- 1Radiation Research Division, Korea Atomic Energy Research Institute, Jeongeup, South Korea
- 2Department of Biological Sciences, College of Biological Sciences and Biotechnology, Chungnam National University, Daejeon, South Korea
- 3Department of Radiation Science and Technology, University of Science and Technology, Daejeon, South Korea
Deinococcus radiodurans is extremely resistant to ionizing radiation and has an exceptional ability to repair DNA damage caused by various DNA-damaging agents. D. radiodurans uses the same DNA-repair strategies as other prokaryotes, but certain proteins involved in the classical DNA repair machinery have characteristics different from their counterparts. RecG helicase, which unwinds a variety of branched DNA molecules, such as Holliday junctions (HJ) and D-loops, plays important roles in DNA repair, recombination, and replication. Primary sequence analysis of RecG from a number of bacterial species revealed that three amino acids (QPW) in the DNA-binding wedge domain (WD) are well-conserved across the Deinococcus RecG proteins. Interactions involving these conserved residues and DNA substrates were predicted in modeled domain structures of D. radiodurans RecG (DrRecG). Compared to the WD of Escherichia coli RecG protein (EcRecG) containing FSA amino acids corresponding to QPW in DrRecG, the HJ binding activity of DrRecG-WD was higher than that of EcRecG-WD. Reciprocal substitution of FSA and QPW increased and decreased the HJ binding activity of the mutant WDs, EcRecG-WDQPW, and DrRecG-WDFSA, respectively. Following γ-irradiation treatment, the reduced survival rate of DrRecG mutants (ΔrecG) was fully restored by the expression of DrRecG, but not by that of EcRecG. EcRecGQPW also enhanced γ-radioresistance of ΔrecG, whereas DrRecGFSA did not. ΔrecG cells complemented in trans by DrRecG and EcRecGQPW reconstituted an intact genome within 3 h post-irradiation, as did the wild-type strain, but ΔrecG with EcRecG and DrRecGFSA exhibited a delay in assembly of chromosomal fragments induced by γ-irradiation. These results suggested that the QPW residues facilitate the association of DrRecG with DNA junctions, thereby enhancing the DNA repair efficiency of DrRecG.
Introduction
Deinococcus radiodurans is well known for its extreme resistance to lethal doses of ionizing radiation (IR) and many other DNA damaging agents, including mitomycin C (MMC), UV-C radiation, and desiccation (Slade and Radman, 2011). This remarkable resistance is thought to be attributed to its highly efficient DNA repair capacity and various anti-oxidative systems (Lim et al., 2019). In D. radiodurans, extensive IR-induced DNA double-strand breaks (DSBs), which are the most lethal form of DNA damage, can be mended within a few hours (Zahradka et al., 2006). The rapid reconstruction of an intact genome from hundreds of chromosomal fragments is achieved through extended synthesis-dependent strand annealing (ESDSA), followed by homologous recombination (HR; Zahradka et al., 2006).
ATP-dependent duplex DNA unwinding enzymes, termed DNA helicases, are prevalent in all kingdoms of life and play important roles in the processes of DNA replication, repair, recombination, etc. (Brosh and Matson, 2020). The human genome encodes for 31 nonredundant DNA helicases (Umate et al., 2011). Given their fundamental roles in DNA metabolism, mutations in some of these genes are associated with certain human diseases characterized by premature aging and cancer, including Xeroderma Pigmentosum, Cockayne Syndrome, and Werner Syndrome (Uchiumi et al., 2015). Since DNA helicase was first discovered in the model bacterium Escherichia coli (Brosh and Matson, 2020), the E. coli helicases have been intensively studied, and their function has been compared to that of counterparts identified in different organisms. In E. coli, HR initiation follows the RecBCD pathway. The RecBCD complex binds to double-stranded DNA (dsDNA) ends and unwinds and degrades the DNA by using a combination of helicase and nuclease activities, which promotes the repair of DSB (Rocha et al., 2005). D. radiodurans is devoid of RecB and RecC proteins but possesses a RecD homolog named RecD2 (Wang and Julin, 2004). Since RecD2 is present in recBC-minus organisms, it is not associated with RecBC (Montague et al., 2009). The D. radiodurans RecD2 protein is a DNA helicase with 5'-3' polarity and low processivity (Wang and Julin, 2004). recD2 mutants are more sensitive than wild type cells to the cytotoxic effect of γ-irradiation, UV light, and hydrogen peroxide (H2O2; Servinsky and Julin, 2007; Zhou et al., 2007), but recD2 mutations does not alter the sensitivity of D. radiodurans to treatment with mitomycin C (MMC), methyl methanesulfonate, and hydroxyurea (Servinsky and Julin, 2007; Montague et al., 2009). D. radiodurans lacks not only RecBC, but also exonuclease I (SbcB), hence DSBs in D. radiodurans are repaired by the RecFOR pathway, which is significantly more common than RecBCD in bacterial genomes (Rocha et al., 2005). RecFOR-dependent DSB repair is initiated by unwinding duplex DNA, followed by DNA end resection that degrades the broken ends in the 5'–3' direction to obtain 3' single-stranded DNA (ssDNA) tails (Rocha et al., 2005).
In E. coli, RecQ is the major helicase implicated in the RecFOR pathway and in nucleolytic degradation catalyzed by RecJ; however, in D. radiodurans, RecQ is dispensable (Bentchikou et al., 2010). Different results have been reported with respect to recQ mutant phenotypes: a recQ mutant strain was reported to be sensitive to γ-irradiation, UV, H2O2, and MMC (Huang et al., 2007), whereas another study showed that recQ mutant cells display wild-type resistance to γ-irradiation (Bentchikou et al., 2010). Recently, RecD2 and RecQ proteins from D. radiodurans were reported to be able to unwind guanine quadruplex (G4) DNA structures (Khairnar et al., 2019; Xue et al., 2020). Instead of RecQ, in D. radiodurans, the UvrD helicase, which can unwind duplex DNA in both the 3'–5' and 5'–3' directions (Stelter et al., 2013), plays a critical role in DSB repair and reconstitution of the genome following IR exposure (Bentchikou et al., 2010). However, the uvrD mutant still retained significant radio-resistance as compared to a repair-deficient recA mutant strain, suggesting that the redundant activity of other helicase(s) is responsible for the residual DNA repair capacity observed (Bentchikou et al., 2010).
The RecFOR complex loads RecA onto ssDNA substrates, thereby resulting in the formation of a RecA nucleoprotein filament that searches for homology and then invades the double-stranded homologous DNA (Bentchikou et al., 2010). RecA-mediated strand invasion creates a D-loop, and primes DNA polymerase III (Pol III)- and/or Pol I-dependent DNA synthesis (Slade et al., 2009). DNA synthesis proceeds via a migrating D-loop, in which the unwinding of the dsDNA template may be mediated by UvrD, RecD2, RecQ, RuvAB, and/or other helicases (Slade and Radman, 2011). The RuvABC system can displace and resolve a four-way DNA intermediate named the Holliday junction (HJ). The RuvAB and RuvC proteins catalyze branch migration and the resolution of HJ recombination intermediates, respectively (Rocha et al., 2005). The D. radiodurans ruvB mutant is modestly sensitive to UV light, γ-irradiation, and MMC (Kitayama et al., 1997). However, the fact that inactivation of both ruv and recG resulted in a more dramatic increase in the sensitivity of cells to DNA damaging-agents has led to the suggestion that RecG and RuvABC are part of two overlapping pathways for processing intermediates in HR and DNA repair (Lloyd and Rudolph, 2016). The deletion of recG resulted in a growth delay and a decrease in the resistance of D. radiodurans to γ-irradiation and H2O2 (Wu et al., 2009).
RecG, a monomeric dsDNA translocase that unwinds a variety of branched DNA molecules, such as replication forks, HJs, D-, and R-loops (Lloyd and Rudolph, 2016), consists of an N-terminal wedge domain (WD), two RecA-like helicase domains, and a C-terminal translocation in RecG (TRG) motif (Fairman-Williams et al., 2010). The WD, which is not found in other DNA helicases, provides specificity for binding branched DNA structures (Rudolph et al., 2010). Amino acid sequence analysis of WDs of RecGs from Deinococcus species revealed that a “Gln(Q)-Pro(P)-Trp(W)” residue motif is highly conserved in Deinococus RecG proteins. In this study, we found that QPW residues contributed to strong binding of RecG to HJ and, consequently, enhanced the ability of RecG to repair DNA damage in D. radiodurans.
Materials and Methods
Bacterial Strains and Culture Conditions
Deinococus radiodurans R1 (ATCC13939) and its isogenic recG mutant strains (ΔrecG), which had been previously constructed (Jeong et al., 2016), were cultivated at 30°C in TGY broth (0.5% tryptone, 0.1% glucose, and 0.3% yeast extract) with aeration or on TGY plates supplemented with 1.5% Bacto-agar. The E. coli strain DH5α was used for routine cloning experiments. Escherichia coli strains were grown at 37°C in Luria-Bertani (LB) medium or on LB plates solidified with 1.5% Bacto-agar. Antibiotics were added to the medium if necessary: ampicillin, 100 μg/ml (E. coli), and chloramphenicol, 3 μg/ml (D. radiodurans).
