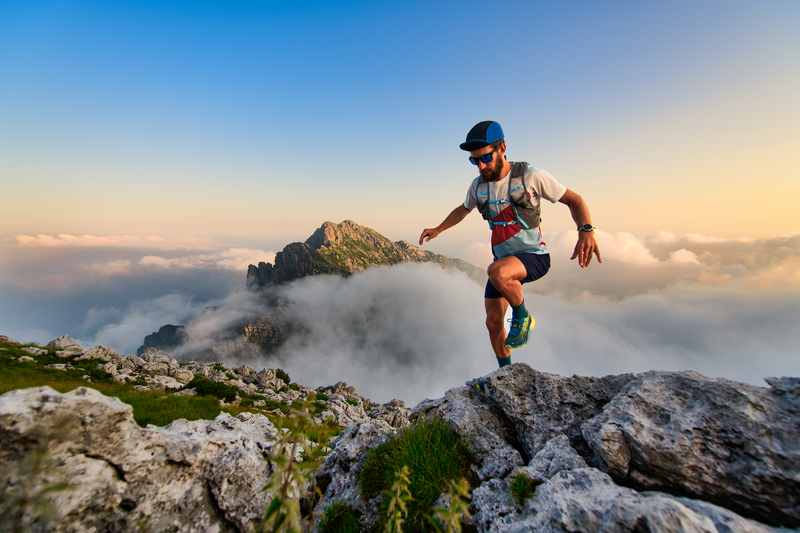
94% of researchers rate our articles as excellent or good
Learn more about the work of our research integrity team to safeguard the quality of each article we publish.
Find out more
REVIEW article
Front. Genet. , 07 June 2021
Sec. Genetics of Common and Rare Diseases
Volume 12 - 2021 | https://doi.org/10.3389/fgene.2021.640954
This article is part of the Research Topic Mechanisms of Skeletal Development, Disease and Treatments View all 9 articles
Multiple myeloma (MM) is a clonal B-cell disorder characterized by the proliferation of malignant plasma cells (PCs) in the bone marrow, the presence of monoclonal serum immunoglobulin, and osteolytic lesions. It is the second most common hematological malignancy and considered an incurable disease despite significant treatment improvements. MM bone disease (MMBD) is defined as the presence of one or more osteolytic bone lesions or diffused osteoporosis with compression fracture attributable to the underlying clonal PC disorder. MMBD causes severe morbidity and increases mortality. Cumulative evidence shows that the interaction of MM cells and bone microenvironment plays a significant role in MM progression, suggesting that these interactions may be good targets for therapy. MM animal models have been developed and studied in various aspects of MM tumorigenesis. In particular, MMBD has been studied in various models, and each model has unique features. As the general features of MM animal models have been reviewed elsewhere, the current review will focus on the features of MMBD animal models.
Multiple myeloma (MM) is a plasma cell (PC) malignancy that represents an accumulation of terminally differentiated monoclonal PC in the bone marrow (BM) (Katz, 2010). It is the second most hematological threat with a new case rate, 7.0 per 100,000/year based on 2013–2017 cases in the United States (National Cancer Institute: Surveillance, Epidemiology, and End Results Program, 2020). In 2020, it was estimated as 32,270 cases of MM (1.8% of all new cancer cases) and 12,830 deaths (2.1% of all cancer deaths) in the United States (National Cancer Institute: Surveillance, Epidemiology, and End Results Program, 2020). In almost all cases, MM is preceded by a premalignant disease known as monoclonal gammopathy of undetermined significance (MGUS) (Weiss et al., 2009; Landgren et al., 2019). During the progression of MGUS to MM, not only that complex genetic changes occur in the PCs, but also changes in the BM microenvironment (BME) occur, including angiogenesis, immune suppression, and increasing bone resorption (Kyle and Rajkumar, 2004). MM bone disease (MMBD) is defined as the presence of one or more osteolytic bone lesions or diffused osteoporosis with compression fracture attributable to the underlying clonal PC disorder (Rajkumar et al., 2014). MM is a paradigm of tumor–stroma interdependence, where MM cells establish tight contacts with the stroma. The osteoclast (OC) activity increases in areas adjacent to myeloma cells, whereas the osteoblast (OB) activity declines (Bjorkstrand et al., 2011). MMBD is found in 79% of patients with newly diagnosed MM patients (Kyle et al., 2003). A substantial improvement in overall survival was made in the past few decades. However, a significant number of patients still develop osteolytic lesions, marking a major pitfall under the current management (Silbermann and Roodman, 2013; Kumar et al., 2014; Ring et al., 2018). The lytic lesion is highly associated with MM’s devastating outcomes, including pathological fractures, debilitating pain, and increased mortality risk (Saad et al., 2007). An assessment of fracture effect on survival after MM diagnosis in the large Sweden population revealed that a fracture significantly reduced a survival risk (Thorsteinsdottir et al., 2020). Furthermore, MMBD often results in reducing patient’s quality of life and survival via hypercalcemia and spinal cord compression syndromes (Marino and Roodman, 2018).
MM therapy has significantly improved by an increased understanding of mechanisms that promote the disease’s onset and progression (Botta et al., 2017). Myeloma animal models contribute significantly by reproducing the pathological features and providing insight into the interactions between MM cells and the BME. In addition, these models serve as a tool to investigate and predict the effectiveness of novel therapeutic strategies that can translate into the clinic. Despite the significant advance in the understanding of disease pathophysiology and the availability of new therapeutics, MM is still considered an incurable disease (Anderson et al., 2002; Chatterjee et al., 2006; Tassone et al., 2006; Podar et al., 2009; Raab et al., 2009).
All animal models have benefits and limitations, and a single model cannot reproduce all pathophysiology. To select appropriate models for the study, we should consider many factors; an intact or deficient host immune system, the extent and severity of induced bone lesions, injection of human cell lines or primary human myeloma cells, similarity of the host microenvironment to human, and so on (Lwin et al., 2016). This review will focus on the highlights of these in widely used MM models on the aspect of MMBD.
The 5T mouse model of myeloma was initially reported by Radl et al. (1979), the most well-characterized syngeneic model. The model was established by serial transplantation of the BM of tumor-bearing mice into young syngeneic recipient C57BL/KaLwRij mice (Radl et al., 1988). It resembles features of the human benign MGUS stage (Radl, 1981). From this model, several mouse myeloma cell lines were established: 5T2MM, 5T33MM, and 5TGM1 (Radl et al., 1979; Radl et al., 1988; Manning et al., 1992; Asosingh et al., 2000).
The 5T2MM cells originate from spontaneously developed myeloma in elderly C57BL/KaLwRij mice (Radl et al., 1979; Radl et al., 1988). The model was generated by intravenously injecting BM cells from spontaneously developed MM mice into young syngeneic mice (Vanderkerken et al., 1999). This model can represent early human disease as it has moderate cell growth exhibiting slow tumor progression, which takes approximately 12–13 weeks post-transplantation. It comprises osteolytic lesions and trabecular bone loss from a reduction in OB number and an increase in OC number (Heath et al., 2009). It was the first animal model to evaluate a bisphosphonate effect on bone lesions (Radl et al., 1985). Zoledronic acid (Zol) is a potent anticatabolic nitrogen-containing bisphosphonate prescribed to high bone turnover patients. Croucher et al. (2003) demonstrated that Zol significantly reduces OC perimeter and bone lesions and increases trabecular bone and bone mineral density (BMD) in these mice compared to controls. Zol reduced a tumor burden and increased mouse survival (47 days from 35 days). Osteoprotegerin (OPG, a known inhibitor of osteoclastic bone resorption) was tested in the 5T2MM model as a therapeutic agent to reduce osteolytic bone disease in MM by inhibiting the interactions of the receptor activator of nuclear factor κB ligand (RANK) and its receptor (RANKL). Recombinant OPG was injected into a 5T2MM-bearing mouse that results in a significant reduction of bone lesions and OC and prevention of trabecular bone loss and increases of BMD and cortical bone thickness (Croucher et al., 2001). The dickkopf 1 (DKK1) has been shown to promote tumor cell–induced bone disease via inhibition of Wnt signaling. Furthermore, anti-DKK1 antibody treatment reduces trabecular and cortical bone loss and osteolytic lesions and increases the OB numbers and perimeter in 5T2MM-bearing mice (Heath et al., 2009). These collected results showed the use of 5T2MM model to explore MM development or bone disease–associated molecules, although the 5T2MM cells cannot be maintained in in vitro system as they are dependent on the BME.
Other 5T sub-cell lines have also been used in C57BL/KaLwRij mice to mimic human disease. 5T33MM cell line is another well-characterized model. This model represents an “aggressive” MM model due to rapid tumor growth (Asosingh et al., 2000). This model resembles advanced or relapsed MM phenotypes compared to the 5T2MM model. 5T33MM cells are no longer dependent on the BM environment and could grow in the in vitro system (Asosingh et al., 2000). Several studies had evaluated the therapeutic benefits of chemotherapies and cytotoxic agents in this model to target tumor burden (Khong et al., 2008; Xu et al., 2012). However, the 5T33MM model did not exhibit bone disease following tumor transplantation but showed destruction of cortical bone and reduced trabeculae at invaded sites (Vanderkerken et al., 1997). Subsequent studies showed no evident osteolytic lesions in the 5T33MM model (Buckle et al., 2012; Deshet-Unger et al., 2016).
