- Department of Microbiology, University of Manitoba, Winnipeg, MB, Canada
Acinetobacter baumannii is classified as a top priority pathogen by the World Health Organization (WHO) because of its widespread resistance to all classes of antibiotics. This makes the need for understanding the mechanisms of resistance and virulence critical. Therefore, tools that allow genetic manipulations are vital to unravel the mechanisms of multidrug resistance (MDR) and virulence in A. baumannii. A host of current strategies are available for genetic manipulations of A. baumannii laboratory-strains, including ATCC® 17978TM and ATCC® 19606T, but depending on susceptibility profiles, these strategies may not be sufficient when targeting strains newly obtained from clinic, primarily due to the latter’s high resistance to antibiotics that are commonly used for selection during genetic manipulations. This review highlights the most recent methods for genetic manipulation of A. baumannii including CRISPR based approaches, transposon mutagenesis, homologous recombination strategies, reporter systems and complementation techniques with the spotlight on those that can be applied to MDR clinical isolates.
Introduction
Acinetobacter baumannii is a Gram-negative opportunistic pathogen causing disease in critically ill patients. It exhibits widespread resistance to all classes of antibiotics leading the World Health Organization (WHO) to classify it as a top priority for research (Tacconelli et al., 2018). This necessitates understanding the mechanisms of multi-drug resistance (MDR) and virulence. Tools that allow genetic manipulations are the key to unravel such mechanisms in A. baumannii. A host of current strategies are beneficial in manipulation of A. baumannii laboratory-strains, including ATCC® 17978TM and ATCC® 19606T, but may be insufficient when targeting strains obtained from hospitals and patients more recently, primarily due to their high resistance to antibiotics and the genomic diversity of A. baumannii (Diancourt et al., 2010; Zarrilli et al., 2013). Recently, genetic tools including the use of non-clinical antibiotic markers as well as those utilizing non-antibiotic markers have been generated for clinical strains of A. baumannii (Trebosc et al., 2016, 2019; Ducas-Mowchun et al., 2019; Lucidi et al., 2019). Many of these tools provide desirable traits in gene manipulation, including unmarked and scarless gene deletions, and complementation that mimic natural systems with no pleiotropic effects. This review compiles challenges with designing genetic tools for antibiotic resistant clinical isolates of A. baumannii as well as new developments in the field including high-throughput screening and genome editing such as CRISPR. More routine techniques such as homologous recombination and complementation are covered in excellent depth by Indranil Biswas and we direct the readers to this review (Biswas, 2015). Our review highlights techniques that have evolved since then with a focus on selection and counter-selection markers developed specifically for use in novel MDR and XDR clinical strains of A. baumannii, an ever-emerging concern in Acinetobacter genetics.
High-Throughput Genetic Screening
Transposon Mutagenesis
Transposon mutagenesis allows for global genetic information of an organism to be collected. Mutagenesis studies remain the mainstay in determining functional correlation with genotype (Russo et al., 2016). Three transposons used in A. baumannii are Tn5, Tn10, and himar1 mariner (Figure 1A). Tn5 and Tn10 integrate at non-specific target sites (Bender and Klecknert, 1992; Goryshin et al., 1998) typically minimizing insertion bias compared to mariner transposons whose insertion targets AT dinucleotides (Chiang and Rubin, 2002). Ideally saturation of insertion is achieved, meaning that for every possible insertion site, a transposon mutant exists. As the GC content of A. baumannii is around 40%, it presents a bias for mariner insertion lending advantages to the use of Tn5 or Tn10 (Chao et al., 2016). Due to the random integration of Tn5 there is a higher degree of saturation in A. baumannii (Gallagher, 2019). However, maximum library density is easily calculated for himar1 mariner due to its known target site, whereas this is more of a challenge with Tn5 as there are integration hotspots (Green et al., 2012), resulting in insertion bias with specific sites being overrepresented. It is critical to validate at least two insertion sites per gene to ensure no phenotype is overlooked (Gallagher et al., 2015). Most mutagenesis studies consist of the same basic strategies. An insertion library is generated via delivery of transposon and its cognate transposase of choice resulting in a single cell with one insertion within a population. The mutants are pooled and those mutants with disrupted genes altering fitness under appropriate conditions are selected for or against (Figure 1).
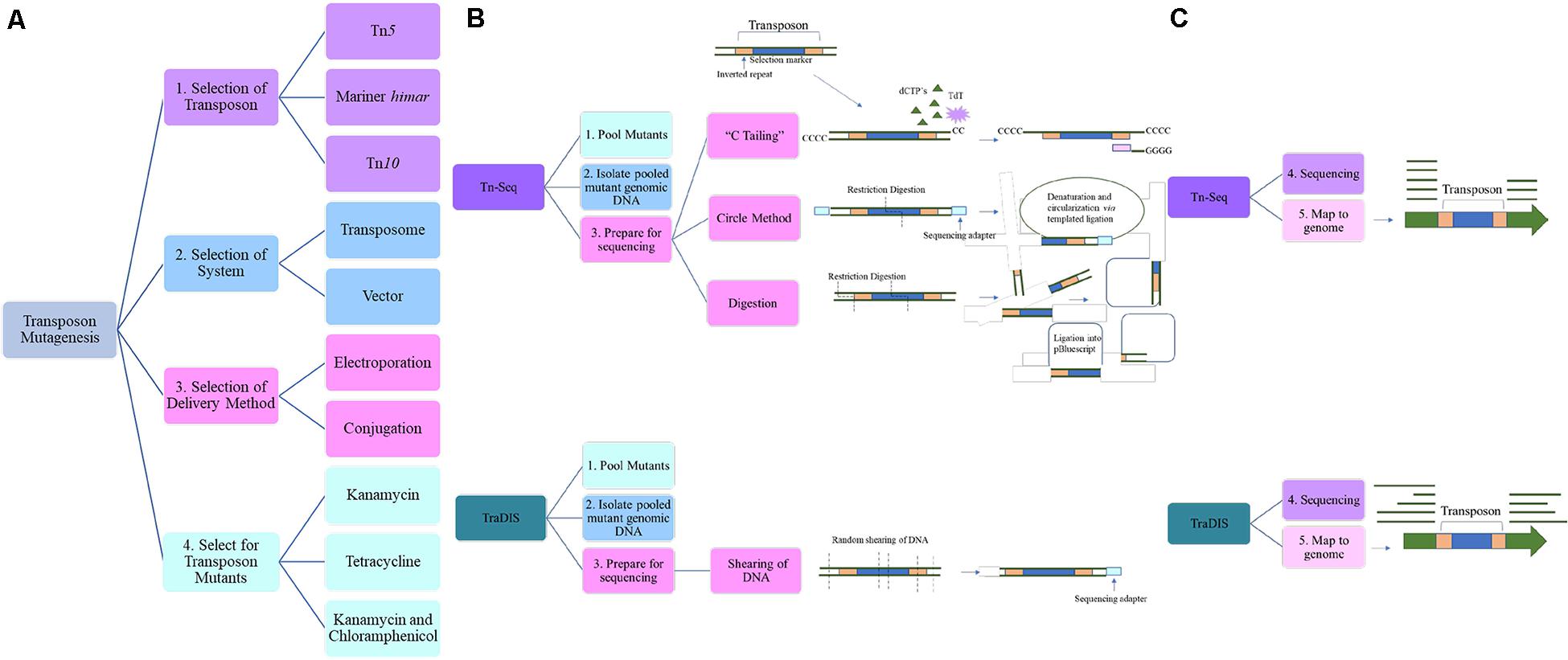
Figure 1. An overview of the steps and considerations when undertaking transposon mutagenesis. (A) Considerations for transposon mutagenesis and Tn-seq. During the selection of the transposon, the type should be taken into consideration and in A. baumannii Tn5, Tn10, and mariner himar have been successful. The determination of the transposon system is the next step and includes both plasmid or transposome based methods and this will play a role in the method of delivery to the strain of interest. Following this, the selection marker must be considered, and susceptibility profile of the strain taken into account. (B) After the mutant library has been selected for and the pooled mutant genomic DNA extracted, the library must be prepared for sequencing. There are three methods of sequencing preparation for Tn-seq. “C-tailing” involves the addition of dCTPs and the terminal deoxynucleotidyl transferase (TdT) and a poly-C tract is added to the 3′ ends of the fragmented DNA. The circle method involves the restriction digestion of pooled DNA using an enzyme that digests within the transposon as well as the addition of a sequencing adapter. This is then circularized via templated ligation. Lastly, random restriction digestion of the DNA is followed by ligation of the varying length fragments into a vector such as pBluescript. (C) Next-generation sequencing (NGS) techniques are then used. Mapping of the reads to the genome, show an absence of reads where the transposon integrated into the gene and thus allows for determination of insertion.
Most commonly, Tn5 saturated libraries have been generated with the EZ-Tn5TM kit (Lucigen Biotechnologies, Middleton, WI, United States) in a wide range of strains, namely, AB5075 (Gallagher et al., 2015; Tipton et al., 2017; Pérez-Varela et al., 2020), BAL062 (Hassan et al., 2016), AB0057 (Crépin et al., 2018), and ATCC® 17978TM (Subashchandrabose et al., 2015). The transposome, composed of the transposon DNA sequence and transposase bound to the 19 base pair mosaic end recognition sequence, are commercially pre-assembled requiring less upstream genetic engineering steps which is beneficial. The selection marker within the transposon is customizable. Electroporation into competent cells allows transposome entry (Gallagher, 2019) however, this step cannot be easily performed in a high through-put manner leading to a preference for conjugation-based protocols (Kazi et al., 2020). One vector-based Tn5 systems also exist; pRL27 system in ATCC® 17978TM (Kim et al., 2019) which can either be delivered to A. baumannii through electroporation or conjugation. Kim et al. mutagenized ATCC® 17978TM and screened for loss of biofilm formation leading to the establishment of BfmRS two-component system’s role in outer membrane vesicle (OMV) production (Kim et al., 2019).
Mariner (Di Venanzio et al., 2019; Kazi et al., 2020; Lopez et al., 2020; Sun et al., 2020) and Tn10 (Giles et al., 2015; Geisinger et al., 2019, 2020; Roy et al., 2020) transposons also prove useful, in A. baumannii. The suicide vector pJNW684 containing the himar1 mariner transposase, transposon and RP4/oriT/oriR6K origin of replication requires the λpir gene product for replication (Wang et al., 2014). Delivery to A. baumannii occurs via bi-parental mating with an Escherichia coli diamenopimelic acid (DAP) auxotroph which allows for selection of recipient cells (Wang et al., 2014; Kazi et al., 2020). This vector also contains the Acinetobacter specific rpoD promoter to control transposase expression. A different mariner-based system, pSAM::OmpAp+Tn903, has been applied to ATCC® 17978TM (Di Venanzio et al., 2019) and the Canadian clinical isolate, AbCAN2 (Lopez et al., 2020). An AbCAN2 transposon mutant library provided the basis for determining the essentiality of the Vgr spike protein in the type VI secretion system (T6SS) and its role in interbacterial competition (Lopez et al., 2020). A similar library in ATCC® 17978TM laid the foundation for the discovery that suppression of the T6SS allows for dissemination of MDR plasmids within A. baumannii but also within the Acinetobacter species itself (Di Venanzio et al., 2019). Tn10 delivery via either electroporation or conjugation, pBSL180 (Roy et al., 2020) pDL1073 (Geisinger et al., 2019, 2020), and pLof (Giles et al., 2015) have successfully generated libraries for A. baumannii. The use of pBSL180 (Alexeyev and Shokolenko, 1995) has been marked in other Gram-negatives such as E. coli (Scaletsky et al., 2005), Shenwanella oneidensis and Desulfovibrio desulfuricans (Groh et al., 2005). In ATCC® 17978TM, the role of carboxy-terminal processing protease in motility and membrane integrity was characterized (Roy et al., 2020). A drawback of using plasmid-based systems is the risk of integration of the backbone into the mutant chromosome. This can be mitigated by the use of the conditional origin of replication ensuring that the transposase is not maintained in A. baumannii (Kazi et al., 2020) which has been engineered into these common systems.
After transposition, insertion mutants are isolated on selective media (Figure 1A). An antibiotic marker is engineered within the transposon that allows for the selection of a successful integration event. Currently tetracycline (Pérez-Varela et al., 2020), kanamycin (Gallagher et al., 2015; Hassan et al., 2016; Geisinger et al., 2019; Kazi et al., 2020; Sun et al., 2020), and a combination of kanamycin and chloramphenicol (Kim et al., 2019; Lopez et al., 2020) are used during library generation in A. baumannii. These selection markers have been used in a variety of clinical isolates including AB5075, AbCAN2, BAL062, and AB0057 (Supplementary Table 1). As these isolates remain susceptible to tetracycline, kanamycin and chloramphenicol, the requirement for non-antibiotic or non-clinical drug selection is negligible. However, selection conditions become increasingly critical factors to consider as the diversity and resistance profile of A. baumannii is hyper-plastic. Following this, a critical step in library establishment is the storage of the mutants. To ensure that unnecessary selection pressures are avoided, freezing of the pooled mutants must occur very shortly after selection (Kazi et al., 2020). However, as research progresses, storing and exposing strains of interest to new conditions/stress allows investigation of novel effects as demonstrated for an XDR clinical lung isolate HUMC1 (Luna et al., 2020). The authors repurposed a previously generated mutant library in AB5075 in order to validate results observed in HUMC1 (Gallagher et al., 2015). A screen for susceptibility of HUMC1 to a small molecule library in the absence of rich media was undertaken. A notable hit, resulting in an increase in susceptibility to rifabutin, led to the investigation of the molecular mechanism in HUMC1 and also in AB5075. Transposon mutant libraries demonstrate their versatility in the foundation of studies and as demonstrated by Luna et al. (2020) can be repurposed years in the future to investigate and validate novel findings.