Plasmid Construction
The pRADZ3 shuttle vector, which functions in E. coli as well as in D. radiodurans, contains the groEL promoter for constitutive gene expression (Jeong et al., 2016). Complete recG coding sequences were PCR-amplified from genomic DNA of D. radiodurans R1 and E. coli MG1655 by using DR1916F/R and B3652F/R primer pairs, respectively, carrying the SpeI and BamHI restriction sites. The PCR products were cloned into pRADZ3 at the SpeI and BamHI sites to generate the plasmids pDrRecG and pEcRecG, respectively. These plasmids were transformed into ΔrecG for complementation studies. For transformation, D. radiodurans cells from exponentially growing cultures were collected by centrifugation and concentrated 50-fold in TGY supplemented with 30 mM CaCl2. The cell mixture (100 μl) containing the constructed plasmid DNAs was held on ice for 30 min and then incubated at 32°C for 90 min. The transformation mixture was diluted 10-fold with TGY broth and incubated at 30°C for 5 h with aeration, prior to being plated on drug-selective agar. The partial nucleotide sequence of recG encoding DrRecG lacking the N-terminal region (residues 1–99) was PCR-amplified using DR1916-ΔNF/-ΔNR primer pairs and cloned into pRADZ3 to generate pDrRecGΔN99 as described above. To produce RecG mutant proteins, EcRecG with QPW instead of 99FSA101 and DrRecG with FSA instead of 201QPW203, mutagenesis was carried out using fusion PCR techniques. Complementary primer pairs, EcQPW-F/-R and DrFSA-F/-R, were designed to contain the desired mutations in the middle of the primers. To introduce the triple point mutation “QPW” into EcRecG, in the first step, two fragments were amplified using the primer pairs B3652F/EcQPW-R and EcQPW-F/B3652R, respectively. In the second step, the two PCR products were annealed at their overlapping homologous regions and were amplified by the 3652F/R primer pair. The fusion PCR product was cloned into pRADZ3 to generate pEcRecGQPW as described above. The plasmid pDrRecGFSA was constructed using primer pairs DR1916F/DrFSA-R and DrFSA-F/DR1916R. The plasmid constructs were transformed into ΔrecG. The plasmids were verified by DNA sequencing. Primers used in this study are listed in Supplementary Table S1.
Construction and Purification of RecG Wedge Domain
A maltose-binding protein (MBP)-RecG-WD fusion was constructed using the pMAL-c2x vector, in which the E. coli malE gene encoding MBP was expressed via an IPTG-inducible promoter. The WD regions of recG genes were amplified using the DrWD-F/-R and EcWD-F/-R primer sets (Supplementary Table S1) by using D. radiodurans and E. coli genomic DNA as template, respectively. The PCR products were digested with EcoRI and HindIII and cloned into pMAL-c2x for purification of the wild-type WDs, named DrRecG-WDWT and EcRrecG-WDWT, respectively. To generate mutant RecG-WDs, DrRecG-WDFSA, and EcRecG-WDQPW, the WD regions were amplified from plasmids pDrRecGFSA and pEcRecGQPw, respectively, and then cloned into pMAL-c2x as described above. The resulting plasmids were transformed into E. coli strain BL21 (DE3) for purification of the RecG-WDs fused to MBP. Cells were grown in 500 ml of LB broth at 37°C. IPTG (0.1 mM) was added to the culture when the cells reached an optical density at 600 nm (OD600) of 0.5 and were further grown at 16°C overnight. The cells were harvested by centrifugation and resuspended in buffer A (20 mM Tris-HCl, pH 7.5; 0.2 M NaCl; and 1 mM EDTA, pH 8.0). Following sonication on ice, cell debris was removed by centrifugation, and the supernatant was loaded onto a 5 ml amylose column. The column was washed with five column volumes of buffer A. The MBP-RecG-WD fusion protein was eluted with 5 ml of buffer B (buffer A containing 10 mM maltose). Following 10% SDS PAGE, fractions containing the approximately 51-kDa fusion protein were collected and concentrated by ultrafiltration using Amicon® Ultra Filters (Merck Millipore, Darmstadt, Germany). Protein concentrations were determined using the Bradford protein assay with bovine serum albumin as the standard.
DNA Binding Assay
To create the HJ DNA substrate, four complementary oligonucleotides (oligo 1, 5'-GTCGGATCCTCTAGACAGCTCCATGATCACTGGCACTGGTAGAATTCGGC-3'; oligo 2, 5'-CAACGTCATAGACGATT ACATTGCTACATGGAGCTGTC TAGAGGATCCGA-3'; oligo 3, 5'-TGCCGAATTCTACCAGT GCCAGTGATGGACATCTTTGCCACGTTGACCC-3'; oligo 4, 5'-TGGGTCAACGTGGGCAAGATGTCCTAGCAATGTAATCGTCTATGACGT-3') were synthesized as described previously (McGlynn et al., 2000). The oligonucleotides were denatured for 15 min at 95°C and allowed to anneal at 25°C for 45 min. To examine the DNA binding activity of RecG-WD, an electrophoretic mobility shift assay kit (Invitrogen, Carlsbad, CA, United States) was used. Purified recombinant RecG-WD proteins and 100 nM of HJ DNA substrates were mixed in binding buffer containing 50 mM Tris-HCl (pH 8.0), 5 mM EDTA, 1 mM dithiothreitol, 100 μg/ml bovine serum albumin, and 6% glycerol (v/v) and incubated on ice for 15 min as described previously (McGlynn et al., 1997; Briggs et al., 2005). The DNA binding reaction was terminated by the addition of 2 μl of 2× loading dye. Samples were loaded onto 6% polyacrylamide gels in low-ionic strength buffer at 120 V for 35 min at room temperature, and the electrophoresed DNA-protein complexes were visualized using a ChemiDoc™ Touch imaging system (Bio-Rad, Hercules, CA, United States). Reaction products were quantified using Image Lab Software (Bio-Rad).
Growth and Survival Assays
A stationary-phase culture that had grown overnight was used as a seed culture. The seed culture was inoculated into 25 ml of TGY broth at 1:100 dilution, and cell growth was monitored at OD600 by using a spectrophotometer. For survival studies, cells grown to log phase (OD600 ≈ 1.0, corresponding to ~108 CFU/ml) in TGY broth were adjusted to OD600 ≈ 0.1 with TGY. After γ-irradiation treatment, cells were serially diluted 10-fold in saline (0.85% NaCl), spotted onto TGY plates, and incubated at 30°C for 3 days to allow colony formation.
Quantitative Real-Time PCR Assay
A 5 ml culture grown to mid-log phase was harvested by centrifugation. RNA preparation and cDNA synthesis were performed as described previously (Jeong et al., 2016). The quantitative real-time PCR (qRT-PCR) was performed on a CFX Connect real-time PCR system (Bio-Rad) using SYBR Premix Ex Taq (Takara Bio Inc., Otsu, Japan). PCR reactions were performed as follows one cycle of 95°C for 5 min and then 40 cycles of 95°C for 10 s and 60°C for 15 s. The housekeeping gene dr1343 encoding glyceraldehyde-3-phosphate dehydrogenase was used as an internal control. Primers used in qRT-PCR are denoted with the prefix “RT-” in Supplementary Table S1.
Pulsed Field Gel Electrophoresis
Irradiated (6 kGy) and unirradiated cells were diluted in 50 ml TGY broth to an OD600 of 0.25, and incubated at 30°C. At the indicated times, 1.5, 3, 4.5, 6, and 8 h after re-inoculation, cells (~ 5 × 108 CFU/ml) were harvested and washed with 10 mM phosphate buffer (pH 7.0). The cells were resuspended in 0.125 M EDTA (pH 8.0) and mixed with low-melt agarose (Bio-Rad) to obtain a final concentration of 0.8% agarose. The DNA plugs were incubated overnight at 37°C in 0.05 M EDTA (pH 7.5) containing 1 mg/ml lysozyme, placed in proteinase K solution at 50°C for 16 h, and washed once with 1 × washing buffer containing 1 mM phenylmethylsulfonyl fluoride (PMSF) and then three times with 1× washing buffer. The prepared DNA plugs were digested with 30 U of NotI restriction enzyme overnight at 37°C and then subjected to pulsed field gel electrophoresis (PFGE). DNA fragments were separated on 1% (wt/vol) agarose gels in 0.5 × TBE. Electrophoresis was performed using a CHEF-Mapper apparatus (Bio-Rad) under the following conditions: pulse ramping from 10 to 60 s, angle of 120°, current of 6 V/cm, and 22 h run time at 12°C. Gels were stained with 0.5 × TBE containing 0.5 μg/ml ethidium bromide for 30 min, destained for 10 min in deionized water, and then visualized using a ChemiDoc™ Touch imaging system (Bio-Rad).
