- 1State Key Laboratory for Conservation and Utilization of Subtropical Agro-Bioresources, Animal Reproduction Institute, Guangxi University, Nanning, China
- 2The Animal Husbandry Research Institute of Guangxi Autonomous, Nanning, China
- 3International Zhuang Medical Hospital Affiliated to Guangxi University Chinese Medicine, Nanning, China
Buffalo breeding has become an important branch of the beef cattle industry. Hence, it is of great significance to study buffalo meat production and meat quality. However, the expression profiles of mRNA and long non-coding RNAs (lncRNA) molecules in muscle stem cells (MuSCs) development in buffalo have not been explored fully. We, therefore, performed mRNA and lncRNA expression profiling analysis during the proliferation and differentiation phases of MuSCs in buffalo. The results showed that there were 4,820 differentially expressed genes as well as 12,227 mRNAs and 1,352 lncRNAs. These genes were shown to be enriched in essential biological processes such as cell cycle, p53 signaling pathway, RNA transport and calcium signaling pathway. We also identified a number of functionally important genes, such as MCMC4, SERDINE1, ISLR, LOC102394806, and LOC102403551, and found that interference with MYLPF expression significantly inhibited the differentiation of MuSCs. In conclusion, our research revealed the characteristics of mRNA and lncRNA expression during the differentiation of buffalo MuSCs. This study can be used as an important reference for the study of RNA regulation during muscle development in buffalo.
Introduction
There is an annual increase in the global consumption of beef and it is an indispensable food in our modern society, and therefore the beef cattle industry occupies an increasingly important position in modern agricultural practices (Bonny et al., 2015). According to statistics, in 2019, China's beef production was 6.85 million tons and beef imports were 1.66 million tons with a year-on-year increase of approximately 57%. It is anticipated that China's future beef demand will continue to rise. Therefore, China urgently needs a viable and thriving beef cattle industry in order to provide its society with larger amounts of high-quality beef (Mwangi et al., 2019; Ornaghi et al., 2020). There is a need for us to conduct research on the growth and meat quality of locally produced beef as well as to explore the potential molecular information of breeding stocks so as to provide reference values for future breeding protocols (Grigoletto et al., 2020).
In ruminants, skeletal muscle tissue accounts for about 40–60% of the adult animal body weight, which not only determines the level of meat production performance, but also has an important impact on meat quality. There is a group of myoblasts-muscle stem cells (MuSCs), which are the source of skeletal muscle formation and regeneration, and these have the potential for differentiation and proliferation of muscle-derived stem cells (Feige and Rudnicki, 2018; Feige et al., 2018). This is also the current cell model for studying skeletal muscle development. Under certain conditions, these cells can be activated causing the MuSCs proliferate and differentiate.
One of the main challenges in the field of muscle research is to understand how the genes that are involved in specialized muscle functions at the transcriptional and post-transcriptional levels are regulated. Undoubtedly, myogenic regulatory factors (MRFs) (Hernandez-Hernandez et al., 2017), myocyte enhancer factor-2 (MEF2) (Taylor and Hughes, 2017), and PAX3/PAX7 genes are the main genes involved in the growth and development of skeletal muscle. Initially, long non-coding RNAs (lncRNAs) were considered to be transcriptional noise but later studies showed these RNAs play an important function in many biological processes (Jae and Dimmeler, 2020). Epigenetic control and transcriptional regulation, translation, RNA metabolism, stem cell maintenance and differentiation, autophagy and apoptosis, embryonic development, and other aspects have also been shown to play important roles (Chen et al., 2020). With the discovery of a large number of important muscle regulators such as lncRNA H19 (Xu et al., 2017), Neat1 (Wang et al., 2019), lnc-133b (Jin et al., 2017), circLOM7 (Wei et al., 2017), more and more ncRNAs related to muscle development have also been widely characterized (Martone et al., 2019). At the same time, the important role of related coding RNAs, lncRNAs, and other molecules in the development of skeletal muscle in agricultural animals are gradually being explored.
So far, with the emergence of RNA structure detection technologies such as Frag-seq (Underwood et al., 2010), (ss/dsRNA)-seq, and SHAPE-seq, have allowed scientists to characterize the structure of RNAs obtained from different tissues and cell components. When these data were combined with knowledge of RNA transformation events, such as miRNA targeting, RNA modification, and the function of RNA binding proteins (RPBs), they have emphasized the importance of RNA structure during gene regulation (Li et al., 2012). Moreover, most of these studies are focused on mRNAs and ncRNAs in order to explore the biological functions of RNA structure.
As a characteristic species of southern China, the potential use of the buffalo as a meat source has gradually attracted attention. The buffalo breeding industry has become a food basket project for urban residents, but the meat production and meat quality of buffalo needs to be improved for it to be an acceptable alternative to cattle (Li et al., 2020). Previously, several breakthroughs have been made in studies of buffalo embryos, stem cells, and somatic cells, covering traits such as milk production, reproduction, and meat production. This culminated in the successful construction of the buffalo genomic DNA sequence map (Low et al., 2019). Recently our laboratory analyzed the regulatory networks of lncRNA-mRNA interactions in the muscle tissue of cattle and buffalo (Li et al., 2020).
However, when compared to cattle, buffalo muscle has the characteristics of possessing greater shear force and consisting of thicker muscle fibers. At present, the molecular mechanisms that regulate buffalo muscle fibers formation are still unclear (Huang et al., 2021). We hypothesized that there are key signaling pathway(s) which control the myogenic differentiation of MuSCs. We, therefore, analyzed the mRNA expression of MuSCs before and after myogenic differentiation through transcriptome sequencing strategies in an attempt to screen the signal pathways that may regulate muscle fiber development. Other recent studies have also shown that differential expression lncRNAs also play an important physiological function during cellular differentiation of MuSCs (Zhu et al., 2017). This study further expands the understanding of skeletal muscle biology, and provides a reference target for the genetic improvement of buffalo and the production and cultivation of meat in vitro and in vivo.
Materials and Methods
MuSCs Culture and Differentiation
All experiments regarding animals were performed in the State Key Laboratory for Conservation and Utilization of Subtropical Agro-bio-resources, and were conducted in accordance with its guidelines for the care and use of laboratory animals. Primary water buffalo MuSCs were isolated and cultured from fetal-derived longissimus muscle as described in Supplementary File 1, using a combination digestion method of type I collagenase and trypsin. MuSCs were cultured in high-glucose DMEM supplemented with fetal bovine serum (Hyclone, USA; 10% FBS and 20% FBS, respectively) and antibiotics [1% penicillin and streptomycin; growth medium (GM)] at 5% CO2, 37°C. To induce MuSCs myogenic differentiation, MuSCs were switched to a differentiation medium (DMEM, 2% horse serum; DM) when cells were almost 90% confluent for up to 4 days.