Transposon-Sequencing (Tn-Seq)
Transposon mutagenesis is coupled with downstream steps for further characterization of the mutant library, making it the backbone of many genetic studies (Figure 1B). With the advent of Next-Generation-Sequencing (NGS), it is less cumbersome to sequence the region of integration, and the whole genome, identifying the disrupted genes. Generally, genomic DNA of the pooled mutants is extracted and NGS is used to sequence the transposon integration site and these reads are mapped to the genome. This approach means that mutants with fitness defects are under-represented due to lack of survival. However, this challenge can be overcome by generating conditional libraries, one exposed to particular in vitro or in vivo conditions, for example and the other generated without any selective pressure. A recent study characterized phenotypic responses to ciprofloxacin in ATCC® 17978TM by comparing those pooled transposon mutants exposed and those untreated (Geisinger et al., 2019). To elucidate the interplay in ciprofloxacin resistance mechanisms, the most common fluoroquinolone resistance alleles, those in gyrA and parC, were generated. These became the parent strains for the transposon mutagenesis study. A target-dependent prophage and SOS response under fluoroquinolone stress conditions was observed which characterizes the hierarchy of induced resistance to ciprofloxacin.
Following mutagenesis, genomic DNA of the pooled mutants is extracted and sheared. A method known as “C-tailing” facilitates the sequencing procedure (Klein et al., 2012). It involves the addition of poly(C) tails to the 3′ end of the fragmented DNA via the use of dCTPs and terminal deoxynucleotidyl transferase (TdT). A round of PCR enriches the insertion sequences via a primer with a 5′ poly(G) tract that binds the poly(C) region previously added and a region that binds the transposon (Gallagher, 2019). Alternatively the use of a protocol originally developed in Pseudomonas aeruginosa, the circle method allows for selection of transposon insertion sites (Gallagher et al., 2011). In this technique, after size selection, a sequencing adapter is ligated onto the end of the transposon and circularized. Any remaining uncircularized and denatured fragments, which is the majority, are degraded. A final PCR step amplifies the transposon junction, initial sequencing primer and introduces the second required sequencing primer. Validating this approach, Gallagher et al. (2015) developed a three-allele library using both the circle and terminal deoxynuclease transferase methods of sequencing preparation, generating similar libraries and demonstrating that any amplification-based bias in the circle method is negligible. Alternate sequence preparation includes digestion of isolated transposon mutant DNA with restriction enzymes and ligation into a cloning vector such as pBluescript. SK II (Giles et al., 2015).
After sequencing, most commonly by Illumina, and mapping the sequencing reads to the genome, the disrupted genes are identified (Figure 1C). Fitness scores are assigned to those mutants based on their abundance compared to an untreated condition. To facilitate the ease of comparison across mutagenesis studies, fitness values are normalized to insertions in neutral sites; pseudogenes or those located in known intergenic regions where insertion is shown to cause no growth defect (Geisinger et al., 2019). The importance of Tn-seq has been demonstrated in a number of studies. It has been used to elucidate many pathways including the morphological switch from opaque to translucent corresponding to virulent and avirulent phenotypes in the American wound isolate AB5075 (Tipton et al., 2017; Chin et al., 2018) as well as the role of RelA in the same strain (Pérez-Varela et al., 2020).
TraDIS and TraDISort
Transposon Directed Insertion Sequencing (TraDIS) (Langridge et al., 2009) is a technique that is highly similar to that of Tn-seq but differs slightly in sample preparation prior to sequencing (Figure 1B). The DNA is randomly cleaved resulting in variable sized fragments and during sequencing library preparation, an additional round of PCR is performed using a transposon specific primer and a sequencing specific adapter primer (Langridge et al., 2009; Barquist et al., 2016). Enrichment, such as this, allows for deeper sequencing coverage and thus higher confidence on transposition site but also introduces additional PCR bias as shorter templates are preferentially amplified over longer ones (Van Opijnen and Camilli, 2013). Utility of TraDIS was recently shown in a Vietnamese clinical isolate, where additional genes involved in colistin resistance such as those involved in membrane maintenance and regulation of transcription and translation were identified (Boinett et al., 2019). TraDIS has evaluated ATCC® 17978TM in respect to genes that are required for murine septicemia (Subashchandrabose et al., 2015). A study from the same group, generated a mutagenesis library in the clinical isolate AB0057 in order to mirror their initial study in ATCC® 17978TM (Crépin et al., 2018). Interestingly, in this study, to successfully select for the transposon insertion mutant pool, a kanamycin susceptible isogenic strain was created by deletion of AB57_0288, a gene that encodes aminoglycoside O-phosphotransferase. The mutagenesis was performed in this susceptible strain which also demonstrated similarities in murine virulence (Crépin et al., 2018). This highlights the challenges when working with MDR clinical strains as a susceptible parent strain is necessary to select for the transposon mutants. Due to this screen, a lytic transglycosylase, MltB, was revealed as essential in the murine infection model. With an elegant approach, Hassan et al. (2016) have identified a novel regulator for the efflux pump AvmA in AB5075 by taking advantage of the fluorescent property of the known efflux pump substrate, ethidium bromide, and combining it with TraDIS to create TraDISort. Using Fluorescence Associated Cell Sorting (FACS) to detect substrate fluorescence with TraDIS, TraDISort allowed for the selection of those mutants that had insertions in efflux pump encoding genes or their regulators based on their response to ethidium bromide. This method was validated with the identification of previously characterized efflux pumps, AdeABC and AdeIJK and although this is the only instance of TraDISort to date, provides insight into the power of TraDIS in combination with other sophisticated techniques in A. baumannii.
A very recent study in Acinetobacter baylyi demonstrated the power of Tn-seq to determine the importance of essential genes. Gallagher et al. (2020) harnessed the mutagenizing and sequencing aspects of Tn-seq coupled with the natural transformation efficiency of Acinetobacter baylyi. A transposon mutant library was generated using a Tn5 transposon. The mutants’ DNA was pooled and transformed into wild-type A. baylyi then Tn-seq was performed over a specified time period. The speed by which the loss of DNA from those mutants whose transposon was integrated into an essential gene was detected via Tn-seq determined the criticality of the gene. In other words, the faster the depletion of mutant DNA from an essential gene integration, the more essential the gene to cellular functions. Although this study is limited to A. baylyi, it demonstrates further applications, similar to those shown by TraDISort, that can be coupled with transposon mutagenesis and their impact on the study of the organism as a whole.
Genome Editing
Homologous Recombination
The genetic tools currently used for genome editing are based on harnessing existing molecular phenomenon. Homologous recombination, CRISPR and DNA repair mechanisms are such examples. One of the most common gene deletion strategies, previously and recently implemented with success in extensively drug resistant (XDR) strains of A. baumannii, uses a two-step homologous recombination approach (Figure 2A; Krasauskas et al., 2019; Xu Q. et al., 2019). Typically, this method involves the design of a knockout cassette that consists of an antibiotic resistance marker flanked by homologous regions of the target gene or adjacent. The cassette can be created using several methods such as PCR (Choi and Schweizer, 2005) or Gibson assembly (Gibson et al., 2009). There are two commonly used approaches to use the knock-out cassette, using suicide plasmids or using the linear DNA fragment.
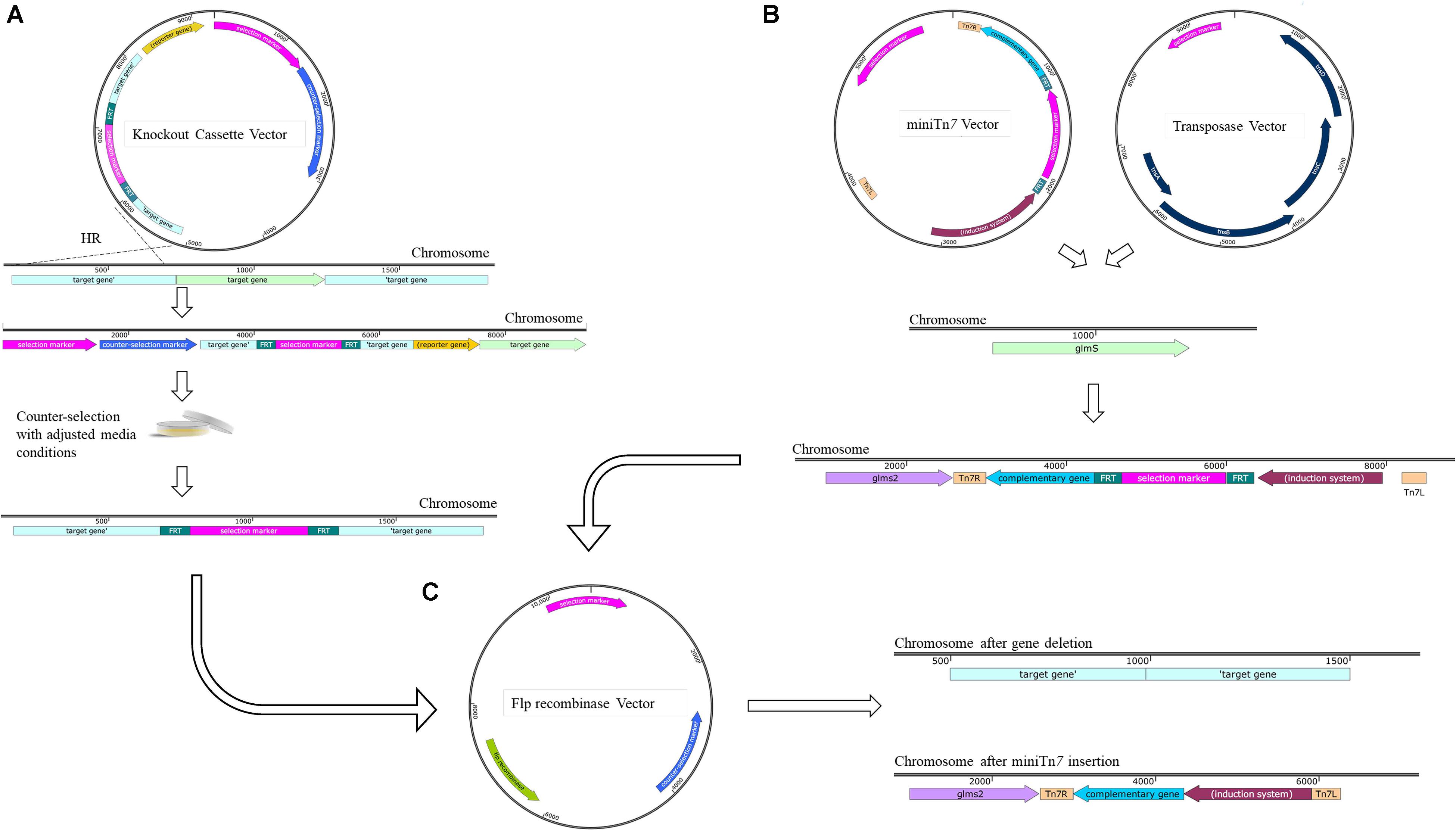
Figure 2. Current genetic techniques available for use in A. baumannii with common removal of selection marker. (A) Gene deletion via homologous recombination (HR) using suicide plasmid and A knockout cassette is designed with non-clinical antibiotic resistance gene of choice such as hygromycin or gentamicin flanked by Flp Recombinase Target (FRT) sites and homologous regions situated up and downstream of gene of interest. The knockout cassette can be then cloned into a suicide vector or maintained as a linear product. The use of counter-selection markers such as sacB in the presence of high quantities of sucrose enable curing of the vector. Unmarked deletions are then generated via the use of the Flp Recombinase acting on the engineered FRT sites. (B) Cloning and complementation with the minTn7 system. In conjunction with the transposase, TnsABCE, integration of the miniTn7 plasmid occurs at the neutral position upstream of the glmS2 gene. (C) Removal of the selection marker such as zeocin, apramycin, or hygromycin occurs via the Flp recombinase and is common between the mini-Tn7 system and gene deletion via suicide plasmid. All maps were created using SnapGene software (from Insightful Science; available at snapgene.com). Not every feature of each plasmid is shown, only those relevant for selection, counter-selection and unique properties are displayed.
Gene Deletion Strategies Using Suicide Plasmids
The knockout cassette created can be cloned into a suicide knockout vector with selection (Table 1) and counter-selection markers (Table 2). Alternatively, the knock-out cassette can be assembled directly into the suicide vector using restriction digestion. However, with the advances in molecular techniques, this is the less desirable method due to limitations in unique restriction-digestion sites that can be used for cloning. The suicide vector containing the knock-out cassette is introduced into the host strain by electroporation, chemical transformation, or mating. The two-step allelic exchange, utilizing a non-replicative plasmid-based method follows (Hamad et al., 2009; Amin et al., 2013; Oh et al., 2015; Lee et al., 2018; Skerniškytė et al., 2019). Variations include those originally designed in P. aeruginosa and Burkholderia spp. The methods of Choi and Schweizer have been adapted for use in A. baumannii (De Silva and Kumar, 2018; De Silva et al., 2020) and includes the initial step of Splicing Overlap Extension (SOE), which introduces an antibiotic resistance gene flanked by Flp Recombinase Target (FRT) sites into the flanking homologous regions of the target gene to allow for selection of recombinants and antibiotic gene removal, respectively (Choi and Schweizer, 2005). Applications in clinical isolates using this method (Supplementary Table 1) have been successful depending on the type of selection and counter-selection markers used (Trebosc et al., 2016, 2019; Xu Q. et al., 2019).
The removal of the antibiotic cassette after the second homologous recombination event is highly desirable to avoid any possible polar effects (Figure 2C). Further, the presence of an antibiotic resistance cassette can interfere with phenotypic characterization such as susceptibility assays. Thus, the evaluation of a true gene deletion event cannot be accomplished, making the removal of the antibiotic resistance cassette necessary. This has been accomplished with remarkable success using the FLP recombinase system (Hoang et al., 1998). This system allows for the generation of unmarked deletions leaving behind the FRT scar sequences (De Silva and Kumar, 2018; Wang Y. et al., 2019). The introduction of additional bases in the form of the FRT site scars, have not been observed to have any pleiotropic effects. Several versions of the Flp recombinase-containing plasmids are now available. For example, those with a temperature-sensitive replicon (Choi et al., 2008) allow for greater ease of selection after the first recombination event. The modifications in the original Flp-recombinase containing vectors has allowed for their use in hyper-resistant clinical isolates of A. baumannii (Ducas-Mowchun et al., 2019).
