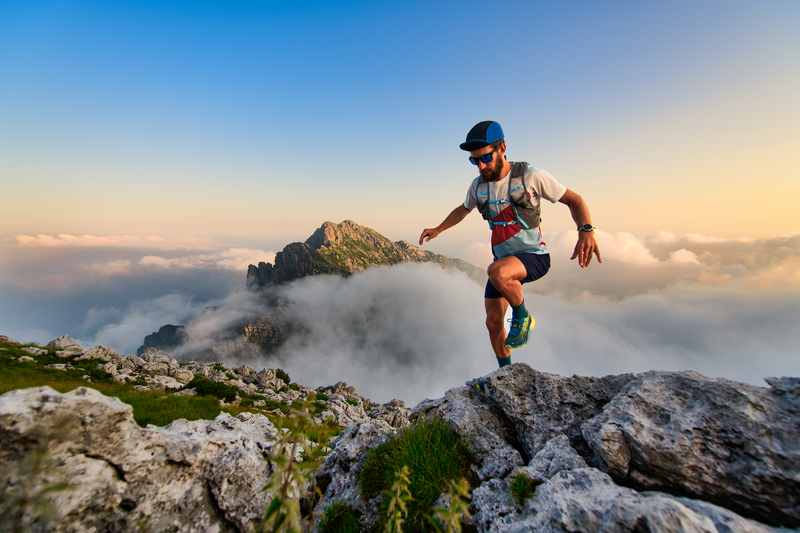
95% of researchers rate our articles as excellent or good
Learn more about the work of our research integrity team to safeguard the quality of each article we publish.
Find out more
REVIEW article
Front. Genet. , 03 May 2021
Sec. Systems Biology Archive
Volume 12 - 2021 | https://doi.org/10.3389/fgene.2021.648524
This article is part of the Research Topic Systems Biology and Omics Approaches to Understand Complex Diseases Biology View all 14 articles
Extracellular vesicles (EVs) are lipid bilayer structures released by organisms from all kingdoms of life. The diverse biogenesis pathways of EVs result in a wide variety of physical properties and functions across different organisms. Fungal EVs were first described in 2007 and different omics approaches have been fundamental to understand their composition, biogenesis, and function. In this review, we discuss the role of omics in elucidating fungal EVs biology. Transcriptomics, proteomics, metabolomics, and lipidomics have each enabled the molecular characterization of fungal EVs, providing evidence that these structures serve a wide array of functions, ranging from key carriers of cell wall biosynthetic machinery to virulence factors. Omics in combination with genetic approaches have been instrumental in determining both biogenesis and cargo loading into EVs. We also discuss how omics technologies are being employed to elucidate the role of EVs in antifungal resistance, disease biomarkers, and their potential use as vaccines. Finally, we review recent advances in analytical technology and multi-omic integration tools, which will help to address key knowledge gaps in EVs biology and translate basic research information into urgently needed clinical applications such as diagnostics, and immuno- and chemotherapies to fungal infections.
Cells secrete a variety of molecules to the extracellular milieu, from the smallest metabolites to large proteins and glycoconjugates. These secreted molecules range from toxic catabolites to cell communication molecules, virulence factors, and enzymes involved in nutrient acquisition. One particularly intriguing mechanism of secretion is the release of extracellular vesicles (EVs). Organisms from all kingdoms have been described to release EVs (Toyofuku et al., 2019; Woith et al., 2019; Rayamajhi and Aryal, 2020; Rizzo et al., 2020b). In fungi, EVs were first described and partially characterized in Cryptococcus neoformans (Rodrigues et al., 2007). Since the original description, different omics approaches have been instrumental for the characterization of EVs, from their putative mechanisms of biogenesis to their function in the fungal biology. In this article, we review the contribution of different omics approaches to study fungal EVs.
Cryptococcus neoformans EVs comprise a heterogeneous population of lipid bilayer vesicles, including pigment-containing, electron-lucid, electron-dense, and membrane-associated electron-dense vesicles. The first proteomic analysis of cryptococcal EVs led to the identification of 76 proteins involved in a variety of functions, including virulence, oxidative stress, unfolded-protein response, cellular metabolism, protein translation, signal transduction, cytoskeleton organization, and also proteins found in the plasma membrane (Rodrigues et al., 2008). Subsequent studies in Histoplasma capsulatum identified 206 EV-associated proteins with a similar diversity of functions to C. neoformans EVs, besides proteins involved in cell synthesis and remodeling (Albuquerque et al., 2008). Lipidomic analysis of H. capsulatum EVs led to the identification of 18 phospholipids, including phosphatidylethanolamine, phosphatidylcholine, and phosphatidylserine species (Albuquerque et al., 2008). These initial characterization of fungal EVs opened new questions about their biogenesis and roles in infection, along with their potential use for clinical and biotechnological applications, as discussed in the subsequent sections.
The precise cellular sites and mechanisms of fungal EVs formation are still not fully defined. At first, there was some skepticism in the field that EVs may be products of dying cells whereby released lipids self-assembled into vesicles. Skepticism about EVs was also fueled by concerns of how such large structures could cross the cell wall, which was viewed as a rigid structure that would preclude vesicular transport. However, we have shown that heat-killed C. neoformans failed to secrete EVs (Rodrigues et al., 2007). With regard to the cell wall transit, recent studies have shown that this structure is easily penetrated by vesicles (Walker et al., 2018). To further investigate this issue, we compared lipidomic analysis data of H. capsulatum EVs (Cleare et al., 2020) and whole cells (Burnet et al., 2020). We found a significant depletion of the energy storage lipid, triacylglycerol, and of the mitochondrial lipid, cardiolipin, in EVs when compared with whole cells (Figure 1). The fact that EVs and whole cells have distinct lipid composition suggests they are formed by specific EVs biogenesis process(es), rather than being a product of cell death, and/or breakdown. Lipidomic analysis of EVs from two Paracoccidioides brasiliensis isolates of different phylogenetic groups showed differences in sterol and fatty acid composition of EVs when compared with whole cells, also suggesting the involvement of specific organelles in EVs biogenesis (Vallejo et al., 2012a). In addition, the deletion of the sterol biosynthesis gene Erg6 induced changes in the lipid and protein content of C. neoformans EVs, suggesting a role for sterols in EVs formation (Oliveira et al., 2020). Studies have shown that fungal EVs can originate from intracellular organelles, such as endosomes (Oliveira et al., 2010; Zarnowski et al., 2018; Zhao et al., 2019; Park et al., 2020), or at the plasma membrane (Rodrigues et al., 2000, 2013; Rizzo et al., 2020a). Morphological studies of C. neoformans showed structures resembling multivesicular bodies (MBVs) that can fuse to the plasma membrane, resulting in the release of intraluminal MBV vesicles into the fungal periplasm (Takeo et al., 1973). Those images suggested that populations of fungal EVs might correspond to exosomes, which are mammalian EVs released to the extracellular milieu by the fusion of MVBs to the plasma membrane (Raposo and Stoorvogel, 2013). This biogenesis pathway was supported by recent studies in C. neoformans (Park et al., 2020) and Candida albicans (Zarnowski et al., 2018), in which deletion of genes affecting MVB formation resulted in aberrant vesicles and/or decreased EVs production. In Saccharomyces cerevisiae, deletion of several regulators of either conventional or unconventional secretion resulted in alterations of EVs composition, as measured by proteomic analysis (Oliveira et al., 2010). In C. neoformans, the deletion of SEC6, a gene participating in the post-Golgi secretory pathway, also resulted in reduced EVs formation (Panepinto et al., 2009). In the filamentous fungus Neurospora crassa, GFP-localization of SEC-6, -5, -8, and -15 subunits of the exocyst complex each form a crescent just beyond the cluster of vesicles of the Spitzenkörper at an extending hyphal tip (Riquelme et al., 2014). The exocyst allows a physical linkage of the vesicle cluster to the apical membrane. The cellular events of these EVs is also a matter of debate, including fusion or vesicle budding and secretion (Rizzoli and Jahn, 2007; Miura and Ueda, 2018). These studies show that intracellular regulators of secretory pathways, such as the post-Golgi pathway, participate in fungal EVs formation, similar to what occurs in mammalian EVs.
Figure 1. Comparative lipidomic analysis of H. capsulatum EVs and whole cells. Lipidomics data from previous publications (Burnet et al., 2020; Cleare et al., 2020) of yeast cells grown in F12 medium. Each lipid class was normalized by the total ion intensity of the identified lipid species. Lipid classes significantly (p ≤ 0.05 by Student’s t-test) enriched in extracellular vesicles and whole cells are shown by asterisks and hash signs, respectively. (A) Lipids quantified by mass spectrometry in the positive ionization mode. (B) Lipids quantified by mass spectrometry in the negative ionization mode.
As observed in protozoan parasites (Marcilla et al., 2014; Szempruch et al., 2016) and mammals (Raposo and Stoorvogel, 2013; Stahl and Raposo, 2019), fungal EVs can also be formed at the plasma membrane. Immunofluorescence of C. neoformans surface lipids revealed plasma membrane projections, suggesting that the plasma membrane could bud and release EVs (Rodrigues et al., 2000). The participation of the plasma membrane in fungal EVs formation was also shown using “wall-less” Aspergillus fumigatus cells (Rizzo et al., 2020a). Ultra-resolution microscopy analyses of these fungal protoplasts demonstrated the occurrence of EVs budding from the plasma membrane. Shedding of these plasma membrane-derived EVs was increased during cell wall synthesis, suggesting their participation in this process. Accordingly, protoplast EVs contain cell-wall polysaccharides and polysaccharide synthases, which are plasma membrane-associated enzymes (Rizzo et al., 2020a). These morphological and compositional studies support the presence of plasma membrane-derived EVs in fungi similar to the mammalian microvesicles (or ectosomes; Raposo and Stoorvogel, 2013; Stahl and Raposo, 2019). However, additional mechanisms of plasma membrane-derived EVs formation that differ from those described for mammalian microvesicles have been described in fungi. In S. cerevisiae, electron tomography studies revealed deep invaginations of the plasma membrane organized as two parallel membranes extending a few hundred nanometers toward the cell center. These structures can curve back to the cell surface, resulting in fusion with the plasma membrane and EVs formation (Rodrigues et al., 2013). Based on these observations, it has been proposed that fungal EVs might also originate from cytoplasmic content loading into a vesicle derived from the reshaping of the plasma membrane. The mechanisms behind this process are currently unknown, but they could represent a new pathway of EVs formation.
Overall, the studies described above show that fungi release exosome-like and microvesicle-like EVs, suggesting a conserved mechanism of EVs formation in lower and higher eukaryotes.