Sample Preparation
The tissues from Chinese buffalo at embryonic stage (90 days) were collected at a local slaughterhouse in Nanning, Guangxi province. Tissue samples, including muscle, liver, heart, lung, skin, kidney, brain, stomach, and intestine, were collected and immediately frozen in liquid nitrogen. Proliferation of MuSCs was labeled as the GM samples (n = 3) and differentiation of these was then called the DM samples (n = 3). The samples were kept at −80°C until RNA was isolated.
Total RNA Extraction
Total RNA from cells and tissues samples were extracted with TRizol reagent (Invitrogen, Carlsbad, CA, USA) in accordance with manufacturer's instructions.
RNA-Seq and Transcriptome Data Analysis
About 3 μg RNA per sample was used as the initial material for RNA sample preparation. PolyA-Seq libraries were prepared following the described protocol at RiboBio (Guangzhou, China) in accordance with the manufacturer's instructions. The identification of mRNAs and lncRNAs was carried out with reference to RiboBio's technical methods. We have provided a detailed description of the methods and analysis in Supplementary Document 1. All data were uploaded to the GEO database.
Analysis of Differentially Expressed Genes, mRNAs, and lncRNAs
The RPKM (expected number of Reads Per Kilobase of transcript sequence per Millions base pairs sequenced) value was used to estimate the expression levels of mRNAs and lncRNAs. Genes with a RPKM value of <1 in no <50% of samples were defined as unreliably expressed genes, while those with a RPKM value of ≥1 in more than 50% of samples were considered as reliably expressed genes. Differentially Expressed Genes DE mRNAs, and DE lncRNAs were analyzed using DESeq2, which defined them as reliably expressed genes with |log2 (Fold Change)|>1 and Q-values <0.05 between any two groups.
Gene Ontology and KEGG Analysis
Gene ontology (GO; http://www.geneontology.org) and KEGG pathway (http://www.kegg.jp) were analyzed as described previously.
Quantitative Real-Time PCR
Total RNA was extracted using TRizol reagent (Invitrogen, Carlsbad, CA, USA) according to the manufacturer's instructions. Reverse transcription was performed by using the HiScript R II One Step RT-PCR kit (Vazyme, Nanjing, China). RT-qPCR was performed with ChamQ SYBR qPCR Master Mix (Vazyme, Nanjing, China) using the 2−ΔΔCt method. Beta-actin was used as the internal control. All primer sequences used are listed in Supplementary File 2.
Western Blotting
Cells were collected from different treatment groups, pelleted by centrifugation, and then lysed in RIPA buffer. Total protein was prepared and protein concentrations were determined using the Bradford method. Proteins were then separated by SDS-polyacrylamide gel electrophoresis (SDS-PAGE) and subsequently transferred to nitrocellulose membranes. These were then blocked with 5% skimmed milk powder solution for 1.5–2 h at room temperature. The membranes were then incubated overnight with primary antibodies. Anti-PCNA, anti-CDK2, and anti-β-actin were purchased from Abcam (Cambridge, MA, USA). After that, the membranes were washed with PBS-tween and incubated for 1.5 h with horseradish peroxidase-conjugated secondary antibodies (Abcam, Cambridge, MA, USA). Protein bands were detected after treatment with Super Signal West Femto reagent purchased from Thermo (Thermo Scientific, Karlsruhe, Germany).
Vector Construction
Construction and sequencing identification of MYLPF interference vectors were completed by a Biological Company (GeneCopoeia, Guangzhou, China). The interference MYLPF expression vector plasmids, which were named sh-MYLPF-A, sh-MYLPF-B, sh-MYLPF-C, and the control plasmids were named NC. All primers sequences used are shown in Supplementary File 2.
Treatment of Cells
Muscle stem cells were grown to 70% confluence and then trypsinized and plated at 5 × 105 cells/well into six-well plates (Thermo Fisher Scientific, USA). They were then transfected with vectors using X-treme GENE HP DNA Transfection Reagent (Roche, Basel, Switzerland). After incubation, the MuSCs were used for the different assays outlined below. In order to induce differentiation of myoblasts, the culture medium was changed to high-glucose DM medium.
Immunofluorescence and Microscopy
Myoblasts of MuSCs were washed three times with PBS buffer (pH 7.4), and permeabilized for 15 min in PBS containing 0.5% Triton X-100 before fixation in PBS containing 4% paraformaldehyde for 20–30 min. Immunostaining was carried out as follows: cells were incubated overnight at 4°C with primary anti-MyoD1 (1:200; Abcam) diluted in 5% bovine serum albumin. After that, cells were washed with PBS and incubated at room temperature for 3–4 h with the corresponding secondary antibody, goat anti-mouse IgG (H+L; 1:1,000; Invitrogen) diluted in PBS. DNA was visualized using 5 mg/ml DAPI staining. Finally, the prepared cells were washed four times with PBS and observed under a fluorescence microscope (Nikon).
Statistical Analysis
The quantitative results are presented as means ± SEMs based on at least three independent experiments. Significant variance by treatments in comparison to the untreated samples was determined by one-way ANOVA performed with GraphPad Prism version 8.0 (GraphPad Software, La Jolla, CA, USA). Differences were considered significant when P-values were ≤ 0.05.
Results
Variation of Phenotypic Characteristics During Differentiation of Buffalo MuSCs
A combination digestion method of type I collagenase and trypsin was used to obtain buffalo fetal-derived MuSCs. This cell type is similar to fibroblasts and spindle-shaped in appearance. These cells have good proliferation capacity (Figure 1A), which is referred to as the proliferation phase (GM samples) of MuSCs. In addition, when the medium was replaced with DM, after 2 days, the cells began to show myotube fusion. On the fifth day, the number of myotubes increased and the myotube fusion became more obvious, which is referred to as the differentiation phase (DM samples) of MuSCs (Figure 1B).
