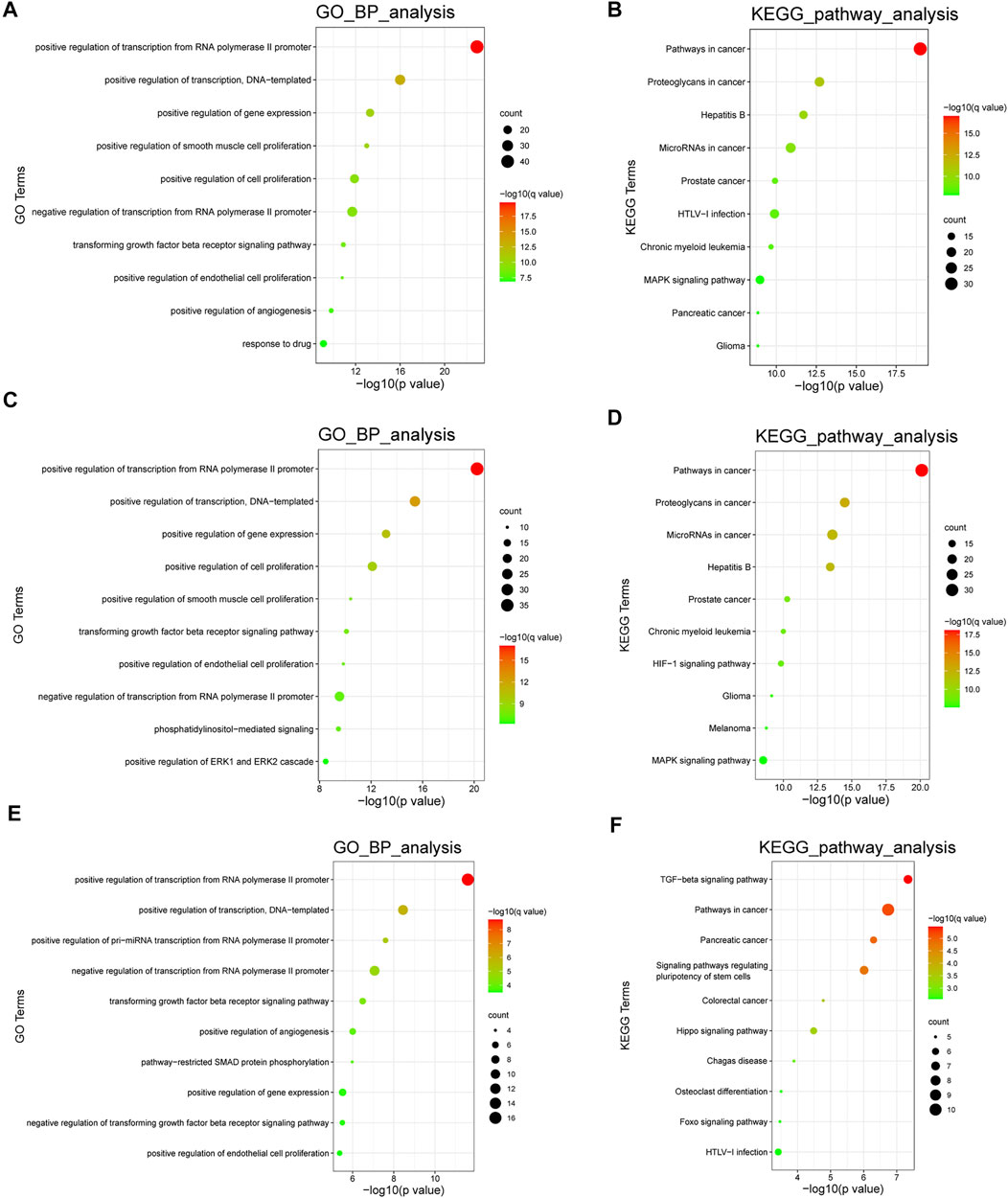
FIGURE 6. Functional analyses of the ncRNAs. (A,B). The top 10 enriched GO biological process terms and KEGG pathways in all cell types. (C,D) The top 10 enriched GO biological process terms and KEGG pathways in PASMCs. (E,F) The top 10 enriched GO biological process terms and KEGG pathways in PAECs. Node sizes indicate the number of genes enriched in functional clusters. Node colors are related to q values. GO: gene ontology; KEGG: Kyoto Encyclopedia of Genes and Genomes.
3.4 Key Non-coding RNA Subnetworks
We built three networks according to the cell types. Here, we discuss several important subnetworks, along with their components and interactions, to improve understanding of the roles of ncRNAs in PH. Subnetworks with more than five nodes were regarded as key subnetworks.
3.4.1 The Hsa_circ_0016070/miR-942-5p/CCND1 Subnetwork
CircRNAs are associated with various cardiovascular diseases. Hsa_circ_0016070 was the only circRNA included in our networks. This circRNA is located at chr1: 203595914-203702528, strand: +, promotes cell proliferation by mediating cell cycle progression, and is increased in PH patients (Zhou et al., 2019). CCND1 is an important regulator of the cell cycle. It interacts with cyclin-dependent kinase 4 (CDK4) to form the cyclin D1–CKD4 complex, which then inactivates retinoblastoma (Rb) protein and induces G0 progression to S phase (Matsushime et al., 1991). The subnetwork showed that hsa_circ_0016070 overexpression induced CCND1 expression by buffering miR-942-5p (Zhou et al., 2019). In addition, according to our network, CCND1 could induce the expression of miR-17-5p, miR-19a-3p, and miR-20a-5p, subsequently regulating the biological activities of PASMCs (Figure 7A). Given the considerable number of identified circRNAs, there should be other PH-related circRNAs. A microarray expression profile in thromboembolic pulmonary hypertension patients indicated that hsa_circ_0002062 and hsa_circ_0022342 might be the key circRNAs for the development of chronic thromboembolic pulmonary hypertension (Miao et al., 2017). However, this finding has not been verified by more reliable experimental methods.
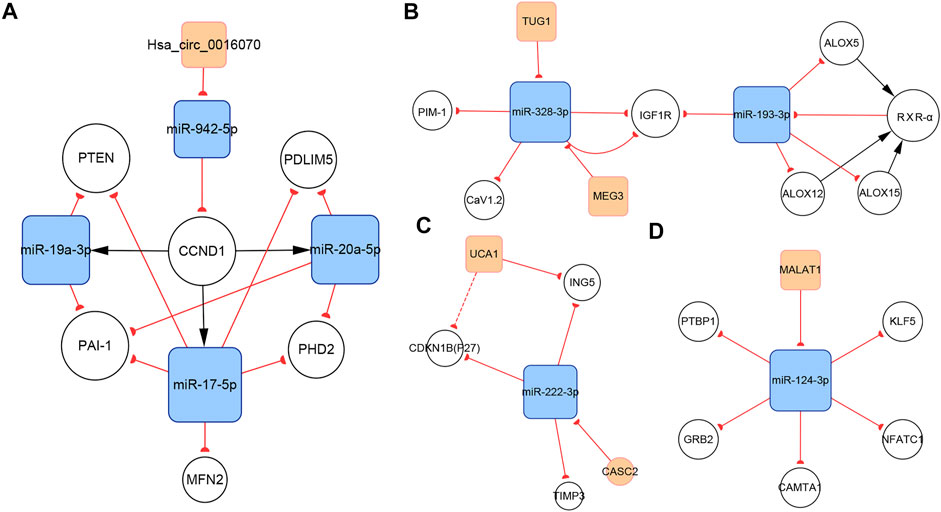
FIGURE 7. Several key ncRNA subnetworks. (A) The hsa_circ_0016070/miR-942-5p/CCND1 subnetwork. (B) The TUG1/MEG3/miR-328-3p/miR-193-3p subnetwork. (C) The CASC2/UCA1/miR-222-3p subnetwork. (D) The MALAT1/miR-124-3p subnetwork.
3.4.2 The TUG1/MEG3/miR-328-3p/miR-193-3p Subnetwork
Regulatory relationships are indicated by the connection lines in the subnetwork. According to this subnetwork (Figure 7B), both TUG1 and MEG3 can function as competing endogenous RNAs (ceRNAs) that sequester miR-328-3p. In the original studies, the TUG1/miR-328-3p and MEG3/miR-328-3p axes were identified (Wang D et al., 2019; Xing X.-Q et al., 2019). IGF1 is reported to inhibit PASMCs apoptosis and activate elastin in PASMCs. Thus, upregulating IGF1R via the TUG1/miR-328-3p and MEG3/miR-328-3p axes can induce PH by amplifying the pathogenic role of IGF1 (Wang S et al., 2019; Xing Y et al., 2019). Calcium voltage-gated channel subunit alpha1 C (CaV1.2), which contributes to vasoconstriction, is also a target gene of miR-328-3p in PASMCs (Guo et al., 2012), indicating that the TUG1/miR-328-3p and MEG3/miR-328-3p axes are involved in regulating pulmonary artery contraction and dilation. In addition, miR-328-3p can inhibit PASMC proliferation by targeting PIM-1 (Qian et al., 2016). Available data show that miR-193-3p has a shared target gene, IGF1R, with miR-328-3p, but no strong regulatory connection with miR-328-3p or TUG1 or MEG3. Thus, downregulation of miR-193-3p contributes to IGF1R overexpression as well. In addition, miR-193-3p is capable of negatively regulating multiple lipoxygenases, including ALOX5, ALOX12, and ALOX15. These lipoxygenases cause abnormal lipid metabolism, which not only directly accelerates the development of PH, but also induces the increasement of RXR-α. Moreover, miR-193-3p can be downregulated by RXR-α, which directly binds to the miR-193 promoter. Therefore, a feedback loop, which dramatically enhances abnormal miR-193-3p expression forms (Sharma et al., 2014).
3.4.3 The CASC2/UCA1/miR-222-3p Subnetwork
LncRNA CASC2 is downregulated in hypoxia-induced PASMCs. As a ceRNA of miR-222-3p, CASC2 reduces the expression of ING5, which is a target gene of miR-222-3p, ultimately promoting PASMC proliferation and migration (Han et al., 2020). P27 and TIMP3 are two additional target genes of miR-222-3p (Xu et al., 2017). P27, a member of the Cip/Kip family of cyclin-dependent kinase inhibitors, negatively regulates cell proliferation (Toyoshima and Hunter, 1994). Meanwhile, TIMP3 is a member of the TIMP family, which regulates cell proliferation, apoptosis, and migration via both MMP-dependent or MMP-independent pathways (Zhou et al., 2015). The present subnetwork links CACS2 to P27 and TIMP3 via miR-222-3p, further elaborating the mechanisms of PH (Figure 7C).
