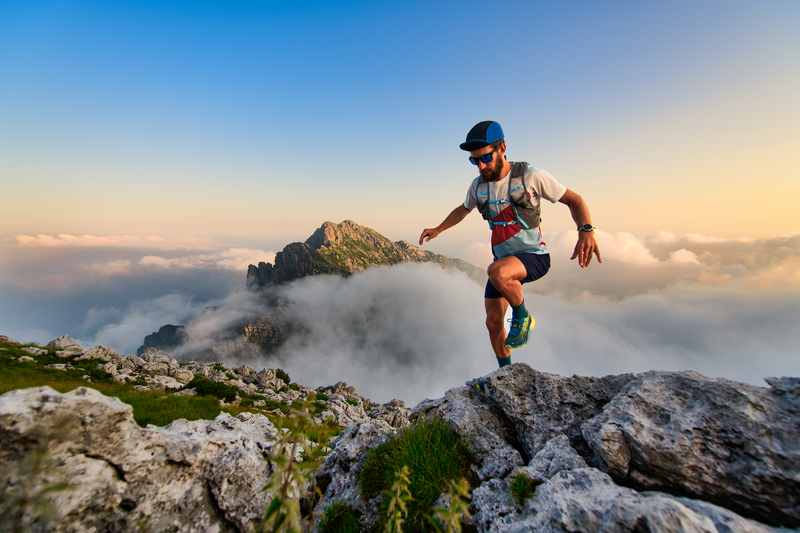
94% of researchers rate our articles as excellent or good
Learn more about the work of our research integrity team to safeguard the quality of each article we publish.
Find out more
ORIGINAL RESEARCH article
Front. Genet. , 23 June 2021
Sec. Evolutionary and Population Genetics
Volume 12 - 2021 | https://doi.org/10.3389/fgene.2021.693541
Genomic size variation has long been a focus for biologists. However, due to the lack of genome size data, the mechanisms behind this variation and the biological significance of insect genome size are rarely studied systematically. The detailed taxonomy and phylogeny of the Ensifera, as well as the extensive documentation concerning their morphological, ecological, behavioral, and distributional characteristics, make them a strong model for studying the important scientific problem of genome size variation. However, data on the genome size of Ensifera are rather sparse. In our study, we used flow cytometry to determine the genome size of 32 species of Ensifera, the smallest one being only 1C = 0.952 pg with the largest species up to 1C = 19.135 pg, representing a 20-fold range. This provides a broader blueprint for the genome size variation of Orthoptera than was previously available. We also completed the assembly of nine mitochondrial genomes and combined mitochondrial genome data from public databases to construct phylogenetic trees containing 32 species of Ensifera and three outgroups. Based on these inferred phylogenetic trees, we detected the phylogenetic signal of genome size variation in Ensifera and found that it was strong in both males and females. Phylogenetic comparative analyses revealed that there were no correlations between genome size and body size or flight ability in Tettigoniidae. Reconstruction of ancestral genome size revealed that the genome size of Ensifera evolved in a complex pattern, in which the genome size of the grylloid clade tended to decrease while that of the non-grylloid clade expanded significantly albeit with fluctuations. However, the evolutionary mechanisms underlying variation of genome size in Ensifera are still unknown.
The haploid DNA content per cell, referred to as the genome size or C value, is a basic biological trait of living organisms (Swift, 1950; Greilhuber et al., 2005). It is typically measured in picograms (pg; 1 pg = 10−12 g) or megabase pairs (Mbp) where 1 pg = 978 Mbp of DNA. The genome size of different organisms varies dramatically, spanning more than 200,000-fold among eukaryotes (Gregory, 2001) and with at least 7,000-fold variation among animals (Dufresne and Jeffery, 2011). Genome size has a major impact on a range of fitness-related parameters such as growth, metabolism, life history traits, and for many species also body size (Dufresne and Jeffery, 2011; Alfsnes et al., 2017; Yu et al., 2020). Universally, an increase in genome size is concomitant with an increase in cell size, confirmed in almost all biological groups (Mirsky and Ris, 1951; Horner and Macgregor, 1983; Cavalier-Smith, 1985; Gregory, 2000, 2002a, 2005a; Hardie and Hebert, 2003). An increase in cell size leads to a significant increase in the duration of cell division (Bennett, 1977). Since development at the organism level consists of division and growth at the cell level, rate of development is negatively related to genome size. This negative correlation has been demonstrated in some diploid plants and some insects, crustaceans, salamanders, and mammals (Bennett, 1987; White and Mclaren, 2000; Gregory, 2002b, 2005a; Alfsnes et al., 2017). In addition, in many animals, metabolic rate (Kozłowski et al., 2003; Gardner et al., 2020), body size (Glazier, 2021), chromosome number (Ardila-Garcia and Gregory, 2009), and latitude (Carta and Peruzzi, 2016) and altitude (Reeves et al., 1998; Akbudak et al., 2018) also exhibit well-established correlations with genome size. Although these organism level correlations are not universal across all taxonomic groups, all taxonomic groups at least exhibit correlations between genome size, cell size, and cell division rate.
So far, the genome sizes of 6,222 animals have been recorded in the Animal Genome Size Database (http://www.genomesize.com/), represented by 3,793 vertebrates and 2,429 invertebrates (Gregory, 2020). Despite being the most diverse lineage on earth, the genome size of the insects is recorded in only 1,244 species, indicating that the genome size data of the Insecta are relatively limited. Moreover, more than two-thirds of these 1,244 records from the Diptera (386 records), Coleoptera (278 records), and Hymenoptera (240 records). The Orthoptera is the only group of Insecta with a significantly enlarged genome (Alfsnes et al., 2017). The largest known Orthoptera genome is more than 1C = 16 pg, and the genome of most Acrididae is over 1C = 6 pg, far larger than that of mammals (1.42~5.68 pg), birds (1.67~2.25 pg), and most other insects (0.98~8.90 pg) (Gregory, 2020). However, there are only 76 records of the genome size of Orthoptera, covering only 50 species. Among the 50 Orthoptera species, most of the records of genome size are for the Caelifera, with 40 species and 60 records, while only 10 species and 16 records represent the suborder Ensifera. Tettigoniidae, as the most diverse group of Ensifera, has only three records for genome size.
Genome size is one of the most fundamental biological traits of living organisms, not only containing genetic information (genotypic) but also providing an organism's structural components (nucleotypic) (Glazier, 2021; Johnson et al., 2021). At the same time, genome size represents an important basis for comparative research into genome evolution. The lack of genome size data will seriously hinder evolutionary genomics research in the era of genomics. In recent years, studies on the genome size of Orthoptera have been reported for several groups, including our lab, but these studies have focused on the suborder of Caelifera, especially Acrididae (Mao et al., 2020; Shah et al., 2020; Husemann et al., 2021). Ensifera, one of the two monophyletic suborders of Orthoptera, are characterized by long, thread-like antennae, usually longer than the body, and thus are also known as “long-horned grasshoppers,” and include the familiar insects such as crickets, katydids, wetas, and their relatives (Song, 2018). Ensifera is the most diverse group in Orthoptera, but until now there has been no report on Ensiferan genome size and evolution. Characterizing and quantifying genome size variation among Ensifera and whether there is an evolutionary correlation between their genome size and other morphological traits will help us to further understand this group and its significantly enlarged genomes.
With the development of sequencing technology, especially the application of the third-generation single-molecule real-time technology involved in genome assembly, more and more genomes of non-model organisms are being dissected (Ma et al., 2021; Yang et al., 2021). However, for non-model organisms with large and complex genomes, there are still huge difficulties in completing their genome assembly. Despite the current momentum in genomics, large and complex genome sequencing is not available or affordable in most laboratories. Determining the genome size of these non-model organisms with large and complex genomes will not only provide important basic data but also reliable information for the design of subsequent whole-genome sequencing schemes.
As one of the most diverse groups in Orthoptera, Ensifera are well-understood taxonomically and phylogenetically, providing a strong basis for studying the mechanisms of variation and the biological significance of their genome size. In our study, we used flow cytometry to determine the genome size of 32 species of Ensifera. At the same time, we assembled the mitochondrial genomes of nine Ensifera species and combined this with the mitochondrial genomes present in a public database to construct a phylogenetic tree containing 32 Ensifera species and three outgroups. Based on the inferred phylogenetic trees, we detected the phylogenetic signal of genome size variation, compared and analyzed the evolutionary correlation of genome size with body size and flight ability, and constructed the ancestral state of genome size. The results of this study have important theoretical significance for solving the evolutionary patterns of, and influencing factors on, size variation in insect genomes, and they lay a solid foundation for subsequent research into the genome of Ensifera.