The cell wall is responsible for shaping fungal cells and for their resistance to diverse types of stress (Nimrichter et al., 2016). Nutrient availability, ambient pH, temperature, osmotic stressors, and other extracellular stimuli can lead to cell wall remodeling, which includes structural changes in their major components such as chitin, glucan, and glycoproteins (Nimrichter et al., 2016). The interplay between rigidity and plasticity of the cell wall is a key factor for fungal adaptation, survival, growth, and virulence (Nimrichter et al., 2016; Beauvais and Latgé, 2018). Although the cell wall synthesis and shaping are classically attributed to plasma membrane proteins, EVs might also play a role in this process (Vallejo et al., 2012b; Nimrichter et al., 2016; Ikeda et al., 2018; Zhao et al., 2019; Dawson et al., 2020). In P. brasiliensis, 60% of the non-covalently bound cell wall proteins, detected by proteomic analysis of two distinct isolates, have been described in fungal EVs (Longo et al., 2014). The EVs content can vary deeply depending on the growth conditions and fungal species (Vallejo et al., 2012a,b; Longo et al., 2014; Peres et al., 2015; Alves et al., 2019; Peres da Silva et al., 2019; Cleare et al., 2020). In addition, enrichment of cell wall remodeling enzymes is a conserved feature across fungal EVs (Vallejo et al., 2012b; Longo et al., 2014; Ikeda et al., 2018; Zhao et al., 2019; Dawson et al., 2020). Cell wall synthases or hydrolases have been found in EVs from Candida auris, C. albicans, Cryptococcus deneoformans, Cryptococcus deuterogattii, C. neoformans, Fusarium oxysporum, P. brasiliensis, Sporothrix brasiliensis, S. cerevisiae, H. capsulatum, and Trichoderma reesei (Vallejo et al., 2012b; Wolf et al., 2014; Ikeda et al., 2018; Bleackley et al., 2019; de Paula et al., 2019; Konečná et al., 2019; Zhao et al., 2019; Cleare et al., 2020; Dawson et al., 2020; Zamith-Miranda et al., 2020; Rizzo et al., 2020c). When we compared the proteomic data of H. capsulatum EVs (Cleare et al., 2020) with whole cells (Burnet et al., 2020), the results showed that EVs were highly enriched in cell wall synthases and hydrolases, such as β-glucanase, β-1,3-glucanosyltransferase, chitin synthase, and chitinase (Figure 2). In S. cerevisiae, supplementation with chitin synthase Chs3-enriched EVs rescued the growth of cells treated with the cell wall-targeting antifungal caspofungin, suggesting that the EVs cargo per se is sufficient to supply components for cell remodeling (Zhao et al., 2019). Enzymes that have already been described in fungal EVs are involved in evasion of the immune system by modifying cell wall epitopes. The presence of lactate or exposure to hypoxia induced β-1,3-glucan masking in C. albicans. This effect was mediated by the secreted exo-β-1,3-glucanase, Xog1, which has been described as an EVs cargo in proteomic studies (Nimrichter et al., 2016; Konečná et al., 2019; Childers et al., 2020; Dawson et al., 2020). However, the direct participation of EVs in this process still needs to be confirmed. The ability to actively secrete cell wall synthases and hydrolases through EVs in response to extracellular environmental signals could represent a new mechanism of cell wall remodeling, which could affect the exposure of epitopes during infection, consequently resulting in modulation of the immune response.
Figure 2. Comparative proteomic analysis of Histoplasma capsulatum extracellular vesicles and whole cells. Proteomics data from previous publications (Burnet et al., 2020; Cleare et al., 2020) of yeast cells grown in F12 medium. The relative copy number of proteins in cells or extracellular vesicles was calculated using intensity based absolute quantification (iBAQ) by normalizing to the total iBAQ of each sample. Proteins significantly (p ≤ 0.05 by Student’s t-test) enriched in extracellular vesicles (A) and whole cells (B) are indicated as asterisk and hash signs, respectively.
A seminal work by Andes laboratory showed that EVs were involved in biofilm formation in C. albicans (Zarnowski et al., 2018). Defective biofilm formation leads to an increased susceptibility to fluconazole. The authors performed gain-of-function experiments and showed that addition of wild-type EVs to biofilm-deficient strains restored biofilm formation and re-established fluconazole resistance. This work is akin to the previously mentioned work of EV-associated Chs3 restoring and rescuing cell wall defects and improving tolerance to antifungals (Zhao et al., 2019). Proteomic analyses performed in both studies showed that EVs carry a myriad of functional enzymes. Interestingly, it is possible that the delivery of a combination of enzymes in EVs allows for higher efficiency in cell wall remodeling.
Omic approaches caused a major impact in deciphering the RNA content carried by EVs, being most of the data available characterized using RNA-seq. In fungi, RNA export via EVs was originally described in S. cerevisiae, C. albicans, C. neoformans, and P. brasiliensis (Peres et al., 2015). Similar to what has been published for mammalian EVs, the fungal EVs transcripts were composed mainly of small RNA (sRNA) sequences of up to 250 nt. The most abundant classes were non-coding (nc)RNA sequences of the small nucleolar RNA (snoRNAs), small nuclear RNA (snRNAs), and tRNA types (Peres et al., 2015). Subsequent transcriptomics analysis of EVs from H. capsulatum (Alves et al., 2019) and Paracoccidioides (Peres da Silva et al., 2019) isolates revealed that the small ncRNAs were mostly represented by short 25-nt fragments that aligned to a specific region of a particular mRNA. The presence of anti-sense RNA fragments in EVs might have a role in gene silencing, maybe similarly to that of micro RNAs (miRNAs) and fungal exonic short interfering RNAs (Nicolás and Ruiz-Vázquez, 2013; Son et al., 2017). The fungus Malassezia sympodialis, a member of the human skin microbiota, also exports EVs containing 16 to 22 nucleotides long RNAs. However, M. sympodialis lacks an RNAi machinery, suggesting that this fungal species might bear an alternative miRNA production pathway (Rayner et al., 2017). In the dimorphic fungus Pichia fermentans, the length of EVs RNAs ranges from 25 to 130 nt. These transcripts are involved in the transition of this fungus from yeast to pseudohyphal morphology, which occurs in response to specific environmental conditions (Leone et al., 2018).
Although most of the fungal EVs transcripts were small ncRNAs, full-length mRNAs have also been found. In general, they corresponded to genes of metabolic pathways, transcription regulation, cell cycle, vesicle-mediated transport, cellular responses to stress, and translation, depending on the species and the species isolate studied (Peres et al., 2015; Alves et al., 2019; Peres da Silva et al., 2019). An in vitro translation experiment has shown that mRNAs carried by P. brasiliensis and P. lutzii EVs are functional (Peres da Silva et al., 2019). Based on these results, it is reasonable to speculate that EVs mRNAs can be transferred and translated into the host cell, possibly modulating gene expression that could benefit the pathogen infection and survival. In Cryptococcus gattii, the EVs derived from a virulent strain induced, inside macrophages, survival and proliferation of a less virulent strain, which would normally be cleared by the host cell. This phenotype was decreased by EVs pre-treatment with RNase, supporting a role for EV-associated RNAs in the transfer of virulence traits (Bielska et al., 2018). However, the nature of these RNAs and their mechanism of action still needs to be further investigated.
Regarding RNA loading into EVs, in mammals, the autophagy protein LC3 has been reported as a recruiter of RNA-binding proteins to these compartments (Leidal et al., 2020). In fungi, the composition of RNA in the EVs can be affected by alterations in the intracellular vesicle and secretion pathway. The knockout of Golgi reassembly and stacking protein in C. neoformans deeply affected the EVs RNA composition, suggesting a role of the Golgi in the EVs RNA loading (Peres et al., 2018). To further evaluate the existence of a specific mechanism of RNA loading into fungal EVs, we compared the published transcriptomics data of H. capsulatum EVs with that of the whole cell (Alves et al., 2019). We observed a striking enrichment of specific RNA sequences in EVs, while the most expressed RNAs in the cells were present only in trace amounts in EVs (Figure 3). These results support the hypothesis that RNA sorting to EVs is finely regulated. In addition, robust RNA-seq data comparing the transcriptomics of EVs with that of their corresponding C. albicans cells cultivated both under control and mild stress conditions. We observed that the EVs and the cell transcriptomics was distinct in all growth conditions and that the RNA content of both EVs and cells was modulated under the stress conditions analyzed (Leitão, 2017).
Figure 3. Comparative transcriptomic analysis of RNA content H. capsulatum extracellular vesicles and whole cells. The expression level is represented as transcripts per million. Transcripts significantly (p ≤ 0.05 by t-test) enriched in extracellular vesicles (A) and whole cells (B) are shown by asterisk and hash signs, respectively.
While the terms biomarkers and markers are often and inappropriately used interchangeably, they have distinct definitions. Biomarkers, by definition, are molecular signatures that can be used in the clinic to diagnose or predict the appearance or outcome of a disease (Strimbu and Tavel, 2010), whereas cell biology markers are molecules that can differentiate cell populations, cellular processes or cellular compartments (Zhang et al., 2019). The distinct composition of EVs from fungi and human cells make them good candidates for clinical diagnostic biomarkers and/or disease follow-up, i.e., for early assessment of chemotherapeutic outcomes and/or disease progression. Unfortunately, this subject has been understudied in fungi. In parasites, proteins from the murine malarial parasite Plasmodium yoelii have been detected in proteomics analysis of EVs from infected reticulocytes (Martin-Jaular et al., 2011). Similar findings have been recently observed in EVs isolated from plasma of a patient with chronic Chagas disease (Cortes-Serra et al., 2020). The use of EVs as biomarkers has been better explored in cancer biology. For instance, in prostate cancer, urinary EVs has been shown to carry RNAs that are signature of the disease outcome and are considered promising biomarker candidates (Pang et al., 2020). More recently, the proteomic analysis of EVs and particles from plasma and tissue showed that they can distinguish between normal and cancer cells with >90% sensitivity and specificity (Hoshino et al., 2020).
The discovery of specific markers of fungal EVs would have a major impact in cell biology research toward understanding their biogenesis, traffic, and function. In mammalian cells, good markers are the tetraspanins CD9, CD63, and CD81 (Andreu and Yáñez-Mó, 2014), which are currently unavailable for fungal EVs. In 2012, Vallejo et al. showed that 63% of the P. brasiliensis EVs proteins had orthologs described in EVs of H. capsulatum, C. neoformans, and S. cerevisiae (Vallejo et al., 2012b). Dawson et al. (2020) analyzed proteins that were enriched in EVs from three different strains of C. albicans when compared to the proteome of whole cells from the same strains. They found 47 commonly enriched proteins including Sur7, Evp1, and a variety of cell-wall synthesis and remodeling proteins. It should be noted, however, that the whole cell fraction that the authors analyzed did not include plasma membrane and, therefore, the results should be carefully considered (Dawson et al., 2020). Due to presence of cell-wall synthases and hydrolases in EVs from a variety of species (as discussed above) and since cell wall is conserved across the kingdom Fungi, it is reasonable to speculate that cell-wall synthesis and remodeling proteins could be a common marker for fungal EVs. The validation of these marker candidates and subsequent development of reagents may open new avenues to study the cell biology of fungal EVs, while biomarkers could be used for translational research as new diagnostic and prognostic tools.
Early characterization of EVs from C. neoformans showed the presence of important previously described virulence factors, specifically, glucoronoxylomannan (GXM), melanin, monohexosylceramide, laccase, urease, and phosphatase (Rodrigues et al., 2008; Eisenman et al., 2009). So far, however, only a few studies have investigated the participation of fungal EVs during infection in vertebrates. Using a murine model of cryptococcosis, Huang and colleagues demonstrated that the co-injection of C. neoformans with EVs facilitated the yeast transversal of the blood-brain barrier and enhanced the disease development (Huang et al., 2012). In addition, EVs are associated with a higher fungal burden and an increased lesion diameter in early stages of sporotrichosis caused by S. brasiliensis (Ikeda et al., 2018). Over the last decade, a number of EVs proteomic analysis carried out in diverse pathogenic fungal species described the finding of proteins that are associated with fungal virulence, but the association between effect and EVs cargo is still speculative, due to the lack of appropriate molecular tools, especially genetically deficient strains for most fungal species and pharmacological inhibitors. These EV-associated proteins include hydrolytic enzymes involved in protein and lipid degradation, proteins that protect against host oxidative responses and other types of stress (Table 1).