UCA1 is the other lncRNA in this subnetwork and is highly expressed in hypoxia-induced PASMCs. Studies indicate that UCA1 does not interact with miR-222-3p, but directly inhibits ING5 by competing with ING5 mRNA for hnRNP I, which binds to ING5 mRNA and enhances its translation. Thus, UCA1 overexpression results in the downregulation of ING5 mRNA expression (Zhu T.-T. et al., 2019). The same regulatory pattern has been found between UCA1 and P27 in breast tumor studies (Huang et al., 2014). This interaction may also work in PH and partly contributes to P27 downregulation (Figure 7C).
3.4.4 The MALAT1/miR-124-3p Subnetwork
LncRNA MALAT1, located at 11q13, is an 8.5-kb molecule that was identified by Ji et al. in a cancer study (Ji et al., 2003). Emerging evidence indicates that MALAT1 plays important roles in various diseases, including PH. Wang et al. reported that MALAT1 is highly expressed in pulmonary artery tissues and PASMCs from patients with PH. MALAT1 controls PASMC proliferation and migration by binding to miR-124-3p, which directly targets KLF5 (Wang D et al., 2019). Kang et al. showed that miR-124-3p also targets three regulators of the NFAT pathway, including NFATc1, CAMTA1, and PTBP1 (Kang B.-Y et al., 2013). The downregulation of miR-124-3p induces PASMC proliferation and reverses the differentiated PASMC phenotype by activating the NFAT pathway. In addition to its role in PASMCs, miR-124-3p also regulates the biological behaviors of PAH endothelial cells (PAH ECs) and PAFs. Studies have confirmed the role of the miR-124-3p/PTBP1 axis in PAH ECs and PAFs (Caruso et al., 2017; Wang et al., 2014; Zhang H et al., 2017). Downregulating miR-124-3p activates PTBP1 expression, which promotes aerobic glycolysis by increasing the PKM2/PKM1 ratio, subsequently inducing PAH EC and PAF proliferation (Anastasiou et al., 2012). Li et al. reported another target of miR-124-3p, GRB2, which enhanced the proliferation of multiple human cells (Li L et al., 2017; Figure 7D).
3.4.5 Subnetworks of the miR-130/301 Family
There are complicated relationships between the miR-130/301 family and other functional molecules associated with the pathogenesis of PH. In the present study, we found that subnetworks of the miR-130/301 family were involved in multiple biological behaviors, such as proliferation, apoptosis, and migration in PASMCs, PAECs, and PAFs. In addition, these subnetworks also mediated the crosstalk of these pulmonary artery cells.
In PASMCs, the miR-130/301 family is involved in many regulatory axes. Among them, the POU5F1/miR-130/301 family/PPARγ axis, which regulates the expression of miR-204-5p and miR-21-5p, is the most explicitly elaborated axis. According to our studies, the identified target genes of the two miRNAs in PASMCs include BRD4, FOXM1, PSCD4, PTEN, RUNX2, and SHP2, which control cell proliferation, apoptosis, differentiation, and mitochondrial function (Courboulin et al., 2011; Meloche et al., 2015a; Green et al., 2015, 2017; Ruffenach et al., 2016; Liu et al., 2017; Bourgeois et al., 2018a). In addition to the miR-130/301 family, miR-27a/b-3p, which is regulated by NF-κB (Xie et al., 2017), can also act as an upstream controller of PPARγ in PASMCs. Interestingly, the subnetwork analysis indicates that the miR-130/301 family indirectly promotes HIF-1α expression by sustaining the RUNX2 level (Ruffenach et al., 2016). Conversely, HIF-1α induces the expression of miR-27a-3p, which depresses the level of PPARγ (Camps et al., 2014). Thus, a feedback loop with PPARγ and HIF-1α forms. This loop leads to a persistent pathological status. Moreover, as a crucial pathogenic molecule for PH, HIF-1α can function through several miRNAs, including miR-145-5p, miR-19a-3p, miR-195-5p, miR-210-3p, miR-223-3p, and miR-361-5p, to regulate the expression of downstream proteins, eventually causing abnormal cellular behaviors (Agrawal et al., 2014; Gou et al., 2012; Meloche et al., 2015b; Zeng et al., 2018; Zhang X et al., 2018, Zhang H et al., 2019; Zhao et al., 2019; Figure 8A).
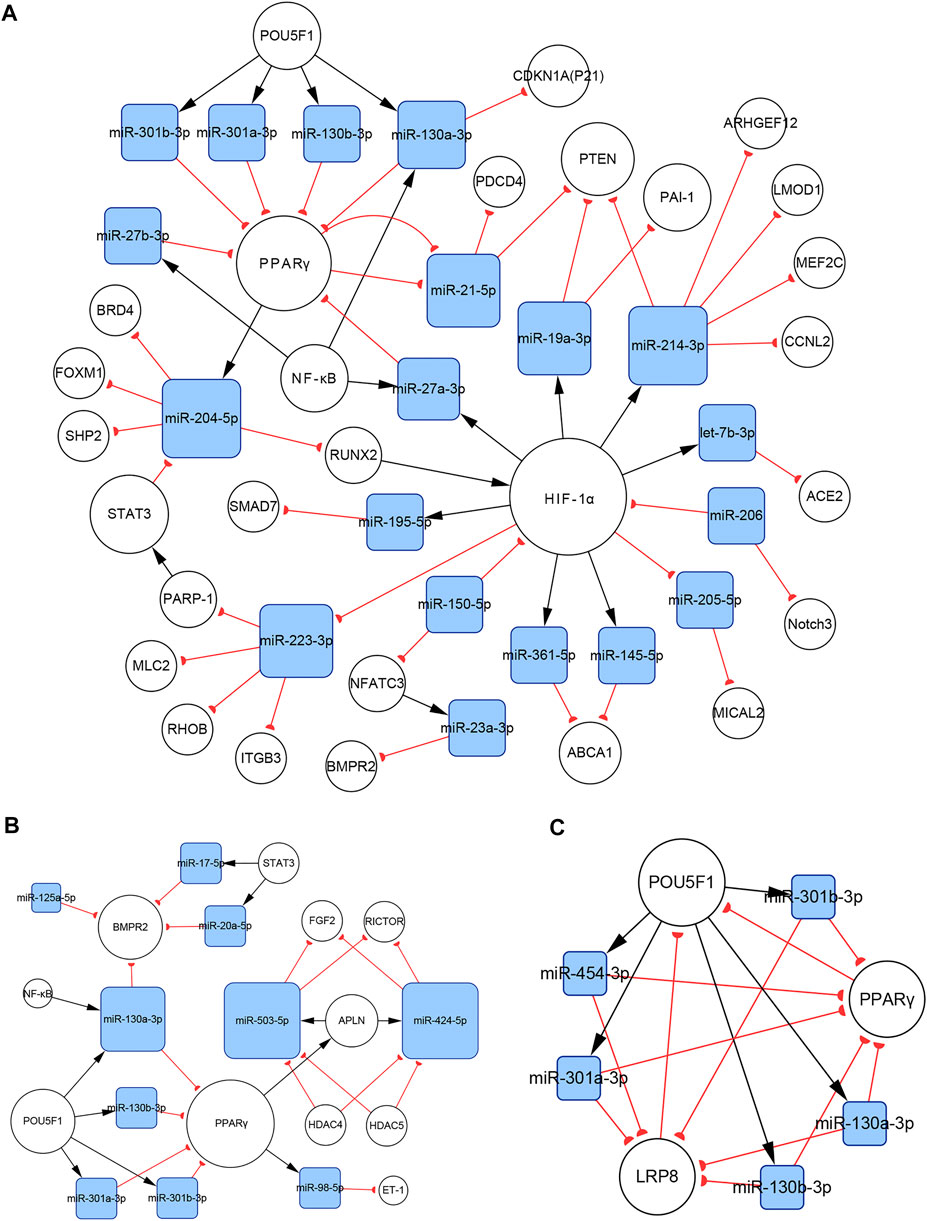
FIGURE 8. The miR-130/301 family subnetworks in (A) PASMCs, (B) PAECs, and (C) PAFs. This miRNA family was involved in multiple processes, such as cell proliferation, apoptosis, migration, endothelial contraction, and matrix remodeling.
In PAECs, the miR-130/301 family also plays an important role. The POU5F1/miR-130/301 family/PPARγ axis indirectly regulates the expression of ET-1 and FGF2 via miR-98-5p and miR-424/503-5p, respectively (Kim et al., 2013; Zhang Y et al., 2018). The roles of ET-1 and FGF2 in PH are well established. ET-1 is synthesized primarily in endothelial cells and mediates pulmonary artery cell proliferation, migration, and constriction through two distinct G protein-coupled receptors: ETA and ETB (Clozel, 2016). Previous studies suggest that excessive FGF2 expression promotes PAEC proliferation by activating ERK1/2 and inhibits apoptosis by inducing BCL2 and BCL-xL activity (Tu et al., 2011). Furthermore, miR-130a-3p controls the level of BMPR2, which triggers idiopathic pulmonary artery hypertension (IPAH) and is involved in the development of other types of PH (Li Q et al., 2017). Considering that miRNAs from the same family have a homologous seed region sequence, other members from the miR-130/301 family may also regulate BMPR2 expression. The transcription of miR-130a-3p is controlled by NF-κB in PAECs. Thus, NF-κB and BMPR2 are linked by miR-130a-3p. In addition, miR-17a-5p, miR-20a-5p, and miR-125a-5p also mediate BMPR2 expression. Besides, two members from the miR-17-92 family, miR-17a-5p and miR-20a-5p, link STAT3 to BMPR2 (Brock et al., 2009; Huber et al., 2015; Figure 8B).
In PAFs, activation of the miR-130/301 family can induce cell proliferation and extracellular matrix remodeling by inhibiting PPARγ and LRP8. Meanwhile, matrix remodeling can activate POU5F1, which subsequently promotes miR-130/301 family expression (Bertero et al., 2015). Thus, a positive feedback circuit is activated that dramatically accelerates the development of PH (Figure 8C).