Results
QPW Residues Are Highly Conserved in the WD of Deinococcal RecG Proteins
In general, RecG proteins largely consist of an N-terminal WD critical to DNA binding, helicase core domains, including seven motifs common to DNA helicases, and the signature RecG TRG motif (Fairman-Williams et al., 2010). Compared to the E. coli RecG protein (EcRecG), the D. radiodurans RecG protein (DrRecG) has an extended region with 99 amino acids at the N-terminus (Figure 1A). When the C-terminal regions containing the helicase domain and TRG motif are considered, sequence identity between the two proteins approaches 53%, whereas there is no significant similarity in the WD (Figure 1A; Supplementary Figure S1). The crystal structure of RecG from Thermatoga maritima (TmRecG) revealed that Phe204 and Tyr208 in its WD are important for the binding of branched DNA molecules (Singleton et al., 2001). Multiple sequence alignment of the WD showed that the phenylalanine residue was highly conserved, and the tyrosine residue was replaced with another aromatic amino acid, tryptophan, in all deinococcal RecG proteins (Figure 1B; Supplementary Figure S2). Interestingly, glutamine (Q), proline (P), and tryptophan (W) at positions 201, 202, and 203, respectively (D. radiodurans numbering) were found in all deinococcal RecGs (Supplementary Figure S2).
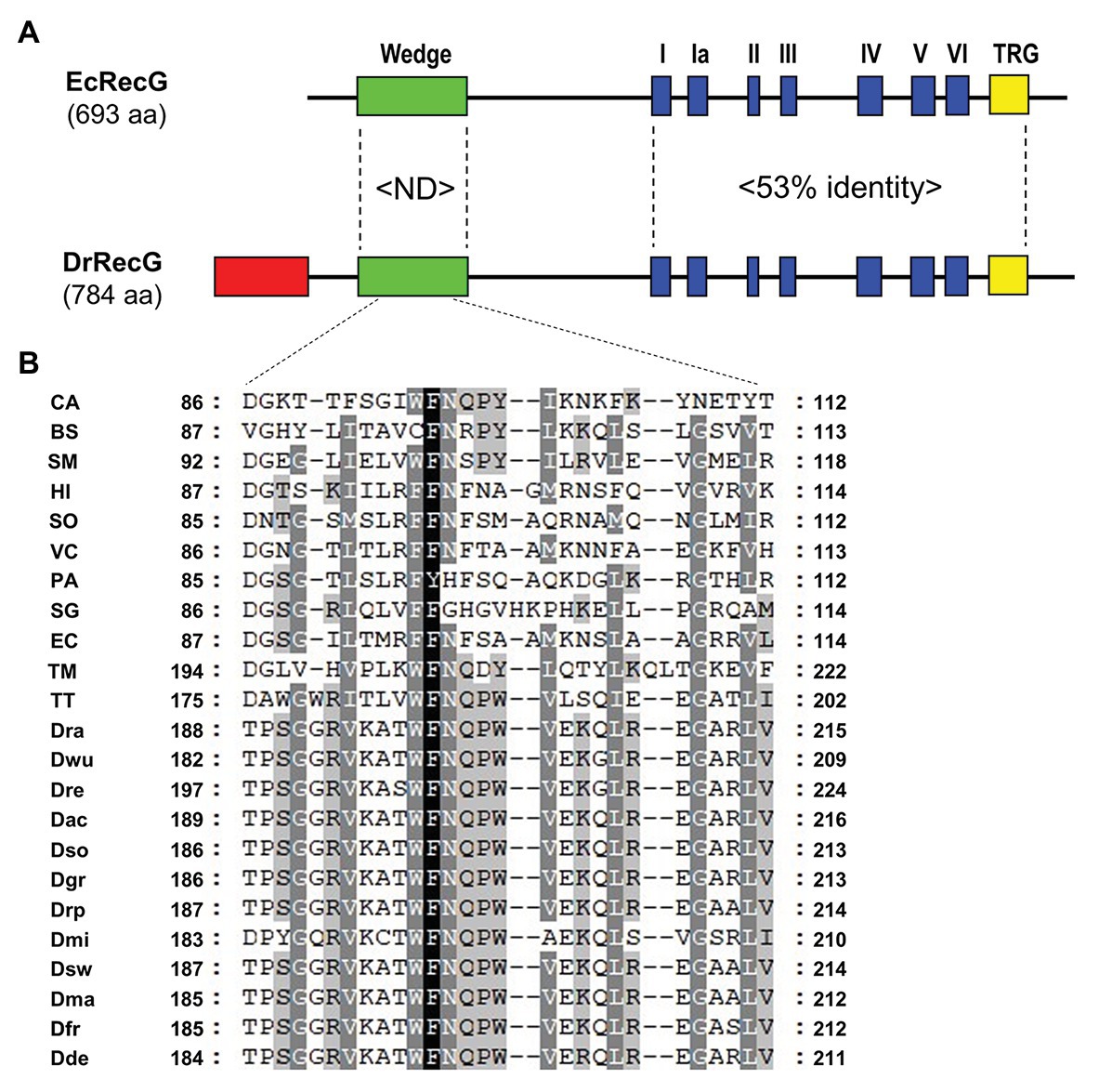
Figure 1. Alignment of Escherichia coli RecG (EcRecG) and Deinococcus radiodurans RecG (DrRecG). (A) Schematic comparison of the domain structures of EcRecG and DrRecG. EcRecG and DrRecG are aligned via wedge (green), helicase (blue), and TRG (yellow) motifs. The N-terminal extended region of DrRecG is shown as a red box. The percentages of conservation of amino acid sequences between EcRecG and DrRecG are indicated for wedge domain (ND, not determined) and helicase and TRG motifs (53% identity). (B) Multiple alignment of amino acid sequences containing the conserved phenylalanine (F) residue within the wedge domain. The program Genedoc (www.psc.edu/biomed/genedoc) was used to visualize the alignment in quantify mode, which highlights residues most-frequently found in each column of the alignment. Gaps introduced to maximize alignment are indicated by dash. Black and white letters on gray shading represent ≥60 and ≥80% identity, respectively. White letters on black shading represent 100% identity. Wedge domain sequences were obtained from RecGs of Clostridium acetobutylicum, CA; Bacillus subtilis, BS; Streptobacillus moniliformis, SM; Haemophilus influenza, HI; Shewanella oneidensis, SO; Vibrio cholera, VC; Pseudomonas aeruginosa, PA; Streptomyces griseus, SG; Escherichia coli, EC; Thermatoga maritima, TM; Thermus thermophilus, TT; D. radiodurans, Dra; D. wulumuqiensis, Dwu; D. reticulitermitis, Dre; D. actinosclerus, Dac; D. soli, Dso; D. grandis, Dgr; D. radiopugnans, Drp; D. maricopensis, Dmi; D. swuensis, Dsw; D. marmoris, Dma; D. frigensis, Dfr; and D. deserti, Dde.
Comparative Modeling of RecG Proteins
To gain insight into the forked-DNA binding mode of DrRecG, we generated structural models of DrRecG and EcRecG with SWISS-MODEL (Biasini et al., 2014) by using the crystal structure of TmRecG (PDB id: 1gm5) as a template. TmRecG (780 amino acids) shares 36.5 and 32.3% sequence identities with DrRecG (784 amino acids) and EcRecG (693 amino acids), respectively. We predicted structural models for DrRecG comprising of 735 amino acid residues (10–744) and EcRecG comprising of 635 amino acids (6–660), respectively. The modeled structures were validated by using QMEAN and ProSA (Supplementary Figure S3) and were superimposed onto the TmRecG-DNA complex structure. Our analyses suggested that Phe199 and Trp203 in DrRecG, which are likely to be equivalent to Phe204 and Tyr208, respectively, in TmRecG, may have roles in the stabilization of the leading and lagging strand templates by base stacking interactions between nucleotide bases and aromatic side chains, whereas Gln201 corresponding to Gln206 in TmRecG could interact with nucleotide bases by electrostatic interactions, thereby participating in the binding of DrRecG to DNA (Figure 2). In the case of EcRecG, only Phe97, corresponding to Phe204 in TmRecG, seemed to directly interact with a DNA base (Figure 2). Phe96 in EcRecG is also required for interaction with branched DNA molecules because it may have a stabilizing effect similar to that of Trp203 in TmRecG, which is partially buried in WD hydrophobic core and preserves WD conformation as a whole (Briggs et al., 2005). Phe96 in EcRecG, Trp203 in TmRecG, and Trp198 in DrRecG, which is equivalent to Trp203 in TmRecG, are located in the same position (Figure 2). Taken together, our homology models obtained for DrRecG and EcRecG fit well with the crystal structure of TmRecG. It can thus be assumed that QPW residues may play important roles in the DNA binding of deinococcal RecGs.