A total of 253 adults from 32 Ensifera species were collected from Shaanxi, Guangxi, Inner Mongolia, and Guizhou provinces of China during July to October 2019. The collection information is shown in Supplementary Table 1. All specimens were transported to the laboratory alive and identified based on morphological descriptions. Then, they were frozen rapidly with liquid nitrogen and the heads of the specimens were collected for flow cytometry.
Genome size was estimated by flow cytometry according to the methods of Mao et al. (2020), Hare and Johnston (2012), and Hanrahan and Johnston (2011). In brief, the full head or half-head of an adult Ensifera specimen (depending on the size for that species) and a suitable internal standard–the head of a male adult Periplaneta americana (PAM; 1C = 3.41 pg) or the red blood cells of male Gallus domesticus (GRBC; 1C = 1.165 pg) (Mao et al., 2020)–was placed into 1 mL of cold Galbraith buffer. Then, a 2 mL Kontes Dounce tissue grinder (Kontes Glass Co., Vineland, NJ, USA) with type A pestle was used to grind the cells in order to release the nuclei. After grinding, the unwanted cellular debris were filtered with 37 μm nylon mesh, and the nuclei released from the solution were collected into a 1 mL centrifuge tube, which was centrifuged at 1,000 g for 5 min, after which the supernatant was discarded. The precipitate was then suspended in 500 μL of phosphate buffer saline (PBS) in the presence of 10 μg/mL Rnase and stained for 30 min in the cold and dark with a final concentration of 50 μg/mL of propidium iodide (PI). Depending on the availability of live samples, 3–16 replicates were measured per species (i.e., biological replicates) with PAM or GRBC as the standard; for each replicate, at least 10,000 nuclei were measured under each 2C peak with the coefficient of variation (CV) of all 2C peaks <5% using a CyAn ADP flow cytometer (Beckman Coulter, Indianapolis, IN, USA) fitted with the laser tuned to 488 nm (Supplementary Table 2; Additional file 2 in Supplementary Material). DNA content was estimated by comparing the ratio of the mean 2C of the sample with the mean 2C of the standard (Lower et al., 2017).
Genome size (bp) was calculated from DNA content (pg) with the following formula (Dolezel et al., 2003): genome size (bp) = (0.978 × 109) × DNA content (pg), which uses the most accurate conversion factor (DoleŽel and Greilhuber, 2010). Mean estimates were calculated for both females and males of a given species derived from the multiple genome size estimates obtained (Table 1). Student's t-tests performed with SPSS Statistics v18.0 software (SPSS Inc., Chicago, IL, USA) were used to test for differences of internal standards (PAM vs. GRBC) in six species (Conocephalus gladiatus, Metrioptera bonneti, Kuwayamaea brachyptera, Deracantha onos, Mecopoda elongata, and Teleogryllus emma) and differences between the sexes (female vs. male) in eight species (Ruspolia lineosa, C. gladiatus, M. bonneti, Elimaea berezovskii, K. brachyptera, Phaneroptera gracilis, D. onos, and M. elongata).
Table 1. Genome sizes (pg) of males and females of 32 Ensifera species, determined using flow cytometry.
Body size and forewing length were measured from 88 ethanol-preserved adult specimens representing 22 Tettigoniidae species (Supplementary Table 3). As the body size of females varies with the ovulation cycle, we used the length of the hind femur as an indicator of body size (Chapman, 1990; Hochkirch and Gröning, 2008). The femur length is a good proxy for adult body size, confirmed in many studies (Laiolo et al., 2013; Bidau et al., 2016; Anichini et al., 2017; García-Navas et al., 2017). Specimens were photographed on 1-mm grids using a VHX-6000 digital microscope (Keyence, Osaka, Japan) and hind femur length and forewing length were measured. Due to the limited number of specimens, the length of the hind femur and forewing of five species (Conocephalus melaenus, Atlanticus sinensis, Gampsocleis sinensis, Tegra novaehollandiae viridinotata, and Phyllomimus sinicus) were obtained from the literature (Supplementary Table 3). The mean lengths of the hind femur and forewing in female and male species were shown in Supplementary Table 4.
Genomic DNA of nine Ensifera species (Supplementary Table 5) was isolated from the leg muscle tissue of one individual per species using the TIANamp Genomic DNA Kit (Tiangen Biotech, Beijing, China) following the manufacturer's protocols. DNA libraries were prepared using the NEB Next Ultra DNA Library Prep Kit for Illumina (NEB, Ipswich, MA, USA) according to the manufacturer's instructions, and 150 bp paired-end reads were sequenced on the Illumina Hiseq X 10 platform, obtaining 4 Gb of raw data for each species (Supplementary Table 5). The library preparation and sequencing was completed by the Biomarker Technology Company, Beijing, China.
The mitogenomes were de novo assembled based on raw reads using MitoZ v.2.4-alpha software (Meng et al., 2019) with the “all” module. First, raw reads were filtered with a Perl script to obtain clean reads, and clean reads were assembled de novo using SOAPdenovo-Trans (Xie et al., 2014) with the quick mode (-K 71). Then HMMER v.3.1b2 (Wheeler and Eddy, 2013) was utilized to construct profile Hidden Markov Models (profile HMMs), and candidate mitogenome sequences were screened based on them. The protein-coding genes (PCGs) were annotated by BLAST v.2.2.19 (Gertz et al., 2006) and GeneWise v.2.2.0 (Birney et al., 2004) using homologous prediction, and tRNA and rRNA were annotated by MiFFi (Jühling et al., 2011) and infernal-1.1.1 (Nawrocki and Eddy, 2013). The mitogenomes of all new sequences have been deposited in GenBank with the accession numbers listed in Supplementary Table 5.
Phylogenetic trees were constructed using the nucleotide sequences of 13 PCGs and two rRNAs (rrnL and rrnS) from 35 mitogenomes including 32 Ensiferan species and three Caeliferan outgroups (Atractomorpha sinensis, Tetrix japonica, and Locusta migratoria migratoria) (Supplementary Table 6). All 13 PCGs were codon-based, aligned using ClustalW in MEGA7.0 software (Kumar et al., 2016) and then reverted back to their nucleotide sequences, and the two rRNAs were individually aligned with ClustalW using the default settings. The alignments of the 15 genes were concatenated into a single data matrix using SequenceMatrix v.1.8 (Vaidya et al., 2011). A total of 41 data blocks (13 PCGs divided into individual codon positions and two rRNAs) were partitioned from the single data matrix, and then PartitionFinder v2.1.1 (Lanfear et al., 2012) using the “greedy” algorithm (heuristic search) and “unlinked” branch lengths was used to identify the best-fit partitioning scheme for the data blocks, and to estimate the optimal nucleotide substitution model for each partition.
We used maximum likelihood (ML) and Bayesian inference (BI) methods to perform phylogenetic inference and assess the support of the clades. ML analysis was performed by RaxML v8.2.12 (Stamatakis, 2014) with the optimal partitions and best models selected by PartitionFinder2 (Lanfear et al., 2012), and 1,000 replicates to evaluate node support. BI analysis was performed by MrBayes v3.2 (Ronquist et al., 2012), and also by employing the optimal partitions and best models selected by PartitionFinder2, with four Markov Chain Monte Carlo (MCMC) chains for 10,000,000 generations. Each set was sampled every 1,000 generations, the first 25% of generations were discarded as burn-in, and the remaining samples were used to obtain the consensus tree. We also performed a divergence time estimate analysis using BEAST v1.10.4 (Suchard et al., 2018) to estimate the relative time of divergence of the studied taxa. The optimal partitions and nucleotide evolution models were recommended by PartitionFinder2. For each partition an uncorrelated lognormal relaxed-clock was implemented, and the tree prior was set to a Yule process. Three calibration points were used to impose age constraints of the tree. The first calibration point we selected was the fossil of Raphogla rubra Béthoux, 2002, known from the Permian of France [260.4–251 million years ago (Mya)] (Bethoux et al., 2002). This is the oldest definitive fossil of Ensifera as recognized by several previous studies (Song et al., 2015; Zhou et al., 2017; Li et al., 2019). The second calibration point we selected was the fossil of Gryllus vociferans Cockerell (1925), known from the Margas Verdes Formation of the Paleocene of Argentina (66.0–56.0 Mya) (Cockerell, 1925). G. vociferans is the oldest definitive fossil of Gryllinae, which we used to calibrate the clade of Gryllinae. The final calibration point we selected was the fossil of Conocephalus martyi (Piton, 1940), known from the Menat Formation of the Paleocene of France (59.2–56.0 Mya) (Piton, 1940), which is the oldest known fossil of Conocephalinae (Zhou et al., 2017; Li et al., 2019). For the BEAST analysis, we ran 1 × 109 generations with sampling every 1,000 generations, and a burn-in of 25% of the trees. Tracer v.1.6 (Rambaut and Drummond, 2009) was used to inspect the results and confirmed that all parameters were achieved ESS > 200. TreeAnnotator v1.10.4 (Rambaut and Drummond, 2002) was used to summarize the maximum clade credibility (MCC) tree, median ages, and 95% highest posterior density (HPD).