The roles of the miR-130/301 family in different pulmonary artery cell types are not independent. Rather, the miR-130/301 family contributes to crosstalk between these cells. Extracellular matrix remodeling, which can be induced by overexpression of the miR-130/301 family, promotes proliferation and contraction of pulmonary artery cells viamiR-130/301 family-dependent and -independent pathways. The remodeled extracellular matrix can activate the POU5F1/miR-130/301 family/PPARγ axis in PASMCs, PAECs, and PAFs, subsequently regulating downstream molecules such as miR-204-5p, miR-424-5p, miR-503-5p, and FGF2 (Bertero et al., 2015). Upregulating miR-424-5p and miR-503-5p or inhibiting FGF2 in PAECs can repress PASMC and PAF proliferation induced by conditioned media from PAECs, indicating that these molecules are involved in the crosstalk among different pulmonary vascular cells. The remodeled extracellular matrix can also induce the expression of the proliferative miRNA, miR-27a/b-3p, in PACEs, and PASMCs, as well as the expression of the vasoconstrictor ET-1, and the inflammatory cytokine IL-6 in PACEs (Bertero et al., 2014; Bertero et al., 2015; Figure 9).
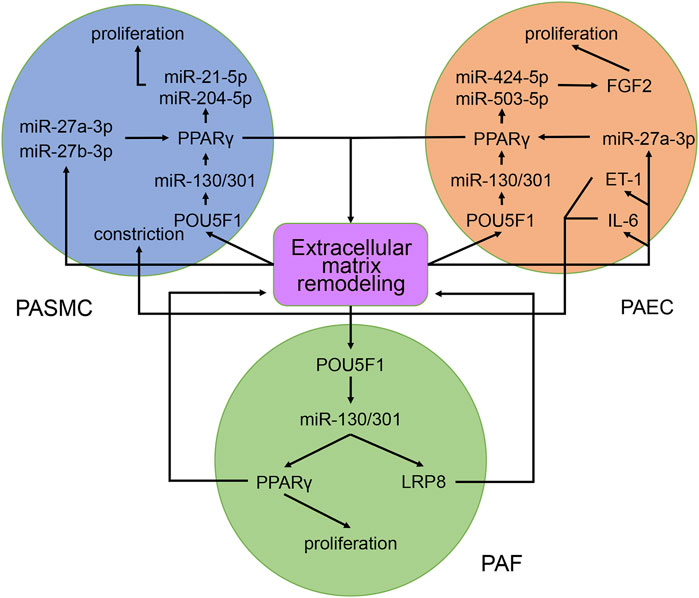
FIGURE 9. Contribution of the miR-130/301 family to the crosstalk between various pulmonary artery cells. The remodeled extracellular matrix induces proliferation and contraction in pulmonary artery cells via miR-130/301 family-dependent and -independent pathways. Meanwhile, matrix remodeling can be induced by overexpression of the miR-130/301 family.
4 Environmental Factors in Pulmonary Hypertension
Noncoding RNA interference is an important epigenetic mechanism. Recent evidence has identified the roles of epigenetic changes in the development of PH. These changes link the pathogenic genes of PH and environmental factors such as hypoxia, virus infection, and air pollution (Gamen et al., 2016). For example, BMPR2 is a transmembrane serine/threonine kinase receptor, which is essential for vascular homeostasis. Although mutations in the BMPR2 gene account for a considerable portion of patients with familial pulmonary artery hypertension (FPAH), only 20–30% of carriers with mutations in this gene suffer from PH, indicating that other factors contribute to the onset of the disease (Orriols et al., 2017; Zhao et al., 2019). According to our network, hypoxia can induce the expression of several miRNAs by HIF-1α, such as, miR-145-5p, miR-19a-3p, miR-191-5p, miR-214-3p, and miR-27a-3p (Agrawal et al., 2014; Camps et al., 2014; el Azzouzi et al., 2013; Song et al., 2014; Zhao et al., 2019). Among them, miR-191-5p can increase cell proliferation, impair apoptosis, and induce phenotypic alteration through inhibiting BMPR2 expression, subsequently contributing to vascular remodeling (Song et al., 2014). Therefore, the HIF-1α/miR-191-5p/BMPR2 axis reveals the connection between hypoxia and BMPR2 expression and partially explains the incomplete penetrance of BMPR2 mutations in FPAH.
5 Potential Applications of Non-coding RNAs
Ultimately, studies on molecular mechanisms aim to inform clinical practices. NcRNAs are potential diagnostic biomarkers for PH. For example, circRNAs are not easily degraded, making them ideal serum biomarkers. Zhang et al. reported hsa_circ_0068481 overexpression in the serum from patients with IPAH. Furthermore, hsa_circ_0068481 expression is significantly correlated with 6-min walk distance, N-terminal pro-B-type natriuretic peptide, H2S, pulmonary hypertension risk stratification, right heart failure, and survival rate (Zhang et al., 2019a). However, because of the absence of an associated molecular mechanism, this circRNA was not included in our networks. NcRNAs may also act as potential therapeutic targets for PH. For example, Rothman et al. identified downregulation of miR-140-5p in a rat PH model. In vitro, miR-140-5p mimics suppressed PASMC proliferation and migration. In vivo, miR-140-5p mimics prevented the progression of established PH in rats (Rothman et al., 2016). The results are encouraging. However, ncRNA therapy is far from being applied in clinical settings, since a ncRNA may have diverse biofunctions. This means that when used as therapeutic agent, a ncRNA may cause adverse effects, some of which may even be life-threatening. In our opinion, carefully selected ncRNA targets and well-designed action sites can be helpful to avoid such adverse effects. These measures require a comprehensive and in-depth understanding of the mechanisms of ncRNAs in diseases. In this study, we constructed networks to demonstrate the current findings on ncRNAs from studies performed in PH patients and animal models. However, shortcomings of these studies, including the paucity of human data, sex bias, and heterogeneity of animal models, limit the translation of these findings into applications for human disease. Therefore, further studies should be performed to confirm these findings in different animal models and patient cohorts of PH. Additionally, large, well-designed, and unbiased clinical studies are required to illuminate further application of ncRNAs.
6 Conclusion
The roles of ncRNAs in PH remained unclear. In this study, we performed an extensive literature search and adopted uniform and strict criteria for the selection of each article to avoid biased outcomes. The ncRNA networks were constructed by assembling ncRNAs and their interacting RNAs or genes from included articles. These networks provide a better understanding of the roles of ncRNAs in PH and can be helpful in elucidating the potential clinical applications of ncRNAs.
Author Contributions
Conceptualization, HZ; writing—original draft preparation HZ and QZ; writing—review and editing, HZ and XL. All authors have read and agreed to the published version of the article.
Conflict of Interest
The authors declare that the research was conducted in the absence of any commercial or financial relationships that could be construed as a potential conflict of interest.
Publisher’s Note
All claims expressed in this article are solely those of the authors and do not necessarily represent those of their affiliated organizations, or those of the publisher, the editors and the reviewers. Any product that may be evaluated in this article, or claim that may be made by its manufacturer, is not guaranteed or endorsed by the publisher.
References
Agrawal, R., Pandey, P., Jha, P., Dwivedi, V., Sarkar, C., and Kulshreshtha, R. (2014). Hypoxic Signature of Micrornas in Glioblastoma: Insights from Small Rna Deep Sequencing. BMC Genomics 15, 686. doi:10.1186/1471-2164-15-686
Anastasiou, D., Yu, Y., Israelsen, W. J., Jiang, J.-K., Boxer, M. B., Hong, B. S., et al. (2012). Pyruvate Kinase M2 Activators Promote Tetramer Formation and Suppress Tumorigenesis. Nat. Chem. Biol. 8, 839–847. doi:10.1038/nchembio.1060
Babicheva, A., Ayon, R. J., Zhao, T., Ek Vitorin, J. F., Pohl, N. M., Yamamura, A., et al. (2020). MicroRNA-mediated Downregulation of K+ Channels in Pulmonary Arterial Hypertension. Am. J. Physiology-Lung Cell Mol. Physiol. 318, L10–L26. doi:10.1152/ajplung.00010.2019
Bertero, T., Cottrill, K. A., Lu, Y., Haeger, C. M., Dieffenbach, P., Annis, S., et al. (2015). Matrix Remodeling Promotes Pulmonary Hypertension through Feedback Mechanoactivation of the Yap/taz-Mir-130/301 Circuit. Cel Rep. 13, 1016–1032. doi:10.1016/j.celrep.2015.09.049
Bertero, T., Lu, Y., Annis, S., Hale, A., Bhat, B., Saggar, R., et al. (2014). Systems-level Regulation of Microrna Networks by Mir-130/301 Promotes Pulmonary Hypertension. J. Clin. Invest. 124, 3514–3528. doi:10.1172/jci74773
Bi, R., Bao, C., Jiang, L., Liu, H., Yang, Y., Mei, J., et al. (2015). MicroRNA-27b Plays a Role in Pulmonary Arterial Hypertension by Modulating Peroxisome Proliferator-Activated Receptor γ Dependent Hsp90-eNOS Signaling and Nitric Oxide Production. Biochem. Biophysical Res. Commun. 460, 469–475. doi:10.1016/j.bbrc.2015.03.057