The 5TGM1 model is a subsequent subclone of the 5T33 line. It was established via serial in vivo passage of the 5T33MM cells, used to investigate the mechanisms underlying MM-induced bone disease, as well as tumor burden (Garrett et al., 1997). Because of its short latency period, the 5TGM1 model can be used to test drugs to target both tumor burden and bone disease, which makes this model a cost-effective and reproducible model of human MM disease. The main advantage of the 5TGM1 model is its “pronounced” osteolytic lesion formation (Asosingh et al., 2000). Several studies have been made to target MMBDs in the 5TGM1 model. One study showed the effect of bisphosphonate ibandronate (IB) upon bone disease in the 5TGM1 model. 5TGM1-bearing mice treated with IB showed a reduction in osteolytic lesions in their vertebrae and long bones (Dallas et al., 1999). It also showed that IB also prevented trabecular and cortical bone loss. Systemic injection of 5TGM1-GFP cells into C57BL/KaLwRij mouse allowed tracing of individual myeloma cells and showed that they colonize the endothelial niche, entering a dormant stage. This dormant stage can be switched “on” by bone lining cells/OBs or “off” by OC. It may contribute to chemotherapy resistance, which targets divided cells (Lawson et al., 2015a). Furthermore, a recent study demonstrated that naive C57BL6/KaLwRij female mice injected with 5TGM1 producing small extracellular vesicle showed an increase in osteolysis, resulting in a significant reduction in trabecular bone volume without 5TGM1 cells. Furthermore, combination therapy of GW4869 (exosome secretion inhibitor) and BTZ in this model reduced tumor burden and bone lesions (Faict et al., 2018).
5T cell–bearing C57BL/KaLwRij model is the most studied animal model as it reproduces many features of human MM features, including MMBD. It has an intact immune system. Despite these benefits, it represents a single clonal murine MM-like disease, whereas human MM is highly heterogeneous.
Another murine MM model, the Myc/Bcl-XL mouse, was generated by the crossing hemizygous Myc transgenic mouse to hemizygous Bcl-XL transgenic mouse (Cheung et al., 2004). It developed PC tumors in a relatively short period (average of 135 days) and 100% incidence. The mouse showed malignant PC infiltration into the BM, production of monoclonal immunoglobulin, and various osteolytic lesions (Cheung et al., 2004). This transgenic model is extensively used as it entails overexpression of recognized MM oncogenes under the control of an immunoglobulin enhancer.
Constitutive expression of a potent oncogene, Myc, in early B cells caused extramedullary lymphoma in Vk-Myc mouse. Vk-Myc mouse was developed under the control of mouse immunoglobulin κ (Igκ) light-chain gene-regulatory elements (Robbiani et al., 2005). Thereafter, Chesi et al. introduced early stop codon at Myc locus in Vk-Myc mouse to prevent its expression. Therefore, Myc can be sporadically activated in post-germinal B cells due to somatic hypermutation, resulting in the transition from the spontaneous MGUS to a disease that recapitulates the biological and clinical features of human MM. This model showed murine MM phenotype analogous to human MM disease with an indolent phenotype at the early stage of the disease and developed skeleton lesions compared to sex- and age-matched littermates (Chesi et al., 2008). A subsequent study performed a BM transplantation from Vk∗MYC mouse into sub-lethally irradiated congenic C57BL/6 mouse as a model representing relapsed refractory MM (Chesi et al., 2012). Comparable to the 5T cells, two bortezomib-resistant myeloma cell lines, Vk12653 and Vk12598, were established from aged Vk∗MYC mouse and develop myeloma after engraftment into young syngeneic mice. The Vk12598 cell line completely responded to melphalan, while it showed partial response against doxorubicin treatment (Lwin et al., 2016). The VK∗MYC mouse with these cell lines demonstrated the in vivo exploration of single and combinatorial therapy (Chesi et al., 2012). These models had been used to study the pathogenesis and the development of preclinical drugs, although skeletal evaluations were limited (Chesi et al., 2012; Matthews et al., 2013). Although it is out of this review’s scope, another beneficial feature of the Vk∗MYC model is imitating the accessible cross-talk among clonal PCs and nearby microenvironment. This characteristic allows for studying the clonal dynamics of the disease. A hypothesis of MM onset is based on the survival of competition among different clones at an early stage, which drives toward apathetic condition (Morgan et al., 2012).
The Vk∗MYC model emphasizes MYC’s multifaceted roles in MM commencement and growth and allows further modifications of MM progressor genes, transcription factors, adhesion molecules, and bone destruction mediators.
XBP-1, a transcription factor, is required for PC differentiation and is overexpressed in MM cells compared to normal PCs (Iwakoshi et al., 2003). The Eμ-xbp-1s transgenic model was developed by the Eu-directed expression of the spliced XBP-1 isoform (XBP-1s) (Carrasco et al., 2007). This mouse developed an MM-like disease by 280 days with changes in their skin, urinary cast, paraprotein production, splenomegaly, increased BM PCs, and shortened life span. The Eμ-xbp-1s mouse developed features such as elevated serum IgM and IgG, PC expansion ranging from 5 to 30% of total BM cellularity in the BM, and plasmacytic tumor development human MGUS or MM, and eventually lytic bone lesions. These features closely resemble MGUS and a significant proportion of MM progression.
Interestingly, this mouse’s disease appeared to advance with people from an MGUS-like state to an asymptomatic MM-like illness state by age. MM’s improvement’s long inertness period proposes that extra hereditary lesions are needed for full dangerous change. The transcriptional profiles of the lymphoid and MM cells show the liberation of qualities with known dysfunction in human MM, including cyclin D1, gp130, MAF, MAFB, CEBPs, BAFF, and APRIL (Carrasco et al., 2007).
Recombinase-Activating Gene-2 (RAG-2) deficient mice with impaired T and B cell development were employed to examine the BME (Fowler et al., 2009). Engraftment of 5TGM1 cells into RAG-2–/– mouse resulted in myeloma development, associated with tumor growth within the bone and osteolytic bone disease and other features similar to humans. This study generated a double deficient (RAG2–/–MMP-9–/–) mouse and demonstrated significant reductions of myeloma cells in the BM and OC number in the 5TGM1 bearing RAG2–/–MMP-9–/– mouse (Fowler et al., 2009). Postnov et al. (2009) generated two human MM cells, U266 and RPMI-8226/S, with the luciferase-GFP genes and engrafted these cells into total-body irradiated RAG2–/–γc–/– mice at 24 h before transplantation. It showed that MM cells spread across the skeleton and severe bone lesions (Postnov et al., 2009).
A study demonstrated that the human immunoglobulin was produced in severe combined immunodeficient (SCID) mice engrafted with human peripheral blood mononuclear cells (Cavacini et al., 1992). It reduced the concern about the inconsistency of the host/donor associated features by human cell engraftment into mice and expanded a xenograft study. Many xenograft MM models are generated using various immune-compromised mice, including SCID, non-obese diabetic (NOD)/SCID, NOD.Cg-PrkdcscidIl2rgTM 1Sug/ShiJic (NOG), and NOD.Cg-PrkdcscidIl2rgTM 1Wjl/SzJ mouse (NSG). In the absence of a mouse immune system, these xenograft models have been used to develop MM via systemic injection or orthotopic injection with human MM cell lines or patient BM/MM cells. These models offer the opportunity to evaluate human MM cells in the BME and test therapeutics’ effectiveness against human myeloma in vivo. But these models have limitations that do not reproduce complete tumor environments. A number of studies exhibited their abilities to reproduce many key features of human MM. Many studies have tested anti-tumor and bone-modulating drugs to target MM in vivo, and some have identified key molecules of bone destruction.
A variety of different myeloma cell lines including RPMI-8226 (Mitsiades et al., 2003; Wu et al., 2007; Thirukkumaran et al., 2012; Kikuchi et al., 2013), U266 (Mirandola et al., 2011; Kikuchi et al., 2013), KMS-11 (Carlo-Stella et al., 2006), KMS-12- BM (Rabin et al., 2007), and MM.1S (Wu et al., 2007; Azab et al., 2014; Roccaro et al., 2014; Swami et al., 2014; Zhang et al., 2014), as well as primary patient-derived myeloma cells (Pilarski et al., 2000), have been transplanted into SCID and NOD/SCID mice.
Human GFP expressing RPMI-8226/S MM cells was intravenously injected into SCID/NOD mice (Mitsiades et al., 2003). Whole-body constant fluorescence imaging was conducted to identify the location of GFP+ MM cells. Their anatomical dispersion and pathophysiological indications were agreed to the clinical course of MM in human patients, i.e., the pivotal skeleton (e.g., spine, skull, and pelvis) damage and advancement of loss of motion auxiliary to spinal lesions. This work first described the diffuse bone infiltration of human MM and a sequential bone disease progression. It subsequently provided a significant in vivo framework for the axial skeleton study.
Human myeloma cell line, U266, was intravenously injected into NOG mice, and all of these animals developed hindlimb paralysis after transplantation. Conversely, U266 cells in SCID or NOD/SCID mice did not show any myeloma features. In these mice, osteolytic injuries in cortical bones and loss of trabecular bones were found (Miyakawa et al., 2004). It demonstrated that this hu-myeloma NOG model might help study the pathogenesis of myeloma and related osteolytic lesions. NOD/SCID mice were intratibially injected with luciferase-expressing U266 cells and evaluated bortezomib, melphalan, aminopeptidase inhibitor, and histone deacetylase inhibitor for its applicability on preclinical drug tests (Fryer et al., 2013). Treatment with these drugs reduced the augmentation in all disease markers in mice. In addition, this model demonstrated successful myeloma development with primary myeloma cells from PC leukemia.