To test for phylogenetic signal in the genome size of the 32 Ensifera species, three common metrics, including Pagel's lambda (λ) (Pagel, 1999), Blomberg's K (Blomberg et al., 2003), and Abouheif's Cmean (Abouheif, 1999) were estimated based on the phylogeny we constructed. Pagel's λ is a branch-length transformation method, estimated by ML to test the best fit of a trait against a Brownian model. The values of Pagel's λ vary from 0 to 1, with 0 indicating no phylogenetic signal and a random distribution of traits, 1 indicating a strong phylogenetic signal and the distribution of traits following the Brownian motion model. λ > 1 is possible because λ is not a correlation but a scaling factor for a correlation. The metrics of Blomberg's K are quite different from Pagel's λ. Blomberg's K is a variance ratio (a scaled ratio of the variance among species over the contrasts variance), which is rescaled by dividing by the Brownian motion expectation. When K < 1, the evolution of traits is independent of the phylogeny, and when K > 1 they are dependent of the phylogeny. Abouheif's Cmean is another way of testing for phylogenetic signal, however it does not rely on an evolutionary model but rather measures autocorrelation among tips by a specific phylogenetic approximation matrix and can be tested by random permutation. Pagel's λ and Blomberg's K were calculated using the phylosig function in the phytools package (Revell, 2012) in R v3.6.1 (Team, 2013), and Abouheif's Cmean was calculated using the adephylo package (Jombart and Dray, 2010).
To identify where in the phylogeny the genome size changed, we reconstructed the ancestral state of genome size using three methods: maximum parsimony (MP) analysis using Mesquite v3.61 (Maddison and Maddison, 2015) with default settings, ML analysis using the fastAnc function from the phytools package (Revell, 2012) in R v3.6.1 (Team, 2013), and BI analysis using the MCMC model A (continuous: random walk) in BayesTraits v3.0.2 (Pagel et al., 2004). For BI analysis, we first considered whether genome size evolution followed models A (random walk) or B (directional) by model testing implemented in BayesTraits. The log marginal likelihoods for models A and B were estimated using stepping-stone sampling, running 1,000 stones with 100,000 iterations for each stone, and then a log Bayes factor (BF) was calculated. The model tests of BF indicated that model A was significantly better than model B (BFfemale = 10.95; BFmale = 11.17), so we used model A for the downstream analysis. To estimate the ancestral genome sizes in BayesTraits, the Markov chain model was generated to run 100 million generations, with a burn-in period of 5 million generations and chain sampling every 10,000 generations.
We also examined the phylogenetic relationships among genome size, body size, and forewing length of 22 Tettigoniidae species using phylogenetic generalized least squares (PGLS). Prior to PGLS analysis, we performed an ordinary least squares (OLS) regression analysis (equivalent of PGLS with λ = 0), which assumed phylogenetic independence among all of the traits, using the function lm in the package stats in R v3.6.1. We then performed a PGLS analysis in the R package caper (Orme et al., 2013), using the ML estimation of λ to transform branch lengths. In the PGLS analysis, we used genome size as the response variable, and body size and forewing length as the predictor variables. The time-calibrated tree obtained by BEAST was pruned to the 22 Tettigoniidae for PGLS analysis. All trait values were log transformed before performing PGLS analysis. We also investigated the phylogenetic signal (Pagel's λ, Blomberg's K, and Abouheif's Cmean) of genome size, body size and forewing length measured in the 22 Tettigoniidae species to evaluate the appropriateness of the test for statistical significance.
We assembled the complete mitogenomes of nine Ensifera species using the Illumina data sequenced in this study (Supplementary Table 5). The size of these mitogenomes ranged from 15,297 bp (Diestrammena sp.) to 16,416 bp (Ruidocollaris sinensis). All mitogenomes have the typical gene content found in metazoan mitogenomes: 13 PCGs, 22 tRNA genes, two rRNA genes, and one non-coding region (the A+T-rich region). Nine PCGs, 14 tRNAs, and the A+T-rich region are located in the major strand (J-strand), and four PCGs, eight tRNAs, and two rRNAs are located in the minor strand (N-strand). The gene order and orientation of the mitogenome of all nine species are identical to that of the ancestral mt gene. The base composition, A+T content, G+C content, AT skew, and GC skew exhibit similar characteristics in the nine species (Supplementary Table 5).
In this study, the genome sizes of 32 Ensifera species were measured by flow cytometry. To accurately estimate the genome size of each species, 3–16 biological replicates were measured per species depending on the availability of living samples, and two internal standards, PAM (1C = 3.41 pg) and GRBC (1C = 1.165 pg), were used for all species except for Pseudorhynchus crassiceps, C. maculatus, R. sinensis, Microconema clavata, T. novaehollandiae viridinotata, P. sinicus, Loxoblemmus equestris, Gryllodes sigillatus, Truljalia hibinonis, Oecanthus sinensis, Diestrammena sp., and Ornebius kanetataki (Supplementary Table 2; Additional file 2 in Supplementary Material). In addition, we also selected six species to compare the genome size estimated by the two internal standards (PAM vs. GRBC), to test the effect of internal standard on estimated genome size (Supplementary Table 7). The results showed that except for C. gladiatus (P = 0.011) and D. onos (P = 0.042) in females and M. bonneti (P = 0.043) in males, there was no significant difference (P > 0.05) in genome size estimated by the two different internal standards of PAM and GRBC. Since multiple genome size estimates were obtained for both females and males of each species, the estimate mean was calculated (Table 1).
Across the estimated genome sizes of the 32 Ensifera species, C-values ranged from 1C = 0.952 pg (the male of O. sinensis) to 1C = 19.135 pg (the female of D. onos) representing a >20-fold range. Within families, the genomes of Tettigoniidae were generally large, the variation of genome size was above 5.5-fold (3.473–19.135 pg), and the average genome size reached 8.276 pg. The genomes of Gryllidae were relatively small, ranging in size from 1C = 0.952 pg to 1C = 2.612 pg (2.7-fold) with an average genome size of 2.044 pg. For the remaining four families, including Gryllotalpidae, Mogoplistidae, Rhaphidophoridae, and Gryllacrididae, only one species of each family had its genome size measured of which the genome size of females of the Ocellarnaca sp. of Gryllacrididae was found to be relatively large, reaching 1C = 9.451 pg.
At present, there are a total of 76 records (representing 50 species) of the genome size of Orthoptera insects in the Animal Genome Size Database (http://www.genomesize.com), most of which are Acrididae (58 records). The Orthoptera genome size recorded in the database ranged from 1.55 pg in the cave cricket Hadenoecus subterraneus to 16.93 pg in the mountain grasshopper Podisma pedestris, representing a nearly 11-fold range. All of these are much larger than the smallest genome we have newly measured (the male of O. sinensis: 1C = 0.952 pg), and much smaller than the largest genome we have newly measured (the female of D. onos: 1C = 19.135 pg). Our study provides a broader blueprint for genome size variation of Orthoptera. To better understand the genome size variation of the Orthoptera in families, we collected the genome size data of Orthoptera from the Animal Genome Size Database and the genome size data of Orthoptera published by Mao et al. (2020), Shah et al. (2020), and Husemann et al. (2021) in recent years. We combined these with the genome size data of 32 newly measured Ensifera species for statistical analysis (Figure 1). The genome sizes of 11 families of Orthoptera were obtained; the genome size variations within the Tettigoniidae and Acrididae each represent a large range. Interestingly, Tettigoniidae and Acrididae are the two most diverse families of Orthoptera and appear to have undergone explosive, adaptive radiation (Song et al., 2015).
Figure 1. Boxplot of genome sizes for families of Orthoptera, including data from the present study and the Animal Genome Size Database (Gregory, 2020).