Botti, G., Marra, L., Malzone, M., Anniciello, A., Botti, C., Franco, R., et al. (2016). Lncrna Hotair as Prognostic Circulating Marker and Potential Therapeutic Target in Patients with Tumor Diseases. Cdt 18, 27–34. doi:10.2174/1389450117666151209122950
Bourgeois, A., Lambert, C., Habbout, K., Ranchoux, B., Paquet-Marceau, S., Trinh, I., et al. (2018a). Foxm1 Promotes Pulmonary Artery Smooth Muscle Cell Expansion in Pulmonary Arterial Hypertension. J. Mol. Med. 96, 223–235. doi:10.1007/s00109-017-1619-0
Bourgeois, A., Omura, J., Habbout, K., Bonnet, S., and Boucherat, O. (2018b). Pulmonary Arterial Hypertension: New Pathophysiological Insights and Emerging Therapeutic Targets. Int. J. Biochem. Cel Biol. 104, 9–13. doi:10.1016/j.biocel.2018.08.015
Bracken, C. P., Gregory, P. A., Kolesnikoff, N., Bert, A. G., Wang, J., Shannon, M. F., et al. (2008). A Double-Negative Feedback Loop between Zeb1-Sip1 and the Microrna-200 Family Regulates Epithelial-Mesenchymal Transition. Cancer Res. 68, 7846–7854. doi:10.1158/0008-5472.Can-08-1942
Brock, M., Haider, T. J., Vogel, J., Gassmann, M., Speich, R., Trenkmann, M., et al. (2015). The Hypoxia-Induced Microrna-130a Controls Pulmonary Smooth Muscle Cell Proliferation by Directly Targeting Cdkn1a. Int. J. Biochem. Cel Biol. 61, 129–137. doi:10.1016/j.biocel.2015.02.002
Brock, M., Trenkmann, M., Gay, R. E., Michel, B. A., Gay, S., Fischler, M., et al. (2009). Interleukin-6 Modulates the Expression of the Bone Morphogenic Protein Receptor Type Ii through a Novel Stat3-Microrna Cluster 17/92 Pathway. Circ. Res. 104, 1184–1191. doi:10.1161/circresaha.109.197491
Cai, Z., Li, J., Zhuang, Q., Zhang, X., Yuan, A., Shen, L., et al. (2018). MiR-125a-5p Ameliorates Monocrotaline-Induced Pulmonary Arterial Hypertension by Targeting the TGF-Β1 and IL-6/STAT3 Signaling Pathways. Exp. Mol. Med. 50, 1–11. doi:10.1038/s12276-018-0068-3
Camps, C., Saini, H. K., Mole, D. R., Choudhry, H., Reczko, M., Guerra-Assunção, J., et al. (2014). Integrated Analysis of Microrna and Mrna Expression and Association with Hif Binding Reveals the Complexity of Microrna Expression Regulation under Hypoxia. Mol. Cancer 13, 28. doi:10.1186/1476-4598-13-28
Caruso, P., Dunmore, B. J., Schlosser, K., Schoors, S., Dos Santos, C., Perez-Iratxeta, C., et al. (2017). Identification of Microrna-124 as a Major Regulator of Enhanced Endothelial Cell Glycolysis in Pulmonary Arterial Hypertension via Ptbp1 (Polypyrimidine Tract Binding Protein) and Pyruvate Kinase M2. Circulation 136, 2451–2467. doi:10.1161/circulationaha.117.028034
Chang, T.-C., Yu, D., Lee, Y.-S., Wentzel, E. A., Arking, D. E., West, K. M., et al. (2008). Widespread Microrna Repression by Myc Contributes to Tumorigenesis. Nat. Genet. 40, 43–50. doi:10.1038/ng.2007.30
Chelladurai, P., Seeger, W., and Pullamsetti, S. S. (2016). Epigenetic Mechanisms in Pulmonary Arterial Hypertension: The Need for Global Perspectives. Eur. Respir. Rev. 25, 135–140. doi:10.1183/16000617.0036-2016
Chen, J., Cui, X., Li, L., Qu, J., Raj, J. U., and Gou, D. (2017). Mir-339 Inhibits Proliferation of Pulmonary Artery Smooth Muscle Cell by Targeting Fgf Signaling. Physiol. Rep. 5, e13441. doi:10.14814/phy2.13441
Chen, K.-H., Dasgupta, A., Lin, J., Potus, F., Bonnet, S., Iremonger, J., et al. (2018). Epigenetic Dysregulation of the Dynamin-Related Protein 1 Binding Partners MiD49 and MiD51 Increases Mitotic Mitochondrial Fission and Promotes Pulmonary Arterial Hypertension. Circulation 138, 287–304. doi:10.1161/circulationaha.117.031258
Chen, M., Shen, C., Zhang, Y., and Shu, H. (2017). MicroRNA-150 Attenuates Hypoxia-Induced Excessive Proliferation and Migration of Pulmonary Arterial Smooth Muscle Cells through Reducing HIF-1α Expression. Biomed. Pharmacother. 93, 861–868. doi:10.1016/j.biopha.2017.07.028
Chen, S., Yu, C., Lu, R., Song, T., Wang, X., Tang, W., et al. (2019). Mir-107 Inhibits Pdgf-Bb-Induced Proliferation of Human Pulmonary Arterial Smooth Muscle Cells and Migration through Targeting Nor1. Int. J. Clin. Exp. Pathol. 12, 1599–1608.
Chen, T., Huang, J. B., Dai, J., Zhou, Q., Raj, J. U., and Zhou, G. (2018). Pai-1 Is a Novel Component of the Mir-17∼92 Signaling that Regulates Pulmonary Artery Smooth Muscle Cell Phenotypes. Am. J. Physiology-Lung Cell Mol. Physiol. 315, L149–L161. doi:10.1152/ajplung.00137.2017
Chen, T., Zhou, G., Zhou, Q., Tang, H., Ibe, J. C. F., Cheng, H., et al. (2015). Loss of MicroRNA-17∼92 in Smooth Muscle Cells Attenuates Experimental Pulmonary Hypertension via Induction of PDZ and LIM Domain 5. Am. J. Respir. Crit. Care Med. 191, 678–692. doi:10.1164/rccm.201405-0941OC
Chen, T., Zhou, Q., Tang, H., Bozkanat, M., Yuan, J. X. J., Raj, J. U., et al. (2016). miR‐17/20 Controls Prolyl Hydroxylase 2 (PHD2)/Hypoxia‐Inducible Factor 1 (HIF1) to Regulate Pulmonary Artery Smooth Muscle Cell Proliferation. Jaha 5, e004510. doi:10.1161/jaha.116.004510
Cheng, G., He, L., and Zhang, Y. (2020). Lincrna-cox2 Promotes Pulmonary Arterial Hypertension by Regulating the Let-7a-Mediated Stat3 Signaling Pathway. Mol. Cel Biochem 475, 239–247. doi:10.1007/s11010-020-03877-6
Clozel, M. (2016). Endothelin Research and the Discovery of Macitentan for the Treatment of Pulmonary Arterial Hypertension. Am. J. Physiology-Regulatory, Integr. Comp. Physiol. 311, R721–R726. doi:10.1152/ajpregu.00475.2015
Correia de Sousa, M., Gjorgjieva, M., Dolicka, D., Sobolewski, C., and Foti, M. (2019). Deciphering Mirnas' Action through Mirna Editing. Ijms 20, 6249. doi:10.3390/ijms20246249
Courboulin, A., Paulin, R., Giguère, N. J., Saksouk, N., Perreault, T., Meloche, J., et al. (2011). Role for Mir-204 in Human Pulmonary Arterial Hypertension. J. Exp. Med. 208, 535–548. doi:10.1084/jem.20101812
Davis, B. N., Hilyard, A. C., Nguyen, P. H., Lagna, G., and Hata, A. (2010). Smad Proteins Bind a Conserved Rna Sequence to Promote Microrna Maturation by Drosha. Mol. Cel 39, 373–384. doi:10.1016/j.molcel.2010.07.011
Deng, B., Du, J., Hu, R., Wang, A.-P., Wu, W.-H., Hu, C.-P., et al. (2016). MicroRNA-103/107 Is Involved in Hypoxia-Induced Proliferation of Pulmonary Arterial Smooth Muscle Cells by Targeting HIF-1β. Life Sci. 147, 117–124. doi:10.1016/j.lfs.2016.01.043
Deng, L., Blanco, F. J., Stevens, H., Lu, R., Caudrillier, A., McBride, M., et al. (2015). Microrna-143 Activation Regulates Smooth Muscle and Endothelial Cell Crosstalk in Pulmonary Arterial Hypertension. Circ. Res. 117, 870–883. doi:10.1161/circresaha.115.306806
Di, X., Jin, X., Li, R., Zhao, M., and Wang, K. (2019). Circrnas and Lung Cancer: Biomarkers and Master Regulators. Life Sci. 220, 177–185. doi:10.1016/j.lfs.2019.01.055
el Azzouzi, H., Leptidis, S., Dirkx, E., Hoeks, J., van Bree, B., Brand, K., et al. (2013). The Hypoxia-Inducible MicroRNA Cluster miR-199a∼214 Targets Myocardial PPARδ and Impairs Mitochondrial Fatty Acid Oxidation. Cel Metab. 18, 341–354. doi:10.1016/j.cmet.2013.08.009
Fu, J., Bai, P., Chen, Y., Yu, T., and Li, F. (2019). Inhibition of Mir-495 Improves Both Vascular Remodeling and Angiogenesis in Pulmonary Hypertension. J. Vasc. Res. 56, 97–106. doi:10.1159/000500024
Galiè, N., Humbert, M., Vachiery, J.-L., Gibbs, S., Lang, I., Torbicki, A., et al. (2016). 2015 ESC/ERS Guidelines for the Diagnosis and Treatment of Pulmonary Hypertension. Eur. Heart J. 37, 67–119. doi:10.1093/eurheartj/ehv317
Gamen, E., Seeger, W., and Pullamsetti, S. S. (2016). The Emerging Role of Epigenetics in Pulmonary Hypertension. Eur. Respir. J. 48, 903–917. doi:10.1183/13993003.01714-2015
Gandellini, P., Giannoni, E., Casamichele, A., Taddei, M. L., Callari, M., Piovan, C., et al. (2014). Mir-205 Hinders the Malignant Interplay between Prostate Cancer Cells and Associated Fibroblasts. Antioxid. Redox Signaling 20, 1045–1059. doi:10.1089/ars.2013.5292