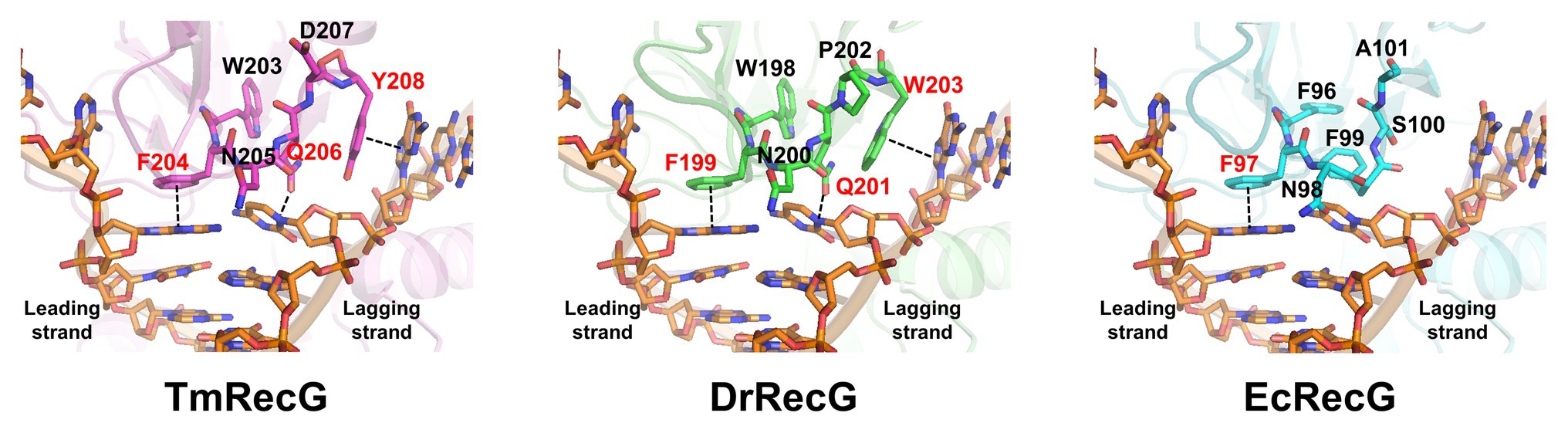
Figure 2. Structural model of RecG wedge domains in complex with a partial replication fork. The modeled wedge domains of RecG from Thermatoga maritima (TmRecG), DrRecG, and EcRecG are shown in purple, green, and cyan, respectively. DNA molecules are displayed in an orange stick model. Nitrogen, oxygen, and phosphorous atoms are colored in blue, red, and orange, respectively.
QPW Residues Enhance RecG DNA Binding Activity
The isolated WD can bind to HJ structures, although its affinity is lower than that of full-length RecG (Briggs et al., 2005). To test whether the QPW residues could affect the DNA binding activity of RecG, we cloned partial recG gene fragments encoding the WD into the expression vector pMAL-c2x to produce an MBP-RecG-WD fusion protein. The constructs encompassed residues 59–135 of EcRecG, and 158–235 of DrRecG (Supplementary Figure S1). We also constructed mutant versions of RecG-WD by replacing the QPW of DrRecG with FSA of EcRecG corresponding to QPW in DrRecG (Figure 1B), and vice versa. DNA binding assays involving the four different kinds of WDs, EcRecG-WDWT, EcRecG-WDQPW, DrRecG-WDWT, and DrRecG-WDFSA, were performed using HJ structures formed by annealing four complementary oligonucleotides, as previously described (McGlynn et al., 2000). As shown in Figure 3, DrRecG-WDWT almost reached a saturation of the total DNA binding at 160 nM protein, whereas EcRecG-WDWT did not even at higher concentrations (to 640 nM). The substitution of QPW with FSA (DrRecG-WDFSA) dramatically reduced binding ability, but EcRecG-WDQPW exhibited a significant increase compared to EcRecG-WDWT (Figure 3). These results clearly indicated that the QPW signature motif in deinococcal RecGs plays a role in enhancing the DNA-binding affinity of RecG.
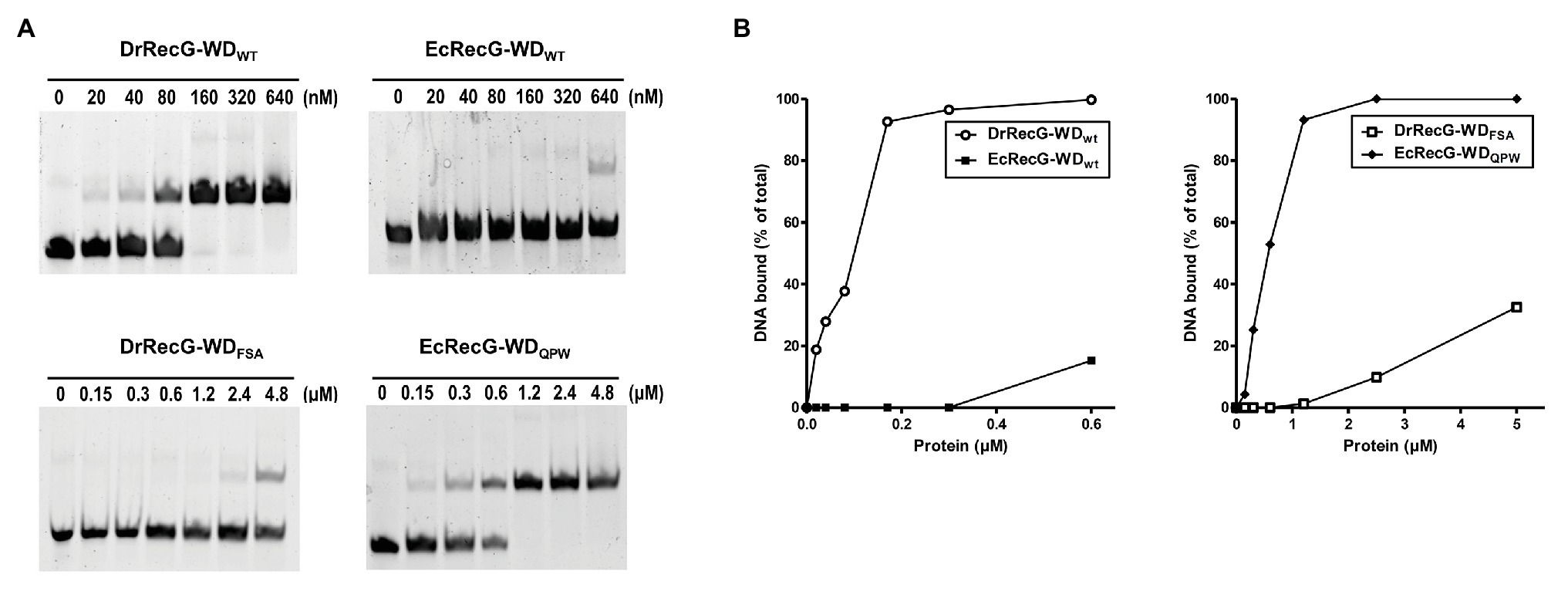
Figure 3. DNA binding activity of RecG wedge domains (RecG-WDs). (A) Binding affinities of RecG-WDs as measured in band shift assays with Holiday junction (HJ) substrate. Native RecG-WDs, DrRecG-WDWT and EcRecG-WDWT from D. radiodurans and E. coli, respectively, and their mutants, DrRecG-WDFSA and EcRecG-WDQPW were used in this assay. Reactions contained 100 nM HJ DNA and native RecG-WDs or mutant RecG-WDs at as shown. (B) Quantification of the binding activity of RecG-WDs. The formation of RecG-WD complexes with HJ in (A) was quantified and plotted as a function of increasing concentration of RecG-WDs.
N-Terminal Extended Region Deletion Does Not Affect DrRecG Activity
Deinococus radiodurans RecG has an extended region, with 99 amino acids in the N-terminus compared to EcRecG (Figure 1A). We constructed plasmid pDrRecGΔN99 expressing the N-terminal deletion mutant (residues 100–784) of DrRecG, with Ala100 converted to methionine, and transformed it into a DrRecG mutant strain (ΔrecG). Disruption of recG is known to result in D. radiodurans cell growth defects (Wu et al., 2009). Thus, we first monitored the growth of the wild-type D. radiodurans strain (WT) and ΔrecG by measuring their OD600 values over time. ΔrecG exhibited delayed growth relative to WT, and expression of the native DrRecG protein (pDrRecG) was able to complement the growth defects. Interestingly, the growth of ΔrecG harboring pDrRecGΔN99 was comparable to that of ΔrecG harboring pDrRecG (Figure 4A). In addition, the resistance of ΔrecG to γ-irradiation was fully restored to the levels of WT by DrRecGΔN99 provided in trans (data not shown). These observations indicate that the N-terminal extended region is not involved in the ΔrecG phenotypes, growth defect, and increased γ-irradiation sensitivity, under the experimental conditions tested here.