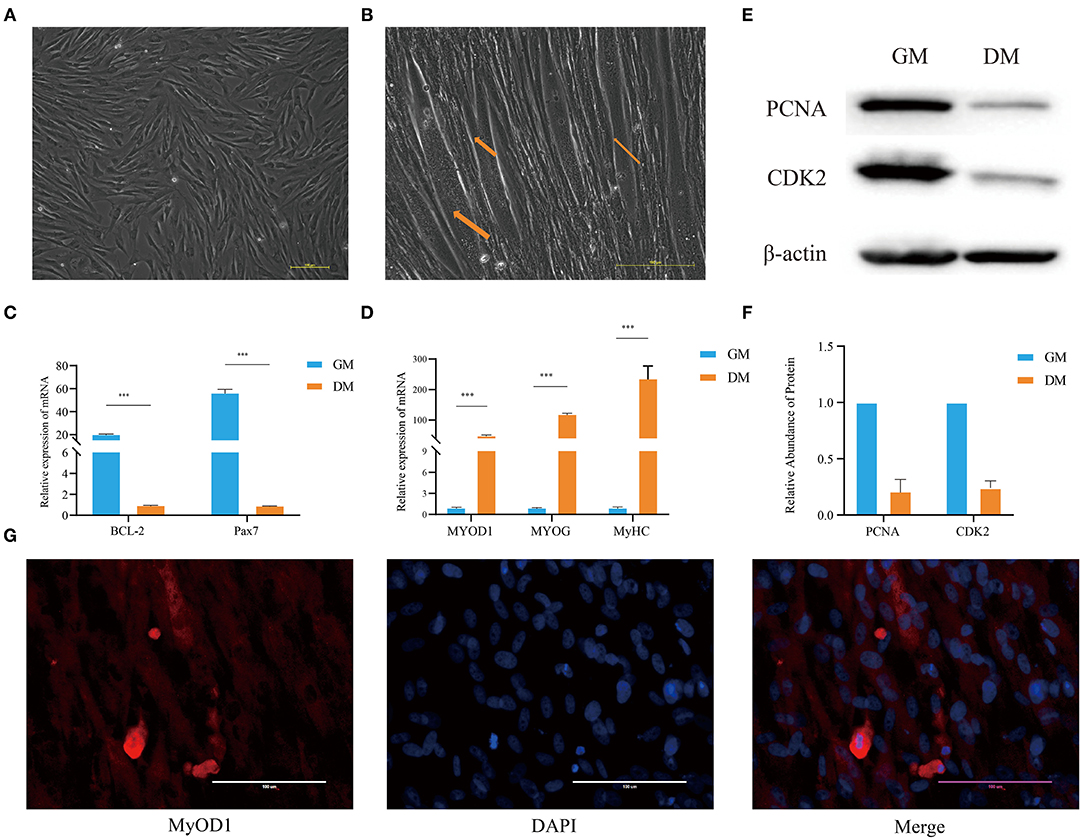
Figure 1. Analysis of the characteristics of buffalo MuSCs during proliferation and differentiation. (A) Proliferation phenotype of muscle stem cells (GM samples). (B) Differentiation phenotype of muscle stem cells (DM samples). (C) MuSCs grown as GM and DM samples were subjected to real-time PCR for BCL-2 and Pax7. (D) The differentiation phenotype (DM) samples of MuSCs were subjected to real-time PCR for MyoD1, MyoG, and MyHC. (E,F) MuSCs grown as GM and DM samples were subjected to western blotting for determination of PCNA and CDK2 proteins. (G) The differentiation phenotype (DM) samples of MuSCs were subjected to immunofluorescence for MyOD1. The data are presented as means ± SDs, n = 3 per group. *P < 0.05; **P < 0.01; ***P < 0.001. Scale bars = 100/200 μm.
Western blotting showed that the expression of PCNA and CDK2 in MuSCs GM samples were significantly higher than that in DM samples (Figures 1E,F). The expression levels of BCL-2 and Pax7 (paired Homeobox transcription factors) in GM samples were significantly higher than those in MuSCs DM samples (Figure 1C). Immunofluorescence experiments showed that the muscle marker molecule, MyOD1, was enriched in DM samples of MuSCs (Figure 1G). The expression levels of muscle-derived marker molecules, MYOD1, MYOG, and MyHC, increased significantly in DM samples of MuSCs (Figure 1D). These results suggest that the cells obtained were MuSCs with the capability of myogenic differentiation.
PolyA-Seq Characteristics of Buffalo MuSCs
In order to identify the mRNAs and lncRNAs involved in proliferation and differentiation, we compared the polyA-seq status of GM and DM samples of MuSCs (Supplementary Figure S1). Analysis of sequencing data revealed that a very large number of clean reads, total maps, and uniquely mapped areas were involved in these processes (Figure 2A). The analysis of uniquely map profiles of MuSCs, showed the distribution for the reads in different chromosomes (Figures 2B,C). Among them, most of the reads from GM and DM samples were found to be targeted to exonic areas.
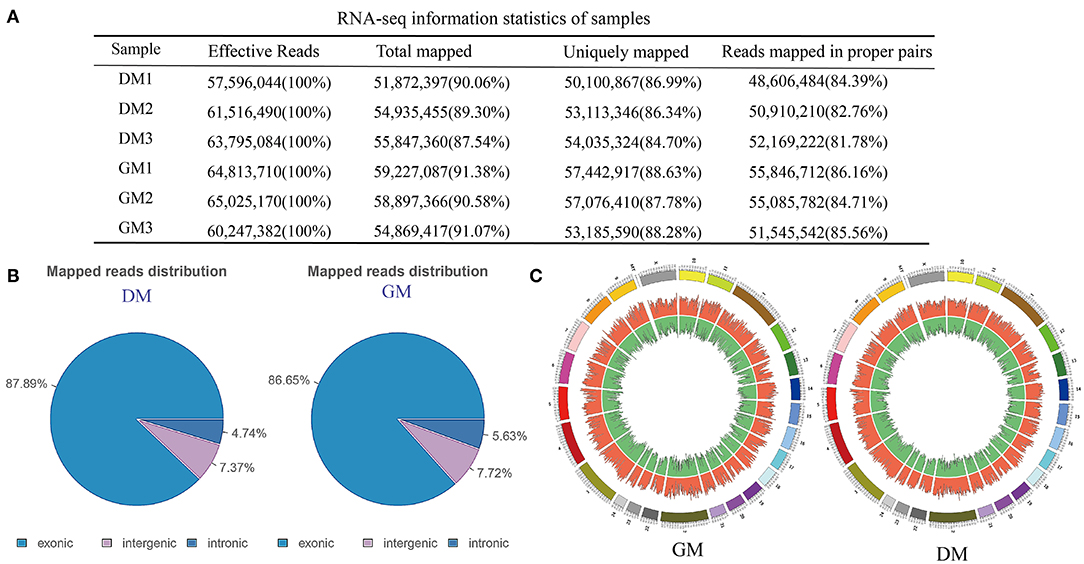
Figure 2. RNA-seq analysis of buffalo MuSCs during proliferation and differentiation. (A) RNA-seq information statistics of samples. (B) Mapped reads distribution. (C) The distribution for the reads in different chromosomes.
Profiles of DE Genes, mRNAs, and lncRNAs During Differentiation of Buffalo MuSCs
Analysis of sequencing data revealed that a total of 31,819 genes, 57,640 mRNAs, and 11,357 lncRNAs were involved in the proliferation and differentiation of MuSCs (Supplementary Table 1). We also performed heatmaps and volcano plots for the genes, mRNAs, and lncRNAs in MuSCs (|log2 (FoldChange)|>1, Q-value <0.05) (Supplementary Figure S2). There were 4,820 DEGs, 12,227 DE mRNAs, and 1,352 DE lncRNAs (Supplementary Tables 2–4). Compared with the GM samples of MuSCs, 2,979 genes (61.80%), 7,505 mRNAs (61.38%), and 831 lncRNAs (61.46%) were upregulated, while 1,841 genes (38.20%), 4,722 mRNAs (38.62%), and 521 lncRNAs (38.54%) were downregulated in DM samples of MuSCs (Table 1).