Gou, D., Ramchandran, R., Peng, X., Yao, L., Kang, K., Sarkar, J., et al. (2012). Mir-210 Has an Antiapoptotic Effect in Pulmonary Artery Smooth Muscle Cells during Hypoxia. Am. J. Physiology-Lung Cell Mol. Physiol. 303, L682–L691. doi:10.1152/ajplung.00344.2011
Green, D. E., Murphy, T. C., Kang, B.-Y., Bedi, B., Yuan, Z., Sadikot, R. T., et al. (2017). Peroxisome Proliferator-Activated Receptor-γ Enhances Human Pulmonary Artery Smooth Muscle Cell Apoptosis through microRNA-21 and Programmed Cell Death 4. Am. J. Physiology-Lung Cell Mol. Physiol. 313, L371–L383. doi:10.1152/ajplung.00532.2016
Green, D. E., Murphy, T. C., Kang, B.-Y., Searles, C. D., and Hart, C. M. (2015). PPARγ Ligands Attenuate Hypoxia-Induced Proliferation in Human Pulmonary Artery Smooth Muscle Cells through Modulation of MicroRNA-21. PLoS One 10, e0133391. doi:10.1371/journal.pone.0133391
Guo, L., Qiu, Z., Wei, L., Yu, X., Gao, X., Jiang, S., et al. (2012). The MicroRNA-328 Regulates Hypoxic Pulmonary Hypertension by Targeting at Insulin Growth Factor 1 Receptor and L-type Calcium Channel-Α1c. Hypertension 59, 1006–1013. doi:10.1161/hypertensionaha.111.185413
Guo, L., Yang, Y., Liu, J., Wang, L., Li, J., Wang, Y., et al. (2014). Differentially Expressed Plasma Micrornas and the Potential Regulatory Function of Let-7b in Chronic Thromboembolic Pulmonary Hypertension. PLoS One 9, e101055. doi:10.1371/journal.pone.0101055
Han, Y., Liu, Y., Yang, C., Gao, C., Guo, X., and Cheng, J. (2020). Lncrna Casc2 Inhibits Hypoxia-Induced Pulmonary Artery Smooth Muscle Cell Proliferation and Migration by Regulating the Mir-222/ing5 axis. Cell Mol Biol Lett 25, 21. doi:10.1186/s11658-020-00215-y
Hao, X., Ma, C., Chen, S., Dang, J., Cheng, X., and Zhu, D. (2018). Reverse the Down Regulation of Mir-92b-3p by Hypoxia Can Suppress the Proliferation of Pulmonary Artery Smooth Muscle Cells by Targeting Usp28. Biochem. Biophysical Res. Commun. 503, 3064–3077. doi:10.1016/j.bbrc.2018.08.095
Hong, Z., Chen, K.-H., DasGupta, A., Potus, F., Dunham-Snary, K., Bonnet, S., et al. (2017). Microrna-138 and Microrna-25 Down-Regulate Mitochondrial Calcium Uniporter, Causing the Pulmonary Arterial Hypertension Cancer Phenotype. Am. J. Respir. Crit. Care Med. 195, 515–529. doi:10.1164/rccm.201604-0814OC
Huang, J., Zhou, N., Watabe, K., Lu, Z., Wu, F., Xu, M., et al. (2014). Long Non-coding Rna uca1 Promotes Breast Tumor Growth by Suppression of P27 (Kip1). Cell Death Dis 5–e1008. e1008. doi:10.1038/cddis.2013.541
Huber, L. C., Ulrich, S., Leuenberger, C., Gassmann, M., Vogel, J., von Blotzheim, L. G., et al. (2015). Featured Article: Microrna-125a in Pulmonary Hypertension: Regulator of a Proliferative Phenotype of Endothelial Cells. Exp. Biol. Med. (Maywood) 240, 1580–1589. doi:10.1177/1535370215579018
Iannone, L., Zhao, L., Dubois, O., Duluc, L., Rhodes, C. J., Wharton, J., et al. (2014). Mir-21/ddah1 Pathway Regulates Pulmonary Vascular Responses to Hypoxia. Biochem. J. 462, 103–112. doi:10.1042/bj20140486
Inoue, K., and Fry, E. A. (2015). Aberrant Expression of Cyclin D1 in Cancer. Signal. Transduction Insights 4, STI.S30306–13. doi:10.4137/sti.S30306
Jalali, S., Ramanathan, G. K., Parthasarathy, P. T., Aljubran, S., Galam, L., Yunus, A., et al. (2012). Mir-206 Regulates Pulmonary Artery Smooth Muscle Cell Proliferation and Differentiation. PLoS One 7, e46808. doi:10.1371/journal.pone.0046808
Jandl, K., Thekkekara Puthenparampil, H., Marsh, L. M., Hoffmann, J., Wilhelm, J., Veith, C., et al. (2019). Long Non‐coding RNAs Influence the Transcriptome in Pulmonary Arterial Hypertension: the Role ofPAXIP1‐AS1. J. Pathol. 247, 357–370. doi:10.1002/path.5195
Ji, P., Diederichs, S., Wang, W., Böing, S., Metzger, R., Schneider, P. M., et al. (2003). MALAT-1, a Novel Noncoding RNA, and Thymosin β4 Predict Metastasis and Survival in Early-Stage Non-small Cell Lung Cancer. Oncogene 22, 8031–8041. doi:10.1038/sj.onc.1206928
Jiang, J., Xia, Y., Liang, Y., Yang, M., Zeng, W., and Zeng, X. (2018). Mir-190a-5p Participates in the Regulation of Hypoxia-Induced Pulmonary Hypertension by Targeting Klf15 and Can Serve as a Biomarker of Diagnosis and Prognosis in Chronic Obstructive Pulmonary Disease Complicated with Pulmonary Hypertension. Copd Vol. 13, 3777–3790. doi:10.2147/copd.S182504
Jin, Y., Pang, T., Nelin, L. D., Wang, W., Wang, Y., Yan, J., et al. (2015). Mkp-1 Is a Target of Mir-210 and Mediate the Negative Regulation of Mir-210 Inhibitor on Hypoxic Hpasmc Proliferation. Cell Biol Int 39, 113–120. doi:10.1002/cbin.10339
Kang, B.-Y., Park, K. K., Green, D. E., Bijli, K. M., Searles, C. D., Sutliff, R. L., et al. (2013). Hypoxia Mediates Mutual Repression between microRNA-27a and PPARγ in the Pulmonary Vasculature. PLoS One 8, e79503. doi:10.1371/journal.pone.0079503
Kang, B.-Y., Park, K. K., Kleinhenz, J. M., Murphy, T. C., Green, D. E., Bijli, K. M., et al. (2016). Peroxisome Proliferator-Activated Receptor γ and microRNA 98 in Hypoxia-Induced Endothelin-1 Signaling. Am. J. Respir. Cel Mol Biol 54, 136–146. doi:10.1165/rcmb.2014-0337OC
Kang, K., Peng, X., Zhang, X., Wang, Y., Zhang, L., Gao, L., et al. (2013). Microrna-124 Suppresses the Transactivation of Nuclear Factor of Activated T Cells by Targeting Multiple Genes and Inhibits the Proliferation of Pulmonary Artery Smooth Muscle Cells. J. Biol. Chem. 288, 25414–25427. doi:10.1074/jbc.M113.460287
Kim, J., Kang, Y., Kojima, Y., Lighthouse, J. K., Hu, X., Aldred, M. A., et al. (2013). An Endothelial Apelin-Fgf Link Mediated by Mir-424 and Mir-503 Is Disrupted in Pulmonary Arterial Hypertension. Nat. Med. 19, 74–82. doi:10.1038/nm.3040
Lee, J., and Kang, H. (2019). Hypoxia Promotes Vascular Smooth Muscle Cell Proliferation through Microrna-Mediated Suppression of Cyclin-dependent Kinase Inhibitors. Cells 8, 802. doi:10.3390/cells8080802
Lei, S., Peng, F., Li, M.-L., Duan, W.-B., Peng, C.-Q., and Wu, S.-J. (2020). Lncrna-smilr Modulates Rhoa/rock Signaling by Targeting Mir-141 to Regulate Vascular Remodeling in Pulmonary Arterial Hypertension. Am. J. Physiology-Heart Circulatory Physiol. 319, H377–H391. doi:10.1152/ajpheart.00717
Leisegang, M. S., Fork, C., Josipovic, I., Richter, F. M., Preussner, J., Hu, J., et al. (2017). Long Noncoding Rna Mantis Facilitates Endothelial Angiogenic Function. Circulation 136, 65–79. doi:10.1161/circulationaha.116.02699110.1152/ajpheart.00717.2019
Li, L., Kim, I.-K., Chiasson, V., Chatterjee, P., and Gupta, S. (2017). NF-κB Mediated miR-130a Modulation in Lung Microvascular Cell Remodeling: Implication in Pulmonary Hypertension. Exp. Cel Res. 359, 235–242. doi:10.1016/j.yexcr.2017.07.024
Li, Q., Qian, Z., and Wang, L. (2017). Pri-microrna-124 Rs531564 Polymorphism Minor Allele Increases the Risk of Pulmonary Artery Hypertension by Abnormally Enhancing Proliferation of Pulmonary Artery Smooth Muscle Cells. Copd 12, 1351–1361. doi:10.2147/copd.S99318
Li, Q., Zhou, X., and Zhou, X. (2019). Downregulation of miR-98 C-ontributes to H-ypoxic P-ulmonary H-ypertension by T-argeting ALK1. Mol. Med. Rep. 20, 2167–2176. doi:10.3892/mmr.2019.10482
Li, S.-S., Ran, Y.-J., Zhang, D.-D., Li, S.-Z., and Zhu, D. (2014). MicroRNA-190 Regulates Hypoxic Pulmonary Vasoconstriction by Targeting a Voltage-Gated K+Channel in Arterial Smooth Muscle Cells. J. Cel. Biochem. 115, 1196–1205. doi:10.1002/jcb.24771
Li, S., Ran, Y., Zhang, D., Chen, J., Li, S., and Zhu, D. (2013). Microrna-138 Plays a Role in Hypoxic Pulmonary Vascular Remodelling by Targeting Mst1. Biochem. J. 452, 281–291. doi:10.1042/bj20120680
Li, Y., Li, L., Qian, Z., Lin, B., Chen, J., Luo, Y., et al. (2018). Phosphatidylinositol 3‐Kinase-DNA Methyltransferase 1-miR‐1281-Histone Deacetylase 4 Regulatory Axis Mediates Platelet‐Derived Growth Factor-Induced Proliferation and Migration of Pulmonary Artery Smooth Muscle Cells. Jaha 7, e007572. doi:10.1161/jaha.117.007572