NOD/SCID animals with the luciferase-expressing myeloma cell (Luc+ MM1S) injection were treated with bone-targeting nanoparticles and evaluated MM progression and MM-induced bone lesions (Swami et al., 2014). This model demonstrated the tremendous potential of the bone-targeted drugs in the BME. The study suggests that NOD/SCID models can adapt interactions between MM cell and BME.
The OPG role was studied in co-transplantation of OPG overexpressing mesenchymal stem cells (MSCs) and KMS-12-BM cell into β2m NOD/SCID mouse (Rabin et al., 2007). This study demonstrated that OPG expressing MSCs caused the reduction in trabecular bone loss in the vertebrae and tibiae of myeloma developed animals.
A SCID mouse with intratibially transplanted 5TGM1 cells developed all myeloma features (Mori et al., 2004). BM stromal cells (BMSCs) from the 5TGM1-injected tibias and 11 MMBD (stage 3) patients express high levels of transcription repressor growth factor independence-1 (Gfi1) to suppress Runx2 via epigenetic changes in the Runx2 promoter (D’Souza et al., 2011). This report demonstrated that Gfi1 is a transcriptional repressor of the OB transcription factor Runx2 under osteogenic conditions, thus preventing osteoblastogenesis in MMBD.
A variety of myeloma cell lines, JJN3 (Lawson et al., 2015b), OPM2 (Fuhler et al., 2012; Lawson et al., 2015b), U266 (Dewan et al., 2004; Miyakawa et al., 2004; Bartee et al., 2012a; Bartee et al., 2012b; Lawson et al., 2015b), RPMI-8226-Luc (Hurchla et al., 2013), MM.1S (Bartee et al., 2012a), HuNS1 (Bartee et al., 2012a), L363 (Udi et al., 2013), and KMM-1 (Dewan et al., 2004), as well as primary patient-derived MCs (Lawson et al., 2015b), have been administered to NSG mice, which results in classical features of MM including paraplegia, paraprotein in the serum, osteolytic lesions, and loss of trabecular bone. A study performed intravenous injection of JJN3, OPM2, and U266 cell lines into NSG mice and demonstrated steady improvements of osteolytic bone lesions without dissemination outside of the BM in these models. Bone loss has been observed after Zol or Carfilzomib treatment with the RPMI-8226 cell engraftment. This study showed that Zol treatment reduced bone damage and tumor burden, whereas Carfilzomib reduced tumor burden alone (Lawson et al., 2015b). Another study showed that the Oprozomib, an oral proteasome inhibitor, reduced tumor burden, tibial trabecular bone loss, decreased serum c-terminal telopeptide (a bone resorption marker), and increased procollagen I N-terminal propeptide (a bone formation marker) levels in RPMI-8226-Luc–bearing NSG mice (Hurchla et al., 2013). The Osteolytica is a newly developed image analysis software to analyze three-dimensional (3D) cancer-induced osteolytic lesions in mice and evaluated U266-bearing NSG model (Evans et al., 2016). It showed that NSG mice are reliable in vivo model for improved clinical representation. In U266-GFP-Luc cell–transplanted NSG mice, MMBD was serially evaluated using in vivo micro–computed tomography (microCT), and it showed the antiresorptive capacity of transforming growth factor β (TGF-β) receptor I kinase inhibitor (SD-208) by prevention and treatment of myeloma bone lesions via enhancing collagen matrix maturation (Green et al., 2019). A combination treatment of anti–TGF-β–neutralizing antibody (1D11) and Zol was tested in the JJN3 and U266 intravenously transplanted NSG mice using ex vivo microCT and in vivo bone assessment at right tibias (Paton-Hough et al., 2019). In both models, the combination therapy showed the prevention of myeloma-induced bone lesions and bone formation from established bone lesions. This study suggests the combined antiresorptive and bone anabolic therapy as a new regimen for the patient. This study examined only tibias of the treated mice. NSG mouse is a potential model to demonstrate the role of cell adhesion molecules such as CD166 in MM progression. CD166 is highly expressed in various MM cell lines and MM patients’ primary BM cells. H929-GFP cells with CD166 were systemic and orthotopically injected into irradiated NSG mice. These models showed that CD166 affects MM progression by manipulating bone remodeling and decreasing trabecular thickness and bone volume, leading to bone lesions (Xu et al., 2016).
The main disadvantage of these models is the use of tumor cell lines. Most of the myeloma cell lines had been derived from the extramedullary MM stage or undergone aggressive transformations. They may not represent the typical apathetic stage of MM, although there were several attempts to use the primary MM cells to evade such limitations. These primary cells were also from relatively late stages, probably due to the low take rate of early-stage primary MM cells.
The SCID-hu model provides a human microenvironment to grow myeloma cells in the mouse and allow primary myeloma cells to grow. Human myeloma cells were inoculated into the human fetal bone chip implanted into irradiated SCID mice in this model (McCune et al., 1988). The advantage of this model is that human myeloma cells will grow in a human BME rather than a mouse.
Urashima et al. (1997) first showed engraftment and proliferation of human MM cell lines (ARH-77, OCI-My5, U-266, or RPMI-8226) in BM cavity of human fetal bone chips implanted into irradiated SCID mice and the MM cells homing exclusively to the human BM, but not in the murine BM. Afterward, Yaccoby et al. demonstrated that engrafted purified primary cells from MM patients in SCID-hu allow expansion and the development of several MM manifestations, including bone lesions, human paraprotein, and high blood calcium levels. Newly formed blood vessels were found in areas infiltrated by MM cells, demonstrating active angiogenesis (Yaccoby et al., 1998). Another study from the same group reported that inhibitor treatments for OC or the RANKL on the primary myeloma cells bearing SCID-hu reduced bone resorption and tumor burden, but such treatments to the mice with primary myeloma cells from the extramedullary disease patient did not affect this (Yaccoby et al., 2002). The SCID-hu model showed engrafting primary myeloma cells from medullary MM cells with slower growth rates and BM dependency (Li et al., 2007). Although these MM cells were hyperdiploid and grew strictly in the BM, these myeloma cells were originated from high-risk myeloma. Although the SCID-hu model with primary MM cells is biologically relevant to study MM pathophysiology, a limited mouse number (2–4 mice) produced from each patient sample is hard to test any therapeutic drug. Inoculation of INA-6 cells [interleukin 6 (IL-6)–dependent human MM cell line] into SCID-hu mice resulted in tumor engraftment and burden in the implanted bone with an increase in soluble human IL-6 receptor (shuIL6R) and human IL-6 in this mouse (Tassone et al., 2005). Although skeletal effects were not evaluated, many studies were performed in the INA-6 SCID-hu model for the anti-MM activity of the anti-inflammatory drug atiprimod (Neri et al., 2007), the anti-DKK1 monoclonal antibody BHQ880 (Fulciniti et al., 2009), the IκB kinase inhibitor, β-MLN120B (Hideshima et al., 2006), and a telomerase inhibitor (Shammas et al., 2008).
Although the SCID-hu MM model remains a consistent model to review the human disease and offers an important preclinical model, some boundaries remained: the allogeneic nature of the fetal BM environment versus MM cells and the genetic heterogeneity of implanted bone chips of human with patient MM cells collected from different individuals at dissimilar ages.
The SCID-rab model employs a rabbit bone subcutaneously implanted into SCID mice to avoid human fetal bone use (Yata and Yaccoby, 2004). The successful engraftment of BM cells from MM patients or CD138+ PCs into the SCID-rab reproduced matching M-protein isotype production and other myeloma clinical signs, including severe bone resorption of the implanted rabbit bone. The myeloma cells grew exclusively in the rabbit bone, whereas the myeloma cells from extramedullary disease patients grew on the outer surface of the implanted rabbit bone (Yata and Yaccoby, 2004). The authors claimed that the SCID-rab model presents a consistent and efficient model. As a rabbit is phylogenetically close to primate than a rodent, rabbit bone use was scientifically reasonable. The microenvironment of a rabbit bone can maintain the sustained primary human MM cell growth from most of the patients they tried.
For the study associated with MMBD, an important weakness of the model is the evident species difference between the rabbit bone and the human adult bone and the possible inconsistency of interactions of human MM cells with rabbit bone.
The SCID-synth-hu was developed to conquer the inadequacy of the SCID-hu system (Calimeri et al., 2011; DeWeerdt, 2011). This model is based on implanting a 3D bone-like poly-caprolactone polymeric scaffold into an SCID mouse. This model reproduces the microarchitecture of a normal human femur adult bone and permits the efficient coating of the 3D scaffold internal surface with human BM stem cells that produce the environment that effectively support the human primary MM cells engraft/growth. It should be noted that the allogeneic nature of the settings overcomes limitations associated with the SCID-hu model. The model achieved primary MM cells engraftment within the microenvironment that characterizes a significant development in the accessibility of prevailing in vivo environments. Preclinical evaluations of anti-MM drugs demonstrated considerable inhibition of the MM growth in vivo. Because of a lack of natural bone, bone absorption was not studied in this model.
Bone disease is a significant component of MM. Many factors have been reported in the pathogenesis of MMBD. These factors play roles in tumor development and endurance. The association of PCs with BMSCs in the BME is critical for OC’s activation and proliferation (Terpos et al., 2003). OC stimulating factors were first described in 1974 (Mundy et al., 1974). Thereafter, many factors have been reported to be involved in the pathogenesis of MMBD. Although IL-1β and tumor necrosis factor α (TNF-α) are potent cytokines observed in MM patients, the IL-1β protein was not detected in clonal PCs from patients with both MM and MGUS, whereas TNF-α protein and mRNA were detected. Sati et al. (1999) concluded that myeloma cells produce TNF-α but not IL-1β. But a later study showed that elevated IL-1β mRNA levels were positively associated with bone lesions in patient biopsies (Donovan et al., 2002). Further research for the role of elevated IL-1β transcripts may be necessary.