Typically, sex has a significant effect on genome size variation at the within-species level, and the significant effects caused by sex cannot be determined with estimates that ignore or merge values (Hanrahan and Johnston, 2011). In our study, we separated genome size estimates by sex for all species except for a few where sample size was very limited (Table 1; Supplementary Table 2). By analyzing the sex differences of eight species for which we had at least five replicates of each sex (Figure 2; Supplementary Table 8), we found that females had significantly larger genomes than males in every case (P < 0.01). In Caelifera, the genome sizes of females are also significantly larger than those of males (Mao et al., 2020). The sex difference in genome size may be caused by the sex chromosome because most Ensifera species are XO sex determined (Warchalowska-Sliwa, 1998). Among the eight species, the genome of females is ~10% larger than that of males, indicating that the X chromosome is ~10% of the genome. Based on this inference, we estimated genome sizes where they were missing: female T. hibinonis (1C = 2.452 pg) and male C. melaenus (1C = 3.871 pg), Tettigonia chinensis (1C = 6.012 pg), G. sinensis (1C = 6.106 pg), T. novaehollandiae viridinotata (1C = 3.126 pg), P. sinicus (1C = 5.322 pg), Gryllotalpa orientalis (1C = 3.785 pg), L. equestris (1C = 2.201 pg), and Ocellarnaca sp. (1C = 8.506 pg). These estimates were used for subsequent analysis.
Figure 2. Comparative analysis of the genome sizes of females and males in eight Ensifera species. *Indicates a significant difference between both sexes (P < 0.01).
To explore the evolutionary pattern of genome size within Ensifera, we constructed three phylogenetic trees for the measured species using the mitogenome sequences of 13 PCGs and two rRNAs based on the software packages RaxML, MrBayes, and BEAST. Phylogenies obtained by these packages were consistent and robust, and largely congruent with those reconstructed previously (Zhou et al., 2017; Li et al., 2019) (Supplementary Figures 1–3). The phylogenetic trees were divided into two clades: grylloid (the infraorder Gryllidea) and non-grylloid. In the grylloid clade there were eight species, six of which were Gryllidae, categorized into a monophyletic group. In the non-grylloid species, Tettigoniidae was the most diverse group with 22 species, also belonging to a monophyletic group. The divergence time analysis showed that the split between grylloid and non-grylloid occurred at the Permian/Triassic boundary, and both Gryllidae and Tettigoniidae originated in the Late Jurassic and diversified into major lineages in the Cretaceous. This estimated timescale is consistent with previous studies (Song et al., 2015; Li et al., 2019).
Based on the inferred phylogenetic relationship, we estimated Pagel's λ, Blomberg's K, and Abouheif's Cmean to determine the phylogenetic signal of genome size variation. In the analysis of 32 Ensifera species, both male and female genome sizes exhibited strong phylogenetic signals (female: Pagel's λ = 1.028, Blomberg's K = 0.769, Abouheif's Cmean = 0.444; male: Pagel's λ = 1.045, Blomberg's K = 0.770, Abouheif's Cmean = 0.440), indicating that the genome size of related species was more similar than expected under Brownian motion. In the analysis of 22 species of Tettigoniidae, there were also strong phylogenetic signals for the genome size of males and females (female: Pagel's λ = 1, Blomberg's K = 0.840, Abouheif's Cmean = 0.221; male: Pagel's λ = 1, Blomberg's K = 0.854, Abouheif's Cmean = 0.222), but the phylogenetic signals were significantly smaller than those of the 32 Ensifera species in terms of Abouheif's Cmean, which might be influenced by the number of taxa sampled (see Supplementary Table 9 for details).
We also assessed the evolution of body size and forewing length of the 22 Tettigoniidae species using phylogenetic signals. We found that both males and females had strong phylogenetic signals in their forewing length (female: Pagel's λ = 1, Blomberg's K = 0.799, Abouheif's Cmean = 0.266; male: Pagel's λ = 1, Blomberg's K = 0.801, Abouheif's Cmean = 0.292), whereas body size showed only moderate phylogenetic signals (female: Pagel's λ = 1, Blomberg's K = 0.698, Abouheif's Cmean = 0.149; male: Pagel's λ = 0.78, Blomberg's K = 0.685, Abouheif's Cmean = 0.139) (Supplementary Table 9). With the 22 Tettigoniidae species, we tested whether the differences in inter-species genome size were related to flight ability in terms of forewing length (where a longer forewing is generally considered to be associated with stronger flight ability) and body size, using OLS and PGLS regression analysis. We found no correlation between genome size and flight ability or body size in both OLS and PGLS regression analyses (Supplementary Table 10).
The genome sizes of the ancestral nodes of Ensifera species were estimated based on the inferred phylogenetic relationship using three methods: MP, ML, and BI (Figure 3; Table 2; Supplementary Figure 4). For females, the genome size of the most common ancestor of Ensifera (Node-07) reconstructed using MP was 5.909 pg, using ML was 5.354 pg, and using BI was 5.372 ± 0.938 pg. For males, the ancestral genome size of Ensifera (Node-07) reconstructed using MP, ML, and BI was 5.367, 4.843, and 4.875 ± 0.910 pg, respectively. In comparing the three methods of ancestral state reconstruction, the results of the MP method were found to be slightly different from those of the ML method and BI method, while there was no difference between the ML and BI methods, and the results of the ML and BI methods were more reliable (Table 2). Across the ancestral state reconstruction of Ensifera genome size, we found several increasing and decreasing events. In the ancestral lineages of the grylloid clade, the genome sizes underwent a dramatic decrease, while in the ancestral lineages of the non-grylloid clade, the genome sizes increased (Figure 3; Table 2; Supplementary Figure 4). For the grylloid clade, the genome size of the ancestral node (Node-06) was 4.469 ± 1.224 pg (female, BI method) and then decreased to 2.693 ± 0.980 pg (the ancestral node of Gryllidae [Node-05, female, BI method]), while the genome size of O. sinensis was the smallest, decreasing to 1.081 pg (female). For the non-grylloid clade, there was a significant expansion in genome size. Up to the ancestral node of Tettigoniidae (Node-04), genome size had increased to 7.975 ± 0.852 pg (female, BI method), and D. onos had the largest expansion, reaching 19.135 pg (female). In addition to the expansion of genome size, there were multiple evolutionary transitions from a very large ancestral genome size to a smaller genome size in the non-grylloid clade. For example, there were three independent lineages in the Tettigoniidae that transitioned to a smaller genome size—Tegra, Conocephalus, and Microconema—all of which have genome sizes smaller than 5 pg. However, genome size expansion from the ancestral state is a general trend in the non-grylloid clade.
Figure 3. Ancestral reconstruction of the genome size of (female) Ensifera based on a time-calibrated phylogenetic tree. The black circles with numbers on the nodes correspond to the estimated ancestral genome size using fastAnc, BayesTrait, and Mesquite in Table 2. The average genome size of each species is shown in a histogram on the right.
Table 2. The estimated genome size in seven ancestral nodes inferred using fastAnc, BayesTrait, and Mesquite.
Although our knowledge of genome sizes in insects is steadily increasing, our knowledge of the suborder Ensifera lags behind. Prior to our study, there were only 10 species and 16 records of Ensifera in the Animal Genome Size Database (http://www.genomesize.com) and most of these were Gryllidae (five species and 11 records). These published data provided only limited information on the pattern of genome size diversity in Ensifera. In our study, we measured the genome size of 32 species of Ensifera using flow cytometry (Table 1; Supplementary Table 2; Additional file 2 in Supplementary Material), which served to considerably increase the number of known genome sizes of Ensifera and provided data for studying the evolution of this suborder's genome size; the first study of its kind for Ensifera. Compared with all other insects in the Animal Genome Size Database, the genome size of Ensifera is very large with considerable variation. The smallest genome size was found in Gryllidae (the male of O. sinensis: 1C = 0.952 pg), and the largest in Tettigoniidae (the female of D. onos: 1C = 19.135 pg). The difference between the largest and smallest genome size was over 20-fold. At the same time, the results of ancestral state reconstruction inferred using Mesquite, fastAnc and BayesTraits revealed that the genome size of Ensifera has evolved in complex ways (Figure 3; Table 2; Supplementary Figure 4). The genome size of the grylloid clade decreased while the genome size of the non-grylloid clade increased significantly, with fluctuations.