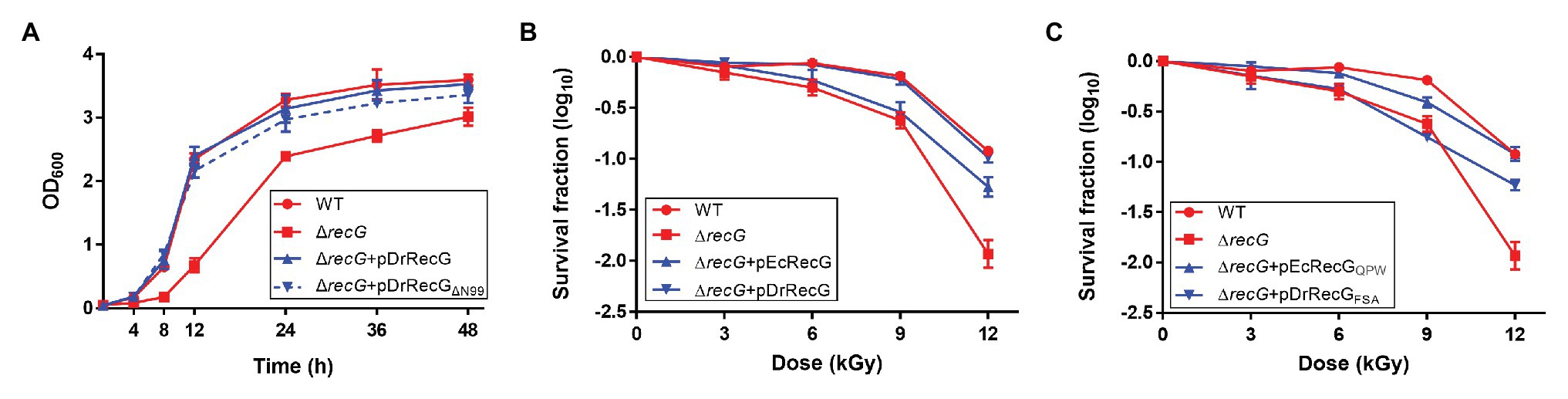
Figure 4. Growth and survival assays of the D. radiodurans recG mutant strain (ΔrecG). (A) Growth curves of D. radiodurans strains. Optical density (OD600) measurements were employed to estimate the growth of D. radiodurans R1 (WT), ΔrecG, and ΔrecG harboring the plasmids pDrRecG and pDrRecGΔN99, which encode the full-length DrRecG and the N-terminal truncation mutant of DrRecG, respectively. Survival curves for ΔrecG with pEcRecG and pDrRecG (B) and with pEcRecGQPW and pDrRecGFSA (C). Cells grown to log phase were exposed to γ-irradiation and spotted onto TGY plates. The survival fraction was calculated by dividing the colony-forming units (CFUs) of γ-irradiation-treated cells by the CFUs of unirradiated cells. The error bars represent the SD of three independent experiments conducted in duplicate (n = 3).
The QPW Motif Increases RecG DNA Repair Capacity
Because the N-terminal extended region was not essential for DrRecG activity (Figure 4A), and EcRecG displays a high degree of sequence conservation with DrRecG in the helicase and TRG motifs (Supplementary Figure S1), we introduced the pEcRecG plasmid carrying the E. coli recG gene into ΔrecG cells and measured cell survival rates following γ-irradiation to determine if EcRecG can functionally replace DrRecG. Compared to WT, ΔrecG showed approximately 0.25-, 0.5-, and 1-log reductions in survival at 6, 9, and 12 kGy of γ-irradiation, respectively, and these reductions were completely restored by DrRecG. However, EcRecG was able to partially restore the γ-irradiation-sensitive phenotype of ΔrecG only at 12 kGy of γ-irradiation (Figure 4B). To test the functional role of the QPW residues, we constructed pEcRecGQPW encoding an EcRecG mutant in which 99FSA101 was substituted with QPW, and pDrRecGFSA encoding a DrRecG mutant in which 201QPW203 was substituted with FSA. The resulting plasmids were transformed into ΔrecG cells, which were then exposed to γ-irradiation. There was no significant difference in recG mRNA levels between ΔrecG harboring pDrRecG and pDrRecGFSA (Supplementary Figure S4), but resistance to γ-irradiation was not recovered by DrRecGFSA (Figure 4C). In contrast, EcRecGQPW restored the ΔrecG survival in a similar way as DrRecG, although the restoration levels were somewhat different at 9 kGy (Figures 4B,C). These results showed that substituting the three amino acids in the WD between EcRecG and DrRecG is sufficient to exchange their abilities to complement the loss-of-function phenotype of ΔrecG after γ-irradiation.
To investigate the role of DrRecG in DSB, the DNA repair kinetics of cells subjected to γ-irradiation were examined using PFGE. DNA fragmented by γ-irradiation was reconstructed within 3 h in WT, whereas ΔrecG was able to compete the repair of shattered genomes 4.5 h after γ-irradiation (Figure 5). ΔrecG harboring pDrRecG and pEcRecG showed repair kinetics similar to those observed in WT and ΔrecG, respectively. Compared to ΔrecG with pEcRecGQPW, the process of genome reassembly was delayed by 1.5 h in ΔrecG with pDrRecGFSA (Figure 5). The similar patterns of results shown in survival assays and PFGE analysis, which are caused by the reciprocal swapping of the three amino acid motifs between DrRecG and EcRecG, strongly suggest that the QPW residues enhance DNA-binding activity, thereby leading to efficient DNA repair in D. radiodurans.
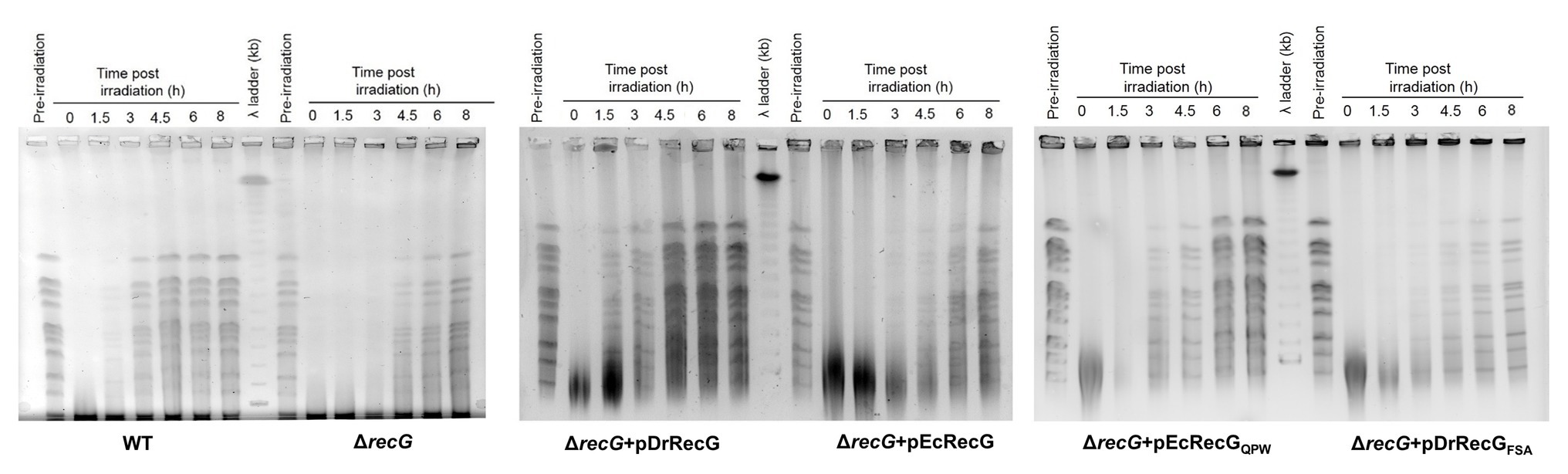
Figure 5. DNA repair in ΔrecG and ΔrecG cells harboring plasmids encoding the native (pDrRecG and pEcRecG) and mutant (pDrRecGFSA and pEcRecGQPW) RecG proteins. Exponentially growing cells were exposed to 6 kGy of γ-irradiation and then recovered in TGY liquid media. At the indicated times (0–8 h), samples were removed, and genomic DNA was isolated. The amount of DNA double-strand breaks (DSBs) was analyzed by pulsed-field gel electrophoresis (PFGE). Pre-irradiation indicates unirradiated cells.