BMSC secretes IL-6 after their interaction with myeloma cells. Also, IL-6 is an intense OC-stimulating factor for OC progenitor and induces bone resorption via OC (Reddy et al., 1994). Its essential impact on OC is an expansion of the early OC pool. However, studies have shown that TGF-β1 is also produced by BMSCs and can regulate IL-6 secretion by several tissues, including BMSCs. Furthermore, anti–TGF-β1 monoclonal antibody treatment blocked IL-6 secretion by BMSCs and also inhibited the increments in IL-6 secretion by BMSCs induced by MM cell adhesion (Urashima et al., 1996), suggesting the role of TGF-β1 in tumor cell proliferation.
TNF is found in the supernatant of PC cultures from MM patients, and hepatocyte growth factor (HGF) makes OC-like cells secrete IL-11. As TGF-β1 and IL-1 overload the impact of HGF on IL-11 production, it was postulated that HGF from myeloma cells induces MMBD via IL-11 (Hjertner et al., 1999). Later, elevated serum HGF levels were found in MM patients (Seidel et al., 2002).
Many studies reported that myeloma cells secrete factors that influence OB, OC, or both. The PC analyses from the patients with myeloma and control subjects revealed unique factors related to osteolytic lesions (Tian et al., 2003). This seminal work narrowed down to four genes significantly overexpressed in the patient PCs with magnetic resonance imaging–detected focal lesions. One of these genes, DKK1, was further investigated because of its known OB function. Immunohistochemical examination on BM biopsy revealed that only myeloma cells contained discernible DKK1. High DKK1 levels in BM plasma and patient blood were correlated to DKK1 expression and associated with the focal bone lesions. Recombinant human DKK1 or high DKK1-containing serum restrained OB differentiation in in vitro test.
High levels of macrophage inhibitory protein-1α (MIP-1α) were found in marrow samples from patients with MM but not in marrow from patients with other hematologic disorders or controls (Choi et al., 2000). Treatment of neutralizing antibody to MIP-1α to human BM cells with MM patients’ marrow plasma prohibited OC differentiation. These results support that MIP-1α plays an important role as the major factors responsible for the increased OC formation in patients with active MM. Furthermore, human MIP-1α enhanced OC formation from OC progenitors in combination with IL-6, parathyroid hormone–related protein, and RANKL at the later stages of OC differentiation (Han et al., 2001).
Vascular endothelial growth factor (VEGF) and its receptor, VEGFR, is upregulated in myeloma BM. VEGF induces vascularization and promotes angiogenesis with the progression of MGUS, leading to myeloma development (Ria et al., 2020). It has been reported that interaction of VEGF secreted by MM cells with OC-producing osteopontin not only increases vascular tubule formation but also induces osteoclastogenic activity by vascular endothelial cells. Thus, the interactions of myeloma cell, OCs, and vascular endothelial cells play a critical role in bone lesions, angiogenesis, and myeloma development (Tanaka et al., 2007). Further study revealed that VEGF binds to its receptor in the OCs and directly increases bone resorption and survival of mature OCs. There is also a positive feedback loop between VEGF expression by MM cells and IL-6 production from stromal cells (Voskaridou and Terpos, 2004).
Understanding the role of the RANKL/RANK/OPG system in bone remodeling provided a new perspective to pathophysiological studies of MMBD. RANK, RANKL, and the decoy receptor, OPG, are responsible for the regulation of OC production and activity. RANKL expressed in T lymphocytes, OBs, osteocytes, and bone stroma cells interacts with RANK secreted by OC precursor cells. The RANK–RANKL axis plays an important role in bone resorption (Voskaridou and Terpos, 2004; Boyce and Xing, 2008; Walsh and Choi, 2014). OPG, a blocker of RANKL-RANK interaction, inhibits activation and differentiation of OC. In fact, bone resorption can be dependent on the balance between RANKL and OPG expression. Studies have shown that RANKL expression in preosteoblastic or stromal cells in the coculture system is increased by human myeloma cells at both mRNA and protein levels, whereas OPG is downregulated (Voskaridou and Terpos, 2004). Studies have shown that serum RANKL/OPG ratios can be considered a potential prognostic marker in MM patients. A greater ratio represents the formation and activation of OCs, leading to shorter survival. Moreover, soluble RANKL level is associated with the extent of bone disease (Sezer et al., 2003; Raje et al., 2019).
Sclerostin produced by osteocytes binds to Wnt coreceptor, LRP5/6, and inhibits the canonical Wnt pathway (Delgado-Calle et al., 2017). MM patients have high serum sclerostin, whereas MGUS and smoldering MM patients have only low levels (Eda et al., 2016). Sclerostin serum level is positively correlated with reduced OB activity and poor survival (Terpos et al., 2012). The osteocytes in JJN3 intratibially injected mice expressed high sclerostin levels and significant bone lesions (Delgado-Calle et al., 2016). In addition, the coculture of osteocytes with myeloma cells showed substantial reductions of OPG and OB differentiation markers. These data provided evidence of interactions among Wnt signaling, OB differentiation, and sclerostin. Besides, coculture of OBs from MM patients with INA-6 or H929 MM cells showed that MM cells inhibit OB differentiation via sclerostin from DKK-1–stimulated premature OBs (Eda et al., 2016). Treatment with antisclerostin increased femur trabecular bone volumes in 5TGM1-bearing or naive C57BL/KaLwRij mice and suppressed MM1S tumor progression in SCID (Reagan et al., 2015). A subsequent study demonstrated that antisclerostin treatment prevented myeloma-induced inhibition of bone formation, but did not affect osteoclastic resorption or tumor burden. A combined treatment with antisclerostin and Zol showed additive effects (McDonald et al., 2017).
Studying the pathophysiology of MMBD provides a better understanding of underlying mechanisms responsible for bone remodeling imbalance, leading to proper design and selection of animal models for the study. These efforts resulted in developing targeted drugs not only for MMBD but also for other bone metastasis disease. The RANKL rivals, OPG-Fc and RANKL-Fc, showed inhibition of the RANKL activity (Schwarz and Ritchlin, 2007). As AMG162, a human monoclonal antibody against RANKL, demonstrated compelling data in diminishing bone turnover markers in postmenopausal women (Bekker et al., 2004), AMG162 has been under scrutiny in clinical preliminaries. Denosumab, AMG162, was a well-tolerated drug and reduced significant and prolonged reduction of bone resorption in MM, as well as bone metastases from breast cancer (Body et al., 2006). A recombinant OPG, AMGN-0007, was developed as a potential therapeutic agent in bone disease. A single dose of AMGN-0007 showed significant decreases of urinary N-telopeptide of collagen/creatine in MM patients (Body et al., 2003).
Animal models allow specific hypothesis-driven researches and enable researchers to address specific questions. Syngeneic models of the tumor are the models in which the tumor cell line is derived from the same species as a recipient. Therefore, it is easy to test the efficacy of immuno-oncology agents as single therapies or in combination with other anticancer drugs. The syngeneic model has several advantages that include the use of mice with an intact immune system. Besides, these models demonstrate high tumor cell engraftment rates and low variability. These are beneficial to study the efficacy of novel antitumor agents. Many MM models have been developed and allowed to interrogate the mechanism of MM tumorigenesis in murine backgrounds. But these models represent a single clonal MM-like disease in the murine system, whereas human MM is a highly heterogeneous disease. Such limitation leaves gaps of understanding of human MM and limits of translation to the clinic.
Employing immunodeficient mice with human MM cell transplantation was tried to human MM cells and reproduced many human MM features. Human MM cell lines and even patient MM cells used in these models represent the aggressive MM stage as transplanted myeloma cells were transformed during passages and/or obtained from the late stages of MM. Besides, a discrepancy of human myeloma cells from mouse BME leads to limit its reproducibility. Xenograft models are functional tools for the preliminary selection of novel drugs predominantly in the absence of pharmacological information, but they are not considered as perfect models for the development of anti-MM drugs. Indeed, the concerns on the non-human host stroma have been increased in relation to the pathophysiological condition in patients. Therefore, mouse xenograft models generally serve as a useful tool for studying the significant antitumor activity and a potent anti-MM drug’s pharmacodynamic assets.
The models from the engraftment of human primary MM cells in a human BME can prevail over the weaknesses of conventional xenografts or genetically modified mice. The SCID-hu models are useful to investigate the disease pathophysiology and evaluate new drugs that target the tumor and the human BME.
We summarized the animal models with their strengths and limitations in the summary Table 1. While we review, we noticed that many studies were done in the femur, tibia, or implanted bone chips rather than the spine, one of the sites frequently found at the clinic and significantly impacting patient’s life quality. The femur or tibia was chosen because it has a relatively simple structure and is accessible to an orthotopic injection. Besides, reports showed relatively mild bone damage at the spine than tibia due to systemic infusion of myeloma cells. The MMBD at the spine may not be the same as the ones at the femur or tibia. It will be worth investigating in future studies.
Table 1. Classification of immunocompetent and immunocompromised animal models of MMBD with strengths and shortcomings.
Bisphosphonates are the only approved treatment for the prevention of MMBD. This anti-absorption therapy does not promote bone formation and cannot correct the established lesion. Current MMBD therapy is still aimed at pain control and prevention of skeletal fractures because of widespread belief that bone lesions do not heal. Recently, several clinical observations revealed that myeloma-induced bone lesions could be recovered (Hinge et al., 2016; Mohan et al., 2017, 2021). Such observations raise a novel question—why some patients heal while others do not. As we previously described, bone anabolic drugs can potentially benefit patients who already have established bone lesions. We found that multidisciplinary efforts from preclinical and clinical sides have been made. Such efforts promise that new bone anabolic drugs will be available soon for MMBD patients.