Genome downsizing may be due to unequal intra-strand homologous recombination, double-strand break repair, illegitimate recombination or retroelement extinction (Chen et al., 1998; Vicient et al., 1999; Shirasu et al., 2000; Orel and Puchta, 2003; Dufresne and Jeffery, 2011). The removal of extra DNA is thought to avoid the extra metabolic costs needed to maintain the harmonization of genome constituents, an energy efficiency that should favor species survival (Gregory and Hebert, 1999). Gryllidae form the second most diverse clade of the ancient lineages within Ensifera, with more than 4,800 known species (Eades, 2000). Recent studies have found no major shifts in the rate of diversification in Gryllidae, which have continually diversified since the origin of the clade (Song et al., 2015). This consistency of diversification rate may be related to the continuous decrease of the Gryllidae genome size. Studies have shown that genome size reductions can sometimes be followed by low rates of extinction (Volff, 2005; Organ et al., 2007; Gregory et al., 2009).
Tettigoniidae represent the most successful lineage within Ensifera in terms of species diversity with over 7,500 known species and may have undergone explosive adaptive radiation (Mugleston et al., 2018). Our study found that the genome size of Tettigoniidae is extremely large, with the average genome size reaching 8.276 pg and the largest exceeding 19 pg—the largest known genome in the Insecta. Genome expansion events usually include small-scale insertions of nucleotides or large-scale alterations such as gene duplications, transposon insertions, or polyploidy (Petrov, 2001; Dufresne and Jeffery, 2011). Although these are very different processes, they have the potential to influence rates of diversification and promote the formation of new species (Kraaijeveld, 2010). Large-scale duplication events are at the base of a number of evolutionary radiations, such as the radiation of teleost fish (Volff, 2005). The radiation evolution of the family Tettigoniidae may be related to the expansion of its genome size, and a large-scale duplication event may have occurred in the early stages of Tettigoniidae radiation.
In our study, we used three methods (Pagel's λ, Blomberg's K, and Abouheif's Cmean) to determine the phylogenetic signals in the genome size variation of 32 Ensifera (Supplementary Table 9). We determined that there was a strongly phylogenetic signal in the genome size of Ensifera species, indicating a tendency for it to be more similar among related species than genome size would be within a random set of species from the same tree (Harvey and Pagel, 1991). Similarly strong phylogenetic signals for genome size have been detected in other insects such as grasshoppers and locusts (Orthoptera, Acrididae) (Mao et al., 2020), fireflies (Coleoptera, Lampyridae) (Lower et al., 2017), butterflies (Lepidotera, Papilionoidea) (Liu et al., 2020), and fruit flies (Diptera, Drosophilidae) (Hjelmen and Johnston, 2017). These findings suggest that genome size is phylogenetically conserved among closely related species, which may be the result of similar gene content and stretches of conserved gene order (Kellogg and Bennetzen, 2004). Meanwhile, we also determined phylogenetic signals for genome size variation in 22 Tettigoniidae (Supplementary Table 9). We found that the phylogenetic signals determined by 32 Ensifera were different from those for 22 Tettigoniidae, especially the value of Abouheif's Cmean, which might be influenced by the number of taxa sampled. Current studies have shown that the method of Abouheif's Cmean is sensitive to taxa number and the measured phylogenetic signal increases with a significant increase in taxa number, whereas Pagel's λ and Blomberg's K are less sensitive to taxa number (Hjelmen and Johnston, 2017). It is therefore suggested that the Abouheif's Cmean can only be used as a preliminary test for the determination of phylogenetic signals while Pagel's λ and Blomberg's K can be considered as definitive measures. In addition, sample sizes of at least 15 are necessary to achieve reliable results in terms of significance for Pagel's λ and Blomberg's K (Hjelmen and Johnston, 2017); our sample size is substantially larger than this.
Morphological measurements of body size and forewing length were also undertaken for 22 Tettigoniidae species (Supplementary Tables 3, 4). We expected to find a positive correlation between genome size and body size, which is assumed to be caused by nucleotypic effects of cell size/volume (Cavalier-Smith, 1978; Gregory, 2005b). However, our PGLS analysis did not find evidence of a positive correlation between genome size and body size (Supplementary Table 10). This is not unusual, for example previous studies of ladybird beetles (Coleoptera, Coccinellidae) (Ryan Gregory et al., 2003) and fireflies (Coleoptera, Lampyridae) (Lower et al., 2017) in Insecta have also not found correlations. Clearly, then, there is not necessarily evolutionary correlation between genome size and body size.
In vertebrates, the association between genome size and flight ability has become increasingly apparent, and strong fliers exhibit smaller genomes than do weak fliers or flightless birds (Hughes, 1999; Gregory, 2005b). In insects, although this relationship has not been well-studied, genome size is related to the flight strategy of dragonflies, with the average genome of “fliers” being much larger than that of “perchers” (Ardila-Garcia and Gregory, 2009). However, the category “fliers” and “perchers” is not a taxonomic artifact of including different suborders in the comparison. In our study, the PGLS analysis found no evidence of a correlation between genome size and flight ability (forewing length) (Supplementary Table 10). Current studies of fireflies have also found that these two variables do not correlate (Lower et al., 2017). However, our sample size is small and more species are needed to rigorously test the correlation between genome size and flight ability, including body size.
In recent decades, it has been recognized that the main mechanism contributing to genome size variation is repetitive DNA, including whole genome duplication (WGD), chromosome aneuploidy/supernumerary, indels, gene duplications/deletions, and transposable elements (TEs) (Petrov, 2001; Gregory, 2005b,c). Polyploidy or WGD is a major contributor to genome evolution and diversity (Li et al., 2018). A recent study reported that ancient polyploidy was found in the ancestors of some insects, including Lepidoptera, Trichoptera, and Odonata, but not in Orthoptera (Li et al., 2018). This indicates that the very large genome of Orthoptera is not caused by paleopolyploidy. Although there has been no report so far on the repetitive DNA content of Ensifera, studies of the L. migratoria genome (Wang et al., 2014), the Schistocerca gregaria genome (Verlinden et al., 2020), and the repetitive elements of several other orthopteran insects (Camacho et al., 2015; Ruiz-Ruano et al., 2018; Shah et al., 2020) have revealed that the proportion of repeats in the large genome of orthopteran insects is very high. In L. migratoria, repetitive elements constituted ~60% of the assembled genome, and the very large genome of L. migratoria may be caused by the continuous proliferation of repetitive elements combined with slow rates of loss for these elements (Wang et al., 2014). The genome size of Ensifera, especially Tettigoniidae, has significantly expanded, which may also be the result of the continuous proliferation of repetitive elements in the genome.
The expansion of the genome is not out of control. Studies have uncovered the Piwi-interacting RNA (piRNA) pathway, a small RNA genome defense system that suppresses TE activity in the animal germline (Mueller, 2017). piRNAs limit the expansion of genome size by guiding the transcription and post-transcriptional silencing of TEs through base complementarity. In addition to piRNA, studies have shown that mate choice can also regulate genome size. In the study of the bow-winged grasshopper Chorthippus biguttulus, males with smaller genomes had more attractive songs and females were more likely to mate with them (Schielzeth et al., 2014). Through this courtship mechanism, the continuous enlargement of the genome is to some extent controlled. The results of ancestral state reconstruction showed that the genomes of the grylloid clade in Ensifera shrank significantly, especially in the Gryllidae. What causes the shrinking of the grylloid clade genome? This is a question worthy of in-depth study, which our future research will focus on.
In conclusion, our study is the first extensive survey of genome size variation within the suborder of Ensifera. We measured the genome size of 32 species of Ensifera using flow cytometry and found that it has an overall 20-fold range from 0.952 to 19.135 pg; our data provide a broader blueprint for genome size variation in Orthoptera. However, the species of Ensifera for which we have genome size data only account for a small percentage of all of the suborder's species. Thus, more species still need to be investigated in order to systematically and comprehensively explore the evolution of Ensifera genome sizes. Nonetheless, the genome size data obtained in this study and the corresponding findings will still be very helpful for in-depth study of the mechanisms of genome size increase and decrease within the Ensifera.
The datasets presented in this study can be found in online repositories. The names of the repository/repositories and accession number(s) can be found at: NCBI [accession: MT849265-MT849273].
HY: conceptualization, methodology, software, formal analysis, investigation, data curation, writing–original draft, writing–review and editing, and visualization. YH: conceptualization, resources, writing–review and editing, project administration, funding acquisition, and supervision. YM: conceptualization, methodology, software, and investigation. NZ: methodology, software, and formal analysis. YN: data curation, methodology, and formal analysis. XZ: data curation and methodology. YZ: investigation, writing-review and editing. SM: conceptualization, methodology, investigation, resources, writing-review and editing, project administration, funding acquisition, and supervision. All authors contributed to the article and approved the submitted version.