Signal Pathway Enrichment Analysis of DEGs Between Proliferation and Differentiation Phases of Buffalo MuSCs
Since we mainly analyzed mRNAs transcripts, and also involved a small number of known lncRNAs, we did not perform functional correlation analysis on these lncRNAs. We performed GO and KEGG enrichment analysis on the related regulatory DEGs in the processes of proliferation, differentiation, transformation, and maturation. This produced signal pathway information which was then used to predict the functions and mechanisms of the mRNAs (Supplementary Tables 5, 6). The results of pathway analysis of DEGs showed that GO analyzed and annotated these into three main categories: biological processes, cellular components, and molecular functions, including ATP binding and nucleus RNA-directed DNA polymerase activity (Figure 3). In addition, we employed KEGG pathway enrichment analysis to further understand the biological functions and molecular interactions of most DEGs with the assumption that the identified pathways may be involved in the development and growth of buffalo skeletal muscle. We found more than 300 pathways to be enriched, and the top 30 most significant terms were uncovered, including biological processes such as cell cycle, p53 signaling pathway, RNA transport, and calcium signaling pathway (Figure 4). In short, these signal pathways related to DEGs play an important role in the regulation of MuSC proliferation and differentiation, which provides an important basis for subsequent research on buffalo myogenesis.
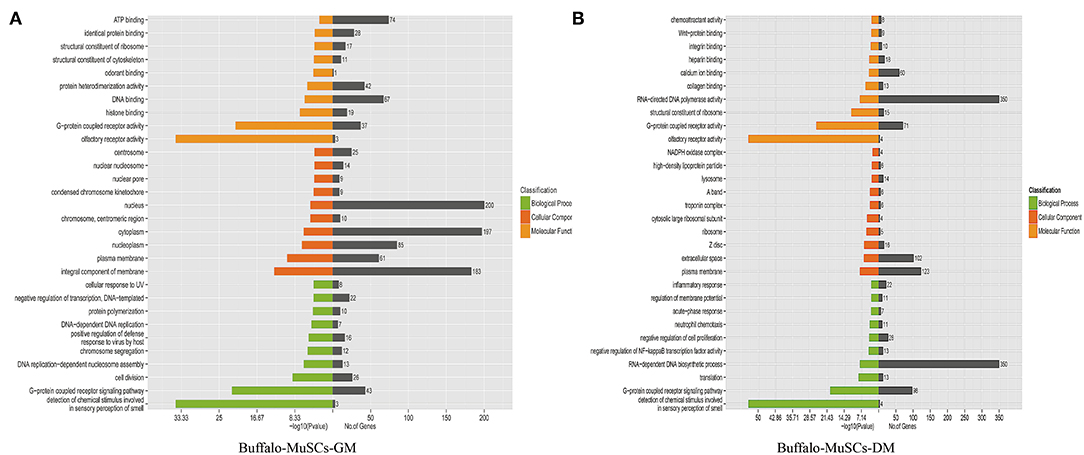
Figure 3. Gene ontology (GO) analysis. (A) GO analysis of DEGs in the GM samples of MuSCs. (B) GO analysis of DEGs in the DM samples of MuSCs.
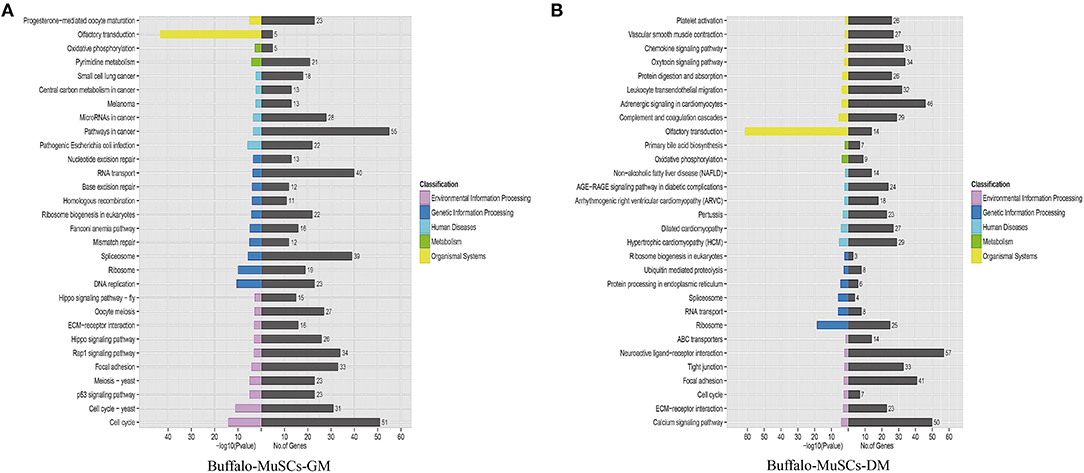
Figure 4. Kyoto Encyclopedia of Genes and Genomes pathway analysis. (A) Top 30 KEGG terms of DEGs in the GM samples of MuSCs. (B) Top 30 KEGG terms of DEGs in the DM samples of MuSCs.
The Verification of DEGs and DE lncRNAs in MuSCs
Based on the expression levels of DEGs and DE lncRNAs, including 12 genes (MCM4, MCM7, SERDINE1, SEMA7A, C1QTNF6, CPZ, VDR, PLAC9, ISLR, MyOG, PCNA, and cyclin D1) related to the cell cycle, actin cytoskeleton, cell differentiation, and lipid metabolism (Figures 5A,B), and seven random lncRNAs (LOC102403551, LOC112586870, LOC112584513, LOC102399397, LOC112581569, LOC102395296, and LOC102394806; Figure 5C), were selected for RT-qPCR verification. After comparisons with the RNA-seq data, similar expression trends for RT-qPCR were discovered, showing the strong consistency between RT-qPCR and RNA-seq data.