Gene Deletion Strategies Using Linear DNA Fragment
A. baumannii is well known to readily uptake exogenous DNA and this characteristic can be exploited for genetic manipulations (Domingues et al., 2019). One particular strategy utilizes the native DNA repair components, in particular the RecET recombinase system in combination with site-specific homologous recombination of a linear knockout cassette to achieve gene disruption in a single step (Tucker et al., 2014). The knockout cassette contains an antibiotic resistance selectable marker (Table 1), allowing for selection of cells with successful homologous recombination events. As with the previously mentioned approaches, removal of the antibiotic resistance marker can be performed by a Flp recombinase.
A recently developed single step homologous recombination technique is quite convenient (Trebosc et al., 2016) and allows for creation of scarless deletions via a single step method. The authors showed the versatility of this technique by deleting adeR (the gene encoding the response regulator that controls the expression of AdeABC efflux pump in tandem with the sensor kinase AdeS) in 10 different clinical strains isolated from various geographical sources (Trebosc et al., 2016). Scarless deletions also allow for the creation of multiple gene deletions in the same strain as the same selection marker can be reused. Again shown by further deleting pmrA, eptA-1, eptA-2, eptA-3 in the same strains (Trebosc et al., 2019). Absence of antibiotic resistance gene in the knockout cassette excludes requirement to use the Flp recombinase making this approach scarless.
Of late, a two-step linear chimeric PCR product deletion method was validated via the deletion of the mobile genetic resistance element AbaR (Godeux et al., 2020). A PCR product is synthesized with aacC4 and sacB genes flanked by regions homologous to those of the target gene (Figure 3). Transformation of such PCR chimera allows the first recombination to result in an interruption of the gene of interest with the apramycin resistance cassette and sacB. Cells after this first recombination event are selected on apramycin. To obtain a markerless deletion, an additional PCR product is generated with similar homologous flanking regions. Successful mutants are selected based on apramycin susceptibility and sucrose resistance. Strains AB5075 and AYE were subjected to this strategy and their AbaR resistance islands deleted as shown by reduced susceptibility of AYE to aminoglycosides, tetracycline and sulfonamides due to the loss of resistance island, similar to previous findings as a result of the loss of AbaR (Kochar et al., 2012). This strategy provides the advantage of a markerless, scarless deletion and is sensitive enough to produce single base-pair mutations (Godeux et al., 2020).
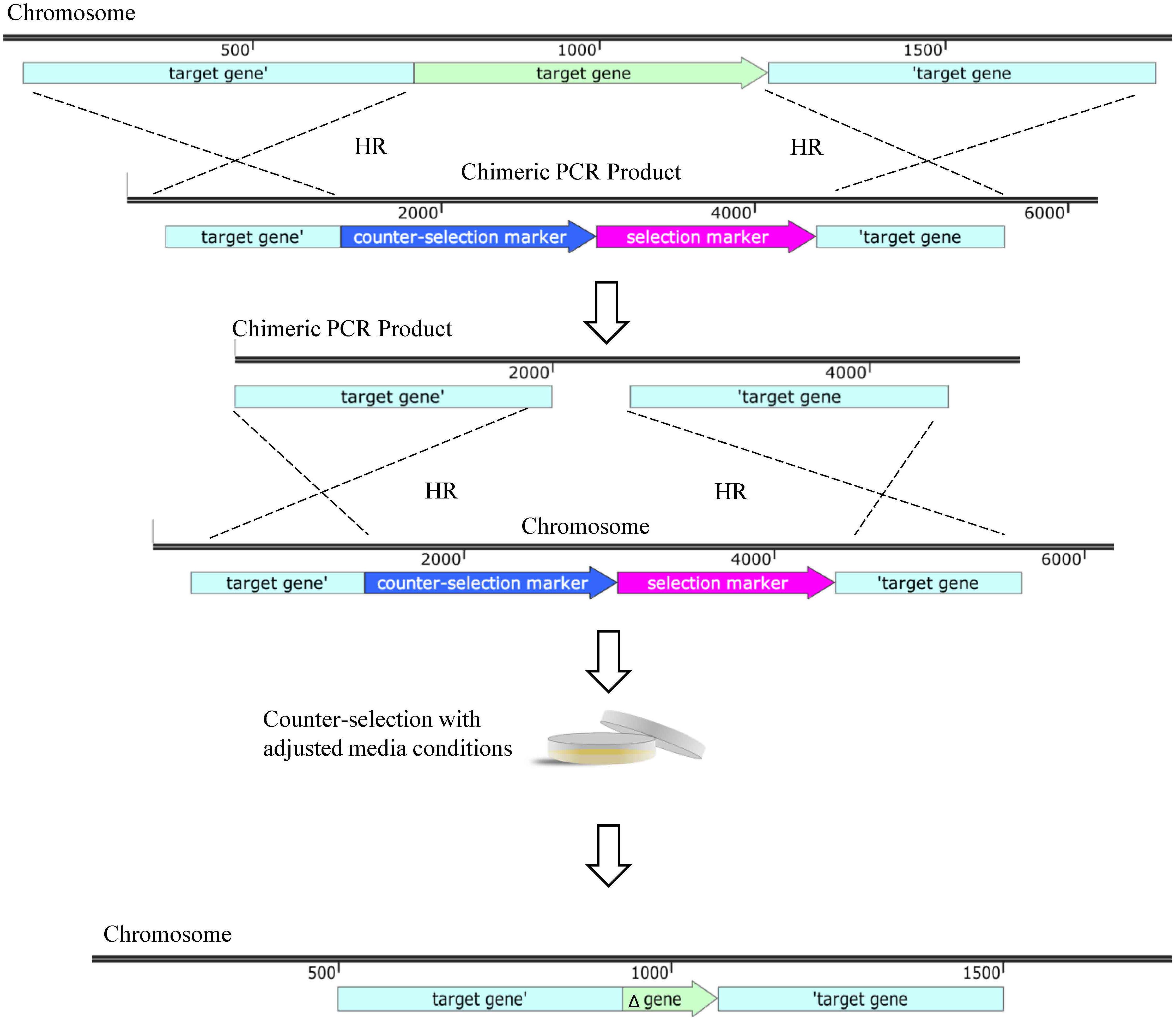
Figure 3. Linear DNA deletion technique. Gene deletion via homologous recombination (HR) using linear knockout cassette. A chimeric PCR product is designed with a selection marker such as apramycin resistance and a counter-selection marker such as sacB flanked by regions of homology to those surrounding the target gene. HR allows for disruption of target gene. A second chimeric PCR product is generated with homologous regions to the target gene and counter-selection allows for the second recombination event to select for removal of the selection and counter-selection markers leaving a scarless deletion. All maps were created using SnapGene software (from Insightful Science; available at snapgene.com). Not every feature of each plasmid is shown, only those relevant for selection, counter-selection and unique properties are displayed.
CRISPR
Immunological Role
Bacteria are constantly preyed upon by bacteriophages and therefore have evolved multiple antiviral strategies. Antiviral immunity of bacteria and archaea consists of a combination of innate and adaptive antiviral defense mechanisms. The innate defense mechanisms include receptor masking (Makarova et al., 2011, 2013; Samson et al., 2013), restriction–modification systems (Tock and Dryden, 2005), bacteriophage exclusion (BREX) (Goldfarb et al., 2015), Argonaute (Ago) family facilitated DNA interference (Swarts et al., 2014), and abortive infection (Chopin et al., 2005). Adaptive immunity is imparted through a Clustered Regularly Interspaced Short Palindromic Repeats (CRISPR) and associated Cas system. CRISPR-Cas is a common antiviral immunity system found in 45% of bacteria (Grissa et al., 2007). In addition to cas genes, a series of direct repeats and spacers termed as CRISPR arrays are responsible for target specificity. These spacers are derived from predatory viruses that infected the bacterium previously, thereby imparting immune memory (Bolotin et al., 2005; Mojica et al., 2005; Barrangou, 2015). By sequence specific targeting of the foreign (viral, plasmid) genetic material, the CRISPR-Cas system protects against mobile genetic elements (MGEs), thereby defending against viral predation (Barrangou et al., 2007; Brouns et al., 2008; Marraffini and Sontheimer, 2008; Hale et al., 2009; Garneau et al., 2010; Terns and Terns, 2011). The complexity of the CRISPR-Cas system warranted a comprehensive classification of the system. This was achieved by basing the classification on phylogenetic analysis of conserved Cas proteins, type and number of cas genes and gene arrangements (Makarova and Koonin, 2015). To date, four types and 12 subtypes (excludes six variant subtypes) of CRISPR-Cas system have been reported.
The target recognition by a CRISPR-Cas system depend on the Cas protein binding Protospacer Adjacent Motif (PAM), leading to unwinding of the adjacent dsDNA helix (Sternberg et al., 2014; Redding et al., 2015; Jones et al., 2017). The RNA-directed aspect of the CRISPR-Cas is dependent on two RNAs, the CRISPR RNA (crRNA) and a trans-acting RNA (tracrRNA). The tracrRNA, which imparts partial complementarity, and the crRNA, can be combined to form a single guide RNA (sgRNA), that performs DNA target recognition and recruits Cas nuclease needed to perform genome editing (Figure 4A; Gasiunas et al., 2012; Jinek et al., 2012). The substrate specificity and the simplicity of the system that includes designing sgRNA makes CRSIPR an obvious choice for genetic manipulation. Following unwinding of the dsDNA, the single guide RNA (sgRNA) hybridizes to form a R-loop structure. This allows recognition of seed sequence near PAM elements enabling complementarity with the crRNA spacer and the CRISPR-Cas system to execute its effect (Figure 4B; Szczelkun et al., 2014; Rutkauskas et al., 2015; Jones et al., 2017; Xue et al., 2017).
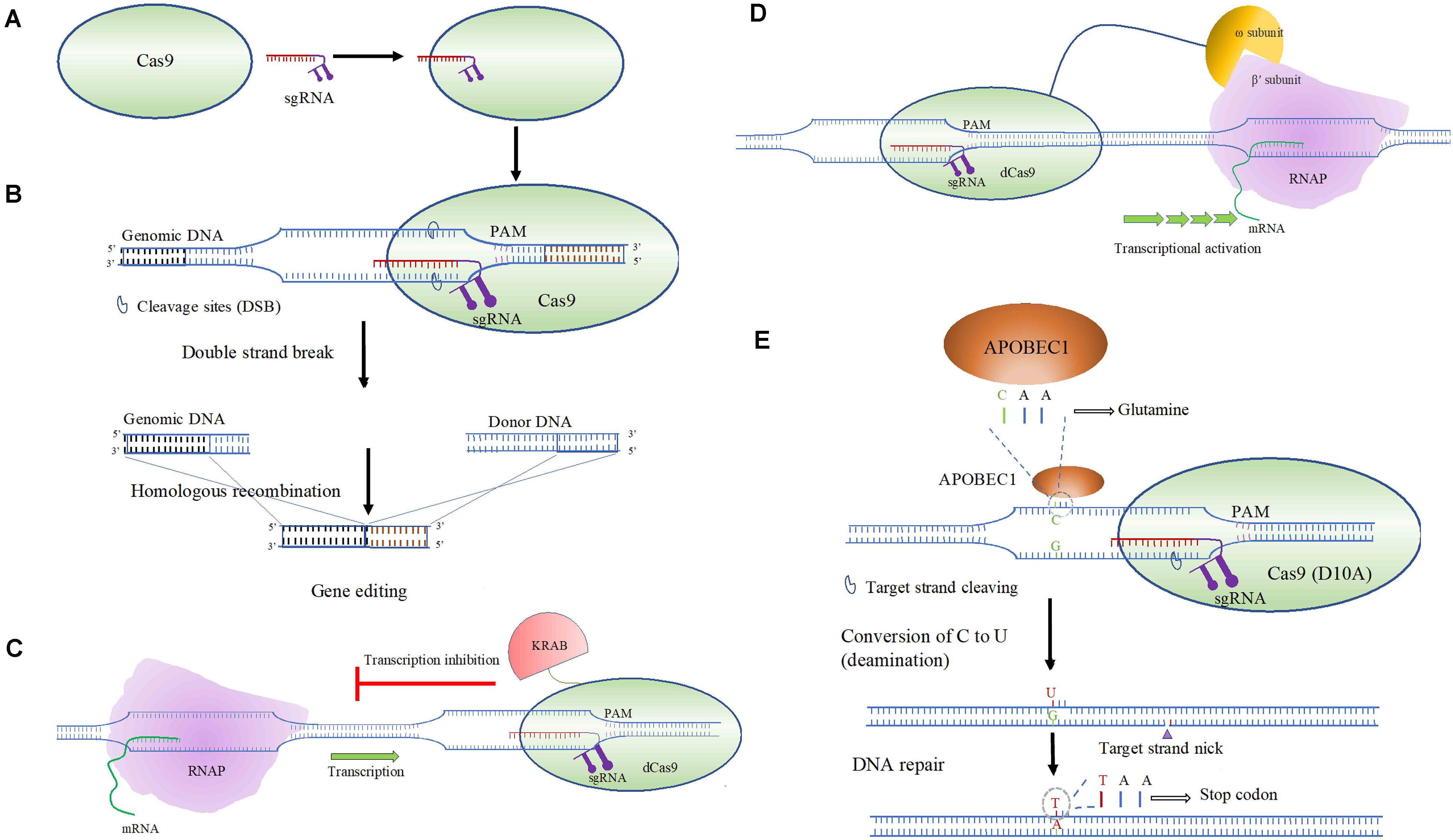
Figure 4. An overview of CRISPR-Cas9-based gene editing in bacteria. (A) Cas9 or a dCas9 binds to sgRNA that leads to an RNA-guided binding of the target DNA. The dCas9 may be assisted with transcriptional effectors (activator or an inhibitor). (B) Gene editing undertaken by Cas9-sgRNA target binding, double strand break and subsequent HR repair. (C) CRISPRi based targeted transcriptional inhibition through Cas9 fused to KRAB transcriptional inhibitor. (D) Activation of transcription driven by ω subunit of RNAP fused to dCas9, enabling RNAP recruitment through binding to the β’ subunit of RNAP. (E) Targeted base-editing performed by APOBEC1 fused to Cas9 (D10A), converting a triplet codon for glutamine to a stop codon.