This research was funded by the National Natural Science Foundation of China to YH (grant numbers 31872217 and 31372192) and SM (grant number 31601887), the Natural Science Foundation of Shaanxi Province to SM (grant number 2019JQ-195), and the Fundamental Research Funds for the Central Universities to YH (grant numbers GK202107011 and GK202101003).
The authors declare that the research was conducted in the absence of any commercial or financial relationships that could be construed as a potential conflict of interest.
We are very grateful to many students that contributed for specimen collection: Xiaoqiang Guo, Xiaolei Feng, Lina Zhao, Huihui Chang, and Mengyue Xu.
The Supplementary Material for this article can be found online at: https://www.frontiersin.org/articles/10.3389/fgene.2021.693541/full#supplementary-material
Abouheif, E. (1999). A method for testing the assumption of phylogenetic independence in comparative data. Evol. Ecol. Res. 1, 895–909.
Akbudak, M. A., Sakiroglu, M., and Tuna, M. (2018). Estimation of nuclear DNA content and determination of relationship between altitude and genome size of USDA Turkish oat (Avena spp.) collection. Gesunde Pflanz. 70, 171–178. doi: 10.1007/s10343-018-0428-x
Alfsnes, K., Leinaas, H. P., and Hessen, D. O. (2017). Genome size in arthropods; different roles of phylogeny, habitat and life history in insects and crustaceans. Ecol. Evol. 7, 5939–5947. doi: 10.1002/ece3.3163
Anichini, M., Kuchenreuther, S., and Lehmann, G. (2017). Allometry of male sound-producing structures indicates sexual selection on wing size and stridulatory teeth density in a bushcricket. J. Zool. 301, 271–279. doi: 10.1111/jzo.12419
Ardila-Garcia, A., and Gregory, T. (2009). An exploration of genome size diversity in dragonflies and damselflies (Insecta: Odonata). J. Zool. 278, 163–173. doi: 10.1111/j.1469-7998.2009.00557.x
Bennett, M. D. (1977). The time and duration of meiosis. Philos. Trans. R. Soc. Lond. B Biol. Sci. 277, 201–226. doi: 10.1098/rstb.1977.0012
Bennett, M. D. (1987). Variation in genomic form in plants and its ecological implications. New Phytol. 106, 177–200. doi: 10.1111/j.1469-8137.1987.tb04689.x
Bethoux, O., Nel, A., Lapeyrie, J., Gand, G., and Galtier, J. (2002). Raphogla rubra gen. n., sp. n., the oldest representative of the clade of modern Ensifera (Orthoptera: Tettigoniidea, Gryllidea). Eur. J. Entomol. 99, 111–116. doi: 10.14411/eje.2002.019
Bidau, C. J., Taffarel, A., and Castillo, E. R. (2016). Breaking the rule: multiple patterns of scaling of sexual size dimorphism with body size in orthopteroid insects. Rev. Soc. Entomoló. Argentina 75, 11–36.
Birney, E., Clamp, M., and Durbin, R. (2004). GeneWise and genomewise. Genome Res. 14, 988–995. doi: 10.1101/gr.1865504
Blomberg, S. P., Garland Jr, T., and Ives, A. R. (2003). Testing for phylogenetic signal in comparative data: behavioral traits are more labile. Evolution 57, 717–745. doi: 10.1111/j.0014-3820.2003.tb00285.x
Camacho, J. M., Ruiz-Ruano, F., Martín-Blázquez, R., López-León, M., Cabrero, J., Lorite, P., et al. (2015). A step to the gigantic genome of the desert locust: chromosome sizes and repeated DNAs. Chromosoma 124, 263–275. doi: 10.1007/s00412-014-0499-0
Carta, A., and Peruzzi, L. (2016). Testing the large genome constraint hypothesis: plant traits, habitat, and climate seasonality in L iliaceae. New Phytol. 210, 709–716. doi: 10.1111/nph.13769
Cavalier-Smith, T. (1978). Nuclear volume control by nucleoskeletal DNA, selection for cell volume and cell growth rate, and the solution of the DNA C-value paradox. J. Cell. Sci. 34, 247–278. doi: 10.1242/jcs.34.1.247
Cavalier-Smith, T., (ed.). (1985). “Cell volume and the evolution of eukaryote genome size,” in Evol. Genome Size (Chichester: John Wiley), 105–184.
Chen, M., Sanmiguel, P., and Bennetzen, J. L. (1998). Sequence organization and conservation in sh2/a1-homologous regions of sorghum and rice. Genetics 148, 435–443. doi: 10.1093/genetics/148.1.435
Cockerell, T. D. A. (1925). Tertiary fossil insects from Argentina. Nature 116, 711–712. doi: 10.1038/116711a0
Dolezel, J., Bartos, J., Voglmayr, H., and Greilhuber, J. (2003). Nuclear DNA content and genome size of trout and human. Cytometry Part A J. Int. Soc. Anal. Cytol. 51, 127–128. doi: 10.1002/cyto.a.10013
DoleŽel, J., and Greilhuber, J. (2010). Nuclear genome size: are we getting closer? Cytometry Part A 77, 635–642. doi: 10.1002/cyto.a.20915
Dufresne, F., and Jeffery, N. (2011). A guided tour of large genome size in animals: what we know and where we are heading. Chromosome Res. 19, 925–938. doi: 10.1007/s10577-011-9248-x
Eades, D. C. (2000). Evolutionary relationships of phallic structures of Acridomorpha (Orthoptera). J. Orthoptera Res. 9, 181–210. doi: 10.2307/3503648
García-Navas, V., Noguerales, V., Cordero, P. J., and Ortego, J. (2017). Ecological drivers of body size evolution and sexual size dimorphism in short-horned grasshoppers (Orthoptera: Acrididae). J. Evol. Biol. 30, 1592–1608. doi: 10.1111/jeb.13131
Gardner, J. D., Laurin, M., and Organ, C. L. (2020). The relationship between genome size and metabolic rate in extant vertebrates. Philos. Trans. R. Soc. B 375:20190146. doi: 10.1098/rstb.2019.0146
Gertz, E. M., Yu, Y.-K., Agarwala, R., Schäffer, A. A., and Altschul, S. F. (2006). Composition-based statistics and translated nucleotide searches: improving the TBLASTN module of BLAST. BMC Biol. 4:41. doi: 10.1186/1741-7007-4-41
Glazier, D. S. (2021). Genome size covaries more positively with propagule size than adult size: new insights into an old problem. Biology 10:270. doi: 10.3390/biology10040270
Gregory, T. R. (2000). Nucleotypic effects without nuclei: genome size and erythrocyte size in mammals. Genome 43, 895–901. doi: 10.1139/g00-069
Gregory, T. R. (2001). Coincidence, coevolution, or causation? DNA content, cell size, and the C-value enigma. Biol. Rev. 76, 65–101. doi: 10.1017/S1464793100005595
Gregory, T. R. (2002a). A bird's-eye view of the C-value enigma: genome size, cell size, and metabolic rate in the class Aves. Evolution 56, 121–130. doi: 10.1111/j.0014-3820.2002.tb00854.x
Gregory, T. R. (2002b). Genome size and developmental complexity. Genetica 115, 131–146. doi: 10.1023/A:1016032400147
Gregory, T. R. (2005a). The C-value enigma in plants and animals: a review of parallels and an appeal for partnership. Ann. Bot. 95, 133–146. doi: 10.1093/aob/mci009
Gregory, T. R. (2005b). “Genome size evolution in animals,” in The Evolution of the Genome, ed T. R. Gregory (Amsterdam: Elsevier), 3–87.
Gregory, T. R. (2005c). Synergy between sequence and size in large-scale genomics. Nat. Rev. Genet. 6:699. doi: 10.1038/nrg1674
Gregory, T. R. (2020). Animal Genome Size Database. Available online at: http://www.genomesize.com (accessed September 22, 2020).
Gregory, T. R., Andrews, C. B., Mcguire, J. A., and Witt, C. C. (2009). The smallest avian genomes are found in hummingbirds. Proc. R. Soc. B Biol. Sci. 276, 3753–3757. doi: 10.1098/rspb.2009.1004
Gregory, T. R., and Hebert, P. D. (1999). The modulation of DNA content: proximate causes and ultimate consequences. Genome Res. 9, 317–324.