Li, Y., Ren, W., Wang, X., Yu, X., Cui, L., Li, X., et al. (2019). Microrna-150 Relieves Vascular Remodeling and Fibrosis in Hypoxia-Induced Pulmonary Hypertension. Biomed. Pharmacother. 109, 1740–1749. doi:10.1016/j.biopha.2018.11.058
Lin, Z., Murtaza, I., Wang, K., Jiao, J., Gao, J., and Li, P.-F. (2009). Mir-23a Functions Downstream of Nfatc3 to Regulate Cardiac Hypertrophy. Proc. Natl. Acad. Sci. 106, 12103–12108. doi:10.1073/pnas.0811371106
Liu, A., Liu, Y., Li, B., Yang, M., Liu, Y., and Su, J. (2019). Role of miR‐223‐3p in Pulmonary Arterial Hypertension via Targeting ITGB3 in the ECM Pathway. Cell Prolif 52, e12550. doi:10.1111/cpr.12550
Liu, G., Hao, P., Xu, J., Wang, L., Wang, Y., Han, R., et al. (2018). Upregulation of Microrna-17-5p Contributes to Hypoxia-Induced Proliferation in Human Pulmonary Artery Smooth Muscle Cells through Modulation of P21 and Pten. Respir. Res. 19, 200. doi:10.1186/s12931-018-0902-0
Liu, H.-M., Jia, Y., Zhang, Y.-x., Yan, J., Liao, N., Li, X.-h., et al. (2019). Dysregulation of Mir-135a-5p Promotes the Development of Rat Pulmonary Arterial Hypertension In Vivo and In Vitro. Acta Pharmacol. Sin 40, 477–485. doi:10.1038/s41401-018-0076-9
Liu, H., Tao, Y., Chen, M., Yu, J., Li, W.-J., Tao, L., et al. (2016). Upregulation of Microrna-214 Contributes to the Development of Vascular Remodeling in Hypoxia-Induced Pulmonary Hypertension via Targeting Ccnl2. Sci. Rep. 6, 24661. doi:10.1038/srep24661
Liu, H., Yin, T., Yan, W., Si, R., Wang, B., Chen, M., et al. (2017). Dysregulation of Microrna-214 and Pten Contributes to the Pathogenesis of Hypoxic Pulmonary Hypertension. Copd Vol. 12, 1781–1791. doi:10.2147/copd.S104627
Liu, J. J., Zhang, H., Xing, F., Tang, B., Wu, S. L., Xuan, L., et al. (2018). MicroRNA-138 P-romotes P-roliferation and S-uppresses M-itochondrial D-epolarization in H-uman P-ulmonary A-rtery S-mooth M-uscle C-ells through T-argeting TASK-1. Mol. Med. Rep. 17, 3021–3027. doi:10.3892/mmr.2017.8200
Liu, S.-C., Chuang, S.-M., Hsu, C.-J., Tsai, C.-H., Wang, S.-W., and Tang, C.-H. (2014). Ctgf Increases Vascular Endothelial Growth Factor-dependent Angiogenesis in Human Synovial Fibroblasts by Increasing Mir-210 Expression. Cel Death Dis 5, e1485. doi:10.1038/cddis.2014.453
Liu, T., Zou, X.-Z., Huang, N., Ge, X.-Y., Yao, M.-Z., Liu, H., et al. (2019a). Down-regulation of Mir-204 Attenuates Endothelial-Mesenchymal Transition by Enhancing Autophagy in Hypoxia-Induced Pulmonary Hypertension. Eur. J. Pharmacol. 863, 172673. doi:10.1016/j.ejphar.2019.172673
Liu, T., Zou, X.-Z., Huang, N., Ge, X.-Y., Yao, M.-Z., Liu, H., et al. (2019b). Mir-27a Promotes Endothelial-Mesenchymal Transition in Hypoxia-Induced Pulmonary Arterial Hypertension by Suppressing Bmp Signaling. Life Sci. 227, 64–73. doi:10.1016/j.lfs.2019.04.038
Liu, Y., Liu, G., Zhang, H., and Wang, J. (2016). Mirna-199a-5p Influences Pulmonary Artery Hypertension via Downregulating Smad3. Biochem. Biophysical Res. Commun. 473, 859–866. doi:10.1016/j.bbrc.2016.03.140
Lu, Z., Li, S., Zhao, S., and Fa, X. (2016). Upregulated Mir-17 Regulates Hypoxia-Mediated Human Pulmonary Artery Smooth Muscle Cell Proliferation and Apoptosis by Targeting Mitofusin 2. Med. Sci. Monit. 22, 3301–3308. doi:10.12659/msm.900487
Ma, C., Zhang, C., Ma, M., Zhang, L., Zhang, L., Zhang, F., et al. (2017). Mir-125a Regulates Mitochondrial Homeostasis through Targeting Mitofusin 1 to Control Hypoxic Pulmonary Vascular Remodeling. J. Mol. Med. 95, 977–993. doi:10.1007/s00109-017-1541-5
Matsushime, H., Roussel, M. F., Ashmun, R. A., and Sherr, C. J. (1991). Colony-stimulating Factor 1 Regulates Novel Cyclins during the G1 Phase of the Cell Cycle. Cell 65, 701–713. doi:10.1016/0092-8674(91)90101-4
Meloche, J., Le Guen, M., Potus, F., Vinck, J., Ranchoux, B., Johnson, I., et al. (2015b). Mir-223 Reverses Experimental Pulmonary Arterial Hypertension. Am. J. Physiology-Cell Physiol. 309, C363–C372. doi:10.1152/ajpcell.00149.2015
Meloche, J., Pflieger, A., Vaillancourt, M., Paulin, R., Potus, F., Zervopoulos, S., et al. (2014). Role for DNA Damage Signaling in Pulmonary Arterial Hypertension. Circulation 129, 786–797. doi:10.1161/circulationaha.113.006167
Meloche, J., Potus, F., Vaillancourt, M., Bourgeois, A., Johnson, I., Deschamps, L., et al. (2015a). Bromodomain-Containing Protein 4. Circ. Res. 117, 525–535. doi:10.1161/circresaha.115.307004
Miao, R., Wang, Y., Wan, J., Leng, D., Gong, J., Li, J., et al. (2017). Microarray Expression Profile of Circular Rnas in Chronic Thromboembolic Pulmonary Hypertension. Medicine (Baltimore) 96, e7354. doi:10.1097/md.0000000000007354
Nie, X., Chen, Y., Tan, J., Dai, Y., Mao, W., Qin, G., et al. (2019). Microrna-221-3p Promotes Pulmonary Artery Smooth Muscle Cells Proliferation by Targeting Axin2 during Pulmonary Arterial Hypertension. Vasc. Pharmacol. 116, 24–35. doi:10.1016/j.vph.2017.07.002
Orriols, M., Gomez-Puerto, M. C., and Ten Dijke, P. (2017). Bmp Type Ii Receptor as a Therapeutic Target in Pulmonary Arterial Hypertension. Cell. Mol. Life Sci. 74, 2979–2995. doi:10.1007/s00018-017-2510-4
Parikh, V. N., Jin, R. C., Rabello, S., Gulbahce, N., White, K., Hale, A., et al. (2012). MicroRNA-21 Integrates Pathogenic Signaling to Control Pulmonary Hypertension. Circulation 125, 1520–1532. doi:10.1161/circulationaha.111.060269
Qian, Z., Li, Y., Chen, J., Li, X., and Gou, D. (2017). Mir-4632 Mediates Pdgf-Bb-Induced Proliferation and Antiapoptosis of Human Pulmonary Artery Smooth Muscle Cells via Targeting Cjun. Am. J. Physiology-Cell Physiol. 313, C380–C391. doi:10.1152/ajpcell.00061.2017
Qian, Z., Li, Y., Yang, H., Chen, J., Li, X., and Gou, D. (2018). Pdgfbb Promotes Proliferation and Migration via Regulating Mir-1181/stat3 axis in Human Pulmonary Arterial Smooth Muscle Cells. Am. J. Physiology-Lung Cell Mol. Physiol. 315, L965–L976. doi:10.1152/ajplung.00224.2018
Qian, Z., Zhang, L., Chen, J., Li, Y., Kang, K., Qu, J., et al. (2016). Mir-328 Targeting Pim-1 Inhibits Proliferation and Migration of Pulmonary Arterial Smooth Muscle Cells in Pdgfbb Signaling Pathway. Oncotarget 7, 54998–55011. doi:10.18632/oncotarget.10714
Rokavec, M., Öner, M. G., Li, H., Jackstadt, R., Jiang, L., Lodygin, D., et al. (2014). Il-6r/stat3/mir-34a Feedback Loop Promotes Emt-Mediated Colorectal Cancer Invasion and Metastasis. J. Clin. Invest. 124, 1853–1867. doi:10.1172/jci73531
Rothman, A. M. K., Arnold, N. D., Pickworth, J. A., Iremonger, J., Ciuclan, L., Allen, R. M. H., et al. (2016). Microrna-140-5p and Smurf1 Regulate Pulmonary Arterial Hypertension. J. Clin. Invest. 126, 2495–2508. doi:10.1172/jci83361
Ruffenach, G., Chabot, S., Tanguay, V. F., Courboulin, A., Boucherat, O., Potus, F., et al. (2016). Role for Runt-Related Transcription Factor 2 in Proliferative and Calcified Vascular Lesions in Pulmonary Arterial Hypertension. Am. J. Respir. Crit. Care Med. 194, 1273–1285. doi:10.1164/rccm.201512-2380OC
Sahoo, S., Meijles, D. N., Al Ghouleh, I., Tandon, M., Cifuentes-Pagano, E., Sembrat, J., et al. (2016). Mef2c-myocd and Leiomodin1 Suppression by Mirna-214 Promotes Smooth Muscle Cell Phenotype Switching in Pulmonary Arterial Hypertension. PLoS One 11, e0153780. doi:10.1371/journal.pone.0153780
Sang, H.-y., Jin, Y.-l., Zhang, W.-q., and Chen, L.-b. (2016). Downregulation of Microrna-637 Increases Risk of Hypoxia-Induced Pulmonary Hypertension by Modulating Expression of Cyclin Dependent Kinase 6 (Cdk6) in Pulmonary Smooth Muscle Cells. Med. Sci. Monit. 22, 4066–4072. doi:10.12659/msm.897254
Schulte, J. H., Horn, S., Otto, T., Samans, B., Heukamp, L. C., Eilers, U.-C., et al. (2008). Mycn Regulates Oncogenic Micrornas in Neuroblastoma. Int. J. Cancer 122, 699–704. doi:10.1002/ijc.23153