Utility in Genome Editing
In addition to creating specific gene knockouts, adaptation of the CRISPR-Cas system is implemented to perform interference or activation, termed CRISPRi and CRISPRa, respectively, enabling transcriptional control (Qi et al., 2013). Transcriptional repression or knockdown via CRISPRi is an alternative to mRNA targeting RNAi and differs from the later as it may block either transcriptional initiation or elongation (Figure 4C). This system uses a deactivated nicking endonuclease Cas9 (dCas9) for a targeted RNA-directed transcriptional control. dCas9 may need additional helper proteins or effector proteins that allows higher degree of transcriptional repression. As shown in Figure 4C, notable effector proteins include KRAB (Krüppel-associated box) domain of Kox1 and the CS (chromoshadow) (Qi and Lo, 2017). Bacterial gene knockdown using CRISPRi has been investigated in Gram-negative E. coli (Jiang et al., 2013; Li et al., 2016) and Gram-positive Bacillus subtilis (Peters et al., 2016). This approach is tunable and can be modified to perform both moderate and high degree of over-expression and knockdown by using inducible promoters (Li et al., 2016). Alternatively, CRISPRa is a targeted gene activation approach, where a transcriptional activator domain or a subunit of RNAP could be fused to dCas9 augmenting rate or initiation of transcription through RNAP recruitment (Figure 4D; Dong et al., 2018). Use of omega (ω) subunit of RNAP has shown to be an effective transcriptional activator in E. coli (Dove and Hochschild, 1998; Bikard et al., 2013).
Diversity of CRISPR Systems
The utility of CRISPR-Cas9 system (Figure 4) has been demonstrated in Gram-negative bacteria including E. coli, P. aeruginosa, and Burkholderia spp. (Jiang et al., 2015; Chen et al., 2018; Hogan et al., 2019). Previous studies showed instances where mismatch and/or partial match of sgRNA to target DNA is tolerated often leading to off-target effects (Graham and Root, 2015; Lee et al., 2016). Although shown in mammalian cell lines, it is possible that in-frame mutations affect the CRISPR based knock-out efficiency. The reduction of in-frame mutation was addressed by the authors by developing a microhomology prediction thereby improving knock-out efficiency (Bae et al., 2014). A large volume of data on genome editing is currently available. To assist a CRISPR based gene editing approach as a method of genome editing, by improving both efficiency and accuracy, we rely on multiple computational tools, which are covered extensively in a review published previously (Chuai et al., 2017). The scope of the use of computational tools encompasses in silico sgRNA design to enhance target specificity, CRISPR based sgRNA off-target profiles and analyzing outcomes of CRISPR experiments. These use posterior NGS to characterize insertions, deletions, and homologous recombination at the intended target sites. Even with the advancement of in silico methods in predicting off-target effects, the measured process recently proposed combining both in silico and experimental approaches (Hendel et al., 2015; Lee et al., 2016). At the core of these approaches remain NGS and subsequent data analysis to identify functional cleavage sites, in combination with genome wide techniques including double-strand break (DSB) capture and protein binding site capture (Lee et al., 2016). Another study (Karlapudi et al., 2018) using A. baumannii strain M2, evaluated the application of web-based tools (CHOPCHOP, CRISPR-ERA, CCTop and E-CRISP) for in silico sgRNA design. Individual web-based tools consider one or multiple relevant factors that includes location of (PAM), sequence alignment scores attributed to GC content and exon position thereby predicting on-target efficacy.
Exogenous CRISPR Systems in A. baumannii
In order to adapt a CRISPR based system to be used in A. baumannii, several modifications to the system were made and tested. Based on the strategy previously outlined by Hsu et al. (2013), the authors (Wang Y. et al., 2019) constructed a plasmid containing expression cassettes for Cas9 nuclease from Streptococcus pyogenes and the corresponding sgRNA (Hsu et al., 2013). In addition, to enhance the recombination efficiency of weak homologous recombination based DSB repair found in A. baumannii, authors developed an exogenous recombination system, that involves a two-plasmid system with each plasmid capable of replicating in both E. coli and A. baumannii (Fürste et al., 1986). The selection of RecAb from the A. baumannii IS-123 strain (Tucker et al., 2014) over recombinases from other species resulted in > 10-fold higher efficiency, reflected in the higher number of colonies selected for during a targeted deletion of the regulator oxyR. The amount and length of the repair template was also optimized, which greatly improved the editing efficiency. The authors also tested genome editing efficiency that is demonstrated in lab strains ATCC® 17978TM, and ATCC® 19606T and in one clinical isolate A. baumannii ABH2. The reason for the use of multiple strains was the expected variation in efficiency of CRISPR-Cas based genome editing owing to differences in genomic background.
To investigate amino acid residues in the active site of OxyR that are critical for H2O2 sensing, point mutations were introduced in ABH2 using the exogenous CRISPR-Cas system (Wang Y. et al., 2019). By inserting an artificial spacer to replace the native spacer and then introducing a point mutation during restoration of the native spacer, 13 mutant strains were generated. Three of these mutations included conserved non-critical amino acids T104, H201, and R270 found in OxyR of other bacteria. These strains as expected did not show any deficiency with respect to H2O2 sensitivity. A known residue, C202, and three new residues, E130, S133, and S226 were determined to be critical for OxyR function. In A. baumannii, many acquired resistance genes are associated with mobile elements (plasmids and transposons), and undesired recombination events or the loss of a plasmid encoding CRISPR elements may occur when performing CRISPR-Cas based gene editing (Wang et al., 2018). An alternative approach is a cytidine base-editing system of targeted C to T conversion to achieve gene disruption. This method is independent of DSB and the need of a donor template. A C to T conversion can change a codon, for example CAA or CAG to a stop codon (TAA or TAG) (Figure 4E). A single plasmid system pBECAb-apr was constructed containing expression cassettes for a sgRNA and a fusion protein. One of the proteins in the fusion was a APOBEC1 deaminase that catalyzes the conversion of C to U in the displaced DNA strands. The other, Cas9(D10A) nickase cleaves the non-edited DNA strand. DNA repair or replication converts U:G originated from C:G to T:A. The functionality of the cytidine base-editing system was confirmed in A. baumannii ATCC® 17978TM.
As an exogenous CRISPR-Cas system may fail in some species, functional endogenous CRISPR-cas in many bacteria had been used for successful gene editing. In Haloferax volcanii, an archaeon, endogenous CRISPR-Cas system was used for gene repression through CRISPRi (Stachler and Marchfelder, 2016). In Clostridium tyrobutyricum, a Gram-positive bacterium, single and multi-gene deletions were successfully realized using the endogenous type I (subtype B) CRISPR-Cas system. In another study, an editing efficiency of 100% was achieved for the CRISPR-cas based gene deletions to develop strains for high-level butanol production (Zhang et al., 2018). A 100% efficiency for CRISPR-Cas based gene deletion was reported for a 643 bp deletion, and lower efficiencies for insertion and single nucleotide substitution (at 36 and 19% efficiency, respectively) performed in Lactobacillus crispatus, a Gram-positive commensal bacteria found in vagina and intestinal tracts (Hidalgo-Cantabrana et al., 2019). The researchers used the endogenous type I (subtype E) CRISPR-Cas system for the three gene editing approaches reported here. In E. coli, two studies reported the use of endogenous type I CRISPR-Cas system to modulate metabolic pathways via gene editing and CRISPRi, using Cas3 and dCas3 proteins, respectively (Chang et al., 2016; Tarasava et al., 2018). In S. aureus, type III (subtype A) CRISPR-Cas system has been used to achieve gene deletions and insertions (Guan et al., 2017). The above examples of successful use of endogenous CRISPR-Cas systems in archaea, Gram-positives and Gram-negatives highlights the possibilities of their use in other bacteria.
Endogenous CRISPR Systems in A. baumannii
Analysis of CRISPR database (CRISPRdb) showed two CRISPR-cas in Acinetobacter spp. The first system is discovered in the genome of clinical isolates A. baumannii AYE, AB0057, and AB037 (Grissa et al., 2007; Hauck et al., 2012). Another study demonstrated the presence of G55 islands in two of the above three strains AB0057 and AYE, and an additional strain 4,190 (Di Nocera et al., 2011). These G55 island contains the CRISPR blocks flanked by a cas gene cluster. The second of the CRISPR-cas system in Acinetobacter spp. was found in A. baumannii type strain ATCC® 19606T and in A. baylyi ADP1. The large volume of sequencing data and available bioinformatics tools have permitted the identification of CRISPRs in various A. baumannii strains (Grissa et al., 2009). The spacer organization in CRISPR-cas have been used for comparison between strains. A pangenome study encompassing 2,500 genomes of A. baumannii, agreed to the presence of two CRISPR-Cas systems (Mangas et al., 2019). By constructing a new phylogeny of Acinetobacter based on presence or absence of 14 common genes, they divided the genomes in to two groups. The first group, sharing fewer common genes, showed gene enrichment for maintaining CRISPR elements and rarity in the presence of plasmids. The authors in addition to confirming type 1 system showed the presence of type IV CRISPR-cas in A. baumannii as previously suggested. The relationship between CRISPR-cas and virulence genes have been discussed in a study (Louwen et al., 2014). With the success of endogenous CRISPR-Cas system including type I in genome editing in multiple bacteria strains, the same may be explored in A. baumannii. In addition, useful information would be obtained by better understanding of the relationship of CRISPR-Cas systems in A. baumannii with the virulence and pathogenesis.
Here we have summarized the use of both exogenous and recently explored endogenous CRISPR-cas system in gene manipulation. Although there are only a handful examples of using the CRISPR-Cas system in A. baumannii, there is certainly a tremendous potential for this technique to be used in this organism.
Complementation and Cloning Approaches
Several vectors are now available for cloning in A. baumannii (Supplementary Table 3). There are two common approaches for complementation that include chromosomal or extra-chromosomal. Extra-chromosomal cloning systems consists of plasmids, which have been mainstay of the cloning strategies historically. However, chromosomal insertion systems are increasingly becoming staple for cloning approaches, particularly since these systems allow expression of complemented genes at levels that mimic what is seen in nature and also because such systems do not require antibiotic selection facilitating in vivo studies.
Single Copy Insertion Systems
The most widely used mini-Tn7 system was originally developed for chromosomal insertions in P. aeruginosa (Choi and Schweizer, 2005). This system has been modified for use in A. baumannii (Figure 2B). With the help of exogenously supplied transposase, single copy Tn7 transposition occurs at a very specific attTn7 site within the genome. In A. baumannii this is located in the intergenic region 24 base pairs upstream of the glmS2 gene (Kumar et al., 2010). Based on its conservation across species, the resulting attTn7 site is presumed to be a neutral site within the chromosome (Choi and Schweizer, 2006; Choi et al., 2006, 2008; Kumar et al., 2006). There are a variety of iterations of this system including various selection markers, and induction parameters (Supplementary Table 3). Versatility of this system has been shown by its successful use in AB5075 (Pérez-Varela et al., 2020; Williams et al., 2020) as well in the clinical isolates AB030, AB031, and LAC-4 with the chromosomal insertion of constitutive fluorescent markers (Supplementary Table 4) including mTurquoise, RubyRed, mCherry, and sfGFP (Ducas-Mowchun et al., 2019), as well as complementation of deletion mutants in ATCC® 17978TM using the gentamicin selectable marker (De Silva et al., 2020). The antibiotic selection marker is removed via the Flp recombinase method described above (Figure 2C) using pFLP2 derivatives (Supplementary Table 2). Insertional complementation provides the benefit of maintaining single copy insertion of the gene of interest, allowing for expression at physiological levels and maintenance of complementation on selectable -free media reducing the risk of mutations via antibiotic stress. While Tn7-based systems have been used successfully in various strains of A. baumannii, it should be noted that A. baumannii strains may contain more than one copy of glmS (Kumar et al., 2010). The insertion of Tn7 element has always been shown to occur downstream of glmS2, but researchers should ideally confirm the insertion site when using this system with a new strain of A. baumannii. As with any transposition, immunity is also a concern using this system which prevents multiple cloning strategies in a single strain. Similar to the removal of the selectable marker during gene deletion, the FRT scar is left behind in the newly generated strain.
Alternatively, the use of endogenous site-specific recombination systems also show success in removal of antibiotic markers. The XerC/D tyrosine recombinases recognize adjacent dif sites within the newly replicated chromosome and aid in the separation of daughter chromosomes during replication. Many dif sites have been identified on plasmids within clinical isolates, flanking blaOXA–58 and contribute to the mobility of the gene (Cameranesi et al., 2018). This system has been modified for use in removal of antibiotic cassettes in reporter strains of clinical A. baumannii isolates (Supplementary Table 2). An autoluminescent strain was generated using the Tn7 system and removal of the cassette performed via the XerC/D recombineering strategy (Jiang et al., 2019). Removing the selectable marker through this approach allows for its removal while leaving no genetic scar.