Greilhuber, J., DoleŽel, J., Lysak, M. A., and Bennett, M. D. (2005). The origin, evolution, and proposed stabilization of the terms “genome size” and “C-value” to describe nuclear DNA contents. Ann. Bot. 95, 255–260. doi: 10.1093/aob/mci019
Hanrahan, S. J., and Johnston, J. S. (2011). New genome size estimates of 134 species of arthropods. Chromosome Res. 19:809. doi: 10.1007/s10577-011-9231-6
Hardie, D. C., and Hebert, P. D. (2003). The nucleotypic effects of cellular DNA content in cartilaginous and ray-finned fishes. Genome 46, 683–706. doi: 10.1139/g03-040
Hare, E. E., and Johnston, J. S. (2012). Genome size determination using flow cytometry of propidium iodide-stained nuclei. Methods Mol. Biol. 2011, 3–12. doi: 10.1007/978-1-61779-228-1_1
Harvey, P. H., and Pagel, M. D. (1991). The Comparative Method in Evolutionary Biology. Oxford: Oxford University Press.
Hjelmen, C. E., and Johnston, J. S. (2017). The mode and tempo of genome size evolution in the subgenus Sophophora. PLoS ONE 12:e0173505. doi: 10.1371/journal.pone.0173505
Hochkirch, A., and Gröning, J. (2008). Sexual size dimorphism in Orthoptera (sens. Str.): a review. J. Orthoptera Res. 17, 189–196. doi: 10.1665/1082-6467-17.2.189
Horner, H. A., and Macgregor, H. C. (1983). C value and cell volume: their significance in the evolution and development of amphibians. J. Cell Sci. 63, 135–146. doi: 10.1242/jcs.63.1.135
Husemann, M., Sadílek, D., Dey, L.-S., Hawlitschek, O., and Seidel, M. (2021). New genome size estimates for band-winged and slant-faced grasshoppers (Orthoptera: Acrididae: Oedipodinae, Gomphocerinae) reveal the so far largest measured insect genome. Caryol. Int. J. Cytol. Cytosyst. Cytogenet. 73, 111–120. doi: 10.13128/CARYOLOGIA-966
Johnson, B. B., Searle, J. B., and Sparks, J. P. (2021). Genome size influences adaptive plasticity of water loss, but not metabolic rates, in lungless salamanders. J. Exp. Biol. 224:jeb242196. doi: 10.1242/jeb.242196
Jombart, T., and Dray, S. (2010). adephylo: exploratory analyses for the phylogenetic comparative method. Bioinformatics 26, 1–21. doi: 10.1093/bioinformatics/btq292
Jühling, F., Pütz, J., Bernt, M., Donath, A., Middendorf, M., Florentz, C., et al. (2011). Improved systematic tRNA gene annotation allows new insights into the evolution of mitochondrial tRNA structures and into the mechanisms of mitochondrial genome rearrangements. Nucleic Acids Res. 40, 2833–2845. doi: 10.1093/nar/gkr1131
Kellogg, E. A., and Bennetzen, J. L. (2004). The evolution of nuclear genome structure in seed plants. Am. J. Bot. 91, 1709–1725. doi: 10.3732/ajb.91.10.1709
Kozłowski, J., Konarzewski, M., and Gawelczyk, A. (2003). Cell size as a link between noncoding DNA and metabolic rate scaling. Proc. Natl. Acad. Sci. U.S.A. 100, 14080–14085. doi: 10.1073/pnas.2334605100
Kraaijeveld, K. (2010). Genome size and species diversification. Evol. Biol. 37, 227–233. doi: 10.1007/s11692-010-9093-4
Kumar, S., Stecher, G., and Tamura, K. (2016). MEGA7: molecular evolutionary genetics analysis version 7.0 for bigger datasets. Mol. Biol. Evol. 33, 1870–1874. doi: 10.1093/molbev/msw054
Laiolo, P., Illera, J. C., and Obeso, J. R. (2013). Local climate determines intra-and interspecific variation in sexual size dimorphism in mountain grasshopper communities. J. Evol. Biol. 26, 2171–2183. doi: 10.1111/jeb.12213
Lanfear, R., Calcott, B., Ho, S. Y., and Guindon, S. (2012). PartitionFinder: combined selection of partitioning schemes and substitution models for phylogenetic analyses. Mol. Biol. Evol. 29, 1695–1701. doi: 10.1093/molbev/mss020
Li, J., Chen, Q., Wen, M., Wang, J., Wang, Y., and Ren, B. (2019). Phylogeny and acoustic signal evolution of a pure tone song katydid Pseudophyllus titan (Orthoptera: Tettigoniidae) based on the complete mitogenome. Mitochondrial DNA Part A 30, 385–396. doi: 10.1080/24701394.2018.1502280
Li, Z., Tiley, G. P., Galuska, S. R., Reardon, C. R., Kidder, T. I., Rundell, R. J., et al. (2018). Multiple large-scale gene and genome duplications during the evolution of hexapods. Proc. Natl. Acad. Sci. U.S.A. 115, 4713–4718. doi: 10.1073/pnas.1710791115
Liu, G., Chang, Z., Chen, L., He, J., Dong, Z., Yang, J., et al. (2020). Genome size variation in butterflies (Insecta, Lepidotera, Papilionoidea): a thorough phylogenetic comparison. Syst. Entomol. 45, 571–582. doi: 10.1111/syen.12417
Lower, S. S., Johnston, J. S., Stanger-Hall, K. F., Hjelmen, C. E., Hanrahan, S. J., Korunes, K., et al. (2017). Genome size in North American fireflies: substantial variation likely driven by neutral processes. Genome Biol. Evol. 9, 1499–1512. doi: 10.1093/gbe/evx097
Ma, W., Xu, L., Hua, H., Chen, M., Guo, M., He, K., et al. (2021). Chromosomal-level genomes of three rice planthoppers provide new insights into sex chromosome evolution. Mol. Ecol. Resour. 21, 226–237. doi: 10.1111/1755-0998.13242
Maddison, W. P., and Maddison, D. R. (2015). Mesquite: a modular system for evolutionary analysis. Evolution. 62, 1103–1118.
Mao, Y., Zhang, N., Nie, Y., Zhang, X., Li, X., and Huang, Y. (2020). Genome size of 17 species from Caelifera (Orthoptera) and determination of internal standards with very large genome size in insecta. Front. Physiol. 11:1321. doi: 10.3389/fphys.2020.567125
Meng, G., Li, Y., Yang, C., and Liu, S. (2019). MitoZ: a toolkit for animal mitochondrial genome assembly, annotation and visualization. Nucleic Acids Res. 47, e63–e63. doi: 10.1093/nar/gkz173
Mirsky, A., and Ris, H. (1951). The desoxyribonucleic acid content of animal cells and its evolutionary significance. J. Gen. Physiol. 34:451. doi: 10.1085/jgp.34.4.451
Mueller, R. L. (2017). piRNAs and evolutionary trajectories in genome size and content. J. Mol. Evol. 85, 169–171. doi: 10.1007/s00239-017-9818-4
Mugleston, J. D., Naegle, M., Song, H., and Whiting, M. F. (2018). A comprehensive phylogeny of Tettigoniidae (Orthoptera: Ensifera) reveals extensive ecomorph convergence and widespread taxonomic incongruence. Insect Syst. Divers. 2:5. doi: 10.1093/isd/ixy010
Nawrocki, E. P., and Eddy, S. R. (2013). Infernal 1.1: 100-fold faster RNA homology searches. Bioinformatics 29, 2933–2935. doi: 10.1093/bioinformatics/btt509
Orel, N., and Puchta, H. (2003). Differences in the processing of DNA ends in Arabidopsis thaliana and tobacco: possible implications for genome evolution. Plant Mol. Biol. 51, 523–531. doi: 10.1023/A:1022324205661
Organ, C. L., Shedlock, A. M., Meade, A., Pagel, M., and Edwards, S. V. (2007). Origin of avian genome size and structure in non-avian dinosaurs. Nature 446, 180–184. doi: 10.1038/nature05621
Orme, D., Freckleton, R., Thomas, G., Petzoldt, T., Fritz, S., and Isaac, N. (2013). The Caper Package: Comparative Analysis of Phylogenetics and Evolution in R. 1–36. Available online at: http://caper.r-forge.r-project.org/
Pagel, M. (1999). Inferring the historical patterns of biological evolution. Nature 401, 877–884. doi: 10.1038/44766
Pagel, M., Meade, A., and Barker, D. (2004). Bayesian estimation of ancestral character states on phylogenies. Syst. Biol. 53, 673–684. doi: 10.1080/10635150490522232
Petrov, D. A. (2001). Evolution of genome size: new approaches to an old problem. Trends Genet. 17, 23–28. doi: 10.1016/S0168-9525(00)02157-0
Piton, L. E. (1940). Paléontologie du gisement éocéne de Menât (Puy-de-Dôme), flore et faune. Mémoire de la Société d'Histoire Naturelle d'Auvergne, 1, 1–303.