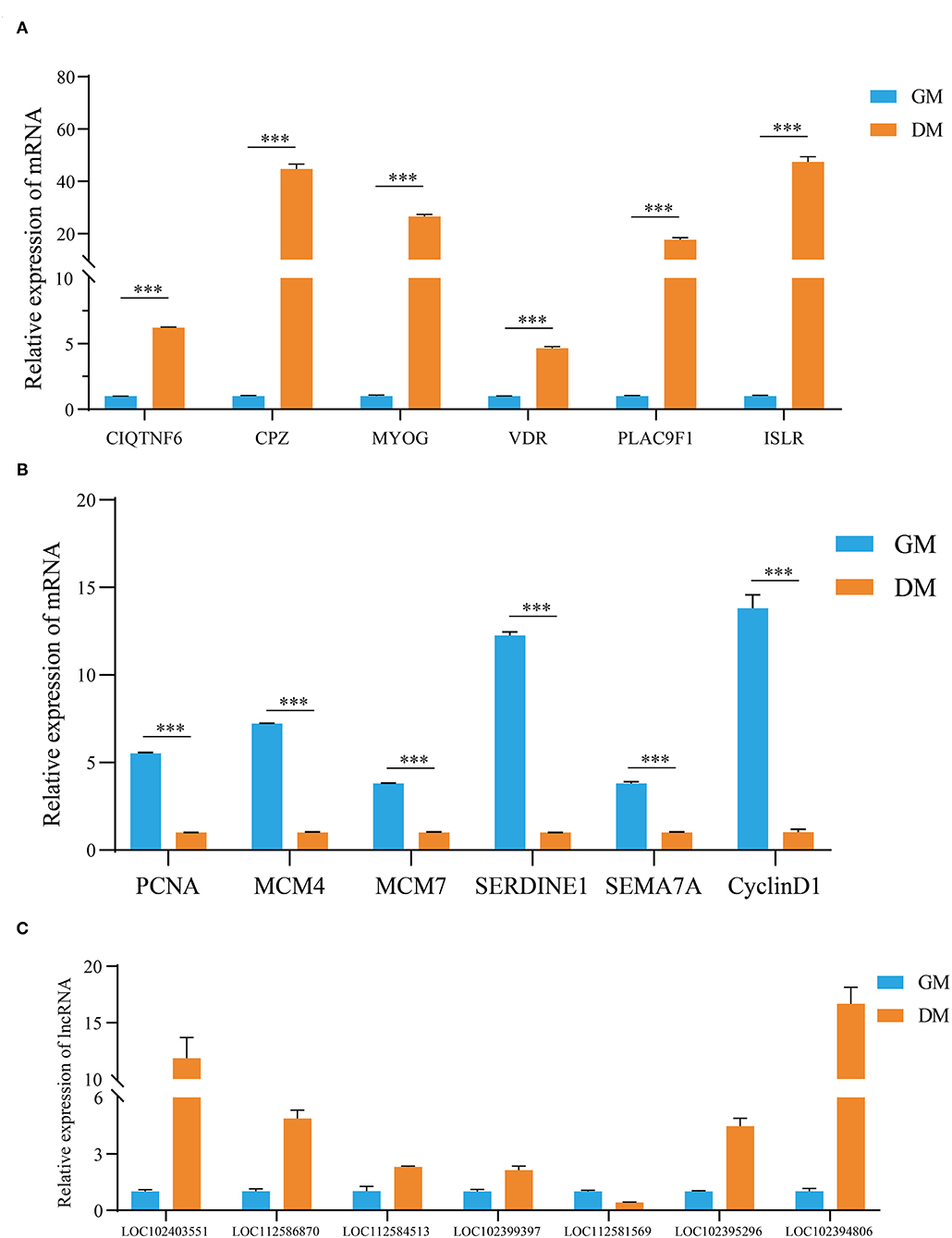
Figure 5. Validation of the expression levels of DEGs, DE lncRNAs by RT-qPCR. (A,B) The validation results of DEGs and (C) DE lncRNAs. The data are present as means ± SDs, n = 3 per group. ***P < 0.001.
The Role of MYLPF in Buffalo MuSCs
We identified a dysregulated gene, MYLPF, which was shown to be upregulated significantly (by almost 60-fold) in DM compared with GM samples when measured by RT-qPCR (Figure 6A). In addition, MYLPF was expressed in various tissues, such as heart and liver and the highest expression levels seen in muscle (Figure 6B). A previous report also showed that the relationship between MYLPF and meat quality can be used as an important genetic consideration when dealing with gene-assisted selection programs. This suggests that MYLPF may play an important role in muscle development. Therefore, MYLPF was selected for more in-depth study in order to further explore its potential functions in MuSCs.
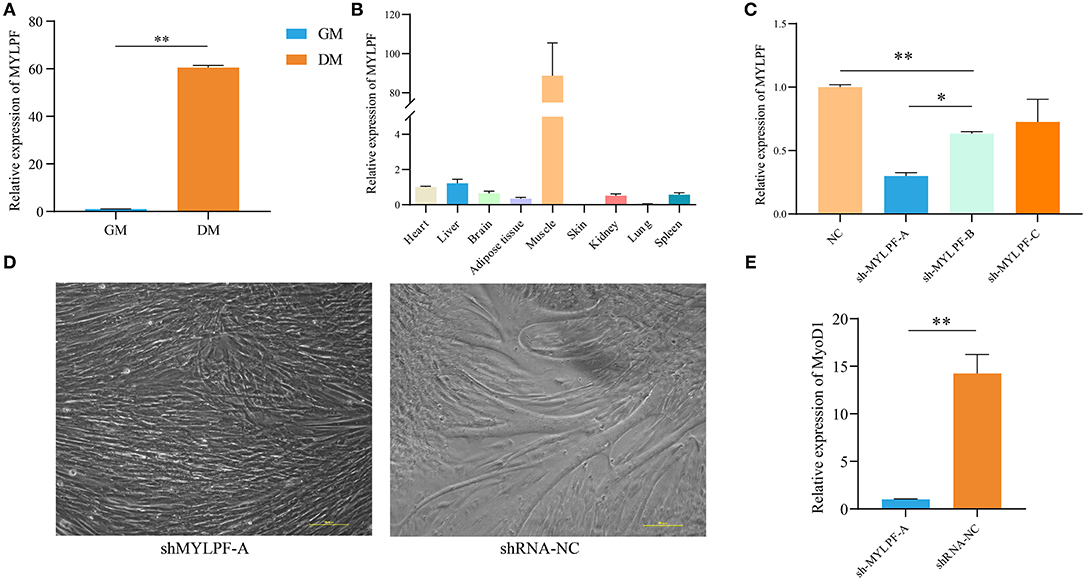
Figure 6. Expression and characterization of MYLPF in buffalo skeletal muscle. (A) Validation of the expression of MYLPF by RT-qPCR. (B) The expression levels of MYLPF in different tissues of buffalo. (C) The interference efficiency of MYLPF was measured by RT-qPCR. (D) Inhibition of MYLPF expression on myotubule formation. (E) The mRNA expression of myogenesis marker gene, MyOD1, was measured by RT-qPCR. The data are presented as means ± SDs, n = 3 per group. *P < 0.05; **P < 0.01. Scale bars = 100 μm.
Subsequently, interference vector (sh-MYLPF-A/B/C/NC) plasmids were introduced into the 293T cell line which is derived from human embryonic kidney 293 cells and contains the SV40 T-antigen. After 24 h, the reporter gene, green fluorescence protein (GFP), was found to be expressed in the transfected cells, with a strong fluorescence observed under the fluorescence microscope (Supplementary Figure S3). Further qPCR showed that the expression levels of MYLPF declined by 70% in the transfected cells with the sh-MYLPF-A plasmid (Figure 6C).
Sh-MYLPF-A vectors were then transferred into P2 MuSCs (Supplementary Figure S4). The cells were cultured for a further 24 h, followed by replacement of the medium with myogenic differentiation medium. On the fourth day, the knockdown of MYLPF was found to have inhibited the formation of myotubes (Figure 6D). The marker gene of myoblast differentiation, MyoD1, was then measured by qPCR. The results showed that there were significantly lower levels of MYLPF in the knockdown group compared to the controls (Figure 6E). These findings suggested that MYLPF knockdown inhibited differentiation of MuSCs.