Discussion
Analysis of the genome sequence of D. radiodurans identifies the typical complement of prokaryotic DNA repair proteins, suggesting the possibility that D. radiodurans uses the same DNA-repair strategies as other prokaryotes, but it does so in a manner that is somehow much more effective than that observed in other species to retain the extraordinary tolerance to DNA damage (Battista et al., 1999; White et al., 1999). A series of biochemical and structural analyses of D. radiodurans proteins involved in DNA repair systems have revealed unusual features distinguishing them from their counterparts in other prokaryotic species. Uracil-DNA glycosylase (UNG) removes uracil from DNA molecules, formed as a result of cytosine deamination, and represents part of the base-excision repair pathway. The uracil-DNA N-glycosylase DrUNG (DR_0689), the main UNG in D. radiodurans, possesses a much higher catalytic efficiency than human UNG, which is attributed to the high substrate affinity caused by an increased number of positively charged residues close to the DNA binding site (Pedersen et al., 2015). Mismatch-specific UNG (MUG) also removes uracil from DNA. The overall structure of DrMUG is similar to that of EcMUG, but DrMUG possesses a novel catalytic residue (Asp-93) that can provide DrMUG with broad substrate specificity (Moe et al., 2006). The novel catalytic residue identified in DrMUG is conserved in other deinococcal MUG proteins (Lim et al., 2019). The D. radiodurans X family DNA polymerase (DrPolX), which not only has polymerase activity, but also exerts strong Mn2+-dependent 3'→5' exonuclease activity affects DSB repair efficiency in D. radiodurans (Blasius et al., 2006). At the active site of the polymerase catalytic domain, the “DXD” motif conserved in almost all pol X-family members is replaced by an “AAE” motif in D. radiodurans (Leulliot et al., 2009; Bienstock et al., 2014). RecA is a critical enzyme in HR for DSB repair. Unlike canonical RecAs, DrRecA first forms a filament on dsDNA and then takes up a homologous single strand to initiate DNA strand exchange, which is the exact inverse of the major pathway seen with other proteins of the RecA family (Kim and Cox, 2002). Although the overall fold of DrRecA is similar to EcRecA, there are a few key amino acid changes in the DrRecA C-terminal domain that interacts with dsDNA. One of the key differences, Phe303 in DrRecA, equivalent to Trp290 in EcRecA, would seem to be a likely candidate in dictating a possible specificity for binding to dsDNA (Rajan and Bell, 2004). It has also been observed that a single amino acid mutation at the C-terminus of EcRecA alters its activity. The RecA variants found in radioresistant E. coli strain CB2000 have mutations at residue 276 (D276A and D276N), which increase the rates of filament nucleation on DNA, and promote DNA strand exchange more efficiently than wild-type RecA proteins (Piechura et al., 2015). These studies imply that DNA repair proteins with altered substrate specificity and enhanced catalytic activity, which might be attributed to unique amino acid residues, likely contribute to the improved DNA repair capacity of D. radiodurans to survive DNA damage caused by γ-irradiation. In this aspect, the highly conserved QPW residues in the WD can be assumed to affect the binding activity of deinococcal RecGs.
The WD found in the N-terminal region of RecG is a DNA-binding domain that has an oligonucleotide/oligosaccharide binding-fold (OB-fold) motif ranging between 70 and 150 amino acids in length (Theobald et al., 2003). The phenylalanine residue, which is critical for RecG binding of branched DNA molecules, is conserved in the WDs (Briggs et al., 2005; Figure 1B). This residue is regarded to stabilize the orphan base of the leading strand template by base stacking, effectively capping the parental duplex (Singleton et al., 2001; Figure 2). In Mycobacterium tuberculosis RecG, substitution of Phe99 corresponding to Phe204 in TmRecG to Ala was seen to significantly lower the DNA binding activity compared to that of wild-type RecG protein (Zegeye et al., 2014). In this study, protein structure modeling analysis showed that two other amino acids, Gln206 and Tyr208 in TmRecG and Gln201 and Trp203 in DrRecG, interacted with the nucleotide bases of the leading and lagging forked-DNA strands, together with the key residues, Phe204 in TmRecG, and Phe199 in DrRecG, respectively (Figure 2). The aromatic side-chain of Tyr participates in DNA binding of the WD through hydrophobic interactions such as base stacking (Singleton et al., 2001). Tyr was not observed in DrRecG, but it was substituted with an equivalent aromatic residue, Trp (Figure 2), suggesting that Trp203 may play a similar role in the stabilization of the lagging DNA strand duplex. Of the 79 deinococcal RecGs analyzed in this study, only two species Deinococcus ruber and Deinococcus aquiradiocola, have QAW instead of the signature QPW residues (Supplementary Figure S2). However, it is noteworthy that Q and W are strictly conserved. EcRecG does not have a signature motif with an aromatic residue in the corresponding position (99FSA101 in EcRecG). Comparisons of the DrRecG and EcRecG models suggested that the conserved QPW residues in the WD confer greater RecG-DNA interaction compared to FSA because of the additional charge interactions and base packing through Gln and Trp, respectively (Figure 2; Supplementary Figure S5). Indeed, DrRecG-WDWT and EcRecG-WDQPW exhibited enhanced affinities for HJ compared to EcRecG-WDWT and DrRecG-WDFSA (Figure 3). Full-length DrRecG and EcRecGQPW restored resistance to γ-irradiation and DNA repair capacity of ΔrecG to that of WT (Figures 4, 5).
Also noteworthy is the N-terminal extended region of DrRecG (Figure 1A). This feature is shared with RecG proteins from thermophilic bacteria, Thermotoga maritima and Aquifex aeolicus, and a number of cyanobacteria, and is longer than in many other RecG proteins such as EcRecG (Wen et al., 2005). RecG from the extreme thermophile A. aeolicus unwinds DNA well at high temperature (60°C), whereas the N-terminal extension present in this protein is dispensable for activity and thermo-stability (Wen et al., 2005). In D. radiodurans, the N-/C-terminal extension (or variation) is found not only in RecG, but also in other helicases, including RecD2 and RecQ. DrRecD2 contains an additional N-terminal region of approximately 200 amino acids, which is longer than the corresponding feature in E. coli RecD protein (RecD1; Montague et al., 2009). In contrast to most other RecQ proteins composed of the helicase, RecQ-C-terminal (RQC), and helicase-and-RNaseD-like-C terminal (HRDC) domains, DrRecQ contains three tandem HRDC domains in the C-terminus (Killoran and Keck, 2006). Full-length DrRecD2 restored the H2O2-resistant phenotype of recD2 mutants, whereas the N-terminal truncation mutant of DrRecD2 could not, suggesting that the N-terminal domain is necessary for the role of DrRecD2 in antioxidant pathways (Zhou et al., 2007). The three tandem HRDC domains increase the efficiency of DNA unwinding and ATPase activities of DrRecQ (Huang et al., 2007), and could work together with other functional motifs to enhance DrRecQ interactions with DNA (Liu et al., 2013). Considering that ΔrecG cells are sensitive to γ-irradiation and H2O2 (Wu et al., 2009; Figure 4), and DrRecG is also involved in catalase gene katE1 regulation (Jeong et al., 2016), further research is needed to determine the role of the additional DrRecG N-terminal region, although it seems not to be implicated in DNA repair under the experimental conditions tested in this study.
RecG provides a more general defense against pathological DNA replication, e.g., the rescue of stalled or damaged replication forks, and is associated with a number of additional cellular processes (Rudolph et al., 2010; Azeroglu et al., 2016; Lloyd and Rudolph, 2016; Romero et al., 2020). Recently, it has become increasingly clear that RecG is an enzyme responsible for regression of stalled DNA replication forks that can be induced by various types of lesions, including single-strand breaks and DSB. RecG is directly loaded onto the forks by binding to ssDNA-binding protein (SSB) and reverses the stalled forks, leading to the formation of HJ-type structures. PriA helicase then targets the repaired fork to reload and assemble a DNA replication machinery (the replisome complex), enabling DNA replication to restart (Lloyd and Rudolph, 2016; Bianco and Lyubchenko, 2017). During HR for DSB repair, RecG is proposed to re-model branched intermediates of recombination to direct the correct binding of PriA and subsequent DNA synthesis (Azeroglu et al., 2016). These studies indicate that SSB-mediated RecG loading onto DNA plays an important role in facilitating stalled replication fork rescue. D. radiodurans SSB (DrSSB) is different from that of the prototype E. coli SSB (EcSSB), in that DrSSB (301 amino acids) contains two OB folds per monomer, and functions as homodimers, in contrast to the homotetrameric EcSSB (178 amino acids), with each monomer encoding a single OB fold (Bernstein et al., 2004). In E. coli, SSB-RecG interactions occur via the WD of RecG and the PXXP motifs within the SSB linker domain. In particular, the SSB binding site on RecG overlaps the residues of the binding site for the leading strand arm of the fork in RecG (Ding et al., 2020). Thus, it is likely that these different features do not enable DrSSB to deliver EcRecG to the fork with an efficiency equal to that of DrRecG, which may explain why EcRecG does not confer resistance to ΔrecG (Figure 4B). EcRecG also partially complements Helicobacter pylori recG mutants but not to the same extent as the H. pylori RecG protein, suggesting that the host context appears to be critical in defining the function of RecG (Kang et al., 2004). In this aspect, the QPW residues found in the WD of DrRecG might be interpreted as the result of evolutionary adaptation to cooperate with the unique DrSSB. D. radiodurans is most closely related to Thermus thermophilus, and the two genera Deinococcus and Thermus belong to a distinct bacterial clade called the Deinococcus-Thermus group (Omelchenko et al., 2005). Regarding RecG and SSB, they share common features. Thermus RecG proteins have either QPW or QTW (Supplementary Figure S6), and the dimeric SSBs like DrSSB are discovered in the Deinococcus-Thermus group (Zhang et al., 2014), supporting that RecG and SSB might be evolutionarily related. T. thermophilus is a thermophile, which is relatively sensitive to IR, whereas D. radiodurans is a mesophile, which is highly IR-resistant (Omelchenko et al., 2005). However, considering that the mechanisms employed by thermophiles to overcome DNA damage by high temperature may also be employed in repair of damage caused by IR (Ranawat and Rawat, 2017), the coevolution between RecG and SSB, which likely occurred in the Deinococcus-Thermus group, may contribute to the enhanced abilities of the Deinococcus and Thermus lineages to survive different kinds of environmental stresses. Further research is warranted to investigate the effects of QPW on RecG-SSB interactions.