A combination of virulence factors is loaded in EVs from Candida species, including aspartyl proteases (SAPs), adhesion molecules, and lipases (Gil-Bona et al., 2015; Vargas et al., 2015; Karkowska-Kuleta et al., 2020; Martínez-López et al., 2020; Zamith-Miranda et al., 2020). In P. brasiliensis, six previously characterized virulence factors were detected in EVs (Vallejo et al., 2012b): gp43 (Torres et al., 2013), 14-3-3 (Marcos et al., 2016), catalase (Tamayo et al., 2017), cytochrome C peroxidase (Parente-Rocha et al., 2015), superoxide dismutase (Tamayo et al., 2016), and PbCDC42 (Almeida et al., 2009). In C. neoformans, proteomic studies revealed the presence of antioxidant enzymes such as catalase, superoxide dismutase, thioredoxin, thioredoxin reductase, and thiol-specific antioxidant protein (Rodrigues et al., 2008). Antioxidant proteins were found in H. capsulatum EVs, including catalase B, superoxide dismutase, and a thiol-specific antioxidant protein (Albuquerque et al., 2008), and A. fumigatus, of which Asp F3 and a putative thioredoxin reductase were found (Souza et al., 2019). Rodrigues and colleagues confirmed the urease and laccase activities in EVs released by C. neoformans (Rodrigues et al., 2008). Urease improves the survival of C. neoformans inside macrophages by modulating the phagosomal pH (Fu et al., 2018). EVs urease seems to be relevant during brain invasion (Huang et al., 2012). Laccase promotes pathogenesis of cryptococcal infections via multiple pathways: (1) synthesizing prostaglandins that may suppress local inflammatory responses (Erb-Downward et al., 2008); (2) inducing extrapulmonary dissemination to the brain (Noverr et al., 2004); (3) inhibiting the Th17-type cytokine response and neutrophils recruitment (Hansakon et al., 2020); (4) enhancing fungal survival in macrophages by mediating its escape through non-lytic exocytosis (De Oliveira Frazão et al., 2020); and (5) catalyzing the synthesis of melanin. However, the role in pathogenesis of these virulence factors present in EVs still need to be investigated.
Lipidomic, glycomic, and metabolomic studies of fungal EVs have also led to the identification of potential virulence factors. Lipidomic analysis comparing EVs from two P. brasiliensis isolates with different degrees of virulence showed distinct phospholipid and sterol contents, which might be associated with differential virulence. The more virulent Pb18 isolate had higher ergosterol to brassicasterol ratio than the Pb3 strain (Vallejo et al., 2012a), and considering the function of ergosterol in triggering macrophage pyroptosis it can represent a virulence mechanism (Koselny et al., 2018). EVs from C. albicans and C. auris carry a variety of lysophospholipids (Zamith-Miranda et al., 2020), which might be correlated with expression of phospholipases in these organisms. In fact, lysophosphatidylcholine well-characterized regulators of the host immune response (Soehnlein et al., 2009; Carneiro et al., 2013; Gazos-Lopes et al., 2014) and might have a role in candidiasis virulence. Monohexosylceramides have been found in EVs released by H. capsulatum, P. brasiliensis, C. neoformans, C. albicans, and C. auris (Rodrigues et al., 2007; Vallejo et al., 2012a; Cleare et al., 2020; Zamith-Miranda et al., 2020). Monohexosylceramide has been associated with C. neoformans ability to grow in neutral and basic pH (Rittershaus et al., 2006), and to promote C. albicans infection (Rittershaus et al., 2006). N-acetylsphingosine (also known as C2-ceramide), a regulator of the T-cell function (Menné et al., 2000; Detre et al., 2006), has been reported in EVs from C. auris (Zamith-Miranda et al., 2020) but its function in virulence still need to be investigated.
Peres da Silva et al. (2015) showed that P. brasiliensis and P. lutzii have a polysaccharide (or hydrolysis fragments) with glycogen structure and a galactofuranosylmannan oligomer as the main glycans in EVs. Small amounts of 1,3- and 1,6-cell wall glucans were also found. Indeed, β-1,3-glucan is an important cell wall inflammatory pathogen-associated molecular pattern. The study also included glycan and plant/mammalian lectin microarray profiling of EVs surface, revealing the presence of ligands of DC-SIGN receptors, exposed mannose and N-acetylglucosamine residues, and N-acetylglucosamine-binding lectin(s) that can potentially mediate interaction with the host. P. brasiliensis EVs carbohydrate content could indeed be implicated in the transcriptome modulation of murine monocyte-derived dendritic cells (Peres da Silva et al., 2015). A mechanism of fungal resistance to the host defenses by EVs has been shown in C. neoformans by shutting off the host inflammasome. Metabolomic analysis identified that the aromatic metabolite DL-Indole-3-lactic acid is secreted inside EVs, which in turn could impair the inflammasome activation by the host cells (Bürgel et al., 2020).
Overall, omics analyses have found a variety of molecules associated with virulence and regulation of the host immune response. However, how EVs promote virulence with their molecules still needs additional investigations.
The presence of virulence factors and antigens suggests that fungal EVs could modulate the host response to infection. A. flavus and P. brasiliensis EVs enhance the phagocytosis of their respective yeast cells by macrophages (da Silva et al., 2016; Brauer et al., 2020). The EVs also induce the polarization of macrophages toward the proinflammatory M1 phenotype, which has been associated with high antifungal activity (da Silva et al., 2016; Brauer et al., 2020). Similar induction of pro-inflammatory cytokines has also been reported for EVs from C. neoformans, C. albicans, and A. fumigatus (Oliveira et al., 2010; Vargas et al., 2015; Souza et al., 2019). Conversely, EVs can also impair specific host responses. EVs released by M. sympodialis drive the production of the cytokine IL-4 by human peripheral blood mononuclear cells (Gehrmann et al., 2011). It is believed M. sympodialis EVs have a function in allergic responses. Proteomic analysis identified that 10 of 13 previously characterized allergens produced by the fungus are present in EVs. Two of these proteins were enriched in EVs as compared to fungal cells (Johansson et al., 2018).
Host immune factors, such as antibodies, can induce changes in the composition of EVs (Matos Baltazar et al., 2016; Baltazar et al., 2018). Incubation of H. capsulatum yeasts with antibodies against HSP60 (heat-shock protein 60), a protein enriched in cell wall and EVs, significantly changed the EVs cargo (Matos Baltazar et al., 2016; Baltazar et al., 2018). This treatment led to an increase in protein content and the virulence factor urease, suggesting a counteraction of the fungal resistance mechanisms against the host defenses (Matos Baltazar et al., 2016). Moreover, the EVs from antibody-treated H. capsulatum have an inhibitory effect on phagocytosis by macrophages (Baltazar et al., 2018). EVs from S. brasiliensis enhanced phagocytosis of the respective cells and increased the fungal burden in dendritic cells (Ikeda et al., 2018).
As potential targets for immunotherapies, EVs from C. neoformans, C. albicans and A. flavus have been shown to elicit at least a partial protection in the moth Galleria mellonella or in mice (Vargas et al., 2015; Colombo et al., 2019; Brauer et al., 2020; Rizzo et al., 2020c). The EVs cargo seems to be crucial to modulate this response. The presence of GXM and sterylglucosides in EVs reduces the protective effects of EVs in G. mellonella (Colombo et al., 2019). In macrophages, C. neoformans EVs containing GXM trigger a lower antifungal immunological response compared to EVs from a strain with reduced GXM production (Oliveira et al., 2010). C. albicans EVs activate murine macrophages and dendritic cells, inducing a protective immune response in immunosuppressed mice (Vargas et al., 2015; Vargas et al., 2020). Proteomic analysis revealed several candidates that could be involved with this response, including immunogenic proteins MP65 and Bgl2 (Nisini et al., 2001; Gil-Bona et al., 2015). Purified MP65 induces the expression of the antigen presentation protein MHC-II and co-stimulatory molecules, such as CD86, in dendritic cells (Pietrella et al., 2006). Similarly, the endo-β-1,3-glucanase Bgl2 has been tested as a vaccine candidate with promising results (Gil-Bona et al., 2015). These studies highlight the potential of fungal EVs as candidates for vaccine development.
Biomass degradation by fungi is of major importance for agriculture and for biotechnological purposes. In agriculture, fungal infections and degradation of plants can cause major losses, while in biotechnology fungi can be used to convert biomass into biofuels or other bioproducts of economic value (Almeida et al., 2019; Oh and Jin, 2020). F. oxysporum is an environmental fungus that attacks cotton crops leading to significant losses in productivity (Gordon, 2017). F. oxysporum EVs induce damage on leaves from cotton and Nicotiana benthamiana, a close relative of tobacco, but its spores or hyphae do not cause the same damage. This effect, however, is not intrinsic to fungal EVs, since EVs from S. cerevisiae are not phytotoxic (Bleackley et al., 2019). Similarly, the wheat pathogen Zymoseptoria tritici (Hill and Solomon, 2020) produces EVs, which may be a part of its transition from apoplastic, non-symptomatic growth to necrotrophy of wheat. While some carbon-active enzymes associated with EVs were produced under media-grown conditions, additional Zymoseptoria effectors are expected in planta.
In biotechnology, degradation of cellulose from plants releases carbon for biofuel production (Oh and Jin, 2020). Soluble sugars released by fungal enzymes are built into ethanol, lipids, secondary metabolites, or other bioproducts accessible by fungal fermentation. T. reesei is a fungus that produces large amounts of cellulolytic enzymes. The proteomic analysis of EVs produced by this fungus revealed that enzymes with cellulase activity are exported through EVs. The cellulolytic activity of EVs from T. reesei is induced when the fungus is cultivated in the presence of cellulose, suggesting that cargo contained in fungal EVs is altered according to the environment (de Paula et al., 2019). Engineering yeasts for direct cellulosic degradation into ethanol producing yeasts could lead to important gains in biofuel production (Oh and Jin, 2020). In further support of environmental cues regulating EVs, growth conditions of submerged media vs. solid state fermentation (SSF) have shown that Aspergilli produce different secreted protein profiles. A. oryzae (Oda et al., 2006) secreted 4-6x more protein in SSF. A. brasiliensis ATCC9642 in SSF produces several differentially expressed proteins which lack secretion signals, suggesting an alternative route of secretion such as EVs (Volke-Sepulveda et al., 2016). Dissecting the molecular trafficking of EVs formation would create a novel compartment for enzyme delivery, or bioproduct collection from a culture without specialized transport proteins.
The small size and scarce amount of material obtainable in preparations of EVs represent a major analytical challenge. However, advances in omics technologies have immensely improved the sensitivity, throughput, and robustness of the measurements, leading to a more comprehensive characterization of the EVs molecular composition. For instance, back in 2008, EVs proteomic analysis in C. neoformans led to identification of 76 proteins (Rodrigues et al., 2008). Current high-resolution tandem mass spectrometry (HR-MS/MS)-based approaches (Lesur and Domon, 2015), including nanoflow liquid chromatography coupled to HR-MS/MS (nanoLC-HR-MS/MS; Sanders and Edwards, 2020), allow to identify and quantify over 2,000 proteins in fungal EVs (Zhao et al., 2019; Cleare et al., 2020). In this section, we will cover recent technological advances and their current impact and perspectives in analyzing fungal EVs.