Plasmid-Based Systems
Plasmid-based systems continue to be utilized by various groups, primarily due to the ease in their use. A number of plasmids used in A. baumannii are described in the review by Biswas (2015). However, since then, some useful plasmids have been designed for use in A. baumannii. We highlight the evolution of the newest systems below.
pVRL System
Extra-chromosomal plasmid-based complementation techniques have also been fine-tuned for A. baumannii. Provide complementation of gnaA in the MDR clinical isolate MDR-ZJ06 (Zhou et al., 2011), using a pET28a based E. coli shuttle vector pYMAb2 (Supplementary Table 3), that was modified to allow for hygromycin selection. Restoration of the phenotype upon complementation was observed except during the virulence assay with the wax worm model, Galleria mellonella as maintenance of the plasmid vector in vivo can be unreliable Xu Q. et al. (2019). Thus, underscoring the utility of genome insertion systems for in vivo experiments. Nevertheless, the pVRL system has been shown to function in A. baumannii as well as in A. baylyi (Lucidi et al., 2018). There are a variety of shuttle vectors in this family. pVRL1 and pVRL2 provide blue/white selection abilities for selection in E. coli as well as a gentamicin cassette for selection in A. baumannii (Lucidi et al., 2018).
pLVP System
A useful set of high copy plasmids were developed by Lucidi et al. (2019) that include reporter systems compatible in the clinical strains ACICU and AYE. Using the uvr-dependent DNA repair pathway as a proof of concept, activation of its promoter was evaluated in ATCC® 19606T by cloning the promoter into pLVP1Z, pLVP2Z, and pLVP3Z (Supplementary Table 4). Luminescence, β-galactosidase activity, and fluorescence were detected from all promoter fusions during exposure to UV, demonstrating the successful generation of a reporter strain in ATCC® 19606T. This system was further validated with the use of the ethanol inducible promoters in ATCC® 17978TM, ATCC® 19606T and AYE using pLVP1Z and the promoter regions of the ethanol dehydrogenase encoding adhP and the aldehyde dehydrogenase yahK. Again, the lux promoter fusion produced luminescence in a dose dependent manner when exposed to ethanol (Lucidi et al., 2019). In a final evaluation, iron regulation involving the Fur-Fe repressor complex and the basA promoter was evaluated in ATCC® 19606T using all three vectors. Reporter strains were exposed to increasing concentrations of FeCl3 in minimal media and a decrease in luminescence, β-galactosidase activity and fluorescence were observed. With the use of pLVP1Z::PbasA, under iron limiting conditions, Lucidi et al. demonstrated that in the animal model, G. mellonella, luminescence was detected and decreased over time as iron was scavenged from the host. This highlights the utility of these vectors for in vivo experiments. As with other extra-chromosomal cloning systems, these vectors must be selected for, which poses challenges as antibiotics modify cellular functions. The pLVP system, however, has been shown to be stable over 40 generations without an antibiotic selection and therefore is still of great value (Lucidi et al., 2019).
Selection Markers for Clinical Isolates
Non-clinical Antibiotic Markers
One major obstacle when working with clinical isolates of A. baumannii is that most, if not all are MDR or even XDR. This presents challenges when selecting for genetically manipulated mutants of interest as historically, those mutants are selected by an antibiotic resistance marker that differentiates them from wild-type cells. For this approach to work, high concentrations of many commonly used antibiotics are used that may introduce additional mutations. Alternatively, for manipulations of clinical isolates, non-clinical antibiotics and toxic compounds are used such as hygromycin, zeocin, apramycin, and tellurite. Novel vectors must be developed with these less common selection markers to increase the success of studies performed in MDR/XDR isolates.
Both hygromycin and apramycin are aminoglycoside antibiotics whose use is restricted to veterinary medicine making it appealing for application in A. baumannii isolates from clinical sources (Kotra et al., 2000). Hygromycin-specific resistance is encoded by a single gene, hph (Gritz and Davies, 1983) highlighting the ease of use in cloning into new vectors. This marker has been used for transposon mutant selection (Jacobs et al., 2014; Gallagher et al., 2015) and complementation experiments (Pérez-Varela et al., 2020) in AB5075. It has also been implemented successfully in AbCAN2 (Lopez et al., 2020), as a selection marker for the Flp recombinase expressing vector (Table 1).
Alternatively, apramycin resistance is conferred by 3-N-acetyltransferase type IV gene, aac(3)-IVa (Paget and Davies, 1996) that also provides a wide range of resistance to other aminoglycosides (Table 1). Successful genetic manipulations include complementation with the mini-Tn7 based integration system in XDR isolates (Ducas-Mowchun et al., 2019), and AB5075 (Pérez-Varela et al., 2020) as well as the CRISPR gene editing maintenance of the Cas expressing vector in clinical isolates ABH6 and XH386, and type-strains ATCC® 17978TM and ATCC® 19606T (Wang Y. et al., 2019). Zeocin is an antibiotic that causes cell damage by intercalation into dsDNA causing lethal double-stranded breaks (Gatignol et al., 1988) and is not in clinical use. Therefore, most clinical isolates of A. baumannii are susceptible to zeocin. Utility of zeocin as a selection marker has been shown in a number of clinical isolates from various countries (Luna et al., 2017; Ducas-Mowchun et al., 2019; Lucidi et al., 2019; Table 1).
Non-antibiotic Markers
Non-antibiotic markers are beneficial because resistance is less readily acquired as these are typically absent from mobile genetic elements. Amin et al. (2013) and Luna et al. (2017) made use of the tellurite resistance cassette and the xylE reporter gene to select for gene deletions in MDR isolates DB, R2, and HUMC1, respectively (Supplementary Table 2). Tellurite oxides are thought to cause damage to cells via oxidative stress and therefore exhibit activity in a wide range of bacterial species and cells expressing TelR-marker also show black pigmentation in Tel-supplemented medium which can further aid in the selection (Sanchez-Romero et al., 1998). The pABBR-TelR (Supplementary Table 3) construct was modified for use in clinical isolates to study the role of alleles of pmrAB in clinical colistin heteroresistance (Charretier et al., 2018). In conjunction with the TelR gene, tpm (Trebosc et al., 2016), or the kilA-telAB operon (Amin et al., 2013), the xylE reporter gene can be used to visualize color change in a colony to signify those with successful first cross-over events. The xylE gene product is a dioxygenase that hydrolyzes pyrocatechol to produce a yellow color, visible unaided, allowing for simple segregation of desired mutants (Ingram et al., 1989). Gene deletion in clinical isolates from Switzerland, United States, Turkey, Greece, Mexico, Spain and China using these selection markers was successful (Trebosc et al., 2016, 2019).
Counter-Selection Markers for Clinical Isolates
In an ideal situation, genetic modifications would be free of selection markers used during strain generation, ensuring no interference in downstream assays. In order to remove plasmids during genetic manipulations, a counter-selection marker allows for curing of the vector. Stringency of conditions for counter-selection is challenging and, in many cases, must be optimized on a strain by strain basis (Khetrapal et al., 2015). There are only two common counter-selection systems (sacB and tdk) currently in use for widespread applications in A. baumannii. These are methods based on lethality in the presence of high amounts of sucrose or 3′-azido-3′-deoxythymidine (AZT). The limited number of counter-selection markers currently available for use in A. baumannii necessitates that more options are needed particularly since the efficiency of existing markers can be quite variable from strain to strain.
Counter-Selection With sacB and tdk
In the case of sucrose counter-selection (Table 2), a vector is engineered with the sacB gene, originally isolated from B. subtilis, which encodes a levanosucrase. This enzyme performs the transfructorylation reaction of sucrose into levan in high sucrose environments and the buildup of this metabolite is toxic to many Gram-negative organisms including A. baumannii (Reyrat et al., 1998). This counter-selection marker has been used mainly to select for the second recombination event during homologous recombination-type strategies (Amin et al., 2013; Oh et al., 2015; De Silva et al., 2020) or to remove the Flp recombinase expressing vector (Ducas-Mowchun et al., 2019; De Silva et al., 2020) in type strains but also with some success in clinical isolates such as AB030 and AB031. sacB is by far the most commonly used counter-selection marker. It has recently been shown useful in curing of CRISPR based plasmids after gene editing in ABH6 and XH386 (Wang Y. et al., 2019). Efficiency of this system can vary from strain to strain and thus standardizing the growth conditions may be required. These include optimizing the sucrose concentration, incubation temperature, and/or the growth medium.
However, it has been observed that sacB can result in toxicity and thus select for mutations in sacB resulting in the maintenance of the vector in cells under counter-selection conditions (Trebosc et al., 2016). As a result, tdk and its cognate small molecule inhibitor AZT (Table 2) has been used as an alternate counterselection marker (Trebosc et al., 2016). In E. coli, the gene tdk encodes a thymidine kinase which is a key enzyme in the phosphorylation of thymine during nucleotide synthesis. With the introduction of the thymidine analog AZT, Tdk incorporates this dideoxynucleotide during DNA replication forcing chain termination, a lethal event. This counter-selection marker has been shown be quite effective and does not appear to select for mutations in tdk (Trebosc et al., 2016, 2019).
Counter-Selection With lpxC
Lee et al. (2018), introduce a variation on the previous counter-selection practices. It is known that the mechanism for colistin resistance results from loss of lipid A (Moffatt et al., 2010), modification of LOS (Beceiro et al., 2011) or mutations in the two-component system pmrAB (Charretier et al., 2018). Linking the gain of lipid A with restored colistin susceptibility, lpxC was cloned into a suicide vector (Supplementary Table 2). Generating a ΔlpxC mutant strain allowed for loss of colistin susceptibility and therefore a method for counter-selection. In order to maintain survival on colistin the mutant strain cures the vector after homologous recombination occurs with the gene of interest. However, the major limitation of the method is that it can only function in the ΔlpxC background, limiting the widespread application of this marker. Colistin, being the last resort antibiotic for A. baumannii infections, is also not an ideal antibiotic to be used for selection in laboratory conditions. Although creative, this method may not be of much use for manipulating clinical isolates of A. baumannii.
Other Common Counter-Selection Systems
Since there are only a limited number of counter-selection markers that have been used in A. baumannii, it is worthwhile investigating other options. A variety of counter-selection markers have been engineered for use in other bacterial species, described below in brief. Some of these counter-selection markers may be useful in A. baumannii.
rpsL and tetAR
rpsL encodes the ribosomal subunit S12, which is the target of the antibiotic streptomycin. Presence of this gene increases the susceptibility of those cells to streptomycin and selective pressure ensure loss of the vector containing rpsL and success has been seen in E. coli, Bortedella pertussis, Mycobacterium smegmatis (Reyrat et al., 1998) and recently in Corynebacterium glutamicium (Wang T. et al., 2019) and Borrelia burgdorferi (Drecktrah et al., 2010). Constant use of this marker selects for streptomycin resistant mutants which if used as a selection marker would be a detriment but further, its use in A. baumannii is plausible as clinical strains tend to be intrinsically resistant to streptomycin (Magnet et al., 2001). Similarly, the use of tetAR, confers resistance to tetracycline but vulnerability to fusaric acid. However, these methods can unfortunately select for tetracycline resistant mutants (Reyrat et al., 1998). With the MDR phenotypes of clinical isolates, antibiotic based counter-selection markers present challenges in manipulations of clinically relevant A. baumannii.
mazF
Recently, the toxin component of a toxin-anti-toxin system from E. coli was used as counter-selection in the Lactic Acid Bacteria (LAB), Lactobacillus plantarum and Enterococcus mundtii (Van Zyl et al., 2019). Expression of mazF results in cell death by the cleavage of mRNA at ACA sites. Cloned with the nisin inducible promoter, this counter-selectable marker is under tight regulatory control to prevent leaky expression and undesired lethality. Genetic manipulation using mazF has been demonstrated in B. subtilis (Zhang et al., 2006), Clostridia spp. (Joseph et al., 2018), and the yeast Pichia pastoris (Yang et al., 2009). Since the nisin inducible promoter’s efficacy in Gram-negatives has also yet to be determined, the utility of this system in A. baumannii remains to be seen.
pheS Variant
In Gram-positives, a common counter-selection marker is pheS∗. Encoding for a variant of the subunit of the phenylalanine tRNA transferase, this allows for the loading of para-chlorophenylalanine (PCPA) instead of only phenylalanine onto the tRNAPhe (Ibba et al., 1994). In the presence of PCPA, this results in misfolding of proteins and eventually cell death. Successful counter-selection has been achieved in Gram-positives such as Streptococcus mutans (Xie et al., 2011) and Enterococcus faecalis (Van Zyl et al., 2019), more recently in Staphylococcus aureus (Schuster et al., 2019) and Gram-negative organisms such as Burkholderia pseudomallei (Barrett et al., 2008). Currently pheS∗ has not been manipulated for use in A. baumannii nor P. aeruginosa but considering its success in B. pseudomallei, could be an addition to the arsenal of counter-selection markers for Acinetobacter spp. A “super lethal” PheS in E. coli has been established (Miyazaki, 2015) which may also lend itself for use in A. baumannii. However, the utility of PheS in A. baumannii remains to be explored.
Discussion
Considering the evolution of A. baumannii as a major Gram-negative pathogen of concern in hospitals around the world, it is critical that research stay current and cutting edge. To evaluate emerging clinical isolates, it is vital to develop and maintain methods for their genetic manipulation. Such methods allow for investigation into mechanisms of virulence and resistance, allowing for further improvements to current treatment options. As the majority of the work in A. baumannii has been carried out in the laboratory strains ATCC® 17978TM and ATCC® 19606T, applying this knowledge and these techniques to more recently isolated clinical strains is important. It has also been noted that these strains may not represent the characteristics that they once had or those strains in the current clinical environment. This is in part because they lack the MDR/XDR and highly virulent phenotypes common among current clinical isolates but also have evolved a distinct resistance profile from their origin (Harding et al., 2018). This has resulted in research groups using modern clinical strains as type-strains for their studies. One such example is AB5075 (Supplementary Table 1). It is a MDR strain, that was first isolated from the United States military health care system, and has been selected as a representative of clonal complex I as well for its increased virulence in a murine model (Jacobs et al., 2014). It is sensitive to hygromycin and tetracycline allowing for genetic manipulations with these antibiotic selectable markers and has been genetically modified extensively to study a multitude of mechanisms encompassing resistance, virulence and global regulation of cellular processes. In addition, this strain exhibits phase variation that has been attributed to virulence (Chin et al., 2018) and this phenomenon is being investigated in other isolates (Ahmad et al., 2019). Depending on the phase, phenotypic characteristics such as biofilm formation, antibiotic susceptibility, motility, and virulence differ suggesting that great care must be taken when working with this strain and others (Tipton et al., 2015).
In Europe, much work has been done on AYE (International Clone I) and ACICU (International Clone II, also known as European Clone II). AYE, a MDR strain endemic to France (Fournier et al., 2006) is susceptible to non-clinical antibiotics such as apramycin and has been manipulated successfully since its isolation in 2006 (Lucidi et al., 2018, 2019). Comparisons of deletions of adeRS and adeB have been made with strain AYE and S1 with differences in virulence, biofilm formation and antibiotic susceptibility (Richmond et al., 2016). The Italian isolate ACICU, also a MDR strain, has been studied extensively (Iacono et al., 2008) and has been used as a comparator for novel mutations in colistin resistance (Gerson et al., 2020) and as a reference for genomic assemblies (Yang-Yi Tan et al., 2013; Hamidian et al., 2019).