Data Availability Statement
The original contributions presented in the study are included in the article/Supplementary Material, further inquiries can be directed to the corresponding author.
Author Contributions
S-WJ performed the experiments and wrote a first draft. M-KK performed the comparative protein structure modeling and revised the first draft. LZ and S-KY constructed the plasmids and performed the survival assays. M-KK, J-HJ, and H-ML guided the experiments and interpreted the results. SL conceived the study and was in charge of overall direction and planning. All authors reviewed and edited the manuscript, and approved the final version.
Funding
This research was supported by the Nuclear R&D program of the Ministry of Science and ICT (MSIT), South Korea.
Conflict of Interest
The authors declare that the research was conducted in the absence of any commercial or financial relationships that could be construed as a potential conflict of interest.
Supplementary Material
The Supplementary Material for this article can be found online at: https://www.frontiersin.org/articles/10.3389/fgene.2021.634615/full#supplementary-material
References
Azeroglu, B., Mawer, J. S., Cockram, C. A., White, M. A., Hasan, A. M., Filatenkova, M., et al. (2016). RecG directs DNA synthesis during double-strand break repair. PLoS Genet. 12:e1005799. doi: 10.1371/journal.pgen.1005799
Battista, J. R., Earl, A. M., and Park, M. J. (1999). Why is Deinococcus radiodurans so resistant to ionizing radiation? Trends Microbiol. 7, 362–365. doi: 10.1016/S0966-842X(99)01566-8
Bentchikou, E., Servant, P., Coste, G., and Sommer, S. (2010). A major role of the RecFOR pathway in DNA double-strand-break repair through ESDSA in Deinococcus radiodurans. PLoS Genet. 6:e1000774. doi: 10.1371/journal.pgen.1000774
Bernstein, D. A., Eggington, J. M., Killoran, M. P., Misic, A. M., Cox, M. M., and Keck, J. L. (2004). Crystal structure of the Deinococcus radiodurans single-stranded DNA-binding protein suggests a mechanism for coping with DNA damage. Proc. Natl. Acad. Sci. U. S. A. 101, 8575–8580. doi: 10.1073/pnas.0401331101
Bianco, P. R., and Lyubchenko, Y. L. (2017). SSB and the RecG DNA helicase: an intimate association to rescue a stalled replication fork. Protein Sci. 26, 638–649. doi: 10.1002/pro.3114
Biasini, M., Bienert, S., Waterhouse, A., Arnold, K., Studer, G., Schmidt, T., et al. (2014). SWISS-MODEL: modelling protein tertiary and quaternary structure using evolutionary information. Nucleic Acids Res. 42, W252–W258. doi: 10.1093/nar/gku340
Bienstock, R. J., Beard, W. A., and Wilson, S. H. (2014). Phylogenetic analysis and evolutionary origins of DNA polymerase X-family members. DNA Repair 22, 77–88. doi: 10.1016/j.dnarep.2014.07.003
Blasius, M., Shevelev, I., Jolivet, E., Sommer, S., and Hübscher, U. (2006). DNA polymerase X from Deinococcus radiodurans possesses a structure-modulated 3′→5′ exonuclease activity involved in radioresistance. Mol. Microbiol. 60, 165–176. doi: 10.1111/j.1365-2958.2006.05077.x
Briggs, G. S., Mahdi, A. A., Wen, Q., and Lloyd, R. G. (2005). DNA binding by the substrate specificity (wedge) domain of RecG helicase suggests a role in processivity. J. Biol. Chem. 280, 13921–13927. doi: 10.1074/jbc.M412054200
Brosh, R. M. Jr., and Matson, S. W. (2020). History of DNA helicases. Gene 11:255. doi: 10.3390/genes11030255
Ding, W., Tan, H. Y., Zhang, J. X., Wilczek, L. A., Hsieh, K. R., Mulkin, J. A., et al. (2020). The mechanism of single strand binding protein-RecG binding: implications for SSB interactome function. Protein Sci. 29, 1211–1227. doi: 10.1002/pro.3855
Fairman-Williams, M. E., Guenther, U. P., and Jankowsky, E. (2010). SF1 and SF2 helicases: family matters. Curr. Opin. Struct. Biol. 20, 313–324. doi: 10.1016/j.sbi.2010.03.011
Huang, L., Hua, X., Lu, H., Gao, G., Tian, B., Shen, B., et al. (2007). Three tandem HRDC domains have synergistic effect on the RecQ functions in Deinococcus radiodurans. DNA Repair 6, 167–176. doi: 10.1016/j.dnarep.2006.09.006
Jeong, S. W., Seo, H. S., Kim, M. K., Choi, J. I., Lim, H. M., and Lim, S. (2016). PprM is necessary for up-regulation of katE1, encoding the major catalase of Deinococcus radiodurans, under unstressed culture conditions. J. Microbiol. 54, 426–431. doi: 10.1007/s12275-016-6175-8
Kang, J., Tavakoli, D., Tschumi, A., Aras, R. A., and Blaser, M. J. (2004). Effect of host species on recG phenotypes in Helicobacter pylori and Escherichia coli. J. Bacteriol. 186, 7704–7713. doi: 10.1128/JB.186.22.7704-7713.2004
Khairnar, N. P., Maurya, G. K., Pandey, N., Das, A., and Misra, H. S. (2019). DrRecQ regulates guanine quadruplex DNA structure dynamics and its impact on radioresistance in Deinococcus radiodurans. Mol. Microbiol. 112, 854–865. doi: 10.1111/mmi.14321
Killoran, M. P., and Keck, J. L. (2006). Three HRDC domains differentially modulate Deinococcus radiodurans RecQ DNA helicase biochemical activity. J. Biol. Chem. 281, 12849–12857. doi: 10.1074/jbc.M600097200
Kim, J. I., and Cox, M. M. (2002). The RecA proteins of Deinococcus radiodurans and Escherichia coli promote DNA strand exchange via inverse pathways. Proc. Natl. Acad. Sci. U. S. A. 99, 7917–7921. doi: 10.1073/pnas.122218499
Kitayama, S., Kohoroku, M., Takagi, A., and Itoh, H. (1997). Mutation of D. radiodurans in a gene homologous to ruvB of E. coli. Mutat. Res. 385, 151–157. doi: 10.1016/s0921-8777(97)00048-7
Leulliot, N., Cladière, L., Lecointe, F., Durand, D., Hübscher, U., and van Tilbeurgh, H. (2009). The family X DNA polymerase from Deinococcus radiodurans adopts a non-standard extended conformation. J. Biol. Chem. 284, 11992–11999. doi: 10.1074/jbc.M809342200
Lim, S., Jung, J. H., Blanchard, L., and de Groot, A. (2019). Conservation and diversity of radiation and oxidative stress resistance mechanisms in Deinococcus species. FEMS Microbiol. Rev. 43, 19–52. doi: 10.1093/femsre/fuy037
Liu, S., Zhang, W., Gao, Z., Ming, Q., Hou, H., Lan, W., et al. (2013). NMR structure of the N-terminal-most HRDC1 domain of RecQ helicase from Deinococcus radiodurans. FEBS Lett. 587, 2635–2642. doi: 10.1016/j.febslet.2013.06.048
Lloyd, R. G., and Rudolph, C. J. (2016). 25 years on and no end in sight: a perspective on the role of RecG protein. Curr. Genet. 62, 827–840. doi: 10.1007/s00294-016-0589-z
McGlynn, P., Al-Deib, A. A., Liu, J., Marians, K. J., and Lloyd, R. G. (1997). The DNA replication protein PriA and the recombination protein RecG bind D-loops. J. Mol. Biol. 270, 212–221. doi: 10.1006/jmbi.1997.1120
McGlynn, P., Mahdi, A. A., and Lloyd, R. G. (2000). Characterisation of the catalytically active form of RecG helicase. Nucleic Acids Res. 28, 2324–2332. doi: 10.1093/nar/28.12.2324