Discussion
Currently, the global population is 7.7 billion, and it is expected to exceed 9 billion by 2050 (Bonny et al., 2015). By then, mankind will face a bigger challenge of food provision for the growing population, and this will have a major impact on global meat consumption which will increase accordingly (Ornaghi et al., 2020). Muscle development is an important factor that affects the growth rate, meat yield, meat quality, and other important economic traits of livestock, and this process is dependent on the proliferation and differentiation of MuSCs (Feige and Rudnicki, 2018; Boscolo Sesillo et al., 2020).
Initially, we established a successful protocol for in vitro culture of buffalo MuSCs, which provided a good working foundation for subsequent research on candidate factors that regulate buffalo muscle development. With the rise of in vitro cultured meat such as laboratory meat, the role played by MuSCs is becoming more important. The in vitro cultured meat production technology is still in its infancy, and it is necessary to strengthen and improve the technical systems involved in MuSCs production of beef (Bhat et al., 2017). Therefore, buffalo MuSCs can play an important role in the emerging research fields of animal husbandry, such as providing improvement of buffalo meat quality and production as well as increasing our biochemical knowledge of MuSCs in vitro.
In this study, we constructed the expression profiles of mRNAs and lncRNAs in the process of myogenic differentiation of buffalo MuSCs. During this process, a total of 4,820 genes, 12,227 mRNAs, and 1,352 lncRNAs were differentially expressed. Among them, 2,979 genes, 7,505 mRNAs, and 831 lncRNAs were significantly related to the myogenic differentiation of these cells, and they affected the formation of myoblasts and the fusion of myotubes. In addition, we performed target gene analysis on differentially expressed lncRNAs, and obtained many lncRNAs-target gene relationship networks. We can indirectly predict the function of the candidate lncRNA through the target gene (Supplementary Tables 7, 8). Previous studies had confirmed that compared with cattle, buffalo muscles have larger muscle fiber diameters and poorer meat texture. Of course, myotube fusion is an important factor affecting the formation of muscle fibers (Picard and Gagaoua, 2020). The mRNAs and lncRNAs which are related to myogenic differentiation of MuSCs may regulate the diameter of muscle fibers through myotube fusion, which further affected the quality of meat. We also found some key signal transduction pathways, such as p53 signal transduction pathway, TGF-β signal pathway, calcium signal transduction pathway that were related to these RNAs. These signal pathways are involved in cell development and maintenance of muscle structure and function, suggesting that they were also likely to be important regulatory signals for regulating buffalo muscle fiber hypertrophy (Liu et al., 2018; Valle-Tenney et al., 2020).
We also randomly selected a batch of candidate molecules for verification, and their expression trends were found to be consistent with the RNA-seq results, indicating that the sequencing data was reliable. We found that genes such as VDR, PLAC9, ISLR were involved in the myogenic differentiation process of MuSCs, but their molecular mechanisms needed to be further explored (Bass et al., 2020; Cui et al., 2020). It had been confirmed that the immunoglobulin superfamily containing leucine-rich repeats (ISLR) promoted skeletal muscle regeneration by activating canonical Wnt signaling. Loss of function of ISLR resulted in defective differentiation of myoblasts leading to a block in myotube formation (Zhang et al., 2018). Therefore, ISLR may be an important biological regulator to control buffalo muscle development. It had also been reported that MYLPF was one of the muscle-derived marker genes involved in the process of muscle metabolism and related to meat quality traits (Rosa et al., 2018; Chong et al., 2020). As one of the muscle markers, MYLPF is expected to become a target for regulating the quality traits of buffalo meat (Silva et al., 2019). We also found that decreased MYLPF was linked to an inhibition of myogenic differentiation of buffalo MuSCs, but the molecular mechanism of this phenomenon is not yet fully understood. Therefore, how MYLPF regulates buffalo muscle regeneration is worthy of further investigation.
At present, lncRNA is also one of the research hotspots in the field of ncRNA (Martone et al., 2019). However, we only discovered the number of known lncRNAs and their expression levels involved in the myogenic differentiation of MuSCs. Then, we screened out a batch of potential candidate lncRNAs, such as LOC102403551, LOC112586870, and LOC102394806. These potential candidate lncRNAs may affect the myoblast differentiation of MuSCs by regulating gene expression through miRNAs, RPBs, and other ways (Chi et al., 2019; Xu et al., 2019; Guo et al., 2020; Liu et al., 2020). In future studies, we and others will also investigate the interactions between lncRNAs and enhancers in order to regulate fate of MuSCs (Lin et al., 2019; Nikonova et al., 2019; Williams et al., 2020). The biological effects of these lncRNAs related to buffalo MuSCs, lncRNA evolution, lncRNA SNP issues, etc. are also worth pursuing (Qian et al., 2019). At the same time, how these lncRNAs and coding genes regulate the molecular mechanisms of farmed beef production and their contributions to the in vitro meat production process also need to be further explored.
In summary, we have established the mRNA and lncRNA expression profiles that regulate the myogenic differentiation of buffalo MuSCs, and further predicted and verified the signaling pathways and candidate regulators involved in cell proliferation and differentiation. These results enrich the expression information of factors that regulate the development of MuSCs in Chinese local fine beef cattle breeds, and provide effective genetic information for future programs of breeding high-yield beef cattle.
Conclusions
In this study, the proliferation and myogenic differentiation phenotypic characteristics of buffalo MuSCs were compared for the first time, and the expression of mRNAs and lncRNAs in these cells were reviewed. Many coding RNAs and lncRNAs were found to be differentially expressed during the proliferation and myogenic differentiation phases of MuSCs. We further identified and verified a number of differentially expressed molecules such as SERDINE1, ISLR, MYLPF, LOC102403551, LOC112586870, and LOC112584513. This study lays the foundation for further research on the role of lncRNAs in the muscle development of buffalo with a view to improving its share as a desirable beef alternative in the marketplace.
Data Availability Statement
The datasets presented in this study can be found in online repositories. The names of the repository/repositories and accession number(s) can be found below: NCBI GEO, accession no: GSE164808.
Author Contributions
YD, SY, YW, JW, and RZ conceived and designed the experiments. RZ, JW, and CZ performed the experiments and analyzed the data. RZ, QA, CZ, XZ, ZW, HL, and DS contributed reagents, materials, and helped to analyze the data. RZ and ZX wrote the manuscript and SY, YD, DS, and YW revised it. All authors contributed to the article and approved the submitted version.
Funding
The research was funded by the National Natural Science Foundation of China (31860644 and 31760334), Natural Science Foundation of Guangxi Province (AA17204051, AA17204052, 2017GXNSFBA198170, and 2019GXNSFDA185002), Nanning Scientific Research Technological Development Foundation (20194147 and 20192087), and Guangxi Innovation Team Construction Project of National Modern Agricultural Industry Technology System (nycytxgxcxtd-09-01).