Rambaut, A., and Drummond, A. (2002). TreeAnnotator v1. 7.5; MCMC Output Analysis. Edinburgh: Institute of Evolutionary Biology, University of Edinburgh.
Rambaut, A., and Drummond, A. (2009). TRACER: MCMC Trace Analysis Tool, Version 1. 5.0. Available online at: http://tree.bio.ed.ac.uk/software/tracer/ (accessed October 20, 2009).
Reeves, G., Francis, D., Davies, M. S., Rogers, H. J., and Hodkinson, T. R. (1998). Genome size is negatively correlated with altitude in natural populations of Dactylis glomerata. Ann. Bot. 82, 99–105. doi: 10.1006/anbo.1998.0751
Revell, L. J. (2012). phytools: an R package for phylogenetic comparative biology (and other things). Methods Ecol. Evol. 3, 217–223. doi: 10.1111/j.2041-210X.2011.00169.x
Ronquist, F., Teslenko, M., Van Der Mark, P., Ayres, D. L., Darling, A., Höhna, S., et al. (2012). MrBayes 3.2: efficient Bayesian phylogenetic inference and model choice across a large model space. Syst. Biol. 61, 539–542. doi: 10.1093/sysbio/sys029
Ruiz-Ruano, F. J., Castillo-Martínez, J., Cabrero, J., Gómez, R., Camacho, J. P. M., and López-León, M. D. (2018). High-throughput analysis of satellite DNA in the grasshopper Pyrgomorpha conica reveals abundance of homologous and heterologous higher-order repeats. Chromosoma 127, 323–340. doi: 10.1007/s00412-018-0666-9
Ryan Gregory, T., Nedvěd, O., and Adamowicz, S. J. (2003). C-value estimates for 31 species of ladybird beetles (Coleoptera: Coccinellidae). Hereditas 139, 121–127. doi: 10.1111/j.1601-5223.2003.01766.x
Schielzeth, H., Streitner, C., Lampe, U., Franzke, A., and Reinhold, K. (2014). Genome size variation affects song attractiveness in grasshoppers: evidence for sexual selection against large genomes. Evolution 68, 3629–3635. doi: 10.1111/evo.12522
Shah, A., Hoffman, J. I., and Schielzeth, H. (2020). Comparative analysis of genomic repeat content in gomphocerine grasshoppers reveals expansion of satellite DNA and helitrons in species with unusually large genomes. Genome Biol. Evol. 12, 1180–1193. doi: 10.1093/gbe/evaa119
Shirasu, K., Schulman, A. H., Lahaye, T., and Schulze-Lefert, P. (2000). A contiguous 66-kb barley DNA sequence provides evidence for reversible genome expansion. Genome Res. 10, 908–915. doi: 10.1101/gr.10.7.908
Song, H. (2018). Biodiversity of Orthoptera. Insect Biodivers. Sci. Soc. 2, 245–279. doi: 10.1002/9781118945582.ch10
Song, H., Amédégnato, C., Cigliano, M. M., Desutter-Grandcolas, L., Heads, S. W., Huang, Y., et al. (2015). 300 million years of diversification: elucidating the patterns of orthopteran evolution based on comprehensive taxon and gene sampling. Cladistics 31, 621–651. doi: 10.1111/cla.12116
Stamatakis, A. (2014). RAxML version 8: a tool for phylogenetic analysis and post-analysis of large phylogenies. Bioinformatics 30, 1312–1313. doi: 10.1093/bioinformatics/btu033
Suchard, M. A., Lemey, P., Baele, G., Ayres, D. L., Drummond, A. J., and Rambaut, A. (2018). Bayesian phylogenetic and phylodynamic data integration using BEAST 1.10. Virus Evol. 4:vey016. doi: 10.1093/ve/vey016
Swift, H. (1950). The constancy of desoxyribose nucleic acid in plant nuclei. Proc. Natl. Acad. Sci. U.S.A. 36:643. doi: 10.1073/pnas.36.11.643
Vaidya, G., Lohman, D. J., and Meier, R. (2011). SequenceMatrix: concatenation software for the fast assembly of multi-gene datasets with character set and codon information. Cladistics 27, 171–180. doi: 10.1111/j.1096-0031.2010.00329.x
Verlinden, H., Sterck, L., Li, J., Li, Z., Yssel, A., Gansemans, Y., et al. (2020). First draft genome assembly of the desert locust, Schistocerca gregaria. F1000Res. 9:775. doi: 10.12688/f1000research.25148.1
Vicient, C. M., Suoniemi, A., Anamthawat-Jónsson, K., Tanskanen, J., Beharav, A., Nevo, E., et al. (1999). Retrotransposon BARE-1 and its role in genome evolution in the genus Hordeum. Plant Cell 11, 1769–1784. doi: 10.1105/tpc.11.9.1769
Volff, J. (2005). Genome evolution and biodiversity in teleost fish. Heredity 94, 280–294. doi: 10.1038/sj.hdy.6800635
Wang, X., Fang, X., Yang, P., Jiang, X., Jiang, F., Zhao, D., et al. (2014). The locust genome provides insight into swarm formation and long-distance flight. Nat. Commun. 5:2957. doi: 10.1038/ncomms3957
Warchalowska-Sliwa, E. (1998). Karyotype characteristics of katydid orthopterans (Ensifera, Tettigoniidae) and remarks on their evolution at different taxonomic levels. Folia Biol. (Kraków) 46, 143–176.
Wheeler, T. J., and Eddy, S. R. (2013). nhmmer: DNA homology search with profile HMMs. Bioinformatics 29, 2487–2489. doi: 10.1093/bioinformatics/btt403
White, M., and Mclaren, I. (2000). Copepod development rates in relation to genome size and 18S rDNA copy number. Genome 43, 750–755. doi: 10.1139/g00-048
Xie, Y., Wu, G., Tang, J., Luo, R., Patterson, J., Liu, S., et al. (2014). SOAPdenovo-Trans: de novo transcriptome assembly with short RNA-Seq reads. Bioinformatics 30, 1660–1666. doi: 10.1093/bioinformatics/btu077
Yang, C., Li, X., Wang, Q., Yuan, H., Huang, Y., and Xiao, H. (2021). Genome-wide analyses of the relict gull (Larus relictus): insights and evolutionary implications. BMC Genomics 22:311. doi: 10.1186/s12864-021-07616-z
Yu, J., Liu, W., Mai, C., and Liao, W. (2020). Genome size variation is associated with life-history traits in birds. J. Zool. 310, 255–260. doi: 10.1111/jzo.12755
Keywords: Ensifera, genome size, C-value, phylogeny, flow cytometry
Citation: Yuan H, Huang Y, Mao Y, Zhang N, Nie Y, Zhang X, Zhou Y and Mao S (2021) The Evolutionary Patterns of Genome Size in Ensifera (Insecta: Orthoptera). Front. Genet. 12:693541. doi: 10.3389/fgene.2021.693541
Received: 11 April 2021; Accepted: 25 May 2021;
Published: 23 June 2021.
Edited by:
Stephane Boissinot, New York University Abu Dhabi, United Arab EmiratesReviewed by:
Douglas Stewart Glazier, Juniata College, United StatesCopyright © 2021 Yuan, Huang, Mao, Zhang, Nie, Zhang, Zhou and Mao. This is an open-access article distributed under the terms of the Creative Commons Attribution License (CC BY). The use, distribution or reproduction in other forums is permitted, provided the original author(s) and the copyright owner(s) are credited and that the original publication in this journal is cited, in accordance with accepted academic practice. No use, distribution or reproduction is permitted which does not comply with these terms.
*Correspondence: Shaoli Mao, bWFvc2hhb2xpQHhhYi5hYy5jbg==
Disclaimer: All claims expressed in this article are solely those of the authors and do not necessarily represent those of their affiliated organizations, or those of the publisher, the editors and the reviewers. Any product that may be evaluated in this article or claim that may be made by its manufacturer is not guaranteed or endorsed by the publisher.
Research integrity at Frontiers
Learn more about the work of our research integrity team to safeguard the quality of each article we publish.