Additionally, AB030 and AB031 are clinical isolates from Canada representing highly virulent strains with contrasting resistance phenotypes. AB030 is highly virulent and XDR, whereas AB031 is highly virulent but less drug-resistant than AB030 (Fernando et al., 2013; Singh et al., 2020). Use of clinically relevant selection markers are critical in AB030 such as apramycin, hygromycin and zeocin whereas these are not required in AB031 as this strain is susceptible to classical markers such as gentamicin. This pair allow for the comparison of those systems that may contribute to resistance and virulence. AbCAN2 is also Canadian in origin and the T6SS has been heavily studied in this isolate (Di Venanzio et al., 2019; Lopez et al., 2020).
In A. baumannii research, it is imperative to be aware of distinctions between strains. Many comparisons have been performed on a wide selection of isolates revealing genomic and phenotypic differences in antibiotic resistance capabilities, virulence factors as well as clonal lineages (Fournier et al., 2006; Fernando et al., 2013; Casella et al., 2017; Cerezales et al., 2020; Liu et al., 2020; Singh et al., 2020). To aid in research efforts, the Multi-drug organism Repository and Surveillance Network (MRSN) has accumulated 3,500 A. baumannii isolates from around the world and have short-listed 100 strains that represent the genetic diversity and maximal phylogenetic distance (Galac et al., 2020). Significant effort must be dedicated to the study of the diverse nature of this pathogen. No matter the strain being studied, whether it be ATCC® 17978TM, ATCC® 19606T or a more recent isolate, these variations must be carefully considered when comparing results.
The advancement of techniques for use in clinical strains has been aided by the use of non-clinical and non-antibiotic selection markers, both in deletion and complementation methods. Recent progress with the introduction of an A. baumannii adapted CRISPR-Cas system to edit and delete genes holds great promise (Wang Y. et al., 2019). CRISPR provides high specificity and allows for base editing and gene deletion in clinical isolates. Although it is relatively new and has only been demonstrated in two strains of different ST’s, it is a promising tool whose use can be expanded to include many clinical isolates.
Challenges with counter-selection remain at bay to date but should be explored to enhance future genetic techniques. Sucrose and AZT remain the gold standards in clinical isolates, but toxicity associated with sacB has already been noted (Trebosc et al., 2016). Promising lethal gene-cognate small molecule pairs have been identified in other species such as pheS∗/PCPA and induction of mazF. Active investigation into these counter-selection markers allows for an increase in quantity of systems to choose from. It also provides options for use in novel A. baumannii strains.
There have been significant efforts made to advance the ability to genetically manipulate A. baumannii, specifically clinical isolates. These advancements significantly help in the understanding of the current disease conditions better and further the ability to develop treatment options for such infections.
Author Contributions
EMES, SD, and AK conceptualized and wrote the manuscript. All authors contributed to the article and approved the submitted version.
Funding
This work was funded by grants from the Natural Sciences and Engineering Council of Canada and University of Manitoba (2015-05550) to AK. EMES was a recipient of the University of Manitoba Graduate Fellowship Award. SD was funded by the fellowship from Mitacs, Canada.
Conflict of Interest
The authors declare that the research was conducted in the absence of any commercial or financial relationships that could be construed as a potential conflict of interest.
Acknowledgments
We thank Sydney McLaughlin for technical assistance and Rakesh Patidar and Vanessa Kornelsen for scientific and thought-provoking discussions.
Supplementary Material
The Supplementary Material for this article can be found online at: https://www.frontiersin.org/articles/10.3389/fgene.2020.601380/full#supplementary-material
References
Ahmad, I., Karah, N., Nadeem, A., Wai, S. N., and Uhlin, B. E. (2019). Analysis of colony phase variation switch in Acinetobacter baumannii clinical isolates. PLoS One 14:e0210082. doi: 10.1371/journal.pone.0210082
Alexeyev, M. F., and Shokolenko, I. N. (1995). Mini-Tn10 transposon derivatives for insertion mutagenesis and gene delivery into the chromosome of Gram-negative bacteria. Gene 160, 59–62. doi: 10.1016/0378-1119(95)00141-R
Amin, I., Richmond, G. E., Sen, P., Koh, T., Piddock, L. J., and Chua, K. (2013). A Method for Generating Marker-less Gene Deletions in Multidrug-resistant Acinetobacter baumannii. BMC Microbiol. 13:158. doi: 10.1186/1471-2180-13-158
Bae, S., Kweon, J., Kim, H. S., and Kim, J. S. (2014). Microhomology-based choice of Cas9 nuclease target sites. Nat. Methods 11, 705–706. doi: 10.1038/nmeth.3015
Barquist, L., Mayho, M., Cummins, C., Cain, A. K., Boinett, C. J., Page, A. J., et al. (2016). The TraDIS toolkit: sequencing and analysis for dense transposon mutant libraries. Bioinformatics 32, 1109–1111. doi: 10.1093/bioinformatics/btw022
Barrangou, R. (2015). The roles of CRISPR–Cas systems in adaptive immunity and beyond. Curr. Opin. Immunol. 32, 36–41. doi: 10.1016/j.coi.2014.12.008
Barrangou, R., Fremaux, C., Deveau, H., Richards, M., Boyaval, P., Moineau, S., et al. (2007). CRISPR provides acquired resistance against viruses in prokaryotes. Science 315, 1709–1712. doi: 10.1126/science.1138140
Barrett, A. R., Kang, Y., Inamasu, K. S., Son, M. S., Vukovich, J. M., and Hoang, T. T. (2008). Genetic tools for allelic replacement in Burkholderia species. Appl. Environ. Microbiol. 74, 4498–4508. doi: 10.1128/AEM.00531-08
Beceiro, A., Llobet, E., Aranda, J., Bengoechea, J. A., Doumith, M., Hornsey, M., et al. (2011). Phosphoethanolamine modification of lipid A in colistin-resistant variants of Acinetobacter baumannii mediated by the pmrAB two-component regulatory system. Antimicrob. Agents Chemother. 55, 3370–3379. doi: 10.1128/AAC.00079-11
Bender, J., and Klecknert, N. (1992). Tn10 insertion specificity is strongly dependent upon sequences immediately adjacent to the target-site consensus sequence. Proc. Natl. Acad. Sci. U.S.A. 89, 7996–8000. doi: 10.1073/pnas.89.17.7996
Bikard, D., Jiang, W., Samai, P., Hochschild, A., Zhang, F., and Marraffini, L. A. (2013). Programmable repression and activation of bacterial gene expression using an engineered CRISPR-Cas system. Nucleic Acids Res. 41, 7429–7437. doi: 10.1093/nar/gkt520
Biswas, I. (2015). Genetic tools for manipulating Acinetobacter baumannii genome: an overview. J. Med. Microbiol. 64, 657–669. doi: 10.1099/jmm.0.000081
Boinett, C. J., Cain, A. K., Hawkey, J., Hoang, N. T., Do, Khanh, N. N. T., Thanh, D. P., et al. (2019). Clinical and laboratory-induced colistin-resistance mechanisms in Acinetobacter baumannii. Microb. Genomics 5:e000246. doi: 10.1099/mgen.0.000246
Bolotin, A., Quinquis, B., Sorokin, A., and Ehrlich, S. D. (2005). Clustered regularly interspaced short palindrome repeats (CRISPRs) have spacers of extrachromosomal origin. Microbiology 151, 2551–2561. doi: 10.1099/mic.0.28048-0
Brouns, S. J. J., Jore, M. M., Lundgren, M., Westra, E. R., Slijkhuis, R. J. H., Snijders, A. P. L., et al. (2008). Small CRISPR RNAs Guide Antiviral Defense in Prokaryotes. Science 321, 960–964. doi: 10.1126/science.1159689
Cameranesi, M. M., Morán-Barrio, J., Limansky, A. S., Repizo, G. D., and Viale, A. M. (2018). Site-Specific Recombination at XerC/D Sites Mediates the Formation and Resolution of Plasmid Co-integrates Carrying a bla OXA-58-and TnaphA6-Resistance Module in Acinetobacter baumannii. Front. Microbiol. 9:66. doi: 10.3389/fmicb.2018.00066
Casella, L. G., Weiss, A., P Erez-Rueda, E., Ibarra, J. A., and Shaw, L. N. (2017). Towards the complete proteinaceous regulome of Acinetobacter baumannii. Microbiology 3:e000107. doi: 10.1099/mgen.0.000107
Cerezales, M., Xanthopoulou, K., Wille, J., Krut, O., Seifert, H., Gallego, L., et al. (2020). Mobile genetic elements harboring antibiotic resistance determinants in Acinetobacter baumannii isolates from Bolivia. Front. Microbiol. 11:919. doi: 10.3389/fmicb.2020.00919
Chang, Y., Su, T., Qi, Q., and Liang, Q. (2016). Easy regulation of metabolic flux in Escherichia coli using an endogenous type I-E CRISPR-Cas system. Microb. Cell Fact. 15:195. doi: 10.1186/s12934-016-0594-4
Chao, M. C., Abel, S., Davis, B. M., and Waldor, M. K. (2016). The design and analysis of transposon insertion sequencing experiments. Nat. Rev. Microbiol. 14, 119–128. doi: 10.1038/nrmicro.2015.7
Charretier, Y., Diene, S. M., Baud, D., Chatellier, S., Santiago-Allexant, E., Van Belkum, A., et al. (2018). Colistin Heteroresistance and Involvement of the PmrAB Regulatory System in Acinetobacter baumannii. Antimicrob. Agents Chemother. 62:AAC.00788-18.
Chen, W., Zhang, Y., Zhang, Y., Song, L., Wang, Y., and Ji, Q. (2018). CRISPR/Cas9-based genome editing in Pseudomonas aeruginosa and cytidine deaminase-mediated base editing in Pseudomonas Species. ISCIENCE 6, 222–231. doi: 10.1016/j.isci.2018.07.024
Chiang, S. L., and Rubin, E. J. (2002). Construction of a mariner-based transposon for epitope-tagging and genomic targeting. Gene 296, 179–185. doi: 10.1016/S0378-1119(02)00856-9
Chin, C. Y., Tipton, K. A., Farokhyfar, M., Burd, E. M., Weiss, D. S., and Rather, P. N. (2018). A High-frequency Phenotypic Switch Links Bacterial Virulence and Environmental Survival in Acinetobacter baumannii. Nat. Microbiol. 3, 563–569. doi: 10.1038/s41564-018-0151-5
Choi, K.-H., Deshazer, D., and Schweizer, H. P. (2006). Mini-Tn7 Insertion in Bacteria with Multiple glmS-linked attTn7 Sites: example Burkholderia mallei ATCC 23344. Nat. Protoc. 1, 162–269. doi: 10.1038/nprot.2006.25
Choi, K.-H., Mima, T., Casart, Y., Rholl, †D., Kumar, A., Beacham, I. R., et al. (2008). Genetic tools for select-agent-compliant manipulation of Burkholderia pseudomallei. Appl. Environ. Microbiol. 74, 1064–1075. doi: 10.1128/AEM.02430-07
Choi, K.-H., and Schweizer, H. P. (2005). An improved method for rapid generation of unmarked Pseudomonas aeruginosa deletion mutants. BMC Microbiol. 5:30. doi: 10.1186/1471-2180-5-30
Choi, K.-H., and Schweizer, H. P. (2006). Mini-Tn7 Insertion in Bacteria with Single attTn7 Sites: example Pseudomonas aeruginosa. Nat. Protoc. 1, 153–161. doi: 10.1038/nprot.2006.24
Chopin, M.-C., Chopin, A., and Bidnenko, E. (2005). Phage abortive infection in lactococci: variations on a theme. Curr. Opin. Microbiol. 8, 473–479. doi: 10.1016/j.mib.2005.06.006
Chuai, G., Wang, Q.-L., and Liu, Q. (2017). In Silico Meets In Vivo: towards Computational CRISPR-Based sgRNA Design. Trends Biotechnol. 35, 12–21. doi: 10.1016/j.tibtech.2016.06.008
Crépin, S., Ottosen, E. N., Peters, K., Smith, S. N., Himpsl, S. D., Vollmer, W., et al. (2018). The lytic transglycosylase MltB connects membrane homeostasis and in vivo fitness of Acinetobacter baumannii. Mol. Microbiol. 109, 745–762. doi: 10.1111/mmi.14000
De Silva, M. P., and Kumar, A. (2018). Effect of sodium chloride on surface-associated motility of Acinetobacter baumannii and the role of AdeRS two- component system. J. Membr. Biol. 251, 5–13. doi: 10.1007/s00232-017-9985-7
De Silva, P. M., Patidar, R., Graham, C. I., Karen, A., Brassinga, C., and Kumar, A. (2020). A response regulator protein with antar domain, AvnR, in Acinetobacter baumannii ATCC 17978 impacts its virulence and amino acid metabolism. Microbiology 166, 554–566. doi: 10.1099/mic.0.000913
Di Nocera, P., Rocco, F., Giannouli, M., Triassi, M., and Zarrilli, R. (2011). Genome organization of epidemic Acinetobacter baumannii strains. BMC Microbiol. 11:224. doi: 10.1186/1471-2180-11-224