Sharma, S., Umar, S., Potus, F., Iorga, A., Wong, G., Meriwether, D., et al. (2014). Apolipoprotein A-I Mimetic Peptide 4f Rescues Pulmonary Hypertension by Inducing Microrna-193-3p. Circulation 130, 776–785. doi:10.1161/circulationaha.114.007405
Song, Z., Ren, H., Gao, S., Zhao, X., Zhang, H., and Hao, J. (2014). The Clinical Significance and Regulation Mechanism of Hypoxia-Inducible Factor-1 and Mir-191 Expression in Pancreatic Cancer. Tumor Biol. 35, 11319–11328. doi:10.1007/s13277-014-2452-5
Su, H., Xu, X., Yan, C., Shi, Y., Hu, Y., Dong, L., et al. (2018). LncRNA H19 Promotes the Proliferation of Pulmonary Artery Smooth Muscle Cells through AT1R via Sponging Let-7b in Monocrotaline-Induced Pulmonary Arterial Hypertension. Respir. Res. 19, 254. doi:10.1186/s12931-018-0956-z
Sun, L., Lin, P., Chen, Y., Yu, H., Ren, S., Wang, J., et al. (2020). Mir-182-3p/myadm Contribute to Pulmonary Artery Hypertension Vascular Remodeling via a Klf4/p21-dependent Mechanism. Theranostics 10, 5581–5599. doi:10.7150/thno.44687
Sysol, J. R., Chen, J., Singla, S., Zhao, S., Comhair, S., Natarajan, V., et al. (2018). Micro-rna-1 Is Decreased by Hypoxia and Contributes to the Development of Pulmonary Vascular Remodeling via Regulation of Sphingosine Kinase 1. Am. J. Physiology-Lung Cell Mol. Physiol. 314, L461–L472. doi:10.1152/ajplung.00057.2017
Takagi, K., Yamakuchi, M., Matsuyama, T., Kondo, K., Uchida, A., Misono, S., et al. (2018). Il-13 Enhances Mesenchymal Transition of Pulmonary Artery Endothelial Cells via Down-Regulation of Mir-424/503 In Vitro. Cell Signal. 42, 270–280. doi:10.1016/j.cellsig.2017.10.019
Tan, H., Yao, H., Lie, Z., Chen, G., Lin, S., and Zhang, Y. (2019). MicroRNA-30a-5p P-romotes P-roliferation and I-nhibits A-poptosis of H-uman P-ulmonary A-rtery E-ndothelial C-ells under H-ypoxia by T-argeting YKL-40. Mol. Med. Rep. 20, 236–244. doi:10.3892/mmr.2019.10251
Tao, W., Sun, W., Zhu, H., and Zhang, J. (2019). Mir-205-5p Suppresses Pulmonary Vascular Smooth Muscle Cell Proliferation by Targeting Mical2-Mediated Erk1/2 Signaling. Microvasc. Res. 124, 43–50. doi:10.1016/j.mvr.2019.03.001
Toyoshima, H., and Hunter, T. (1994). P27, a Novel Inhibitor of G1 Cyclin-Cdk Protein Kinase Activity, Is Related to P21. Cell 78, 67–74. doi:10.1016/0092-8674(94)90573-8
Tu, L., Dewachter, L., Gore, B., Fadel, E., Dartevelle, P., Simonneau, G., et al. (2011). Autocrine Fibroblast Growth Factor-2 Signaling Contributes to Altered Endothelial Phenotype in Pulmonary Hypertension. Am. J. Respir. Cel Mol Biol 45, 311–322. doi:10.1165/rcmb.2010-0317OC
Veith, C., Schermuly, R. T., Brandes, R. P., and Weissmann, N. (2016). Molecular Mechanisms of Hypoxia-Inducible Factor-Induced Pulmonary Arterial Smooth Muscle Cell Alterations in Pulmonary Hypertension. J. Physiol. 594, 1167–1177. doi:10.1113/jp270689
Wakiyama, M., and Yokoyama, S. (2014). Microrna-mediated Deadenylation in a Mammalian Cell-free System. Methods Mol. Biol. 1125, 341–351. doi:10.1007/978-1-62703-971-0_27
Wallace, E., Morrell, N. W., Yang, X. D., Long, L., Stevens, H., Nilsen, M., et al. (2015). A Sex-specific Microrna-96/5-Hydroxytryptamine 1b axis Influences Development of Pulmonary Hypertension. Am. J. Respir. Crit. Care Med. 191, 1432–1442. doi:10.1164/rccm.201412-2148OC
Wang, A.-p., Li, X.-h., Gong, S.-x., Li, W.-q., Hu, C.-p., Zhang, Z., et al. (2015). Mir-100 Suppresses Mtor Signaling in Hypoxia-Induced Pulmonary Hypertension in Rats. Eur. J. Pharmacol. 765, 565–573. doi:10.1016/j.ejphar.2015.09.031
Wang, D., Xu, H., Wu, B., Jiang, S., Pan, H., Wang, R., et al. (2019). Long Non-coding RNA MALAT1 S-ponges miR-124-3p.1/KLF5 to P-romote P-ulmonary V-ascular R-emodeling and C-ell C-ycle P-rogression of P-ulmonary A-rtery H-ypertension. Int. J. Mol. Med. 44, 871–884. doi:10.3892/ijmm.2019.4256
Wang, D., Zhang, H., Li, M., Frid, M. G., Flockton, A. R., McKeon, B. A., et al. (2014). Microrna-124 Controls the Proliferative, Migratory, and Inflammatory Phenotype of Pulmonary Vascular Fibroblasts. Circ. Res. 114, 67–78. doi:10.1161/circresaha.114.301633
Wang, H., Qin, R., and Cheng, Y. (2020). Lncrna-ang362 Promotes Pulmonary Arterial Hypertension by Regulating Mir-221 and Mir-222. Shock 53, 723–729. doi:10.1097/shk.0000000000001410
Wang, L. N., Yu, W. C., Du, C. H., Tong, L., and Cheng, Z. Z. (2018). Hypoxia Is Involved in Hypoxic Pulmonary Hypertension through Inhibiting the Activation of Fgf2 by Mir-203. Eur. Rev. Med. Pharmacol. Sci. 22, 8866–8876. doi:10.26355/eurrev_201812_16655
Wang, P., Xu, J., Hou, Z., Wang, F., Song, Y., Wang, J., et al. (2016). Mirna-34a Promotes Proliferation of Human Pulmonary Artery Smooth Muscle Cells by Targeting Pdgfra. Cell Prolif. 49, 484–493. doi:10.1111/cpr.12265
Wang, R., Ding, X., Zhou, S., Li, M., Sun, L., Xu, X., et al. (2016). Microrna-26b Attenuates Monocrotaline-Induced Pulmonary Vascular Remodeling via Targeting Connective Tissue Growth Factor (Ctgf) and Cyclin D1 (Ccnd1). Oncotarget 7, 72746–72757. doi:10.18632/oncotarget.10125
Wang, S., Cao, W., Gao, S., Nie, X., Zheng, X., Xing, Y., et al. (2019). Tug1 Regulates Pulmonary Arterial Smooth Muscle Cell Proliferation in Pulmonary Arterial Hypertension. Can. J. Cardiol. 35, 1534–1545. doi:10.1016/j.cjca.2019.07.630
Weber, L., Rickli, H., Joerg, L., Weilenmann, D., Brenner, R., Taramasso, M., et al. (2018). Haemodynamic Mechanisms and Long-Term Prognostic Impact of Pulmonary Hypertension in Patients with Severe Aortic Stenosis Undergoing Valve Replacement. Eur. J. Heart Fail. 21, 172–181. doi:10.1002/ejhf.1322
White, K., Lu, Y., Annis, S., Hale, A. E., Chau, B. N., Dahlman, J. E., et al. (2015). Genetic and Hypoxic Alterations of the Micro RNA ‐210‐ ISCU 1/2 axis Promote Iron-Sulfur Deficiency and Pulmonary Hypertension. EMBO Mol. Med. 7, 695–713. doi:10.15252/emmm.201404511
Wu, D., Talbot, C. C., Liu, Q., Jing, Z.-C., Damico, R. L., Tuder, R., et al. (2016). Identifying microRNAs Targeting Wnt/β-Catenin Pathway in End-Stage Idiopathic Pulmonary Arterial Hypertension. J. Mol. Med. 94, 875–885. doi:10.1007/s00109-016-1426-z
Xie, X., Li, S., Zhu, Y., Liu, L., Pan, Y., Wang, J., et al. (2017). MicroRNA-27a/b Mediates Endothelin-1-Induced PPARγ Reduction and Proliferation of Pulmonary Artery Smooth Muscle Cells. Cell Tissue Res 369, 527–539. doi:10.1007/s00441-017-2625-9
Xing, X.-Q., Li, B., Xu, S.-L., Liu, J., Zhang, C.-F., and Yang, J. (2019). Microrna-214-3p Regulates Hypoxia-Mediated Pulmonary Artery Smooth Muscle Cell Proliferation and Migration by Targeting Arhgef12. Med. Sci. Monit. 25, 5738–5746. doi:10.12659/msm.915709
Xing, Y., Zheng, X., Fu, Y., Qi, J., Li, M., Ma, M., et al. (2019). Long Noncoding Rna-Maternally Expressed Gene 3 Contributes to Hypoxic Pulmonary Hypertension. Mol. Ther. 27, 2166–2181. doi:10.1016/j.ymthe.2019.07.022
Xing, Y., Zheng, X., Li, G., Liao, L., Cao, W., Xing, H., et al. (2015). MicroRNA-30c Contributes to the Development of Hypoxia Pulmonary Hypertension by Inhibiting Platelet-Derived Growth Factor Receptor β Expression. Int. J. Biochem. Cel Biol. 64, 155–166. doi:10.1016/j.biocel.2015.04.001
Xu, G., Chen, J., Jing, G., and Shalev, A. (2013). Thioredoxin-interacting Protein Regulates Insulin Transcription through Microrna-204. Nat. Med. 19, 1141–1146. doi:10.1038/nm.3287
Xu, Y., Bei, Y., Shen, S., Zhang, J., Lu, Y., Xiao, J., et al. (2017). Microrna-222 Promotes the Proliferation of Pulmonary Arterial Smooth Muscle Cells by Targeting P27 and Timp3. Cell Physiol Biochem 43, 282–292. doi:10.1159/000480371
Yang, Y. Z., Zhang, Y. F., Yang, L., Xu, J., Mo, X. M., and Peng, W. (2018). miR-760 M-ediates H-ypoxia-I-nduced P-roliferation and A-poptosis of H-uman P-ulmonary A-rtery S-mooth M-uscle C-ells via T-argeting TLR4. Int. J. Mol. Med. 42, 2437–2446. doi:10.3892/ijmm.2018.3862