At present, there is no perfect model for the study of MMBD. Therefore, a thorough understanding of each model’s advantages and disadvantages is necessary to choose an appropriate model to study or test the specific question(s).
SHM wrote the initial draft. SN revised and wrote several sections. SJM wrote a part of the section. CM and LM wrote sections of this article and edited the manuscript. DY planned, revised, and finalized this article. All authors contributed to the article and approved the submitted version.
This work was supported by the National Institute of Health (1 R21 OD026618) and the National Institute of General Medical Sciences of the National Institutes of Health under Award No. P20GM125503.
The authors declare that the research was conducted in the absence of any commercial or financial relationships that could be construed as a potential conflict of interest.
Anderson, K. C., Shaughnessy, J. D. Jr., Barlogie, B., Harousseau, J. L., and Roodman, G. D. (2002). Multiple myeloma. Hematol. Am. Soc. Hematol. Educ. Program 2002, 214–240.
Asosingh, K., Radl, J., Van Riet, I., Van Camp, B., and Vanderkerken, K. (2000). The 5TMM series: a useful in vivo mouse model of human multiple myeloma. Hematol. J. 1, 351–356. doi: 10.1038/sj/thj/6200052
Azab, A. K., Sahin, I., Moschetta, M., Mishima, Y., Burwick, N., Zimmermann, J., et al. (2014). CXCR7-dependent angiogenic mononuclear cell trafficking regulates tumor progression in multiple myeloma. Blood 124, 1905–1914. doi: 10.1182/blood-2014-02-558742
Bartee, E., Chan, W. M., Moreb, J. S., Cogle, C. R., and McFadden, G. (2012a). Selective purging of human multiple myeloma cells from autologous stem cell transplantation grafts using oncolytic myxoma virus. Biol. Blood Marrow Transplant. 18, 1540–1551. doi: 10.1016/j.bbmt.2012.04.004
Bartee, E., Meacham, A., Wise, E., Cogle, C. R., and McFadden, G. (2012b). Virotherapy using myxoma virus prevents lethal graft-versus-host disease following xeno-transplantation with primary human hematopoietic stem cells. PLoS One 7:e43298. doi: 10.1371/journal.pone.0043298
Bekker, P. J., Holloway, D. L., Rasmussen, A. S., Murphy, R., Martin, S. W., Leese, P. T., et al. (2004). A single-dose placebo-controlled study of AMG 162, a fully human monoclonal antibody to RANKL, in postmenopausal women. J. Bone Miner. Res. 19, 1059–1066. doi: 10.1359/JBMR.040305
Bjorkstrand, B., Iacobelli, S., Hegenbart, U., Gruber, A., Greinix, H., Volin, L., et al. (2011). Tandem autologous/reduced-intensity conditioning allogeneic stem-cell transplantation versus autologous transplantation in myeloma: long-term follow-up. J. Clin. Oncol. 29, 3016–3022. doi: 10.1200/JCO.2010.32.7312
Body, J. J., Facon, T., Coleman, R. E., Lipton, A., Geurs, F., Fan, M., et al. (2006). A study of the biological receptor activator of nuclear factor-kappaB ligand inhibitor, denosumab, in patients with multiple myeloma or bone metastases from breast cancer. Clin. Cancer Res. 12, 1221–1228. doi: 10.1158/1078-0432.CCR-05-1933
Body, J. J., Greipp, P., Coleman, R. E., Facon, T., Geurs, F., Fermand, J. P., et al. (2003). A phase I study of AMGN-0007, a recombinant osteoprotegerin construct, in patients with multiple myeloma or breast carcinoma related bone metastases. Cancer 97 3(Suppl.), 887–892. doi: 10.1002/cncr.11138
Botta, C., Ciliberto, D., Rossi, M., Staropoli, N., Cuce, M., Galeano, T., et al. (2017). Network meta-analysis of randomized trials in multiple myeloma: efficacy and safety in relapsed/refractory patients. Blood Adv. 1, 455–466. doi: 10.1182/bloodadvances.2016003905
Boyce, B. F., and Xing, L. (2008). Functions of RANKL/RANK/OPG in bone modeling and remodeling. Arch. Biochem. Biophys. 473, 139–146.
Buckle, C. H., De Leenheer, E., Lawson, M. A., Yong, K., Rabin, N., Perry, M., et al. (2012). Soluble rank ligand produced by myeloma cells causes generalised bone loss in multiple myeloma. PLoS One 7:e41127. doi: 10.1371/journal.pone.0041127
Calimeri, T., Battista, E., Conforti, F., Neri, P., Di Martino, M. T., Rossi, M., et al. (2011). A unique three-dimensional SCID-polymeric scaffold (SCID-synth-hu) model for in vivo expansion of human primary multiple myeloma cells. Leukemia 25, 707–711. doi: 10.1038/leu.2010.300
Carlo-Stella, C., Guidetti, A., Di Nicola, M., Longoni, P., Cleris, L., Lavazza, C., et al. (2006). CD52 antigen expressed by malignant plasma cells can be targeted by alemtuzumab in vivo in NOD/SCID mice. Exp. Hematol. 34, 721–727. doi: 10.1016/j.exphem.2006.03.005
Carrasco, D. R., Sukhdeo, K., Protopopova, M., Sinha, R., Enos, M., Carrasco, D. E., et al. (2007). The differentiation and stress response factor XBP-1 drives multiple myeloma pathogenesis. Cancer Cell 11, 349–360. doi: 10.1016/j.ccr.2007.02.015
Cavacini, L. A., Kennel, M., Lally, E. V., Posner, M. R., and Quinn, A. (1992). Human immunoglobulin production in immunodeficient mice: enhancement by immunosuppression of host and in vitro activation of human mononuclear cells. Clin. Exp. Immunol. 90, 135–140. doi: 10.1111/j.1365-2249.1992.tb05845.x
Chatterjee, M., Chakraborty, T., and Tassone, P. (2006). Multiple myeloma: monoclonal antibodies-based immunotherapeutic strategies and targeted radiotherapy. Eur. J. Cancer 42, 1640–1652. doi: 10.1016/j.ejca.2006.02.016
Chesi, M., Matthews, G. M., Garbitt, V. M., Palmer, S. E., Shortt, J., Lefebure, M., et al. (2012). Drug response in a genetically engineered mouse model of multiple myeloma is predictive of clinical efficacy. Blood 120, 376–385. doi: 10.1182/blood-2012-02-412783
Chesi, M., Robbiani, D. F., Sebag, M., Chng, W. J., Affer, M., Tiedemann, R., et al. (2008). AID-dependent activation of a MYC transgene induces multiple myeloma in a conditional mouse model of post-germinal center malignancies. Cancer Cell 13, 167–180. doi: 10.1016/j.ccr.2008.01.007
Cheung, W. C., Kim, J. S., Linden, M., Peng, L., Van Ness, B., Polakiewicz, R. D., et al. (2004). Novel targeted deregulation of c-Myc cooperates with Bcl-X(L) to cause plasma cell neoplasms in mice. J. Clin. Invest. 113, 1763–1773. doi: 10.1172/JCI20369
Choi, S. J., Cruz, J. C., Craig, F., Chung, H., Devlin, R. D., Roodman, G. D., et al. (2000). Macrophage inflammatory protein 1-alpha is a potential osteoclast stimulatory factor in multiple myeloma. Blood 96, 671–675.
Croucher, P. I., De Hendrik, R., Perry, M. J., Hijzen, A., Shipman, C. M., Lippitt, J., et al. (2003). Zoledronic acid treatment of 5T2MM-bearing mice inhibits the development of myeloma bone disease: evidence for decreased osteolysis, tumor burden and angiogenesis, and increased survival. J. Bone Miner. Res. 18, 482–492. doi: 10.1359/jbmr.2003.18.3.482
Croucher, P. I., Shipman, C. M., Lippitt, J., Perry, M., Asosingh, K., Hijzen, A., et al. (2001). Osteoprotegerin inhibits the development of osteolytic bone disease in multiple myeloma. Blood 98, 3534–3540.
Dallas, S. L., Garrett, I. R., Oyajobi, B. O., Dallas, M. R., Boyce, B. F., Bauss, F., et al. (1999). Ibandronate reduces osteolytic lesions but not tumor burden in a murine model of myeloma bone disease. Blood 93, 1697–1706.
Delgado-Calle, J., Anderson, J., Cregor, M. D., Hiasa, M., Chirgwin, J. M., Carlesso, N., et al. (2016). Bidirectional Notch Signaling and Osteocyte-Derived Factors in the Bone Marrow Microenvironment Promote Tumor Cell Proliferation and Bone Destruction in Multiple Myeloma. Cancer Res. 76, 1089–1100. doi: 10.1158/0008-5472.CAN-15-1703
Delgado-Calle, J., Sato, A. Y., and Bellido, T. (2017). Role and mechanism of action of sclerostin in bone. Bone 96, 29–37.