Di Venanzio, G., Moon, K. H., Weber, B. S., Lopez, J., Ly, P. M., Potter, R. F., et al. (2019). Multidrug-resistant plasmids repress chromosomally encoded T6SS to enable their dissemination. Proc. Natl. Acad. Sci. U.S.A. 116, 1378–1383. doi: 10.1073/pnas.1812557116
Diancourt, L., Passet, V., Nemec, A., Dijkshoorn, L., and Brisse, S. (2010). The population structure of Acinetobacter baumannii: expanding multiresistant clones from an ancestral susceptible genetic pool. PLoS One 5:e10034. doi: 10.1371/journal.pone.0010034
Domingues, S., Rosário, N., Cândido, Â, Neto, D., Nielsen, K. M., and Da Silva, G. J. (2019). Competence for natural transformation is common among clinical strains of resistant acinetobacter spp. Microorganisms 7:30. doi: 10.3390/microorganisms7020030
Dong, C., Fontana, J., Patel, A., Carothers, J. M., and Zalatan, J. G. (2018). Synthetic CRISPR-Cas gene activators for transcriptional reprogramming in bacteria. Nat. Commun. 9, 1–11. doi: 10.1038/s41467-018-04901-6
Dove, S. L., and Hochschild, A. (1998). Conversion of the ω subunit of Escherichia coli RNA polymerase into a transcriptional activator or an activation target. Genes Dev. 12, 745–754. doi: 10.1101/gad.12.5.745
Drecktrah, D., Miles Douglas, J., and Scott Samuels, D. (2010). Use of rpsL as a counterselectable marker in Borrelia burgdorferi. Appl. Environ. Microbiol. 76, 985–987. doi: 10.1128/AEM.02172-09
Ducas-Mowchun, K., Malaka, P., Silva, D., Crisostomo, L., Fernando, D. M., Chao, T.-C., et al. (2019). Next generation of Tn7-based single-copy insertion elements for use in multi- and pan-drug-resistant strains of Acinetobacter baumannii. Appl. Environ. Microbiol. 85:e00066-19. doi: 10.1128/AEM.00066-19
Fernando, D., Zhanel, G., and Kumar, A. (2013). Antibiotic resistance and expression of resistance-nodulation-division pump- and outer membrane porin-encoding genes in Acinetobacter species isolated from Canadian Hospitals. Can. J. Infect. Dis. Med. Microbiol. 24, 17–21. doi: 10.1155/2013/696043
Fournier, P. E., Vallenet, D., Barbe, V., Audic, S., Ogata, H., Poirel, L., et al. (2006). Comparative genomics of multidrug resistance in Acinetobacter baumannii. PLoS Genet. 2:e20007. doi: 10.1371/journal.pgen.0020007
Fürste, J. P., Pansegrau, W., Frank, R., Blöcker, H., Scholz, P., Bagdasarian, M., et al. (1986). Molecular cloning of the plasmid RP4 primase region in a multi-host-range tacP expression vector. Gene 48, 119–131. doi: 10.1016/0378-1119(86)90358-6
Galac, M. R., Snesrud, E., LeBreton, F., Stam, J., Julius, M., Ong, A. C., et al. (2020). A diverse panel of clinical Acinetobacter baumannii for research and development. Antimicrob. Agents Chemother. 64:AAC.00840-20. doi: 10.1128/AAC.00840-20
Gallagher, L. A. (2019). “Methods for Tn-Seq analysis in Acinetobacter baumannii,” in Methods in Molecular Biology, Ed. J. M. Walker (Totowa, NJ: Humana Press Inc), 115–134. doi: 10.1007/978-1-4939-9118-1_12
Gallagher, L. A., Bailey, J., and Manoil, C. (2020). Ranking essential bacterial processes by speed of mutant death. Proc. Natl. Acad. Sci. U.S.A. 117, 18010–18017. doi: 10.1073/pnas.2001507117
Gallagher, L. A., Ramage, E., Weiss, E. J., Radey, M., Hayden, H. S., Held, K. G., et al. (2015). Resources for genetic and genomic analysis of emerging pathogen Acinetobacter baumannii. J. Bacteriol. 197, 2027–2035. doi: 10.1128/JB.00131-15
Gallagher, L. A., Shendure, J., and Manoil, C. (2011). Genome-Scale Identification of Resistance Functions in Pseudomonas aeruginosa Using Tn-seq. mBio 2:e00315-10. doi: 10.1128/mBio.00315-10
Garneau, J. E., Dupuis, M. -È, Villion, M., Romero, D. A., Barrangou, R., Boyaval, P., et al. (2010). The CRISPR/Cas bacterial immune system cleaves bacteriophage and plasmid DNA. Nature 468, 67–71. doi: 10.1038/nature09523
Gasiunas, G., Barrangou, R., Horvath, P., and Siksnys, V. (2012). Cas9-crRNA ribonucleoprotein complex mediates specific DNA cleavage for adaptive immunity in bacteria. Proc. Natl. Acad. Sci. U.S.A. 4, E2579–E2586. doi: 10.1073/pnas.1208507109
Gatignol, A., Durand, H., and Tiraby, G. (1988). Bleomycin resistance conferred by a drug-binding protein. FEBS Lett. 230, 171–175. doi: 10.1016/0014-5793(88)80665-3
Geisinger, E., Mortman, N. J., Dai, Y., Cokol, M., Syal, S., Farinha, A., et al. (2020). Antibiotic susceptibility signatures identify potential antimicrobial targets in the Acinetobacter baumannii cell envelope. Nat. Commun. 11:6107. doi: 10.1038/s41467-020-18301-2
Geisinger, E., Vargas-Cuebas, G., Mortman, N. J., Syal, S., Dai, Y., Wainwright, E. L., et al. (2019). The landscape of phenotypic and transcriptional responses to ciprofloxacin in Acinetobacter baumannii: acquired resistance alleles modulate drug-induced sos response and prophage replication. mBio 10:e01127-19. doi: 10.1128/mBio.01127-19
Gerson, S., Lucaßen, K., Wille, J., Nodari, C. S., Stefanik, D., Nowak, J., et al. (2020). Diversity of amino acid substitutions in PmrCAB associated with colistin resistance in clinical isolates of Acinetobacter baumannii. Int. J. Antimicrob. Agents 55:105862. doi: 10.1016/j.ijantimicag.2019.105862
Gibson, D. G., Young, L., Chuang, R. Y., Venter, J. C., Hutchison, C. A., and Smith, H. O. (2009). Enzymatic assembly of DNA molecules up to several hundred kilobases. Nat. Methods 6, 343–345. doi: 10.1038/nmeth.1318
Giles, S. K., Stroeher, U. H., Eijkelkamp, B. A., and Brown, M. H. (2015). Identification of genes essential for pellicle formation in Acinetobacter baumannii. BMC Microbiol. 15:116. doi: 10.1186/s12866-015-0440-6
Godeux, A.-S., Svedholm, E., Lupo, A., Haenni, M., Venner, S., Laaberki, M.-H., et al. (2020). Scarless removal of large resistance island AbaR results in antibiotic susceptibility and increased natural transformability in Acinetobacter baumannii. Antimicrob. Agents Chemother. 64:e00951-20. doi: 10.1128/aac.00951-20
Goldfarb, T., Sberro, H., Weinstock, E., Cohen, O., Doron, S., Charpak-Amikam, Y., et al. (2015). BREX is a novel phage resistance system widespread in microbial genomes. EMBO J. 34, 169–183. doi: 10.15252/embj.201489455
Goryshin, I. Y., Miller, J. A., Kil, Y. V., Lanzov, V. A., and Reznikoff, W. S. (1998). Tn5IS50 target recognition Escherichia coli composite transposons insertion specificity. Proc. Natl. Acad. Sci. U.S.A. 95, 10716–10721.
Graham, D. B., and Root, D. E. (2015). Resources for the design of CRISPR gene editing experiments. Genome Biol. 16, 1–21. doi: 10.1186/s13059-015-0823-x
Green, B., Bouchier, C., Fairhead, C., Craig, N. L., and Cormack, B. P. (2012). Insertion site preference of Mu, Tn5, and Tn7 transposons. Mob. DNA 3:3. doi: 10.1186/1759-8753-3-3
Grissa, I., Vergnaud, G., and Pourcel, C. (2007). The CRISPRdb database and tools to display CRISPRs and to generate dictionaries of spacers and repeats. BMC Bioinform. 8:172. doi: 10.1186/1471-2105-8-172
Grissa, I., Vergnaud, G., and Pourcel, C. (2009). Clustered regularly interspaced short palindromic repeats (CRISPRs) for the genotyping of bacterial pathogens. Methods Mol. Biol. 551, 105–116. doi: 10.1007/978-1-60327-999-4_9
Gritz, L., and Davies, J. (1983). Plasmid-encoded Hygromycin B resistance: the sequence of Hygromycin B Phosphotransferease gene and its Expression in Escherichia coli and Sacchromyces cerevisiae. Gene 25, 179–188. doi: 10.1016/0378-1119(83)90223-8
Groh, J. L., Luo, Q., Ballard, J. D., and Krumholz, L. R. (2005). A method adapting microarray technology for signature-tagged mutagenesis of Desulfovibrio desulfuricans G20 and Shewanella oneidensis MR-1 in anaerobic sediment survival experiments. Appl. Environ. Microbiol. 71, 7064–7074. doi: 10.1128/AEM.71.11.7064-7074.2005
Guan, J., Wang, W., and Sun, B. (2017). Chromosomal Targeting by the Type III-A CRISPR-Cas System Can Reshape Genomes in Staphylococcus aureus. mSphere 2:e00403-17. doi: 10.1128/msphere.00403-17
Hale, C. R., Zhao, P., Olson, S., Duff, M. O., Graveley, B. R., Wells, L., et al. (2009). RNA-Guided RNA cleavage by a CRISPR RNA-cas protein complex. Cell 139, 945–956. doi: 10.1016/j.cell.2009.07.040
Hamad, M. A., Zajdowicz, S. L., Holmes, R. K., and Voskuil, M. I. (2009). An Allelic exchange system for compliant genetic manipulation of the select agents Burkholderia pseudomallei and Burkholderia mallei. Gene 430, 123–131. doi: 10.1016/j.gene.2008.10.011
Hamidian, M., Wick, R. R., Hartstein, R. M., Judd, L. M., Holt, K. E., and Hall, R. M. (2019). Insights from the revised complete genome sequences of Acinetobacter baumannii strains AB307-0294 and ACICU belonging to global clones 1 and 2. Microb. Genomics 5:e000298. doi: 10.1099/mgen.0.000298
Harding, C. M., Hennon, S. W., and Feldman, M. F. (2018). Uncovering the mechanisms of Acinetobacter baumannii virulence. Nat. Rev. Microbiol. 16, 91–102. doi: 10.1038/nrmicro.2017.148
Hassan, K. A., Cain, A. K., Huang, T. T., Liu, Q., Elbourne, L. D. H., Boinett, C. J., et al. (2016). Fluorescence-based flow sorting in parallel with transposon insertion site sequencing identifies multidrug efflux systems in Acinetobacter baumannii. mBio 7:e01200-16. doi: 10.1128/mBio.01200-16
Hauck, Y., Soler, C., Jault, P., Mérens, A., Gérome, P., Nab, C. M., et al. (2012). Diversity of Acinetobacter baumannii in four french military hospitals, as assessed by multiple locus variable number of tandem repeats analysis. PLoS One 7:e44597. doi: 10.1371/journal.pone.0044597
Hendel, A., Fine, E. J., Bao, G., and Porteus, M. H. (2015). Quantifying on- and off-target genome editing. Trends Biotechnol. 33, 132–140. doi: 10.1016/j.tibtech.2014.12.001
Hidalgo-Cantabrana, C., Goh, Y. J., Pan, M., Sanozky-Dawes, R., and Barrangou, R. (2019). Genome editing using the endogenous type I CRISPR-Cas system in Lactobacillus crispatus. Proc. Natl. Acad. Sci. U.S.A. 116, 15774–15783. doi: 10.1073/pnas.1905421116
Hoang, T. T., Karkhoff-Schweizer, R. R., Kutchma, A. J., and Schweizer, H. P. (1998). A broad-host-range Flp-FRT recombination system for site-specific excision of chromosomally-located DNA sequences: application for isolation of unmarked Pseudomonas aeruginosa mutants. Gene 212, 77–86. doi: 10.1016/s0378-1119(98)00130-9
Hogan, A. M., Rahman, A. S. M. Z., Lightly, T. J., and Cardona, S. T. (2019). A broad-host-range CRISPRi toolkit for silencing gene expression in Burkholderia. ACS Synth. Biol. 8, 2372–2384. doi: 10.1021/acssynbio.9b00232
Hsu, P. D., Scott, D. A., Weinstein, J. A., Ran, F. A., Konermann, S., Agarwala, V., et al. (2013). DNA targeting specificity of RNA-guided Cas9 nucleases. Nat. Biotechnol. 31, 827–832. doi: 10.1038/nbt.2647
Iacono, M., Villa, L., Fortini, D., Bordoni, R., Imperi, F., Bonnal, R. J. P., et al. (2008). Whole-genome pyrosequencing of an epidemic multidrug-resistant Acinetobacter baumannii strain belonging to the European clone II group. Antimicrob. Agents Chemother. 52, 2616–2625. doi: 10.1128/AAC.01643-07
Ibba, M., Kast, P., and Hennecke, H. (1994). Substrate specificity is determined by amino acid binding pocket size in Escherichia coli Phenylalanyl-tRNA synthetase. Biochemistry 33, 7107–7112. doi: 10.1021/bi00189a013
Ingram, C., Brawner, M., Youngman, P., and Westphelingl, J. (1989). xylE Functions as an Efficient Reporter Gene in Streptomyces spp.: use for the Study of galPI, a Catabolite-Controlled Promoter. J. Bacteriol. 171, 6617–6624. doi: 10.1128/jb.171.12.6617-6624.1989
Jacobs, A. C., Thompson, M. G., Black, C. C., Kessler, J. L., Clark, L. P., McQueary, C. N., et al. (2014). AB5075, a Highly Virulent Isolate of Acinetobacter baumannii, as a model strain for the evaluation of pathogenesis and antimicrobial treatments. mBio 5:e01076-14. doi: 10.1128/mBio.01076-14
Jiang, H., Gao, Y., Zeng, S., Wang, S., Cao, Z., Tan, Y., et al. (2019). One-step engineering of a stable, selectable marker-free autoluminescent Acinetobacter baumannii for rapid continuous assessment of drug activity S. J. Microbiol. Biotechnol. 29, 1488–1493. doi: 10.4014/jmb.1905.05006
Jiang, W., Cox, D., Zhang, F., Bikard, D., and Marraffini, L. A. (2013). RNA-guided editing of bacterial genomes using CRISPR-Cas systems. Nat. Biotechnol. 31, 233–241. doi: 10.1038/nbt.2508