Conflict of Interest
The authors declare that the research was conducted in the absence of any commercial or financial relationships that could be construed as a potential conflict of interest.
Acknowledgments
We would like to thank RiboBio (Guangzhou, China) for conducting the whole-genome RNA sequencing. We are also grateful to Dr. Dev Sooranna of Imperial College London for English language edits of the manuscript.
Supplementary Material
The Supplementary Material for this article can be found online at: https://www.frontiersin.org/articles/10.3389/fgene.2021.643497/full#supplementary-material
Abbreviations
MuSCs, muscle stem cells; GO, gene ontology; KEGG, Kyoto Encyclopedia of Genes and Genomes; lncRNAs, long non-coding RNAs; RT-qPCR, quantitative real time PCR.
References
Bass, J. J., Nakhuda, A., Deane, C. S., Brook, M. S., Wilkinson, D. J., Phillips, B. E., et al. (2020). Overexpression of the vitamin D receptor (VDR) induces skeletal muscle hypertrophy. Mol. Metab. 42:101059. doi: 10.1016/j.molmet.2020.101059
Bhat, Z. F., Kumar, S., and Bhat, H. F. (2017). In vitro meat: a future animal-free harvest. Crit. Rev. Food Sci. Nutr. 57, 782–789. doi: 10.1080/10408398.2014.924899
Bonny, S. P. F., Gardner, G. E., Pethick, D. W., and Hocquette, J.-F. (2015). What is artificial meat and what does it mean for the future of the meat industry? J. Integr. Agric. 14, 255–263. doi: 10.1016/S2095-3119(14)60888-1
Boscolo Sesillo, F., Wong, M., Cortez, A., and Alperin, M. (2020). Isolation of muscle stem cells from rat skeletal muscles. Stem. Cell Res. 43:101684. doi: 10.1016/j.scr.2019.101684
Chen, R., Lei, S., Jiang, T., Zeng, J., Zhou, S., and She, Y. (2020). Roles of lncRNAs and circRNAs in regulating skeletal muscle development. Acta Physiol. 228:e13356. doi: 10.1111/apha.13356
Chi, Y., Wang, D., Wang, J., Yu, W., and Yang, J. (2019). Long non-coding RNA in the pathogenesis of cancers. Cells 8:1015. doi: 10.3390/cells8091015
Chong, J. X., Talbot, J. C., Teets, E. M., Previs, S., Martin, B. L., Shively, K. M., et al. (2020). Mutations in MYLPF cause a novel segmental amyoplasia that manifests as distal arthrogryposis. Am. J. Hum. Genet. 107, 293–310. doi: 10.1016/j.ajhg.2020.06.014
Cui, C., Han, S., Shen, X., He, H., Chen, Y., Zhao, J., et al. (2020). ISLR regulates skeletal muscle atrophy via IGF1-PI3K/Akt-Foxo signaling pathway. Cell Tissue Res. 381, 479–492. doi: 10.1007/s00441-020-03251-4
Feige, P., Brun, C. E., Ritso, M., and Rudnicki, M. A. (2018). Orienting muscle stem cells for regeneration in homeostasis, aging, and disease. Cell Stem Cell 23, 653–664. doi: 10.1016/j.stem.2018.10.006
Feige, P., and Rudnicki, M. A. (2018). Muscle stem cells. Curr. Biol. 28, R589–R590. doi: 10.1016/j.cub.2018.02.064
Grigoletto, L., Ferraz, J. B. S., Oliveira, H. R., Eler, J. P., Bussiman, F. O., Abreu Silva, B. C., et al. (2020). Genetic architecture of carcass and meat quality traits in montana tropical[(R)] composite beef cattle. Front. Genet. 11:123. doi: 10.3389/fgene.2020.00123
Guo, C. J., Ma, X. K., Xing, Y. H., Zheng, C. C., Xu, Y. F., Shan, L., et al. (2020). Distinct processing of lncRNAs contributes to non-conserved functions in stem cells. 181, 621.e22–636.e22. doi: 10.1016/j.cell.2020.03.006
Hernandez-Hernandez, J. M., Garcia-Gonzalez, E. G., Brun, C. E., and Rudnicki, M. A. (2017). The myogenic regulatory factors, determinants of muscle development, cell identity and regeneration. Semin. Cell Dev. Biol. 72, 10–18. doi: 10.1016/j.semcdb.2017.11.010
Huang, K., Chen, M., Zhong, D., Luo, X., Feng, T., Song, M., et al. (2021). Circular RNA profiling reveals an abundant circEch1 that promotes myogenesis and differentiation of bovine skeletal muscle. J. Agric. Food Chem. 69, 592–601. doi: 10.1021/acs.jafc.0c06400
Jae, N., and Dimmeler, S. (2020). Noncoding RNAs in vascular diseases. Circ. Res. 126, 1127–1145. doi: 10.1161/CIRCRESAHA.119.315938
Jin, C. F., Li, Y., Ding, X. B., Li, X., Zhang, L. L., Liu, X. F., et al. (2017). lnc133b, a novel, long non-coding RNA, regulates bovine skeletal muscle satellite cell proliferation and differentiation by mediating miR-133b. Gene 630, 35–43. doi: 10.1016/j.gene.2017.07.066
Li, F., Zheng, Q., Ryvkin, P., Dragomir, I., Desai, Y., Aiyer, S., et al. (2012). Global analysis of RNA secondary structure in two metazoans. Cell. Rep. 1, 69–82. doi: 10.1016/j.celrep.2011.10.002
Li, H., Huang, K., Wang, P., Feng, T., Shi, D., Cui, K., et al. (2020). Comparison of long non-coding RNA expression profiles of cattle and buffalo differing in muscle characteristics. Front. Genet. 11:98. doi: 10.3389/fgene.2020.00098
Lin, X., Spindler, T. J., de Souza Fonseca, M. A., Corona, R. I., Seo, J. H., Dezem, F. S., et al. (2019). Super-enhancer-associated LncRNA UCA1 interacts directly with AMOT to activate YAP target genes in epithelial ovarian cancer. iScience 17, 242–255. doi: 10.1016/j.isci.2019.06.025
Liu, L., Charville, G. W., Cheung, T. H., Yoo, B., Santos, P. J., Schroeder, M., et al. (2018). Impaired notch signaling leads to a decrease in p53 activity and mitotic catastrophe in aged muscle stem cells. Cell Stem Cell 23, 544.e4–556.e4. doi: 10.1016/j.stem.2018.08.019
Liu, W., Wang, Z., Liu, L., Yang, Z., Liu, S., Ma, Z., et al. (2020). LncRNA Malat1 inhibition of TDP43 cleavage suppresses IRF3-initiated antiviral innate immunity. Proc. Natl. Acad. Sci. U.S.A. 117, 23695–23706. doi: 10.1073/pnas.2003932117