The RNA yield recovered from EVs is quite variable when we compare samples from different origins and that have been isolated using distinct protocols. For fungal cells, there are many media and growth conditions that can affect the number of EVs obtained and, consequently, the amount of RNA. Usually, the EVs RNA yield for C. neoformans, C. albicans, P. brasiliensis, and H. capsulatum ranges from 1 to 15 ng when the EVs are isolated from culture supernatant after two ultracentrifugation steps, corresponding to EVs enrichment and washing (Peres et al., 2015). Growing fungi in solid media, instead liquid cultures, can improve the EV RNA recovery yield to up to 50 ng, as shown for C. neoformans and C. gattii preparations (Reis et al., 2019).
In the recent years, the next-generation sequencing has emerged as a robust tool to resolve the diversity of RNA sequences in EVs. RNA-seq has enabled major advances in the analysis of EVs RNAs, allowing the identification of low input amounts of distinct RNA populations (Kim et al., 2017). Such technology allows the comparison of EVs RNA across samples generated under a variety of experimental designs, such as different growth conditions, stresses, or even interspecies studies (Mateescu et al., 2017; Yeri et al., 2018). Given that small RNAs are highly enriched in EVs, most of the library construction protocols focus on the fractioning of this RNA population. There are many kits available, but most of them follow similar procedures, involving multiple steps for the small RNA purification (Giraldez et al., 2018). Overall, we believe that RNA-seq will have a major impact in identifying EVs RNAs that could have a role in the host-pathogen interaction. Especially those sequences regulating expression of mRNAs in recipient cells and consequently, affecting the host immune response or the fungal pathogenicity.
The eternal challenge of an analytical chemist is to improve sensitivity, precision, and speed of the instrumentation, enabling the accurate analysis of trace amounts of samples in large scale. In this context, the recent developments in single-cell analysis might have a major impact in analysis of EVs as most of the procedures are easily adaptable for analyzing EVs. An important concept is to keep the volumes and contact surfaces as small as possible during the sample preparation and analysis to reduce losses due to contact absorption. Online sample preparations can eliminate losses associated with pipetting and sample transfer. One of such techniques, called SNaPP (simplified nanoproteomics platform for reproducible global proteomics), allows the preparation and analysis of proteomic samples from nanograms of proteins (Huang et al., 2016). SNaPP has been successfully used to analyze Nipah virus-like particles (Johnston et al., 2019), which are secreted structures that share many characteristics with EVs, including size and the presence of a lipid membrane. Therefore, SNaPP has a great potential to be used for preparing EVs samples. Another technique to prepare small scale samples, but that has not yet been applied to study EVs, is the nanoPOTS (nanodroplet processing in one pot for trace samples; Zhu et al., 2018). nanoPOTS uses a microfluidic robot to prepare samples in nanoliter volumes, virtually eliminating any losses associated with absorption of proteins and peptides to the walls of pipettes and tubes. This technique has enabled to identify and quantify up to 2,500 proteins in single-cell proteomics analysis (Tsai et al., 2020), therefore, it might have an impact on analyzing EVs in the future.
Another analytical challenge in EVs studies is to obtain multiple omics measurements from the same samples. Having multiple measurements from the same samples is highly desirable since it decreases variability between datasets and efforts/costs associated with EVs preparation. Solvent phase separation-based extraction of metabolites, lipids, and proteins have been developed (Coman et al., 2016; Nakayasu et al., 2016). While the simultaneous metabolite, protein, lipid extraction (SIMPLEX) approach is based on methyl tert-butyl ether, methanol, and water; the metabolite, protein, and lipid extraction (MPLEx) technique uses a mixture of chloroform, methanol, and water for phase separation. To our knowledge, there are no papers in the literature reporting the analysis of EVs using SIMPLEX to extract the samples, even though its potential has been highlighted in a few review articles (Rosa-Fernandes et al., 2017; Ramirez et al., 2018). MPLEx, on the other hand, has been successfully used to analyze EVs from H. capsulatum, C. auris, and from the Gram-positive bacterium Listeria monocytogenes (Coelho et al., 2019; Cleare et al., 2020; Zamith-Miranda et al., 2020).
When compared to conventional LC-MS/MS, advanced multidimensional analytical platforms such as LC-ion mobility spectrometry (IMS)-MS/MS, which combines liquid chromatography, ion mobility spectrometry, and tandem mass spectrometry, offers improvements in separation peak capacity, dynamic range, number of analytes detected, and quality of mass spectra (Baker et al., 2010; Rainville et al., 2017). Thus, the addition of IMS allows for improved coverage of metabolites, lipids, and proteins in EVs. Ion mobility also allows to separate isobaric molecules and enables detailed characterization of lipid molecular structure, including double-bond location, cis/trans orientation, and sn-positions of alkyl and/or acyl chains, none of which are possible using conventional LC-MS technologies (Zheng et al., 2018). Poad et al. (2018) have previously demonstrated the successful incorporation of the online ozonolysis into the existing LC-IMS-MS/MS instrumentation to enable characterization of double bonds in lipid standards. The IMS dimension significantly improves assignment of the ozonolysis products to their precursor ions.
One important challenge of the metabolomics and lipidomics fields is the reliable identification of molecules. Most of the identifications to date are validated by comparing MS/MS spectra and chromatographic elution profiles to bona fide standards. The inclusion of IMS separations opens a new perspective in the identification of small molecules without standards. The separation in IMS can be predicted with high precision (<5% error; Colby et al., 2019). Therefore, using multiple pieces of information, i.e., high mass accuracy, ion mobility separation, and tandem mass fragmentation might allow the identification of molecules without the need to validate them against standards, which would make metabolomic and lipidomic analyses much faster. A major bottleneck for metabolite identification is the reliance on reference databases that are constructed from authentic reference materials. This approach is highly limiting due to the cost and availability of authentic standards. Both commercial and publicly available reference databases currently only represent a small fraction of the possible metabolites that exist in biological systems (Dobson, 2004). The recent emergence of standards-free methods based on in silico methods such as quantum chemistry, machine learning, and deep learning have enabled accelerated building of very large reference libraries (Colby et al., 2020) with better coverage of the known chemical space, which was not feasible using authentic standards. Standards-free metabolomic approaches can therefore provide comprehensive coverage of the metabolome in EVs analyses, accelerating the discovery of small molecules and improving our understanding of their biological functions.
The low throughput of EVs analysis is a major challenge to conduct clinical studies to assess the EVs potential as a disease biomarker or to better understand their function in human health. One way to increase the throughput of analysis is by multiplexing samples. For proteomic analysis, isotope labeling or isobaric labeling can be used to multiplex samples. In this context, isobaric tags, such as isobaric tags for relative and absolute quantitation (iTRAQ) and tandem mass tag (TMT), allow multiplexing up to 16 samples (Li et al., 2020), thus increasing the speed of sample analysis. Another advantage of multiplexing is the relative increase in sample amount, as compared to analyzing them individually, providing gains in sensitivity. However, such techniques are not available for lipidomic and metabolomic analyses. Therefore, the gain in throughput of such analyses relies on reducing the time needed for the analysis of each sample. Ion mobility spectrometry is not only fast (milliseconds per scan), but also adds another dimension of separation to the LC-MS analysis, which allows to shorten the chromatographic separation time without compromising the depth of coverage. One of such concepts has been developed by the Evosep company (Odense, Denmark). The Evosep chromatographic system performs offline sample solid-phase extraction (SPE) and elution gradient, which is accumulated in a sample loop. During the analysis, the flow passes through the loop carrying the samples to an analytical column and subsequently to the mass spectrometer. This process eliminates the time needed for column regeneration and re-equilibration, consequently increasing the number of samples that can be analyzed in a day. The Evosep system coupled to IMS-MS allows to analyze up to 60 samples a day with a coverage that exceeds 5,000 proteins or more than 1,000 proteins with 5-min separation gradients (Meier et al., 2018; Bekker-Jensen et al., 2020). For the analysis of lipids and metabolites, due to the overall lower intrinsic complexity of their structures and MS fragmentation profile, as compared to peptides, it is possible to even reduce the time needed for the analysis each sample by an IMS-MS-based approach, as recently proposed by Zhang et al. (2016). These authors used an automated SPE platform coupled to IMS-MS to analyze metabolites and xenobiotics in the human urine (Zhang et al., 2016). They employed six SPE columns with a broad range of chemical properties that allowed them to capture distinct sets of molecules. In this configuration, each sample is run 6 times, or 12 times if analyzed in both positive and negative modes, but each cycle only takes 10 s. Therefore, each sample only takes about 2 min to be analyzed, allowing the analysis of hundreds of samples a day.
The combination of multiple omics measurements allows to obtain a much deeper view of the EVs composition. Despite the challenges associated with processing large amounts of data, there are excellent tools to handle such tasks, which we will not cover in this review. Integrating the results of each omics measurement provides an opportunity to understand much deeper details and the processes occurring in EVs and cells. Despite some interpretations might be limited in EVs, the integration of proteomics with metabolomics and lipidomics, for instance, might show consistent changes in the levels of enzymes, substrates, and products. We have applied this approach to study whole cells of the multidrug-resistant fungus C. auris, which showed consistent changes in the levels of enzymes with their respective lipid and metabolite products in drug-resistant strains (Zamith-Miranda et al., 2019). Further integration of such data with RNA-seq results may further provide insights into the transcriptional and post-transcriptional regulation of genes. Nematode parasites, for instance, secrete miRNAs via EVs that target and modulate the expression of host immune factors (Buck et al., 2014).
Omic approaches have been contributing to the field of fungal EVs since the initial characterization of these extracellular “organelles.” Proteomic, lipidomic, metabolomic and RNA-seq technologies enabled a detailed characterization of the molecular composition of the fungal EVs, bringing new insights into their biogenesis, and biological and pathophysiological functions. Multiple omics analyses of mutant strains defective in different secretory pathway components have shown the participation of the Golgi complex in EVs biogenesis. In terms of EVs functions, omics analyses have played a key role in showing the participation of EVs in cell-wall remodeling and downstream function in antifungal resistance. Fungal EVs have also been shown to carry a variety of virulence factors, which opens new perspectives on how they are delivered to and interact with the host. Here are some major knowledge gaps in the field and how omics can contribute to close such gaps:
• Biogenesis and EVs populations. Despite numerous efforts of the field, the question regarding different EVs populations, their composition, and biogenesis processes is still open. Novel EVs purification techniques, such as differential centrifugation and affinity purification, might allow to separate different EVs populations, which in combination with genetics can lead to the identification of biogenesis pathways. Omics analyses can contribute by comprehensively characterizing the composition of different EVs populations.
• Markers and biomarkers. The absence of markers and biomarkers is a major impairment to perform cell biology and clinical studies on EVs, respectively. Ideally, would be to perform omics analysis of dozens of fungal species to identify EVs markers, whereas for developing clinically relevant biomarkers it often requires the analysis of hundreds to thousands of samples from multiple cohorts. Therefore, faster and more sensitive techniques will empower such studies.