Deshet-Unger, N., Hiram-Bab, S., Haim-Ohana, Y., Mittelman, M., Gabet, Y., and Neumann, D. (2016). Erythropoietin treatment in murine multiple myeloma: immune gain and bone loss. Sci. Rep. 6:30998. doi: 10.1038/srep30998
Dewan, M. Z., Watanabe, M., Terashima, K., Aoki, M., Sata, T., Honda, M., et al. (2004). Prompt tumor formation and maintenance of constitutive NF-kappaB activity of multiple myeloma cells in NOD/SCID/gammacnull mice. Cancer Sci. 95, 564–568. doi: 10.1111/j.1349-7006.2004.tb02487.x
DeWeerdt, S. (2011). Animal models: Towards a myeloma mouse. Nature 480, S38–S39. doi: 10.1038/480S38a
Donovan, K., Lacy, M., Gertz, M., and Lust, J. (2002). IL-1β expression in IgM monoclonal gammopathy and its relationship to multiple myeloma. Leukemia 16, 382–385.
D’Souza, S., del Prete, D., Jin, S., Sun, Q., Huston, A. J., Kostov, F. E., et al. (2011). Gfi1 expressed in bone marrow stromal cells is a novel osteoblast suppressor in patients with multiple myeloma bone disease. Blood 118, 6871–6880. doi: 10.1182/blood-2011-04-346775
Eda, H., Santo, L., Wein, M. N., Hu, D. Z., Cirstea, D. D., Nemani, N., et al. (2016). Regulation of Sclerostin Expression in Multiple Myeloma by Dkk-1: A Potential Therapeutic Strategy for Myeloma Bone Disease. J. Bone Miner. Res. 31, 1225–1234. doi: 10.1002/jbmr.2789
Evans, H. R., Karmakharm, T., Lawson, M. A., Walker, R. E., Harris, W., Fellows, C., et al. (2016). Osteolytica: An automated image analysis software package that rapidly measures cancer-induced osteolytic lesions in in vivo models with greater reproducibility compared to other commonly used methods. Bone 83, 9–16. doi: 10.1016/j.bone.2015.10.004
Faict, S., Muller, J., De Veirman, K., De Bruyne, E., Maes, K., Vrancken, L., et al. (2018). Exosomes play a role in multiple myeloma bone disease and tumor development by targeting osteoclasts and osteoblasts. Blood Cancer J. 8:105. doi: 10.1038/s41408-018-0139-7
Fowler, J. A., Mundy, G. R., Lwin, S. T., Lynch, C. C., and Edwards, C. M. (2009). A murine model of myeloma that allows genetic manipulation of the host microenvironment. Dis. Model Mech. 2, 604–611. doi: 10.1242/dmm.003160
Fryer, R. A., Graham, T. J., Smith, E. M., Walker-Samuel, S., Morgan, G. J., Robinson, S. P., et al. (2013). Characterization of a novel mouse model of multiple myeloma and its use in preclinical therapeutic assessment. PLoS One 8:e57641. doi: 10.1371/journal.pone.0057641
Fuhler, G. M., Brooks, R., Toms, B., Iyer, S., Gengo, E. A., Park, M. Y., et al. (2012). Therapeutic potential of SH2 domain-containing inositol-5’-phosphatase 1 (SHIP1) and SHIP2 inhibition in cancer. Mol. Med. 18, 65–75. doi: 10.2119/molmed.2011.00178
Fulciniti, M., Tassone, P., Hideshima, T., Vallet, S., Nanjappa, P., Ettenberg, S. A., et al. (2009). Anti-DKK1 mAb (BHQ880) as a potential therapeutic agent for multiple myeloma. Blood 114, 371–379. doi: 10.1182/blood-2008-11-191577
Garrett, I. R., Dallas, S., Radl, J., and Mundy, G. R. (1997). A murine model of human myeloma bone disease. Bone 20, 515–520.
Green, A. C., Lath, D., Hudson, K., Walkley, B., Down, J. M., Owen, R., et al. (2019). TGFbeta Inhibition Stimulates Collagen Maturation to Enhance Bone Repair and Fracture Resistance in a Murine Myeloma Model. J. Bone Miner. Res. 34, 2311–2326. doi: 10.1002/jbmr.3859
Han, J. H., Choi, S. J., Kurihara, N., Koide, M., Oba, Y., and Roodman, G. D. (2001). Macrophage inflammatory protein-1alpha is an osteoclastogenic factor in myeloma that is independent of receptor activator of nuclear factor kappaB ligand. Blood 97, 3349–3353. doi: 10.1182/blood.v97.11.3349
Heath, D. J., Chantry, A. D., Buckle, C. H., Coulton, L., Shaughnessy, J. D. Jr., Evans, H. R., et al. (2009). Inhibiting Dickkopf-1 (Dkk1) removes suppression of bone formation and prevents the development of osteolytic bone disease in multiple myeloma. J. Bone Miner. Res. 24, 425–436. doi: 10.1359/jbmr.081104
Hideshima, T., Neri, P., Tassone, P., Yasui, H., Ishitsuka, K., Raje, N., et al. (2006). MLN120B, a novel IkappaB kinase beta inhibitor, blocks multiple myeloma cell growth in vitro and in vivo. Clin. Cancer Res. 12, 5887–5894. doi: 10.1158/1078-0432.CCR-05-2501
Hinge, M., Andersen, K. T., Lund, T., Jorgensen, H. B., Holdgaard, P. C., Ormstrup, T. E., et al. (2016). Bone healing in multiple myeloma: a prospective evaluation of the impact of first-line anti-myeloma treatment. Haematologica 101, 419–422e. doi: 10.3324/haematol.2016.144477
Hjertner, O., Torgersen, M. L., Seidel, C., Hjorth-Hansen, H., Waage, A., Borset, M., et al. (1999). Hepatocyte growth factor (HGF) induces interleukin-11 secretion from osteoblasts: a possible role for HGF in myeloma-associated osteolytic bone disease. Blood 94, 3883–3888.
Hurchla, M. A., Garcia-Gomez, A., Hornick, M. C., Ocio, E. M., Li, A., Blanco, J. F., et al. (2013). The epoxyketone-based proteasome inhibitors carfilzomib and orally bioavailable oprozomib have anti-resorptive and bone-anabolic activity in addition to anti-myeloma effects. Leukemia 27, 430–440. doi: 10.1038/leu.2012.183
Iwakoshi, N. N., Lee, A. H., Vallabhajosyula, P., Otipoby, K. L., Rajewsky, K., and Glimcher, L. H. (2003). Plasma cell differentiation and the unfolded protein response intersect at the transcription factor XBP-1. Nat. Immunol. 4, 321–329. doi: 10.1038/ni907
Katz, B. Z. (2010). Adhesive interactions: The multi-task biochemical toolbox of cancer cells. Semin. Cancer Biol. 20, 125–127. doi: 10.1016/j.semcancer.2010.08.001
Khong, T., Sharkey, J., and Spencer, A. (2008). The effect of azacitidine on interleukin-6 signaling and nuclear factor-kappaB activation and its in vitro and in vivo activity against multiple myeloma. Haematologica 93, 860–869. doi: 10.3324/haematol.12261
Kikuchi, J., Yamada, S., Koyama, D., Wada, T., Nobuyoshi, M., Izumi, T., et al. (2013). The novel orally active proteasome inhibitor K-7174 exerts anti-myeloma activity in vitro and in vivo by down-regulating the expression of class I histone deacetylases. J. Biol. Chem. 288, 25593–25602. doi: 10.1074/jbc.M113.480574
Kuehl, W. M. (2008). Modeling multiple myeloma by AID-dependent conditional activation of MYC. Cancer Cell 13, 85–87.
Kumar, S. K., Dispenzieri, A., Lacy, M. Q., Gertz, M. A., Buadi, F. K., Pandey, S., et al. (2014). Continued improvement in survival in multiple myeloma: changes in early mortality and outcomes in older patients. Leukemia 28, 1122–1128. doi: 10.1038/leu.2013.313
Kyle, R. A., and Rajkumar, S. V. (2004). Multiple myeloma. N. Engl. J. Med. 351, 1860–1873. doi: 10.1056/NEJMra041875
Kyle, R. A., Gertz, M. A., Witzig, T. E., Lust, J. A., Lacy, M. Q., Dispenzieri, A., et al. (2003). Review of 1027 patients with newly diagnosed multiple myeloma. Mayo Clin. Proc. 78, 21–33. doi: 10.4065/78.1.21
Landgren, O., Hofmann, J. N., McShane, C. M., Santo, L., Hultcrantz, M., Korde, N., et al. (2019). Association of Immune Marker Changes With Progression of Monoclonal Gammopathy of Undetermined Significance to Multiple Myeloma. JAMA Oncol. 5, 1293–1301. doi: 10.1001/jamaoncol.2019.1568
Lawson, M. A., McDonald, M. M., Kovacic, N., Hua Khoo, W., Terry, R. L., Down, J., et al. (2015a). Osteoclasts control reactivation of dormant myeloma cells by remodelling the endosteal niche. Nat. Commun. 6:8983. doi: 10.1038/ncomms9983
Lawson, M. A., Paton-Hough, J. M., Evans, H. R., Walker, R. E., Harris, W., Ratnabalan, D., et al. (2015b). NOD/SCID-GAMMA mice are an ideal strain to assess the efficacy of therapeutic agents used in the treatment of myeloma bone disease. PLoS One 10:e0119546. doi: 10.1371/journal.pone.0119546
Li, X., Pennisi, A., Zhan, F., Sawyer, J. R., Shaughnessy, J. D., and Yaccoby, S. (2007). Establishment and exploitation of hyperdiploid and non-hyperdiploid human myeloma cell lines. Br. J. Haematol. 138, 802–811. doi: 10.1111/j.1365-2141.2007.06742.x
Lwin, S. T., Edwards, C. M., and Silbermann, R. (2016). Preclinical animal models of multiple myeloma. BoneKEy Rep. 5:772.