Yu, H., Xu, M., Dong, Y., Liu, J., Li, Y., Mao, W., et al. (2018). 1,25(OH) 2 D 3 Attenuates Pulmonary Arterial Hypertension via microRNA-204 Mediated Tgfbr2/Smad Signaling. Exp. Cel Res. 362, 311–323. doi:10.1016/j.yexcr.2017.11.032
Yu, Z., Wang, C., Wang, M., Li, Z., Casimiro, M. C., Liu, M., et al. (2008). A Cyclin D1/microrna 17/20 Regulatory Feedback Loop in Control of Breast Cancer Cell Proliferation. J. Cel Biol 182, 509–517. doi:10.1083/jcb.200801079
Yuan, C., Xu, M., Rong, R., Mei, Y., Cai, W., Li, L., et al. (2017). Mir-200c Regulates Endothelin-1 Induced Pasmcs Abnormal Proliferation and Apoptosis. IUBMB Life 69, 877–886. doi:10.1002/iub.1686
Yue, J., Guan, J., Wang, X., Zhang, L., Yang, Z., Ao, Q., et al. (2013). MicroRNA-206 Is Involved in Hypoxia-Induced Pulmonary Hypertension through Targeting of the HIF-1α/Fhl-1 Pathway. Lab. Invest. 93, 748–759. doi:10.1038/labinvest.2013.63
Yue, Y., Zhang, Z., Zhang, L., Chen, S., Guo, Y., and Hong, Y. (2018). Mir-143 and Mir-145 Promote Hypoxia-Induced Proliferation and Migration of Pulmonary Arterial Smooth Muscle Cells through Regulating Abca1 Expression. Cardiovasc. Pathol. 37, 15–25. doi:10.1016/j.carpath.2018.08.003
Zehendner, C. M., Valasarajan, C., Werner, A., Boeckel, J.-N., Bischoff, F. C., John, D., et al. (2020). Long Noncoding RNA TYKRIL Plays a Role in Pulmonary Hypertension via the P53-Mediated Regulation of PDGFRβ. Am. J. Respir. Crit. Care Med. 202, 1445–1457. doi:10.1164/rccm.201910-2041OC
Zeng, Y., Zhang, X., Kang, K., Chen, J., Wu, Z., Huang, J., et al. (2016). Microrna-223 Attenuates Hypoxia-Induced Vascular Remodeling by Targeting Rhob/mlc2 in Pulmonary Arterial Smooth Muscle Cells. Sci. Rep. 6, 24900. doi:10.1038/srep24900
Zeng, Z. H., Wu, W. H., Peng, Q., Sun, Y. H., and Liu, J. X. (2019). MicroRNA-132 M-ediates P-roliferation and M-igration of P-ulmonary S-mooth M-uscle C-ells via T-argeting PTEN. Mol. Med. Rep. 19, 3823–3830. doi:10.3892/mmr.2019.10053
Zeng, Z., Yao, J., Li, Y., Xue, Y., Zou, Y., Shu, Z., et al. (2018). Anti‐apoptosis Endothelial Cell‐secreted microRNA‐195‐5p Promotes Pulmonary Arterial Smooth Muscle Cell Proliferation and Migration in Pulmonary Arterial Hypertension. J. Cel. Biochem. 119, 2144–2155. doi:10.1002/jcb.26376
Zhang, C., Ma, C., Zhang, L., Zhang, L., Zhang, F., Ma, M., et al. (2019a). Mir-449a-5p Mediates Mitochondrial Dysfunction and Phenotypic Transition by Targeting Myc in Pulmonary Arterial Smooth Muscle Cells. J. Mol. Med. 97, 409–422. doi:10.1007/s00109-019-01751-7
Zhang, C., Wang, P., Mohammed, A., Zhou, Z., Zhang, S., Ni, S., et al. (2019b). Function of Adipose-Derived Mesenchymal Stem Cells in Monocrotaline-Induced Pulmonary Arterial Hypertension through Mir-191 via Regulation of Bmpr2. Biomed. Res. Int. 2019, 1–12. doi:10.1155/2019/2858750
Zhang, H., Liu, Y., Yan, L., Wang, S., Zhang, M., Ma, C., et al. (2019). Long Noncoding Rna Hoxaas3 Contributes to Hypoxia-Induced Pulmonary Artery Smooth Muscle Cell Proliferation. Cardiovasc. Res. 115, 647–657. doi:10.1093/cvr/cvy250
Zhang, H., Wang, D., Li, M., Plecitá-Hlavatá, L., D’Alessandro, A., Tauber, J., et al. (2017). Metabolic and Proliferative State of Vascular Adventitial Fibroblasts in Pulmonary Hypertension Is Regulated through a Microrna-124/ptbp1 (Polypyrimidine Tract Binding Protein 1)/pyruvate Kinase Muscle axis. Circulation 136, 2468–2485. doi:10.1161/circulationaha.117.028069
Zhang, R., Su, H., Ma, X., Xu, X., Liang, L., Ma, G., et al. (2019). Mirna Let-7b Promotes the Development of Hypoxic Pulmonary Hypertension by Targeting Ace2. Am. J. Physiology-Lung Cell Mol. Physiol. 316, L547–L557. doi:10.1152/ajplung.00387.2018
Zhang, W.-F., Xiong, Y.-W., Zhu, T.-T., Xiong, A.-Z., Bao, H.-h., and Cheng, X.-S. (2017). MicroRNA Let-7g Inhibited Hypoxia-Induced Proliferation of PASMCs via G0/G1 Cell Cycle Arrest by Targeting C-Myc. Life Sci. 170, 9–15. doi:10.1016/j.lfs.2016.11.020
Zhang, W., Li, Y., Xi, X., Zhu, G., Wang, S., Liu, Y., et al. (2020). MicroRNA-15a-5p I-nduces P-ulmonary A-rtery S-mooth M-uscle C-ell A-poptosis in a P-ulmonary A-rterial H-ypertension M-odel via the VEGF/p38/MMP-2 S-ignaling P-athway. Int. J. Mol. Med. 45, 461–474. doi:10.3892/ijmm.2019.4434
Zhang, X., Shao, R., Gao, W., Sun, G., Liu, Y., and Fa, X. e. (2018). Inhibition of Mir-361-5p Suppressed Pulmonary Artery Smooth Muscle Cell Survival and Migration by Targeting Abca1 and Inhibiting the Jak2/stat3 Pathway. Exp. Cel Res. 363, 255–261. doi:10.1016/j.yexcr.2018.01.015
Zhang, Y., Chen, Y., Yao, H., Lie, Z., Chen, G., Tan, H., et al. (2019). Elevated Serum Circ_0068481 Levels as a Potential Diagnostic and Prognostic Indicator in Idiopathic Pulmonary Arterial Hypertension. Pulm. Circ. 9, 204589401988841. doi:10.1177/2045894019888416
Zhang, Y., Peng, B., and Han, Y. (2018). Mir-23a Regulates the Proliferation and Migration of Human Pulmonary Artery Smooth Muscle Cells (Hpasmcs) through Targeting Bmpr2/smad1 Signaling. Biomed. Pharmacother. 103, 1279–1286. doi:10.1016/j.biopha.2018.04.172
Zhang, Y., and Xu, J. (2016). Mir-140-5p Regulates Hypoxia-Mediated Human Pulmonary Artery Smooth Muscle Cell Proliferation, Apoptosis and Differentiation by Targeting Dnmt1 and Promoting Sod2 Expression. Biochem. Biophysical Res. Commun. 473, 342–348. doi:10.1016/j.bbrc.2016.03.116
Zhang, Z., Li, Z., Wang, Y., Wei, L., and Chen, H. (2019). Overexpressed Long Noncoding RNA CPS1‐IT Alleviates Pulmonary Arterial Hypertension in Obstructive Sleep Apnea by Reducing Interleukin‐1β Expression via HIF1 Transcriptional Activity. J. Cel Physiol 234, 19715–19727. doi:10.1002/jcp.28571
Zhao, M., Chen, N., Li, X., Lin, L., and Chen, X. (2019). Mir-19a Modulates Hypoxia-Mediated Cell Proliferation and Migration via Repressing Pten in Human Pulmonary Arterial Smooth Muscle. Life Sci. 239, 116928. doi:10.1016/j.lfs.2019.116928
Zhou, S., Jiang, H., Li, M., Wu, P., Sun, L., Liu, Y., et al. (2019). Circular Rna Hsa_circ_0016070 Is Associated with Pulmonary Arterial Hypertension by Promoting Pasmc Proliferation. Mol. Ther. - Nucleic Acids 18, 275–284. doi:10.1016/j.omtn.2019.08.026
Zhou, S., Sun, L., Cao, C., Wu, P., Li, M., Sun, G., et al. (2018). Hypoxia‐induced microRNA‐26b Inhibition Contributes to Hypoxic Pulmonary Hypertension via CTGF. J. Cel. Biochem. 119, 1942–1952. doi:10.1002/jcb.26355
Zhou, S., Zhang, S., Wang, Y., Yi, S., Zhao, L., Tang, X., et al. (2015). Mir-21 and Mir-222 Inhibit Apoptosis of Adult Dorsal Root Ganglion Neurons by Repressing Timp3 Following Sciatic Nerve Injury. Neurosci. Lett. 586, 43–49. doi:10.1016/j.neulet.2014.12.006
Zhu, T.-T., Sun, R.-L., Yin, Y.-L., Quan, J.-P., Song, P., Xu, J., et al. (2019a). Long Noncoding Rna uca1 Promotes the Proliferation of Hypoxic Human Pulmonary Artery Smooth Muscle Cells. Pflugers Arch. - Eur. J. Physiol. 471, 347–355. doi:10.1007/s00424-018-2219-8
Keywords: pulmonary hypertension, long non-coding RNA, circular RNA, microRNA, network
Citation: Zang H, Zhang Q and Li X (2021) Non-Coding RNA Networks in Pulmonary Hypertension. Front. Genet. 12:703860. doi: 10.3389/fgene.2021.703860
Received: 30 April 2021; Accepted: 08 November 2021;
Published: 30 November 2021.
Edited by:
Shaveta Kanoria, Wadsworth Center, United StatesReviewed by:
Yuan Zhou, Peking University, ChinaHugo E. Verdejo, Pontificia Universidad Católica de Chile, Chile
Hui Zhang, University of Colorado, United States
Copyright © 2021 Zang, Zhang and Li. This is an open-access article distributed under the terms of the Creative Commons Attribution License (CC BY). The use, distribution or reproduction in other forums is permitted, provided the original author(s) and the copyright owner(s) are credited and that the original publication in this journal is cited, in accordance with accepted academic practice. No use, distribution or reproduction is permitted which does not comply with these terms.
*Correspondence: Xiaodong Li, TGljYXJkaW9AMTYzLmNvbQ==