• Mechanisms of virulence. Omics analysis will continue to detect or identify new virulence factors and their mechanisms. A major bottleneck is to study their mechanisms of action in host cells and animal models. We believe that techniques, such as co-affinity purification, followed by nanoLC-HR-MS/MS or an orthogonal analytical approach such as IMS-MS/MS, may have a pivotal role in identifying targets of virulence factors in the host cells, leading to a better understanding of the pathogenic mechanisms.
• Structure-function relationship of fungal EVs molecules. Structure-function relationship is another major gap in fungal EVs research. Thus far, most aforementioned studies that have identified fungal EVs molecules by proteomic, lipidomic, transcriptomic, and other omic approaches are descriptive in nature. In the coming years, investigators in the field should make greater strides to study the structure-function relationship of some of these fungal molecules, particularly those that have known or potential bioactivity, based on published data on fungi or other pathogen(s). This would require considerable improvement in (a) gene expression and knockout techniques for fungi; (b) purification and structural analysis of fungal molecules; and (c) chemical and/or enzymatic synthesis of fungus-specific molecular targets such as lipids, glycoconjugates, and metabolites.
Technological advances and Science are highly dependent on each other to progress, which is not different for EVs biology and omic analyses. We foresee that advances in omic technologies will continue having major impact in studying EVs biology.
All authors contributed to the literature review, writing of the manuscript and revision, and editing of the manuscript. All authors revised and approved the final version of the manuscript.
DZ-M, JN, and EN were supported by NIH R21 AI124797. EB and EN were supported by a Laboratory Directed Research and Development project from Pacific Northwest National Laboratory (PNNL). AC was supported in part by NIH grants AI052733, AI15207, and HL059842. We were also very grateful to the Biomolecule Analysis and Omics Unit (formerly, Biomolecule Analysis Core Facility), supported by the NIH/NIMHD grants 2G12MD007592-21 and 5U54MD007592 (to Robert A. Kirken), for the access to mass spectrometry systems and other analytical instruments used in several of the studies described here. IA was partially supported by NIH/NIMHD grant 5U54MD007592, and a Special Visiting Researcher of the CNPq/Science Without Borders Science Program, Brazil. EC and AC were funded by the Johns Hopkins Malaria Research Institute Pilot Grant Casadevall_123. RPu was supported by the Brazilian funding agencies FAPESP, CAPES, and CNPq. RPe and CC were funded by Medical Research Council Centre for Medical Mycology at University of Exeter (MR/N006364/2). Parts of this work were performed in the Environmental Molecular Science Laboratory, a United States Department of Energy (DOE) national scientific user facility at PNNL in Richland, WA, United States. EC and AC are funded by NIAID R01 AI052733. EN was also supported by R01 AI127465 from NIAID.
The authors declare that the research was conducted in the absence of any commercial or financial relationships that could be construed as a potential conflict of interest.
This review is dedicated to the memory of Luiz Rodolpho Raja Gabaglia Travassos (1938–2020; Universidade Federal de Sao Paulo, Escola Paulista de Medicina, Brazil), who made numerous seminal contributions to the fields of fungal molecular and cellular biology, and glycobiology. Travassos was one the first investigators who proposed extracellular vesicles as carriers of fungal cell wall, plasma membrane, and intracellular bioactive molecules that could eventually modulate mammalian host infection and immune response, and fungal immunoevasion mechanisms.
Albuquerque, P. C., Nakayasu, E. S., Rodrigues, M. L., Frases, S., Casadevall, A., Zancope-Oliveira, R. M., et al. (2008). Vesicular transport in Histoplasma capsulatum: an effective mechanism for trans-cell wall transfer of proteins and lipids in ascomycetes. Cell. Microbiol. 10, 1695–1710. doi: 10.1111/j.1462-5822.2008.01160.x
Almeida, A. J., Cunha, C., Carmona, J. A., Sampaio-Marques, B., Carvalho, A., Malavazi, I., et al. (2009). Cdc42p controls yeast-cell shape and virulence of Paracoccidioides brasiliensis. Fungal Genet. Biol. 46, 919–926. doi: 10.1016/j.fgb.2009.08.004
Almeida, F., Rodrigues, M. L., and Coelho, C. (2019). The still underestimated problem of fungal diseases worldwide. Front. Microbiol. 10:214. doi: 10.3389/fmicb.2019.00214
Alves, L. R., Peres da Silva, R., Sanchez, D. A., Zamith-Miranda, D., Rodrigues, M. L., Goldenberg, S., et al. (2019). Extracellular vesicle-mediated RNA Release in Histoplasma capsulatum. mSphere 4, e00176-19. doi: 10.1128/mSphere.00176-19
Andreu, Z., and Yáñez-Mó, M. (2014). Tetraspanins in extracellular vesicle formation and function. Front. Immunol. 5:442. doi: 10.3389/fimmu.2014.00442
Baker, E. S., Livesay, E. A., Orton, D. J., Moore, R. J., Danielson, W. F. III., Prior, D. C., et al. (2010). An LC-IMS-MS platform providing increased dynamic range for high-throughput proteomic studies. J. Proteome Res. 9, 997–1006. doi: 10.1021/pr900888b
Baltazar, L. M., Zamith-Miranda, D., Burnet, M. C., Choi, H., Nimrichter, L., Nakayasu, E. S., et al. (2018). Concentration-dependent protein loading of extracellular vesicles released by Histoplasma capsulatum after antibody treatment and its modulatory action upon macrophages. Sci. Rep. 8:8065. doi: 10.1038/s41598-018-25665-5
Beauvais, A., and Latgé, J. P. (2018). Special issue: fungal cell wall. J. Fungi 4:91. doi: 10.3390/jof4030091
Bekker-Jensen, D. B., Martínez-Val, A., Steigerwald, S., Rüther, P., Fort, K. L., Arrey, T. N., et al. (2020). A compact quadrupole-orbitrap mass spectrometer with FAIMS interface improves proteome coverage in short LC gradients. Mol. Cell Proteom. 19, 716–729. doi: 10.1074/mcp.TIR119.001906
Bielska, E., Sisquella, M. A., Aldeieg, M., Birch, C., O’Donoghue, E. J., and May, R. C. (2018). Pathogen-derived extracellular vesicles mediate virulence in the fatal human pathogen Cryptococcus gattii. Nat. Commun. 9:1556. doi: 10.1038/s41467-018-03991-6
Bleackley, M. R., Samuel, M., Garcia-Ceron, D., McKenna, J. A., Lowe, R. G. T., Pathan, M., et al. (2019). Extracellular vesicles from the cotton pathogen Fusarium oxysporum f. sp. vasinfectum induce a phytotoxic response in plants. Front. Plant Sci. 10:1610. doi: 10.3389/fpls.2019.01610
Brauer, V. S., Pessoni, A. M., Bitencourt, T. A., de Paula, R. G., de Oliveira Rocha, L., Goldman, G. H., et al. (2020). Extracellular vesicles from Aspergillus flavus Induce M1 polarization in vitro. mSphere 5, e00190-20. doi: 10.1128/mSphere.00190-20
Buck, A. H., Coakley, G., Simbari, F., McSorley, H. J., Quintana, J. F., Le Bihan, T., et al. (2014). Exosomes secreted by nematode parasites transfer small RNAs to mammalian cells and modulate innate immunity. Nat. Commun. 5:5488. doi: 10.1038/ncomms6488
Bürgel, P. H., Marina, C. L., Saavedra, P. H. V., Albuquerque, P., de Oliveira, S. A. M., and Veloso Janior, P. H. H. (2020). Cryptococcus neoformans secretes small molecules that inhibit IL-1β inflammasome-dependent secretion. Mediators Inflamm. 2020:3412763. doi: 10.1155/2020/3412763
Burnet, M. C., Zamith-Miranda, D., Heyman, H. M., Weitz, K. K., Bredeweg, E. L., Nosanchuk, J. D., et al. (2020). Remodeling of the Histoplasma capsulatum membrane induced by monoclonal antibodies. Vaccines 8:269. doi: 10.3390/vaccines8020269
Carneiro, A. B., Iaciura, B. M., Nohara, L. L., Lopes, C. D., Veas, E. M., Mariano, V. S., et al. (2013). Lysophosphatidylcholine triggers TLR2- and TLR4-mediated signaling pathways but counteracts LPS-induced NO synthesis in peritoneal macrophages by inhibiting NF-κB translocation and MAPK/ERK phosphorylation. PLoS One 8:e76233. doi: 10.1371/journal.pone.0076233
Childers, D. S., Avelar, G. M., Bain, J. M., Pradhan, A., Larcombe, D. E., Netea, M. G., et al. (2020). Epitope shaving promotes fungal immune evasion. mBio 11:e00984-20. doi: 10.1128/mBio.00984-20
Cleare, L. G., Zamith, D., Heyman, H. M., Couvillion, S. P., Nimrichter, L., Rodrigues, M. L., et al. (2020). Media matters! alterations in the loading and release of Histoplasma capsulatum extracellular vesicles in response to different nutritional milieus. Cell Microbiol. 22:e13217. doi: 10.1111/cmi.13217