Manning, L. S., Berger, J. D., O’Donoghue, H. L., Sheridan, G. N., Claringbold, P. G., and Turner, J. H. (1992). A model of multiple myeloma: culture of 5T33 murine myeloma cells and evaluation of tumorigenicity in the C57BL/KaLwRij mouse. Br. J. Cancer 66, 1088–1093.
Marino, S., and Roodman, G. D. (2018). Multiple Myeloma and Bone: The Fatal Interaction. Cold Spring Harb. Perspect. Med. 8:a031286. doi: 10.1101/cshperspect.a031286
Matthews, G. M., Lefebure, M., Doyle, M. A., Shortt, J., Ellul, J., Chesi, M., et al. (2013). Preclinical screening of histone deacetylase inhibitors combined with ABT-737, rhTRAIL/MD5-1 or 5-azacytidine using syngeneic Vk∗MYC multiple myeloma. Cell Death Dis. 4:e798. doi: 10.1038/cddis.2013.306
McCune, J. M., Namikawa, R., Kaneshima, H., Shultz, L. D., Lieberman, M., and Weissman, I. L. (1988). The SCID-hu mouse: murine model for the analysis of human hematolymphoid differentiation and function. Science 241, 1632–1639. doi: 10.1126/science.2971269
McDonald, M. M., Reagan, M. R., Youlten, S. E., Mohanty, S. T., Seckinger, A., Terry, R. L., et al. (2017). Inhibiting the osteocyte-specific protein sclerostin increases bone mass and fracture resistance in multiple myeloma. Blood 129, 3452–3464. doi: 10.1182/blood-2017-03-773341
Mirandola, L., Yu, Y., Chui, K., Jenkins, M. R., Cobos, E., John, C. M., et al. (2011). Galectin-3C inhibits tumor growth and increases the anticancer activity of bortezomib in a murine model of human multiple myeloma. PLoS One 6:e21811. doi: 10.1371/journal.pone.0021811
Mitsiades, C. S., Mitsiades, N. S., Bronson, R. T., Chauhan, D., Munshi, N., Treon, S. P., et al. (2003). Fluorescence imaging of multiple myeloma cells in a clinically relevant SCID/NOD in vivo model: biologic and clinical implications. Cancer Res. 63, 6689–6696.
Miyakawa, Y., Ohnishi, Y., Tomisawa, M., Monnai, M., Kohmura, K., Ueyama, Y., et al. (2004). Establishment of a new model of human multiple myeloma using NOD/SCID/gammac(null) (NOG) mice. Biochem. Biophys. Res. Commun. 313, 258–262. doi: 10.1016/j.bbrc.2003.11.120
Mohan, M., Kumar, M., Samant, R., Van Hemert, R. Jr., Tian, E., Desai, S., et al. (2021). Bone remineralization Of Lytic Lesions in Multiple Myeloma –The Arkansas Experience. Bone 2021:115876. doi: 10.1016/j.bone.2021.115876
Mohan, M., Samant, R. S., Yoon, D., Buros, A. F., Branca, A., Montgomery, C. O., et al. (2017). Extensive Remineralization of Large Pelvic Lytic Lesions Following Total Therapy Treatment in Patients With Multiple Myeloma. J. Bone Miner. Res. 32, 1261–1266. doi: 10.1002/jbmr.3111
Morgan, G. J., Walker, B. A., and Davies, F. E. (2012). The genetic architecture of multiple myeloma. Nat. Rev. Cancer 12, 335–348. doi: 10.1038/nrc3257
Mori, Y., Shimizu, N., Dallas, M., Niewolna, M., Story, B., Williams, P. J., et al. (2004). Anti-alpha4 integrin antibody suppresses the development of multiple myeloma and associated osteoclastic osteolysis. Blood 104, 2149–2154. doi: 10.1182/blood-2004-01-0236
Mundy, G. R., Raisz, L. G., Cooper, R. A., Schechter, G. P., and Salmon, S. E. (1974). Evidence for the secretion of an osteoclast stimulating factor in myeloma. N. Engl. J. Med. 291, 1041–1046.
National Cancer Institute: Surveillance, Epidemiology, and End Results Program (2020). Cancer Stat Facts: Myeloma. Maryland: National Cancer Institute.
Neri, P., Tassone, P., Shammas, M., Yasui, H., Schipani, E., Batchu, R. B., et al. (2007). Biological pathways and in vivo antitumor activity induced by Atiprimod in myeloma. Leukemia 21, 2519–2526. doi: 10.1038/sj.leu.2404912
Paton-Hough, J., Tazzyman, S., Evans, H., Lath, D., Down, J. M., Green, A. C., et al. (2019). Preventing and Repairing Myeloma Bone Disease by Combining Conventional Antiresorptive Treatment With a Bone Anabolic Agent in Murine Models. J. Bone Miner. Res. 34, 783–796. doi: 10.1002/jbmr.3606
Pilarski, L. M., Hipperson, G., Seeberger, K., Pruski, E., Coupland, R. W., and Belch, A. R. (2000). Myeloma progenitors in the blood of patients with aggressive or minimal disease: engraftment and self-renewal of primary human myeloma in the bone marrow of NOD SCID mice. Blood 95, 1056–1065.
Podar, K., Tai, Y. T., Hideshima, T., Vallet, S., Richardson, P. G., and Anderson, K. C. (2009). Emerging therapies for multiple myeloma. Expert Opin. Emerg. Drugs 14, 99–127. doi: 10.1517/14728210802676278
Postnov, A. A., Rozemuller, H., Verwey, V., Lokhorst, H., De Clerck, N., and Martens, A. C. (2009). Correlation of high-resolution X-ray micro-computed tomography with bioluminescence imaging of multiple myeloma growth in a xenograft mouse model. Calcif. Tissue Int. 85, 434–443. doi: 10.1007/s00223-009-9284-0
Raab, M. S., Podar, K., Breitkreutz, I., Richardson, P. G., and Anderson, K. C. (2009). Multiple myeloma. Lancet 374, 324–339. doi: 10.1016/S0140-6736(09)60221-X
Rabin, N., Kyriakou, C., Coulton, L., Gallagher, O. M., Buckle, C., Benjamin, R., et al. (2007). A new xenograft model of myeloma bone disease demonstrating the efficacy of human mesenchymal stem cells expressing osteoprotegerin by lentiviral gene transfer. Leukemia 21, 2181–2191. doi: 10.1038/sj.leu.2404814
Radl, J. (1981). Animal model of human disease. Benign monoclonal gammopathy (idiopathic paraproteinemia). Am. J. Pathol. 105, 91–93.
Radl, J., Croese, J. W., Zurcher, C., van den Enden-Vieveen, M. H., Brondijk, R. J., Kazil, M., et al. (1985). Influence of treatment with APD-bisphosphonate on the bone lesions in the mouse 5T2 multiple myeloma. Cancer 55, 1030–1040.
Radl, J., Croese, J. W., Zurcher, C., Van den Enden-Vieveen, M. H., and de Leeuw, A. M. (1988). Animal model of human disease. Multiple myeloma. Am. J. Pathol. 132, 593–597.
Radl, J., De Glopper, E. D., Schuit, H. R., and Zurcher, C. (1979). Idiopathic paraproteinemia. II. Transplantation of the paraprotein-producing clone from old to young C57BL/KaLwRij mice. J. Immunol. 122, 609–613.
Raje, N. S., Bhatta, S., and Terpos, E. (2019). Role of the RANK/RANKL pathway in multiple myeloma. Clin. Cancer Res. 25, 12–20.
Rajkumar, S. V., Dimopoulos, M. A., Palumbo, A., Blade, J., Merlini, G., Mateos, M. V., et al. (2014). International Myeloma Working Group updated criteria for the diagnosis of multiple myeloma. Lancet Oncol. 15, 538–548e. doi: 10.1016/S1470-2045(14)70442-5
Reagan, M. R., McDonald, M., Terry, R., Pettitt, J., Le, L., Mohanty, S., et al. (2015). Anti-Sclerostin Treatment Prevents Multiple Myeloma Induced Bone Loss and Reduces Tumor Burden. Blood 126, 119–119.
Reddy, S. V., Takahashi, S., Dallas, M., Williams, R. E., Neckers, L., and Roodman, G. D. (1994). Interleukin−6 antisense deoxyoligonucleotides inhibit bone resorption by giant cells from human giant cell tumors of bone. J. Bone Miner. Res. 9, 753–757.
Ria, R., Melaccio, A., Racanelli, V., and Vacca, A. (2020). Anti-VEGF drugs in the treatment of multiple myeloma patients. J. Clin. Med. 9:1765.
Ring, E. S., Lawson, M. A., Snowden, J. A., Jolley, I., and Chantry, A. D. (2018). New agents in the treatment of myeloma bone disease. Calcif. Tissue Int. 102, 196–209.
Robbiani, D. F., Colon, K., Affer, M., Chesi, M., and Bergsagel, P. L. (2005). Maintained rules of development in a mouse B-cell tumor. Leukemia 19, 1278–1280. doi: 10.1038/sj.leu.2403774
Roccaro, A. M., Sacco, A., Purschke, W. G., Moschetta, M., Buchner, K., Maasch, C., et al. (2014). SDF-1 inhibition targets the bone marrow niche for cancer therapy. Cell Rep. 9, 118–128. doi: 10.1016/j.celrep.2014.08.042
Saad, F., Lipton, A., Cook, R., Chen, Y. M., Smith, M., and Coleman, R. (2007). Pathologic fractures correlate with reduced survival in patients with malignant bone disease. Cancer 110, 1860–1867. doi: 10.1002/cncr.22991
Sati, H. I., Greaves, M., Apperley, J. F., Russell, R. G. G., and Croucher, P. I. (1999). Expression of interleukin−1β and tumour necrosis factor−α in plasma cells from patients with multiple myeloma. Br. J. Haematol. 104, 350–357.