Low, W. Y., Tearle, R., Bickhart, D. M., Rosen, B. D., Kingan, S. B., Swale, T., et al. (2019). Chromosome-level assembly of the water buffalo genome surpasses human and goat genomes in sequence contiguity. Nat. Commun. 10:260. doi: 10.1038/s41467-018-08260-0
Martone, J., Mariani, D., Desideri, F., and Ballarino, M. (2019). Non-coding RNAs shaping muscle. Front. Cell Dev. Biol. 7:394. doi: 10.3389/fcell.2019.00394
Mwangi, F. W., Charmley, E., Gardiner, C. P., Malau-Aduli, B. S., Kinobe, R. T., and Malau-Aduli, A. E. O. (2019). Diet and genetics influence beef cattle performance and meat quality characteristics. Foods 8:648. doi: 10.3390/foods8120648
Nikonova, E., Kao, S. Y., Ravichandran, K., Wittner, A., and Spletter, M. L. (2019). Conserved functions of RNA-binding proteins in muscle. Int. J. Biochem. Cell Biol. 110, 29–49. doi: 10.1016/j.biocel.2019.02.008
Ornaghi, M. G., Guerrero, A., Vital, A. C. P., de Souza, K. A., Passetti, R. A. C., Mottin, C., et al. (2020). Improvements in the quality of meat from beef cattle fed natural additives. Meat. Sci. 163:108059. doi: 10.1016/j.meatsci.2020.108059
Picard, B., and Gagaoua, M. (2020). Muscle fiber properties in cattle and their relationships with meat qualities: an overview. J. Agric. Food Chem. 68, 6021–6039. doi: 10.1021/acs.jafc.0c02086
Qian, X., Zhao, J., Yeung, P. Y., Zhang, Q. C., and Kwok, C. K. (2019). Revealing lncRNA structures and interactions by sequencing-based approaches. Trends Biochem. Sci. 44, 33–52. doi: 10.1016/j.tibs.2018.09.012
Rosa, A. F., Moncau, C. T., Poleti, M. D., Fonseca, L. D., Balieiro, J. C. C., Silva, S. L. E., et al. (2018). Proteome changes of beef in Nellore cattle with different genotypes for tenderness. Meat Sci. 138, 1–9. doi: 10.1016/j.meatsci.2017.12.006
Silva, L. H. P., Rodrigues, R. T. S., Assis, D. E. F., Benedeti, P. D. B., Duarte, M. S., and Chizzotti, M. L. (2019). Explaining meat quality of bulls and steers by differential proteome and phosphoproteome analysis of skeletal muscle. J. Proteomics 199, 51–66. doi: 10.1016/j.jprot.2019.03.004
Taylor, M. V., and Hughes, S. M. (2017). Mef2 and the skeletal muscle differentiation program. Semin. Cell Dev. Biol. 72, 33–44. doi: 10.1016/j.semcdb.2017.11.020
Underwood, J. G., Uzilov, A. V., Katzman, S., Onodera, C. S., Mainzer, J. E., Mathews, D. H., et al. (2010). FragSeq: transcriptome-wide RNA structure probing using high-throughput sequencing. Nat. Methods 7, 995–1001. doi: 10.1038/nmeth.1529
Valle-Tenney, R., Rebolledo, D. L., Lipson, K. E., and Brandan, E. (2020). Role of hypoxia in skeletal muscle fibrosis: synergism between hypoxia and TGF-beta signaling upregulates CCN2/CTGF expression specifically in muscle fibers. Matrix Biol. 87, 48–65. doi: 10.1016/j.matbio.2019.09.003
Wang, S., Zuo, H., Jin, J., Lv, W., Xu, Z., Fan, Y., et al. (2019). Long noncoding RNA Neat1 modulates myogenesis by recruiting Ezh2. Cell Death Dis. 10:505. doi: 10.1038/s41419-019-1742-7
Wei, X., Li, H., Yang, J., Hao, D., Dong, D., Huang, Y., et al. (2017). Circular RNA profiling reveals an abundant circLMO7 that regulates myoblasts differentiation and survival by sponging miR-378a-3p. Cell Death Dis. 8:e3153. doi: 10.1038/cddis.2017.541
Williams, K., Ingerslev, L. R., Bork-Jensen, J., Wohlwend, M., Hansen, A. N., Small, L., et al. (2020). Skeletal muscle enhancer interactions identify genes controlling whole-body metabolism. Nat. Commun. 11:2695. doi: 10.1038/s41467-020-16537-6
Xu, X., Ji, S., Li, W., Yi, B., Li, H., Zhang, H., et al. (2017). LncRNA H19 promotes the differentiation of bovine skeletal muscle satellite cells by suppressing Sirt1/FoxO1. Cell Mol. Biol. Lett. 22:10. doi: 10.1186/s11658-017-0040-6
Xu, Y., Wu, W., Han, Q., Wang, Y., Li, C., Zhang, P., et al. (2019). New insights into the interplay between non-coding RNAs and RNA-binding protein HnRNPK in regulating cellular functions. Cells 8:63. doi: 10.3390/cells8010062
Zhang, K., Zhang, Y., Gu, L., Lan, M., Liu, C., Wang, M., et al. (2018). Islr regulates canonical Wnt signaling-mediated skeletal muscle regeneration by stabilizing Dishevelled-2 and preventing autophagy. Nat. Commun. 9:5129. doi: 10.1038/s41467-018-07638-4
Keywords: buffalo, muscle stem cells, mRNAs, non-coding RNAs, myogenesis
Citation: Zhang R, Wang J, Xiao Z, Zou C, An Q, Li H, Zhou X, Wu Z, Shi D, Deng Y, Yang S and Wei Y (2021) The Expression Profiles of mRNAs and lncRNAs in Buffalo Muscle Stem Cells Driving Myogenic Differentiation. Front. Genet. 12:643497. doi: 10.3389/fgene.2021.643497
Received: 18 December 2020; Accepted: 25 May 2021;
Published: 07 July 2021.
Edited by:
Guohua Hua, Huazhong Agricultural University, ChinaReviewed by:
Ikhide G. Imumorin, Georgia Institute of Technology, United StatesBo Wang, China Agricultural University, China
Copyright © 2021 Zhang, Wang, Xiao, Zou, An, Li, Zhou, Wu, Shi, Deng, Yang and Wei. This is an open-access article distributed under the terms of the Creative Commons Attribution License (CC BY). The use, distribution or reproduction in other forums is permitted, provided the original author(s) and the copyright owner(s) are credited and that the original publication in this journal is cited, in accordance with accepted academic practice. No use, distribution or reproduction is permitted which does not comply with these terms.
*Correspondence: Yanfei Deng, eWFuZmVpLWR1biYjeDAwMDQwOzE2My5jb20=; Sufang Yang, eXNmYW5nMzUxMSYjeDAwMDQwOzE2My5jb20=; Yingming Wei, ZGt5d3ltJiN4MDAwNDA7MTYzLmNvbQ==
†These authors have contributed equally to this work