Coelho, C., Brown, L., Maryam, M., Vij, R., Smith, D. F. Q., Burnet, M. C., et al. (2019). Listeria monocytogenes virulence factors, including listeriolysin O, are secreted in biologically active extracellular vesicles. J. Biol. Chem. 294, 1202–1217. doi: 10.1074/jbc.RA118.006472
Colby, S. M., Nuñez, J. R., Hodas, N. O., Corley, C. D., and Renslow, R. R. (2020). Deep learning to generate in silico chemical property libraries and candidate molecules for small molecule identification in complex samples. Anal. Chem. 92, 1720–1729. doi: 10.1021/acs.analchem.9b02348
Colby, S. M., Thomas, D. G., Nuñez, J. R., Baxter, D. J., Glaesemann, K. R., Brown, J. M., et al. (2019). ISiCLE: a quantum chemistry pipeline for establishing in silico collision cross section libraries. Anal. Chem. 91, 4346–4356. doi: 10.1021/acs.analchem.8b04567
Colombo, A. C., Rella, A., Normile, T., Joffe, L. S., Tavares, P. M., Araújo, G. R. S., et al. (2019). Cryptococcus neoformans glucuronoxylomannan and sterylglucoside are required for host protection in an animal vaccination model. mBio 10:e02909-18. doi: 10.1128/mBio.02909-18
Coman, C., Solari, F. A., Hentschel, A., Sickmann, A., Zahedi, R. P., and Ahrends, R. (2016). Simultaneous metabolite, protein, lipid extraction (SIMPLEX): a combinatorial multimolecular omics approach for systems biology. Mol. Cell Proteom. 15, 1453–1466. doi: 10.1074/mcp.M115.053702
Cortes-Serra, N., Mendes, M. T., Mazagatos, C., Segui-Barber, J., Ellis, C. C., Ballart, C., et al. (2020). Plasma-derived extracellular vesicles as potential biomarkers in heart transplant patient with chronic chagas disease. Emerg. Infect. Dis. 26, 1846–1851. doi: 10.3201/eid2608.191042
da Silva, T. A., Roque-Barreira, M. C., Casadevall, A., and Almeida, F. (2016). Extracellular vesicles from Paracoccidioides brasiliensis induced M1 polarization in vitro. Sci. Rep. 6:35867. doi: 10.1038/srep35867
Dawson, C. S., Garcia-Ceron, D., Rajapaksha, H., Faou, P., Bleackley, M. R., and Anderson, M. A. (2020). Protein markers for Candida albicans EVs include claudin-like Sur7 family proteins. J. Extracell. Vesicles 9:1750810. doi: 10.1080/20013078.2020.1750810
De Oliveira Frazão, S., De Sousa, H. R., Silva, L. G. D., Folha, J. D. S., Gorgonha, K. C. M., and Oliveira, G. P. Jr., et al. (2020). Laccase affects the rate of Cryptococcus neoformans nonlytic exocytosis from macrophages. mBio 11:e02085-20. doi: 10.1128/mBio.02085-20
de Paula, R. G., Antoniêto, A. C. C., Nogueira, K. M. V., Ribeiro, L. F. C., Rocha, M. C., Malavazi, I., et al. (2019). Extracellular vesicles carry cellulases in the industrial fungus Trichoderma reesei. Biotechnol. Biofuels 12:146. doi: 10.1186/s13068-019-1487-7
Detre, C., Kiss, E., Varga, Z., Ludányi, K., Pászty, K., Enyedi, A., et al. (2006). Death or survival: membrane ceramide controls the fate and activation of antigen-specific T-cells depending on signal strength and duration. Cell. Signal. 18, 294–306. doi: 10.1016/j.cellsig.2005.05.012
Eisenman, H. C., Frases, S., Nicola, A. M., Rodrigues, M. L., and Casadevall, A. (2009). Vesicle-associated melanization in Cryptococcus neoformans. Microbiology 155(Pt. 12), 3860–3867. doi: 10.1099/mic.0.032854-0
Erb-Downward, J. R., Noggle, R. M., Williamson, P. R., and Huffnagle, G. B. (2008). The role of laccase in prostaglandin production by Cryptococcus neoformans. Mol. Microbiol. 68, 1428–1437. doi: 10.1111/j.1365-2958.2008.06245.x
Fu, M. S., Coelho, C., De Leon-Rodriguez, C. M., Rossi, D. C. P., Camacho, E., Jung, E. H., et al. (2018). Cryptococcus neoformans urease affects the outcome of intracellular pathogenesis by modulating phagolysosomal PH. PLoS Pathog. 14:e1007144. doi: 10.1371/journal.ppat.1007144
Gazos-Lopes, F., Oliveira, M. M., Hoelz, L. V., Vieira, D. P., Marques, A. F., Nakayasu, E. S., et al. (2014). Structural and functional analysis of a platelet-activating lysophosphatidylcholine of Trypanosoma cruzi. PLoS Negl. Trop. Dis. 8:e3077. doi: 10.1371/journal.pntd.0003077
Gehrmann, U., Qazi, K. R., Johansson, C., Hultenby, K., Karlsson, M., Lundeberg, L., et al. (2011). Nanovesicles from Malassezia sympodialis and host exosomes induce cytokine responses – novel mechanisms for host-microbe interactions in atopic eczema. PLoS One 6:e21480. doi: 10.1371/journal.pone.0021480
Gil-Bona, A., Llama-Palacios, A., Parra, C. M., Vivanco, F., Nombela, C., Monteoliva, L., et al. (2015). Proteomics unravels extracellular vesicles as carriers of classical cytoplasmic proteins in Candida albicans. J. Proteome Res. 14, 142–153. doi: 10.1021/pr5007944
Giraldez, M. D., Spengler, R. M., Etheridge, A., Godoy, P. M., Barczak, A. J., Srinivasan, S., et al. (2018). Comprehensive multi-center assessment of small RNA-seq methods for quantitative miRNA profiling. Nat. Biotechnol. 36, 746–757. doi: 10.1038/nbt.4183
Gordon, T. R. (2017). Fusarium oxysporum and the fusarium wilt syndrome. Annu. Rev. Phytopathol. 55, 23–39. doi: 10.1146/annurev-phyto-080615-095919
Hansakon, A., Ngamskulrungroj, P., and Angkasekwinai, P. (2020). Contribution of laccase expression to immune response against Cryptococcus gattii infection. Infect. Immun. 88, e712–e719. doi: 10.1128/iai.00712-19
Hill, E. H., and Solomon, P. S. (2020). Extracellular vesicles from the apoplastic fungal wheat pathogen Zymoseptoria tritici. Fungal Biol. Biotechnol. 7:13. doi: 10.1186/s40694-020-00103-2
Hoshino, A., Kim, H. S., Bojmar, L., Gyan, K. E., Cioffi, M., Hernandez, J., et al. (2020). Extracellular vesicle and particle biomarkers define multiple human cancers. Cell 182, 1044-1061.e18. doi: 10.1016/j.cell.2020.07.009
Huang, E. L., Piehowski, P. D., Orton, D. J., Moore, R. J., Qian, W. J., Casey, C. P., et al. (2016). SNaPP: simplified nanoproteomics platform for reproducible global proteomic analysis of nanogram protein quantities. Endocrinology 157, 1307–1314. doi: 10.1210/en.2015-1821
Huang, S. H., Wu, C. H., Chang, Y. C., Kwon-Chung, K. J., Brown, R. J., and Jong, A. (2012). Cryptococcus neoformans-derived microvesicles enhance the pathogenesis of fungal brain infection. PLoS One 7:e48570. doi: 10.1371/journal.pone.0048570
Ikeda, M. A. K., de Almeida, J. R. F., Jannuzzi, G. P., Cronemberger-Andrade, A., Torrecilhas, A. C. T., Moretti, N. S., et al. (2018). Extracellular vesicles from Sporothrix brasiliensis are an important virulence factor that induce an increase in fungal burden in experimental sporotrichosis. Front. Microbiol. 9:2286. doi: 10.3389/fmicb.2018.02286
Johansson, H. J., Vallhov, H., Holm, T., Gehrmann, U., Andersson, A., Johansson, C., et al. (2018). Extracellular nanovesicles released from the commensal yeast Malassezia sympodialis are enriched in allergens and interact with cells in human skin. Sci. Rep. 8:9182. doi: 10.1038/s41598-018-27451-9
Johnston, G. P., Bradel-Tretheway, B., Piehowski, P. D., Brewer, H. M., Lee, B. N. R., Usher, N. T., et al. (2019). Nipah virus-like particle egress is modulated by cytoskeletal and vesicular trafficking pathways: a validated particle proteomics analysis. mSystems 4, e00194-19. doi: 10.1128/mSystems.00194-19
Karkowska-Kuleta, J., Kulig, K., Karnas, E., Zuba-Surma, E., Woznicka, O., Pyza, E., et al. (2020). Characteristics of extracellular vesicles released by the pathogenic yeast-like fungi Candida glabrata, Candida parapsilosis and Candida tropicalis. Cells 9:1722.
Kim, K. M., Abdelmohsen, K., Mustapic, M., Kapogiannis, D., and Gorospe, M. (2017). RNA in extracellular vesicles. Wiley Interdiscip. Rev. RNA 8:e1413. doi: 10.1002/wrna.1413
Konečná, K., Klimentová, J., Benada, O., Němečková, I., Jand’ourek, O., Jílek, P., et al. (2019). A comparative analysis of protein virulence factors released via extracellular vesicles in two Candida albicans strains cultivated in a nutrient-limited medium. Microb. Pathog. 136:103666. doi: 10.1016/j.micpath.2019.103666
Koselny, K., Mutlu, N., Minard, A. Y., Kumar, A., Krysan, D. J., and Wellington, M. (2018). A Genome-wide screen of deletion mutants in the filamentous Saccharomyces cerevisiae background identifies ergosterol as a direct trigger of macrophage pyroptosis. mBio 9:e01204-18. doi: 10.1128/mBio.01204-18
Leidal, A. M., Huang, H. H., Marsh, T., Solvik, T., Zhang, D., Ye, J., et al. (2020). The LC3-conjugation machinery specifies the loading of RNA-binding proteins into extracellular vesicles. Nat. Cell Biol. 22, 187–199. doi: 10.1038/s41556-019-0450-y
Leitão, N. P. Jr. (2017). Characterization of Extracellular Vesicles Isolated From Pathogenic Fungi Cultivated Under Stress and Their Role in Cell Communication. Ph.D. thesis, Federal University of São Paulo, São Paulo.