Schwarz, E. M., and Ritchlin, C. T. (2007). Clinical development of anti-RANKL therapy. Arthritis Res. Ther. 9, (Suppl. 1), S7. doi: 10.1186/ar2171
Seidel, C., Lenhoff, S., Brabrand, S., Anderson, G., Standal, T., Lanng−Nielsen, J., et al. (2002). Hepatocyte growth factor in myeloma patients treated with high−dose chemotherapy. Br. J. Haematol. 119, 672–676.
Sezer, O., Heider, U., Zavrski, I., Kühne, C. A., and Hofbauer, L. C. (2003). RANK ligand and osteoprotegerin in myeloma bone disease. Blood 101, 2094–2098.
Shammas, M. A., Koley, H., Bertheau, R. C., Neri, P., Fulciniti, M., Tassone, P., et al. (2008). Telomerase inhibitor GRN163L inhibits myeloma cell growth in vitro and in vivo. Leukemia 22, 1410–1418. doi: 10.1038/leu.2008.81
Silbermann, R., and Roodman, G. D. (2013). Myeloma bone disease: Pathophysiology and management. J. Bone Oncol. 2, 59–69. doi: 10.1016/j.jbo.2013.04.001
Swami, A., Reagan, M. R., Basto, P., Mishima, Y., Kamaly, N., Glavey, S., et al. (2014). Engineered nanomedicine for myeloma and bone microenvironment targeting. Proc. Natl. Acad. Sci. U S A. 111, 10287–10292. doi: 10.1073/pnas.1401337111
Tanaka, Y., Abe, M., Hiasa, M., Oda, A., Amou, H., Nakano, A., et al. (2007). Myeloma cell-osteoclast interaction enhances angiogenesis together with bone resorption: a role for vascular endothelial cell growth factor and osteopontin. Clin. Cancer Res. 13, 816–823.
Tassone, P., Neri, P., Carrasco, D. R., Burger, R., Goldmacher, V. S., Fram, R., et al. (2005). A clinically relevant SCID-hu in vivo model of human multiple myeloma. Blood 106, 713–716. doi: 10.1182/blood-2005-01-0373
Tassone, P., Tagliaferri, P., Rossi, M., Gaspari, M., Terracciano, R., and Venuta, S. (2006). Genetics and molecular profiling of multiple myeloma: novel tools for clinical management? Eur. J. Cancer 42, 1530–1538. doi: 10.1016/j.ejca.2006.04.005
Terpos, E., Christoulas, D., Katodritou, E., Bratengeier, C., Gkotzamanidou, M., Michalis, E., et al. (2012). Elevated circulating sclerostin correlates with advanced disease features and abnormal bone remodeling in symptomatic myeloma: reduction post-bortezomib monotherapy. Int. J. Cancer 131, 1466–1471. doi: 10.1002/ijc.27342
Terpos, E., Politou, M., and Rahemtulla, A. (2003). New insights into the pathophysiology and management of bone disease in multiple myeloma. Br. J. Haematol. 123, 758–769. doi: 10.1046/j.1365-2141.2003.04712.x
Thirukkumaran, C. M., Shi, Z. Q., Luider, J., Kopciuk, K., Gao, H., Bahlis, N., et al. (2012). Reovirus as a viable therapeutic option for the treatment of multiple myeloma. Clin. Cancer Res. 18, 4962–4972. doi: 10.1158/1078-0432.CCR-11-3085
Thorsteinsdottir, S., Gislason, G., Aspelund, T., Sverrisdottir, I., Landgren, O., Turesson, I., et al. (2020). Fractures and survival in multiple myeloma: results from a population-based study. Haematologica 105, 1067–1073. doi: 10.3324/haematol.2019.230011
Tian, E., Zhan, F., Walker, R., Rasmussen, E., Ma, Y., Barlogie, B., et al. (2003). The role of the Wnt-signaling antagonist DKK1 in the development of osteolytic lesions in multiple myeloma. N. Engl. J. Med. 349, 2483–2494.
Udi, J., Schuler, J., Wider, D., Ihorst, G., Catusse, J., Waldschmidt, J., et al. (2013). Potent in vitro and in vivo activity of sorafenib in multiple myeloma: induction of cell death, CD138-downregulation and inhibition of migration through actin depolymerization. Br. J. Haematol. 161, 104–116. doi: 10.1111/bjh.12226
Urashima, M., Chen, B. P., Chen, S., Pinkus, G. S., Bronson, R. T., Dedera, D. A., et al. (1997). The development of a model for the homing of multiple myeloma cells to human bone marrow. Blood 90, 754–765.
Urashima, M., Ogata, A., Chauhan, D., Vidriales, M. B., Teoh, G., Hoshi, Y., et al. (1996). Interleukin-6 promotes multiple myeloma cell growth via phosphorylation of retinoblastoma protein. Blood 88, 2219–2227.
Vanderkerken, K., Asosingh, K., Braet, F., Van Riet, I., and Van Camp, B. (1999). Insulin-like growth factor-1 acts as a chemoattractant factor for 5T2 multiple myeloma cells. Blood 93, 235–241.
Vanderkerken, K., De Raeve, H., Goes, E., Van Meirvenne, S., Radl, J., Van Riet, I., et al. (1997). Organ involvement and phenotypic adhesion profile of 5T2 and 5T33 myeloma cells in the C57BL/KaLwRij mouse. Br. J. Cancer 76, 451–460.
Voskaridou, E., and Terpos, E. (2004). New insights into the pathophysiology and management of osteoporosis in patients with beta thalassaemia. Br. J. Haematol. 127, 127–139.
Walsh, M. C., and Choi, Y. (2014). Biology of the RANKL–RANK–OPG system in immunity, bone, and beyond. Front. Immunol. 5:511. doi: 10.3389/fimmu.2014.00511
Weiss, B. M., Abadie, J., Verma, P., Howard, R. S., and Kuehl, W. M. (2009). A monoclonal gammopathy precedes multiple myeloma in most patients. Blood 113, 5418–5422. doi: 10.1182/blood-2008-12-195008
Wu, K. D., Zhou, L., Burtrum, D., Ludwig, D. L., and Moore, M. A. (2007). Antibody targeting of the insulin-like growth factor I receptor enhances the anti-tumor response of multiple myeloma to chemotherapy through inhibition of tumor proliferation and angiogenesis. Cancer Immunol. Immunother. 56, 343–357. doi: 10.1007/s00262-006-0196-9
Xu, D., Hu, J., De Bruyne, E., Menu, E., Schots, R., Vanderkerken, K., et al. (2012). Dll1/Notch activation contributes to bortezomib resistance by upregulating CYP1A1 in multiple myeloma. Biochem. Biophys. Res. Commun. 428, 518–524. doi: 10.1016/j.bbrc.2012.10.071
Xu, L., Mohammad, K. S., Wu, H., Crean, C., Poteat, B., Cheng, Y., et al. (2016). Cell Adhesion Molecule CD166 Drives Malignant Progression and Osteolytic Disease in Multiple Myeloma. Cancer Res. 76, 6901–6910. doi: 10.1158/0008-5472.CAN-16-0517
Yaccoby, S., Barlogie, B., and Epstein, J. (1998). Primary myeloma cells growing in SCID-hu mice: a model for studying the biology and treatment of myeloma and its manifestations. Blood 92, 2908–2913.
Yaccoby, S., Pearse, R. N., Johnson, C. L., Barlogie, B., Choi, Y., and Epstein, J. (2002). Myeloma interacts with the bone marrow microenvironment to induce osteoclastogenesis and is dependent on osteoclast activity. Br. J. Haematol. 116, 278–290.
Yata, K., and Yaccoby, S. (2004). The SCID-rab model: a novel in vivo system for primary human myeloma demonstrating growth of CD138-expressing malignant cells. Leukemia 18, 1891–1897. doi: 10.1038/sj.leu.2403513
Keywords: mouse model, C57BL/KalwRij, 5TMM, MYC derived model, SCID-hu MODEL, SCID-rab MODEL, multiple myeloma bone disease, multiple myeloma xenograft model
Citation: Mehdi SH, Nafees S, Mehdi SJ, Morris CA, Mashouri L and Yoon D (2021) Animal Models of Multiple Myeloma Bone Disease. Front. Genet. 12:640954. doi: 10.3389/fgene.2021.640954
Received: 12 December 2020; Accepted: 22 March 2021;
Published: 07 June 2021.
Edited by:
Fabiana Csukasi, University of California, Los Angeles, United StatesReviewed by:
Nicola Giuliani, University of Parma, ItalyCopyright © 2021 Mehdi, Nafees, Mehdi, Morris, Mashouri and Yoon. This is an open-access article distributed under the terms of the Creative Commons Attribution License (CC BY). The use, distribution or reproduction in other forums is permitted, provided the original author(s) and the copyright owner(s) are credited and that the original publication in this journal is cited, in accordance with accepted academic practice. No use, distribution or reproduction is permitted which does not comply with these terms.
*Correspondence: Donghoon Yoon, RFlvb25AdWFtcy5lZHU=
Disclaimer: All claims expressed in this article are solely those of the authors and do not necessarily represent those of their affiliated organizations, or those of the publisher, the editors and the reviewers. Any product that may be evaluated in this article or claim that may be made by its manufacturer is not guaranteed or endorsed by the publisher.
Research integrity at Frontiers
Learn more about the work of our research integrity team to safeguard the quality of each article we publish.