Moe, E., Leiros, I., Smalås, A. O., and McSweeney, S. (2006). The crystal structure of mismatch-specific uracil-DNA glycosylase (MUG) from Deinococcus radiodurans reveals a novel catalytic residue and broad substrate specificity. J. Biol. Chem. 281, 569–577. doi: 10.1074/jbc.M508032200
Montague, M., Barnes, D., Smith, H. O., Chuang, R. -Y., and Vashee, S. (2009). The evolution of RecD outside of the RecBCD complex. J. Mol. Evol. 69, 360–371. doi: 10.1007/s00239-009-9290-x
Omelchenko, M. V., Wolf, Y. I., Gaidamakova, E. K., Matrosova, V. Y., Vasilenko, A., Zhai, M., et al. (2005). Comparative genomics of Thermus thermophilus and Deinococcus radiodurans: divergent routes of adaptation to thermophily and radiation resistance. BMC Evol. Biol. 5:57. doi: 10.1186/1471-2148-5-57
Pedersen, H. L., Johnson, K. A., McVey, C. E., Leiros, I., and Moe, E. (2015). Structure determination of uracil-DNA N-glycosylase from Deinococcus radiodurans in complex with DNA. Acta Crystallogr. D Biol. Crystallogr. 71, 2137–2149. doi: 10.1107/S1399004715014157
Piechura, J. R., Tseng, T. L., Hsu, H. F., Byrne, R. T., Windgassen, T. A., Chitteni-Pattu, S., et al. (2015). Biochemical characterization of RecA variants that contribute to extreme resistance to ionizing radiation. DNA Repair 26, 30–43. doi: 10.1016/j.dnarep.2014.12.001
Rajan, R., and Bell, C. E. (2004). Crystal structure of RecA from Deinococcus radiodurans: insights into the structural basis of extreme radioresistance. J. Mol. Biol. 344, 951–963. doi: 10.1016/j.jmb.2004.09.087
Ranawat, P., and Rawat, S. (2017). Radiation resistance in thermophiles: mechanisms and applications. World J. Microbiol. Biotechnol. 33:112. doi: 10.1007/s11274-017-2279-5
Rocha, E. P., Cornet, E., and Michel, B. (2005). Comparative and evolutionary analysis of the bacterial homologous recombination systems. PLoS Genet. 1:e15. doi: 10.1371/journal.pgen.0010015
Romero, Z. J., Chen, S. H., Armstrong, T., Wood, E. A., van Oijen, A., Robinson, A., et al. (2020). Resolving toxic DNA repair intermediates in every E. coli replication cycle: critical roles for RecG, Uup and RadD. Nucleic Acids Res. 48, 8445–8460. doi: 10.1093/nar/gkaa579
Rudolph, C. J., Upton, A. L., Briggs, G. S., and Lloyd, R. G. (2010). Is RecG a general guardian of the bacterial genome? DNA Repair 9, 210–223. doi: 10.1016/j.dnarep.2009.12.014
Servinsky, M. D., and Julin, D. A. (2007). Effect of a recD mutation on DNA damage resistance and transformation in Deinococcus radiodurans. J. Bacteriol. 189, 5101–5107. doi: 10.1128/JB.00409-07
Singleton, M. R., Scaife, S., and Wigley, D. B. (2001). Structural analysis of DNA replication fork reversal by RecG. Cell 107, 79–89. doi: 10.1016/S0092-8674(01)00501-3
Slade, D., Lindner, A. B., Paul, G., and Radman, M. (2009). Recombination and replication in DNA repair of heavily irradiated Deinococcus radiodurans. Cell 136, 1044–1055. doi: 10.1016/j.cell.2009.01.018
Slade, D., and Radman, M. (2011). Oxidative stress resistance in Deinococcus radiodurans. Microbiol. Mol. Biol. Rev. 75, 133–191. doi: 10.1128/MMBR.00015-10
Stelter, M., Acajjaoui, S., McSweeney, S., and Timmins, J. (2013). Structural and mechanistic insight into DNA unwinding by Deinococcus radiodurans UvrD. PLoS One 8:e77364. doi: 10.1371/journal.pone.0077364
Theobald, D. L., Mitton-Fry, R. M., and Wuttke, D. S. (2003). Nucleic acid recognition by OB-fold proteins. Annu. Rev. Biophys. Biomol. Struct. 32, 115–133. doi: 10.1146/annurev.biophys.32.110601.142506
Uchiumi, F., Seki, M., and Furuichi, Y. (2015). Helicases and human diseases. Front. Genet. 6:39. doi: 10.3389/fgene.2015.00039
Umate, P., Tuteja, N., and Tuteja, R. (2011). Genome-wide comprehensive analysis of human helicases. Commun. Integr. Biol. 4, 118–137. doi: 10.4161/cib.4.1.13844
Wang, J., and Julin, D. A. (2004). DNA helicase activity of the RecD protein from Deinococcus radiodurans. J. Biol. Chem. 279, 52024–52032. doi: 10.1074/jbc.M408645200
Wen, Q., Mahdi, A. A., Briggs, G. S., Sharples, G. J., and Lloyd, R. G. (2005). Conservation of RecG activity from pathogens to hyperthermophiles. DNA Repair 4, 23–31. doi: 10.1016/j.dnarep.2004.07.008
White, O., Eisen, J. A., Heidelberg, J. F., Hickey, E. K., Peterson, J. D., Dodson, R. J., et al. (1999). Genome sequence of the radioresistant bacterium Deinococcus radiodurans R1. Science 286, 1571–1577. doi: 10.1126/science.286.5444.1571
Wu, Y., Chen, W., Zhao, Y., Xu, H., and Hua, Y. (2009). Involvement of RecG in H2O2-induced damage repair in Deinococcus radiodurans. Can. J. Microbiol. 55, 841–848. doi: 10.1139/W09-028
Xue, Z. Y., Wu, W. Q., Zhao, X. C., Kumar, A., Ran, X., Zhang, X. H., et al. (2020). Single-molecule probing the duplex and G4 unwinding patterns of a RecD family helicase. Int. J. Biol. Macromol. 164, 902–910. doi: 10.1016/j.ijbiomac.2020.07.158
Zahradka, K., Slade, D., Bailone, A., Sommer, S., Averbeck, D., Petranovic, M., et al. (2006). Reassembly of shattered chromosomes in Deinococcus radiodurans. Nature 443, 569–573. doi: 10.1038/nature05160
Zegeye, E. D., Balasingham, S. V., Laerdahl, J. K., Homberset, H., Kristiansen, P. E., and Tonjum, T. (2014). Effects of conserved residues and naturally occurring mutations on Mycobacterium tuberculosis RecG helicase activity. Microbiology 160, 217–227. doi: 10.1099/mic.0.072140-0
Zhang, J., Zhou, R., Inoue, J., Mikawa, T., and Ha, T. (2014). Single molecule analysis of Thermus thermophilus SSB protein dynamics on single-stranded DNA. Nucleic Acids Res. 42, 3821–3832. doi: 10.1093/nar/gkt1316
Keywords: Deinococcus radiodurans, radiation resistance, DNA repair, RecG helicase, wedge domain
Citation: Jeong S-W, Kim M-K, Zhao L, Yang S-K, Jung J-H, Lim H-M and Lim S (2021) Effects of Conserved Wedge Domain Residues on DNA Binding Activity of Deinococcus radiodurans RecG Helicase. Front. Genet. 12:634615. doi: 10.3389/fgene.2021.634615
Edited by:
Hari S. Misra, Bhabha Atomic Research Centre (BARC), IndiaReviewed by:
Issay Narumi, Toyo University, JapanHuiming Lu, University of Texas Southwestern Medical Center, United States
Joanna Timmins, UMR5075 Institut de Biologie Structurale (IBS), France
Copyright © 2021 Jeong, Kim, Zhao, Yang, Jung, Lim and Lim. This is an open-access article distributed under the terms of the Creative Commons Attribution License (CC BY). The use, distribution or reproduction in other forums is permitted, provided the original author(s) and the copyright owner(s) are credited and that the original publication in this journal is cited, in accordance with accepted academic practice. No use, distribution or reproduction is permitted which does not comply with these terms.
*Correspondence: Sangyong Lim, c2F5bGltQGthZXJpLnJlLmty
†These authors have contributed equally to this work