Leone, F., Bellani, L., Muccifora, S., Giorgetti, L., Bongioanni, P., Simili, M., et al. (2018). Analysis of extracellular vesicles produced in the biofilm by the dimorphic yeast Pichia fermentans. J. Cell Physiol. 233, 2759–2767. doi: 10.1002/jcp.25885
Lesur, A., and Domon, B. (2015). Advances in high-resolution accurate mass spectrometry application to targeted proteomics. Proteomics 15, 880–890. doi: 10.1002/pmic.201400450
Li, J., Van Vranken, J. G., Pontano Vaites, L., Schweppe, D. K., Huttlin, E. L., Etienne, C., et al. (2020). TMTpro reagents: a set of isobaric labeling mass tags enables simultaneous proteome-wide measurements across 16 samples. Nat. Methods 17, 399–404. doi: 10.1038/s41592-020-0781-4
Longo, L. V. G., da Cunha, J. P. C., Sobreira, T., and Puccia, R. (2014). Proteome of cell wall-extracts from pathogenic Paracoccidioides brasiliensis: comparison among morphological phases, isolates, and reported fungal extracellular vesicle proteins. EuPA Open Proteom. 3, 216–228. doi: 10.1016/j.euprot.2014.03.003
Marcilla, A., Martin-Jaular, L., Trelis, M., de Menezes-Neto, A., Osuna, A., Bernal, D., et al. (2014). Extracellular vesicles in parasitic diseases. J. Extracell. Vesicles 3:25040. doi: 10.3402/jev.v3.25040
Marcos, C. M., Silva, J. F., Oliveira, H. C., Assato, P. A., Singulani, J. L., Lopez, A. M., et al. (2016). Decreased expression of 14-3-3 in Paracoccidioides brasiliensis confirms its involvement in fungal pathogenesis. Virulence 7, 72–84. doi: 10.1080/21505594.2015.1122166
Martínez-López, R., Luisa Hernáez, M., Redondo, E., Calvo, G., Radau, S., Gil, C., et al. (2020). Small extracellular vesicles secreted by Candida albicans hyphae have highly diverse protein cargoes that include virulence factors and stimulate macrophages. bioRxiv [Preprint] doi: 10.1101/2020.10.02.323774
Martin-Jaular, L., Nakayasu, E. S., Ferrer, M., Almeida, I. C., and Del Portillo, H. A. (2011). Exosomes from Plasmodium yoelii-infected reticulocytes protect mice from lethal infections. PLoS One 6:e26588. doi: 10.1371/journal.pone.0026588
Mateescu, B., Kowal, E. J., van Balkom, B. W., Bartel, S., Bhattacharyya, S. N., Buzás, E. I., et al. (2017). Obstacles and opportunities in the functional analysis of extracellular vesicle RNA - an ISEV position paper. J. Extracell. Vesicles 6:1286095. doi: 10.1080/20013078.2017.1286095
Matos Baltazar, L., Nakayasu, E. S., Sobreira, T. J., Choi, H., Casadevall, A., Nimrichter, L., et al. (2016). Antibody binding alters the characteristics and contents of extracellular vesicles released by Histoplasma capsulatum. mSphere 1, e00085-15. doi: 10.1128/mSphere.00085-15
Meier, F., Brunner, A. D., Koch, S., Koch, H., Lubeck, M., Krause, M., et al. (2018). Online parallel accumulation-serial fragmentation (PASEF) with a novel trapped ion mobility mass spectrometer. Mol. Cell Proteom. 17, 2534–2545. doi: 10.1074/mcp.TIR118.000900
Menné, C., Lauritsen, J. P., Dietrich, J., Kastrup, J., Wegener, A. M., Odum, N., et al. (2000). Ceramide-induced TCR up-regulation. J. Immunol. 165, 3065–3072. doi: 10.4049/jimmunol.165.6.3065
Miura, N., and Ueda, M. (2018). Evaluation of unconventional protein secretion by Saccharomyces cerevisiae and other fungi. Cells 7:128. doi: 10.3390/cells7090128
Nakayasu, E. S., Nicora, C. D., Sims, A. C., Burnum-Johnson, K. E., Kim, Y. M., Kyle, J. E., et al. (2016). MPLEx: a robust and universal protocol for single-sample integrative proteomic, metabolomic, and lipidomic analyses. mSystems 1, e00043-16. doi: 10.1128/mSystems.00043-16
Nicolás, F. E., and Ruiz-Vázquez, R. M. (2013). Functional diversity of RNAi-associated sRNAs in fungi. Int. J. Mol. Sci. 14, 15348–15360. doi: 10.3390/ijms140815348
Nimrichter, L., de Souza, M. M., Del Poeta, M., Nosanchuk, J. D., Joffe, L., Tavares, P. M., et al. (2016). Extracellular vesicle-associated transitory cell wall components and their impact on the interaction of fungi with host cells. Front. Microbiol. 7:1034. doi: 10.3389/fmicb.2016.01034
Nisini, R., Romagnoli, G., Gomez, M. J., La Valle, R., Torosantucci, A., Mariotti, S., et al. (2001). Antigenic properties and processing requirements of 65-kilodalton mannoprotein, a major antigen target of anti-Candida human T-cell response, as disclosed by specific human T-cell clones. Infect. Immun. 69, 3728–3736. doi: 10.1128/iai.69.6.3728-3736.2001
Noverr, M. C., Williamson, P. R., Fajardo, R. S., and Huffnagle, G. B. (2004). CNLAC1 is required for extrapulmonary dissemination of Cryptococcus neoformans but not pulmonary persistence. Infect. Immun. 72, 1693–1699. doi: 10.1128/iai.72.3.1693-1699.2004
Oda, K., Kakizono, D., Yamada, O., Iefuji, H., Akita, O., and Iwashita, K. (2006). Proteomic analysis of extracellular proteins from Aspergillus oryzae grown under submerged and solid-state culture conditions. Appl. Environ. Microbiol. 72, 3448–3457. doi: 10.1128/aem.72.5.3448-3457.2006
Oh, E. J., and Jin, Y. S. (2020). Engineering of Saccharomyces cerevisiae for efficient fermentation of cellulose. FEMS Yeast Res. 20:foz089. doi: 10.1093/femsyr/foz089
Oliveira, D. L., Nakayasu, E. S., Joffe, L. S., Guimaraes, A. J., Sobreira, T. J., Nosanchuk, J. D., et al. (2010). Characterization of yeast extracellular vesicles: evidence for the participation of different pathways of cellular traffic in vesicle biogenesis. PLoS One 5:e11113. doi: 10.1371/journal.pone.0011113
Oliveira, F. F. M., Paes, H. C., Peconick, L. D. F., Fonseca, F. L., Marina, C. L. F., Bocca, A. L., et al. (2020). Erg6 affects membrane composition and virulence of the human fungal pathogen Cryptococcus neoformans. Fungal Genet. Biol. 140:103368. doi: 10.1016/j.fgb.2020.103368
Panepinto, J., Komperda, K., Frases, S., Park, Y. D., Djordjevic, J. T., Casadevall, A., et al. (2009). Sec6-dependent sorting of fungal extracellular exosomes and laccase of Cryptococcus neoformans. Mol. Microbiol. 71, 1165–1176. doi: 10.1111/j.1365-2958.2008.06588.x
Pang, B., Zhu, Y., Ni, J., Thompson, J., Malouf, D., Bucci, J., et al. (2020). Extracellular vesicles: the next generation of biomarkers for liquid biopsy-based prostate cancer diagnosis. Theranostics 10, 2309–2326. doi: 10.7150/thno.39486
Parente-Rocha, J. A., Parente, A. F., Baeza, L. C., Bonfim, S. M., Hernandez, O., McEwen, J. G., et al. (2015). Macrophage interaction with Paracoccidioides brasiliensis yeast cells modulates fungal metabolism and generates a response to oxidative stress. PLoS One 10:e0137619. doi: 10.1371/journal.pone.0137619
Park, Y. D., Chen, S. H., Camacho, E., Casadevall, A., and Williamson, P. R. (2020). Role of the ESCRT pathway in laccase trafficking and virulence of Cryptococcus neoformans. Infect. Immun. 88, e00954-19. doi: 10.1128/iai.00954-19
Peres da Silva, R., Heiss, C., Black, I., Azadi, P., Gerlach, J. Q., Travassos, L. R., et al. (2015). vesicles from Paracoccidioides pathogenic species transport polysaccharide and expose ligands for DC-SIGN receptors. Sci. Rep. 5:14213. doi: 10.1038/srep14213
Peres da Silva, R., Longo, L. G. V., Cunha, J. P. C., Sobreira, T. J. P., Rodrigues, M. L., Faoro, H., et al. (2019). Comparison of the RNA content of extracellular vesicles derived from Paracoccidioides brasiliensis and Paracoccidioides lutzii. Cells 8:765. doi: 10.3390/cells8070765
Peres, R., Martins, S. T., Rizzo, J., Dos Reis, F. C. G., Joffe, L. S., Vainstein, M., et al. (2018). Golgi reassembly and stacking protein (GRASP) participates in vesicle-mediated RNA export in Cryptococcus neoformans. Genes 9:400. doi: 10.3390/genes9080400
Peres, R., Puccia, R., Rodrigues, M. L., Oliveira, D. L., Joffe, L. S., Cesar, G. V., et al. (2015). Extracellular vesicle-mediated export of fungal RNA. Sci. Rep. 5:7763. doi: 10.1038/srep07763
Pietrella, D., Bistoni, G., Corbucci, C., Perito, S., and Vecchiarelli, A. (2006). Candida albicans mannoprotein influences the biological function of dendritic cells. Cell Microbiol. 8, 602–612. doi: 10.1111/j.1462-5822.2005.00651.x
Poad, B. L. J., Zheng, X., Mitchell, T. W., Smith, R. D., Baker, E. S., and Blanksby, S. J. (2018). Online Ozonolysis Combined with ion mobility-mass spectrometry provides a new platform for lipid isomer analyses. Anal. Chem. 90, 1292–1300. doi: 10.1021/acs.analchem.7b04091
Rainville, P. D., Wilson, I. D., Nicholson, J. K., Isaac, G., Mullin, L., Langridge, J. I., et al. (2017). Ion mobility spectrometry combined with ultra performance liquid chromatography/mass spectrometry for metabolic phenotyping of urine: effects of column length, gradient duration and ion mobility spectrometry on metabolite detection. Anal. Chim. Acta 982, 1–8. doi: 10.1016/j.aca.2017.06.020
Ramirez, M. I., Amorim, M. G., Gadelha, C., Milic, I., Welsh, J. A., Freitas, V. M., et al. (2018). Technical challenges of working with extracellular vesicles. Nanoscale 10, 881–906. doi: 10.1039/c7nr08360b
Raposo, G., and Stoorvogel, W. (2013). Extracellular vesicles: exosomes, microvesicles, and friends. J. Cell Biol. 200, 373–383. doi: 10.1083/jcb.201211138
Rayamajhi, S., and Aryal, S. (2020). Surface functionalization strategies of extracellular vesicles. J. Mater. Chem. B 8, 4552–4569. doi: 10.1039/d0tb00744g
Rayner, S., Bruhn, S., Vallhov, H., Andersson, A., Billmyre, R. B., and Scheynius, A. (2017). Identification of small RNAs in extracellular vesicles from the commensal yeast Malassezia sympodialis. Sci. Rep. 7:39742. doi: 10.1038/srep39742
Reis, F. C. G., Borges, B. S., Jozefowicz, L. J., Sena, B. A. G., Garcia, A. W. A., Medeiros, L. C., et al. (2019). A Novel protocol for the isolation of fungal extracellular vesicles reveals the participation of a putative scramblase in polysaccharide export and capsule construction in Cryptococcus gattii. mSphere 4, e00080-19. doi: 10.1128/mSphere.00080-19
Riquelme, M., Bredeweg, E. L., Callejas-Negrete, O., Roberson, R. W., Ludwig, S., Beltrán-Aguilar, A., et al. (2014). The Neurospora crassa exocyst complex tethers Spitzenkörper vesicles to the apical plasma membrane during polarized growth. Mol. Biol. Cell 25, 1312–1326. doi: 10.1091/mbc.E13-06-0299
Rittershaus, P. C., Kechichian, T. B., Allegood, J. C., Merrill, A. H. Jr., Hennig, M., Luberto, C., et al. (2006). Glucosylceramide synthase is an essential regulator of pathogenicity of Cryptococcus neoformans. J. Clin. Invest. 116, 1651–1659. doi: 10.1172/JCI27890
Rizzo, J., Chaze, T., Miranda, K., Roberson, R. W., Gorgette, O., Nimrichter, L., et al. (2020a). Characterization of extracellular vesicles produced by Aspergillus fumigatus protoplasts. mSphere 5, e00476-20. doi: 10.1128/mSphere.00476-20
Rizzo, J., Rodrigues, M. L., and Janbon, G. (2020b). Extracellular vesicles in fungi: past, present, and future perspectives. Front. Cell Infect. Microbiol. 10:346. doi: 10.3389/fcimb.2020.00346
Rizzo, J., Wong, S. S. W., Gazi, A. D., Moyrand, F., Chaze, T., Commere, P. H., et al. (2020c). New insights into Cryptococcus extracellular vesicles suggest a new structural model and an antifungal vaccine strategy. bioRxiv [Preprint] doi: 10.1101/2020.08.17.253716
Rizzoli, S. O., and Jahn, R. (2007). Kiss-and-run, collapse and ‘readily retrievable’ vesicles. Traffic 8, 1137–1144. doi: 10.1111/j.1600-0854.2007.00614.x
Rodrigues, M. L., Franzen, A. J., Nimrichter, L., and Miranda, K. (2013). Vesicular mechanisms of traffic of fungal molecules to the extracellular space. Curr. Opin. Microbiol. 16, 414–420. doi: 10.1016/j.mib.2013.04.002
Rodrigues, M. L., Nakayasu, E. S., Oliveira, D. L., Nimrichter, L., Nosanchuk, J. D., Almeida, I. C., et al. (2008). Extracellular vesicles produced by Cryptococcus neoformans contain protein components associated with virulence. Eukaryot. Cell 7, 58–67. doi: 10.1128/EC.00370-07
Rodrigues, M. L., Nimrichter, L., Oliveira, D. L., Frases, S., Miranda, K., Zaragoza, O., et al. (2007). Vesicular polysaccharide export in Cryptococcus neoformans is a eukaryotic solution to the problem of fungal trans-cell wall transport. Eukaryot. Cell 6, 48–59. doi: 10.1128/EC.00318-06
Rodrigues, M. L., Travassos, L. R., Miranda, K. R., Franzen, A. J., Rozental, S., de Souza, W., et al. (2000). Human antibodies against a purified glucosylceramide from Cryptococcus neoformans inhibit cell budding and fungal growth. Infect. Immun. 68, 7049–7060. doi: 10.1128/iai.68.12.7049-7060.2000
Rosa-Fernandes, L., Rocha, V. B., Carregari, V. C., Urbani, A., and Palmisano, G. (2017). A perspective on extracellular vesicles proteomics. Front. Chem. 5:102. doi: 10.3389/fchem.2017.00102
Sanders, K. L., and Edwards, J. L. (2020). Nano-liquid chromatography-mass spectrometry and recent applications in omics investigations. Anal. Methods 12, 4404–4417. doi: 10.1039/d0ay01194k
Soehnlein, O., Lindbom, L., and Weber, C. (2009). Mechanisms underlying neutrophil-mediated monocyte recruitment. Blood 114, 4613–4623. doi: 10.1182/blood-2009-06-221630
Son, H., Park, A. R., Lim, J. Y., Shin, C., and Lee, Y. W. (2017). Genome-wide exonic small interference RNA-mediated gene silencing regulates sexual reproduction in the homothallic fungus Fusarium graminearum. PLoS Genet. 13:e1006595. doi: 10.1371/journal.pgen.1006595
Souza, J. A. M., Baltazar, L. M., Carregal, V. M., Gouveia-Eufrasio, L., Oliveira, A. G. D., Dias, W. G., et al. (2019). Characterization of Aspergillus fumigatus extracellular vesicles and their effects on macrophages and neutrophils functions. Front. Microbiol. 10:2008. doi: 10.3389/fmicb.2019.02008
Stahl, P. D., and Raposo, G. (2019). Extracellular vesicles: exosomes and microvesicles, integrators of homeostasis. Physiology 34, 169–177. doi: 10.1152/physiol.00045.2018
Strimbu, K., and Tavel, J. A. (2010). What are biomarkers? Curr. Opin. HIV AIDS 5, 463–466. doi: 10.1097/COH.0b013e32833ed177
Szempruch, A. J., Dennison, L., Kieft, R., Harrington, J. M., and Hajduk, S. L. (2016). Sending a message: extracellular vesicles of pathogenic protozoan parasites. Nat. Rev. Microbiol. 14, 669–675. doi: 10.1038/nrmicro.2016.110
Takeo, K., Uesaka, I., Uehira, K., and Nishiura, M. (1973). Fine structure of Cryptococcus neoformans grown in vivo as observed by freeze-etching. J. Bacteriol. 113, 1449–1454. doi: 10.1128/jb.113.3.1449-1454.1973
Tamayo, D., Muñoz, J. F., Almeida, A. J., Puerta, J. D., Restrepo, Á, Cuomo, C. A., et al. (2017). Paracoccidioides spp. catalases and their role in antioxidant defense against host defense responses. Fungal Genet. Biol. 100, 22–32. doi: 10.1016/j.fgb.2017.01.005
Tamayo, D., Muñoz, J. F., Lopez, Á, Urán, M., Herrera, J., Borges, C. L., et al. (2016). Identification and Analysis of the role of superoxide dismutases isoforms in the pathogenesis of Paracoccidioides spp. PLoS Negl. Trop. Dis. 10:e0004481. doi: 10.1371/journal.pntd.0004481
Torres, I., Hernandez, O., Tamayo, D., Muñoz, J. F., Leitão, N. P. Jr., García, A. M., et al. (2013). Inhibition of PbGP43 expression may suggest that gp43 is a virulence factor in Paracoccidioides brasiliensis. PLoS One 8:e68434. doi: 10.1371/journal.pone.0068434
Toyofuku, M., Nomura, N., and Eberl, L. (2019). Types and origins of bacterial membrane vesicles. Nat. Rev. Microbiol. 17, 13–24. doi: 10.1038/s41579-018-0112-2
Tsai, C. F., Zhao, R., Williams, S. M., Moore, R. J., Schultz, K., Chrisler, W. B., et al. (2020). An improved boosting to amplify signal with isobaric labeling (iBASIL) strategy for precise quantitative single-cell proteomics. Mol. Cell Proteom. 19, 828–838. doi: 10.1074/mcp.RA119.001857
Vallejo, M. C., Nakayasu, E. S., Longo, L. V., Ganiko, L., Lopes, F. G., Matsuo, A. L., et al. (2012a). Lipidomic analysis of extracellular vesicles from the pathogenic phase of Paracoccidioides brasiliensis. PLoS One 7:e39463. doi: 10.1371/journal.pone.0039463
Vallejo, M. C., Nakayasu, E. S., Matsuo, A. L., Sobreira, T. J., Longo, L. V., Ganiko, L., et al. (2012b). Vesicle and vesicle-free extracellular proteome of Paracoccidioides brasiliensis: comparative analysis with other pathogenic fungi. J. Proteome Res. 11, 1676–1685. doi: 10.1021/pr200872s
Vargas, G., Honorato, L., Guimaraes, A. J., Rodrigues, M. L., Reis, F. C. G., Vale, A. M., et al. (2020). Protective effect of fungal extracellular vesicles against murine candidiasis. Cell. Microbiol. 22:e13238. doi: 10.1111/cmi.13238
Vargas, G., Rocha, J. D., Oliveira, D. L., Albuquerque, P. C., Frases, S., Santos, S. S., et al. (2015). Compositional and immunobiological analyses of extracellular vesicles released by Candida albicans. Cell Microbiol. 17, 389–407. doi: 10.1111/cmi.12374
Volke-Sepulveda, T., Salgado-Bautista, D., Bergmann, C., Wells, L., Gutierrez-Sanchez, G., and Favela-Torres, E. (2016). Secretomic insight into glucose metabolism of Aspergillus brasiliensis in solid-state fermentation. J. Proteome Res. 15, 3856–3871. doi: 10.1021/acs.jproteome.6b00663
Walker, L., Sood, P., Lenardon, M. D., Milne, G., Olson, J., Jensen, G., et al. (2018). The viscoelastic properties of the fungal cell wall allow traffic of ambisome as intact liposome vesicles. mBio 9:e02383-17. doi: 10.1128/mBio.02383-17
Woith, E., Fuhrmann, G., and Melzig, M. F. (2019). Extracellular vesicles-connecting kingdoms. Int. J. Mol. Sci. 20:5695. doi: 10.3390/ijms20225695
Wolf, J. M., Espadas-Moreno, J., Luque-Garcia, J. L., and Casadevall, A. (2014). Interaction of Cryptococcus neoformans extracellular vesicles with the cell wall. Eukaryot. Cell 13, 1484–1493. doi: 10.1128/ec.00111-14
Yeri, A., Courtright, A., Danielson, K., Hutchins, E., Alsop, E., Carlson, E., et al. (2018). Evaluation of commercially available small RNASeq library preparation kits using low input RNA. BMC Genomics 19:331. doi: 10.1186/s12864-018-4726-6
Zamith-Miranda, D., Heyman, H. M., Cleare, L. G., Couvillion, S. P., Clair, G., Bredeweg, E. L., et al. (2019). Multi-omics signature of Candida auris, an emerging and multidrug-resistant pathogen. mSystems 4, e257–e219. doi: 10.1128/mSystems.00257-19
Zamith-Miranda, D., Heyman, H. M., Couvillion, S. P., Cordero, R. J. B., Rodrigues, M. L., Nimrichter, L., et al. (2020). Comparative molecular and immunoregulatory analysis of extracellular vesicles from Candida albicans and Candida auris. bioRxiv [Preprint] doi: 10.1101/2020.11.04.368472
Zarnowski, R., Sanchez, H., Covelli, A. S., Dominguez, E., Jaromin, A., Bernhardt, J., et al. (2018). Candida albicans biofilm–induced vesicles confer drug resistance through matrix biogenesis. PLoS Biol. 16:e2006872. doi: 10.1371/journal.pbio.2006872
Zhang, X., Lan, Y., Xu, J., Quan, F., Zhao, E., Deng, C., et al. (2019). CellMarker: a manually curated resource of cell markers in human and mouse. Nucleic Acids Res. 47, D721–D728. doi: 10.1093/nar/gky900
Zhang, X., Romm, M., Zheng, X., Zink, E. M., Kim, Y. M., Burnum-Johnson, K. E., et al. (2016). SPE-IMS-MS: an automated platform for sub-sixty second surveillance of endogenous metabolites and xenobiotics in biofluids. Clin. Mass Spectrom. 2, 1–10. doi: 10.1016/j.clinms.2016.11.002
Zhao, K., Bleackley, M., Chisanga, D., Gangoda, L., Fonseka, P., Liem, M., et al. (2019). Extracellular vesicles secreted by Saccharomyces cerevisiae are involved in cell wall remodelling. Commun. Biol. 2:305. doi: 10.1038/s42003-019-0538-8
Zheng, X., Smith, R. D., and Baker, E. S. (2018). Recent advances in lipid separations and structural elucidation using mass spectrometry combined with ion mobility spectrometry, ion-molecule reactions and fragmentation approaches. Curr. Opin. Chem. Biol. 42, 111–118. doi: 10.1016/j.cbpa.2017.11.009
Keywords: extracellular vesicles, fungi, virulence, systems biology, proteomics, metabolomics, lipidomics, transcriptomics
Citation: Zamith-Miranda D, Peres da Silva R, Couvillion SP, Bredeweg EL, Burnet MC, Coelho C, Camacho E, Nimrichter L, Puccia R, Almeida IC, Casadevall A, Rodrigues ML, Alves LR, Nosanchuk JD and Nakayasu ES (2021) Omics Approaches for Understanding Biogenesis, Composition and Functions of Fungal Extracellular Vesicles. Front. Genet. 12:648524. doi: 10.3389/fgene.2021.648524
Received: 31 December 2020; Accepted: 06 April 2021;
Published: 03 May 2021.
Edited by:
Bhabatosh Das, Translational Health Science and Technology Institute (THSTI), IndiaReviewed by:
Yifan Ge, Massachusetts General Hospital and Harvard Medical School, United StatesCopyright © 2021 Zamith-Miranda, Peres da Silva, Couvillion, Bredeweg, Burnet, Coelho, Camacho, Nimrichter, Puccia, Almeida, Casadevall, Rodrigues, Alves, Nosanchuk and Nakayasu. This is an open-access article distributed under the terms of the Creative Commons Attribution License (CC BY). The use, distribution or reproduction in other forums is permitted, provided the original author(s) and the copyright owner(s) are credited and that the original publication in this journal is cited, in accordance with accepted academic practice. No use, distribution or reproduction is permitted which does not comply with these terms.
*Correspondence: Daniel Zamith-Miranda, ZGFuaWVsLnphbWl0aG1pcmFuZGFAZWluc3RlaW5tZWQub3Jn; Ernesto S. Nakayasu, ZXJuZXN0by5uYWtheWFzdUBwbm5sLmdvdg==
†These authors have contributed equally to this work
Disclaimer: All claims expressed in this article are solely those of the authors and do not necessarily represent those of their affiliated organizations, or those of the publisher, the editors and the reviewers. Any product that may be evaluated in this article or claim that may be made by its manufacturer is not guaranteed or endorsed by the publisher.
Research integrity at Frontiers
Learn more about the work of our research integrity team to safeguard the quality of each article we publish.