Jiang, Y., Chen, B., Duan, C., Sun, B., Yang, J., and Yang, S. (2015). Multigene editing in the Escherichia coli genome via the CRISPR-Cas9 system. Appl. Environ. Microbiol. 81, 2506–2514. doi: 10.1128/AEM.04023-14
Jinek, M., Chylinski, K., Fonfara, I., Hauer, M., Doudna, J. A., and Charpentier, E. (2012). A programmable Dual-RNA-Guided DNA endonuclease in adaptive bacterial immunity. Science 337, 816–821. doi: 10.1126/science.1225829
Jones, D. L., Leroy, P., Unoson, C., Fange, D., Ćurić, V., Lawson, M. J., et al. (2017). Kinetics of dCas9 target search in Escherichia coli. Science 357, 1420–1424. doi: 10.1126/science.aah7084
Joseph, R. C., Kim, N. M., and Sandoval, N. R. (2018). Recent developments of the synthetic biology toolkit for Clostridium. Front. Microbiol. 9:154. doi: 10.3389/fmicb.2018.00154
Karlapudi, A. P., Taa, C. V., Tammineedi, J., Srirama, K., Kanumuri, L., and Prabhakar Kodali, V. (2018). In silico sgRNA tool design for CRISPR control of quorum sensing in Acinetobacter species. Genes Dis. 5, 123–129. doi: 10.1016/j.gendis.2018.03.004
Kazi, M. I., Schargel, R. D., and Boll, J. M. (2020). Generating transposon insertion libraries in gram-negative bacteria for high-throughput sequencing. J. Vis. Exp. 2020, 1–25. doi: 10.3791/61612
Khetrapal, V., Mehershahi, K., Rafee, S., Chen, S., Lim, C. L., and Chen, S. L. (2015). A set of powerful negative selection systems for unmodified Enterobacteriaceae. Nucleic Acids Res. 43:e83. doi: 10.1093/nar/gkv248
Kim, S. Y., Kim, M. H., Kim, S. I., Son, J. H., Kim, S., Lee, Y. C., et al. (2019). The sensor kinase BfmS controls production of outer membrane vesicles in Acinetobacter baumannii. BMC Microbiol. 19:301. doi: 10.1186/s12866-019-1679-0
Klein, B. A., Tenorio, E. L., Lazinski, D. W., Camilli, A., Duncan, M. J., and Hu, L. T. (2012). Identification of essential genes of the periodontal pathogen Porphyromonas gingivalis. BMC Genomics 13:578. doi: 10.1186/1471-2164-13-578
Kochar, M., Crosatti, M., Harrison, E. M., Rieck, B., Chan, J., Constantinidou, C., et al. (2012). Deletion of TnAbaR23 results in both expected and unexpected antibiogram changes in a multidrug-resistant Acinetobacter baumannii strain. Antimicrob. Agents Chemother. 56, 1845–1853. doi: 10.1128/AAC.05334-11
Kotra, L. P., Haddad, J., and Mobashery, S. (2000). Aminoglycosides: perspectives on mechanisms of action and resistance and strategies to counter resistance. Antimicrob. Agents Chemother. 44, 3249–3256. doi: 10.1128/AAC.44.12.3249-3256.2000
Krasauskas, R., Skerniškytė, J., Armalytė, J., and Sužiedėlienė, E. (2019). The role of Acinetobacter baumannii response regulator BfmR in pellicle formation and competitiveness via contact-dependent inhibition system. BMC Microbiol. 19:241. doi: 10.1186/s12866-019-1621-5
Kumar, A., Chua, K. L., and Schweizer, H. P. (2006). Method for regulated expression of single-copy efflux pump genes in a surrogate Pseudomonas aeruginosa strain: identification of the BpeEF-OprC chloramphenicol and trimethoprim efflux pump of Burkholderia pseudomallei 1026b. Antimicrob. Agents Chemother. 50, 3460–3463. doi: 10.1128/AAC.00440-06
Kumar, A., Dalton, C., Cortez-Cordova, J., and Schweizer, H. P. (2010). Mini-Tn7 vectors as genetic tools for single copy gene cloning in Acinetobacter baumannii. J. Microbiol. Methods 82, 296–300. doi: 10.1016/j.mimet.2010.07.002
Langridge, G. C., Phan, M.-D., Turner, D. J., Perkins, T. T., Parts, L., Haase, J., et al. (2009). Simultaneous assay of every Salmonella Typhi gene using one million transposon mutants. Genome Res. 19, 2308–2316. doi: 10.1101/gr.097097.109
Lee, C. M., Cradick, T. J., Fine, E. J., and Bao, G. (2016). Nuclease target site selection for maximizing on-target activity and minimizing off-target effects in genome editing. Mol. Ther. 24, 475–487. doi: 10.1038/mt.2016.1
Lee, W., Do, T., Zhang, G., Kahne, D., Meredith, T. C., and Walker, S. (2018). Antibiotic combinations that enable one-step, targeted mutagenesis of chromosomal genes. ACS Infect. Dis. 4, 1007–1018. doi: 10.1021/acsinfecdis.8b00017
Li, X. T., Jun, Y., Erickstad, M. J., Brown, S. D., Parks, A., Court, D. L., et al. (2016). TCRISPRi: tunable and reversible, one-step control of gene expression. Sci. Rep. 6, 1–12. doi: 10.1038/srep39076
Liu, L., Shen, P., Zheng, B., Yu, W., Ji, J., and Xiao, Y. (2020). Comparative genomic analysis of 19 clinical isolates of Tigecycline-Resistant Acinetobacter baumannii. Front. Microbiol. 11:1321. doi: 10.3389/fmicb.2020.01321
Lopez, J., Ly, P. M., and Feldman, M. (2020). The Tip of the VgrG spike is essential to functional type VI secretion system assembly in Acinetobacter baumanni. mBio 11:e02761-19. doi: 10.1128/mBio.02761-19
Louwen, R., Staals, R. H. J., Endtz, H. P., van Baarlen, P., and van der Oost, J. (2014). The Role of CRISPR-cas systems in virulence of pathogenic bacteria. Microbiol. Mol. Biol. Rev. 78, 74–88. doi: 10.1128/mmbr.00039-13
Lucidi, M., Runci, F., Rampioni, G., Frangipani, E., Leoni, L., and Visca, P. (2018). New shuttle vectors for gene cloning and expression in multidrug-resistant Acinetobacter species. Antimicrob. Agents Chemother. 62:e02480-17. doi: 10.1128/AAC.02480-17
Lucidi, M., Visaggio, D., Prencipe, E., Imperi, F., Rampioni, G., Cincotti, G., et al. (2019). New shuttle vectors for real-time gene expression analysis in multidrug-resistant Acinetobacter species: in vitro and in vivo responses to environmental stressors. Appl. Environ. Microbiol. 85, 1334–1353. doi: 10.1128/AEM.01334-19
Luna, B., Trebosc, V., Lee, B., Bakowski, M., Ulhaq, A., Yan, J., et al. (2020). A nutrient-limited screen unmasks rifabutin hyperactivity for extensively drug-resistant Acinetobacter baumannii. Nat. Microbiol. 5, 1134–1143. doi: 10.1038/s41564-020-0737-6
Luna, B. M., Ulhaq, A., Yan, J., Pantapalangkoor, P., Nielsen, T. B., Davies, B. W., et al. (2017). Selectable Markers for Use in Genetic Manipulation of Extensively Drug- Resistant (XDR) Acinetobacter baumannii HUMC1. mSphere 2:e00140-17. doi: 10.1128/mSphere.00140-17
Magnet, S., Courvalin, P., and Lambert, T. (2001). Resistance-Nodulation-Cell division-type efflux pump involved in aminoglycoside resistance in Acinetobacter baumannii strain BM4454. Antimicrob. Agents Chemother. 45, 3375–3380. doi: 10.1128/AAC.45.12.3375-3380.2001
Makarova, K. S., and Koonin, E. V. (2015). Annotation and classification of CRISPR-Cas systems. Methods Mol. Biol. 1311, 47–75. doi: 10.1007/978-1-4939-2687-9_4
Makarova, K. S., Wolf, Y. I., and Koonin, E. V. (2013). Comparative genomics of defense systems in archaea and bacteria. Nucleic Acids Res. 41, 4360–4377. doi: 10.1093/nar/gkt157
Makarova, K. S., Wolf, Y. I., Snir, S., and Koonin, E. V. (2011). Defense islands in bacterial and archaeal genomes and prediction of novel defense systems. J. Bacteriol. 193, 6039–6056. doi: 10.1128/JB.05535-11
Mangas, E. L., Rubio, A., Álvarez-Marín, R., Labrador-Herrera, G., Pachón, J., Pachón-Ibáñez, M. E., et al. (2019). Pangenome of Acinetobacter baumannii uncovers two groups of genomes, one of them with genes involved in CRISPR/Cas defence systems associated with the absence of plasmids and exclusive genes for biofilm formation. Microb. Genomics. 5:e000309. doi: 10.1099/mgen.0.000309
Marraffini, L. A., and Sontheimer, E. J. (2008). CRISPR interference limits horizontal gene transfer in Staphylococci by Targeting DNA. Science 322, 1843–1845. doi: 10.1126/science.1165771
Miyazaki, K. (2015). Molecular engineering of a PheS counterselection marker for improved operating efficiency in Escherichia coli. Biotechniques 58, 86–88. doi: 10.2144/000114257
Moffatt, J. H., Harper, M., Harrison, P., Hale, J. D. F., Vinogradov, E., Seemann, T., et al. (2010). Colistin resistance in Acinetobacter baumannii is mediated by complete loss of Lipopolysaccharide production. Antimicrob. Agents Chemother. 54, 4971–4977. doi: 10.1128/AAC.00834-10
Mojica, F. J. M., Diez-Villaseior, C., Garcia-Martinez, J., and Soria, E. (2005). Intervening sequences of regularly spaced prokaryotic repeats derive from foreign genetic elements. J. Mol. Evol. 60, 174–182. doi: 10.1007/s00239-004-0046-3
Oh, M. H., Lee, J. C., Kim, J., Choi, C. H., and Han, K. (2015). Simple method for markerless gene deletion in multidrug-resistant Acinetobacter baumannii. Appl. Environ. Microbiol. 81, 3357–3368. doi: 10.1128/AEM.03975-14
Paget, E., and Davies, J. (1996). Apramycin resistance as a selective marker for gene transfer in Mycobacteria. J. Bacteriol. 178, 6357–6360. doi: 10.1128/jb.178.21.6357-6360.1996
Pérez-Varela, M., Tierney, A. R. P., Kim, J.-S., Vázquez-Torres, A., and Rather, P. (2020). Characterization of RelA in Acinetobacter baumannii. J. Bacteriol. 202:e00045-20. doi: 10.1128/JB.00045-20
Peters, J. M., Colavin, A., Shi, H., Czarny, T. L., Larson, M. H., Wong, S., et al. (2016). A comprehensive, CRISPR-based functional analysis of essential genes in bacteria. Cell 165, 1493–1506. doi: 10.1016/j.cell.2016.05.003
Qi, L., and Lo, A. (2017). Genetic and epigenetic control of gene expression by CRISPR-Cas systems. F1000Research 6:F1000 Faculty Rev-747. doi: 10.12688/f1000research.11113.1
Qi, L. S., Larson, M. H., Gilbert, L. A., Doudna, J. A., Weissman, J. S., Arkin, A. P., et al. (2013). Repurposing CRISPR as an RNA-γuided platform for sequence-specific control of gene expression. Cell 152, 1173–1183. doi: 10.1016/j.cell.2013.02.022
Redding, S., Sternberg, S. H., Marshall, M., Gibb, B., Bhat, P., Guegler, C. K., et al. (2015). Surveillance and processing of foreign DNA by the Escherichia coli CRISPR-Cas system. Cell 163, 854–865. doi: 10.1016/j.cell.2015.10.003
Reyrat, J.-M., Pelicic, V., Gicquel, B., and Rappuoli, R. (1998). Counterselectable markers: untapped tools for bacterial genetics and pathogenesis. Infect. Immun. 66, 4011–4017. doi: 10.1128/.66.9.4011-4017.1998
Richmond, G. E., Evans, L. P., Anderson, M. J., Wand, M. E., Bonney, L. C., Ivens, A., et al. (2016). The Acinetobacter baumannii two-component system AdeRS regulates genes required for multidrug efflux, biofilm formation, and virulence in a strain-specific manner. mBio 7:e00430-16. doi: 10.1128/mBio.00430-16
Roy, R., You, R.-I., Lin, M.-D., and Lin, N.-T. (2020). Mutation of the Carboxy-Terminal processing protease in Acinetobacter baumannii affects motility, leads to loss of membrane integrity, and reduces virulence. Pathogens 9:322. doi: 10.3390/pathogens9050322
Russo, T. A., Manohar, A., Beanan, J. M., Olson, R., Macdonald, U., Graham, J., et al. (2016). The response regulator BfmR is a potential drug target for Acinetobacter baumannii. mSphere 1:e00082-16. doi: 10.1128/mSphere.00082-16
Rutkauskas, M., Sinkunas, T., Songailiene, I., Tikhomirova, M. S., Siksnys, V., and Seidel, R. (2015). Directional R-Loop Formation by the CRISPR-Cas Surveillance Complex Cascade Provides Efficient Off-Target Site Rejection. Cell Rep. 10, 1534–1543. doi: 10.1016/j.celrep.2015.01.067
Samson, J. E., Magadán, A. H., Sabri, M., and Moineau, S. (2013). Revenge of the phages: defeating bacterial defences. Nat. Rev. Microbiol. 11, 675–687. doi: 10.1038/nrmicro3096
Sanchez-Romero, J. M., Diaz-Orejas, R., and De Lorenzo, V. (1998). Resistance to tellurite as a selection marker for genetic manipulations of Pseudomonas strains. Appl. Environ. Microbiol. 64, 4040–4046. doi: 10.1128/aem.64.10.4040-4046.1998
Scaletsky, I. C. A., Michalski, J., Torres, A. G., Dulguer, M. V., and Kaper, J. B. (2005). Identification and characterization of the locus for diffuse adherence, which encodes a novel afimbrial adhesin found in atypical enteropathogenic Escherichia coli. Infect. Immun. 73, 4753–4765. doi: 10.1128/IAI.73.8.4753-4765.2005
Schuster, C. F., Howard, S. A., and Gründling, A. (2019). Use of the counter selectable marker PheS∗ for genome engineering in Staphylococcus aureus. Microbiology 165, 572–584. doi: 10.1099/mic.0.000791
Singh, M., De Silva, P. M., Al-Saadi, Y., Switala, J., Loewen, P. C., Hausner, G., et al. (2020). Antibiotics Characterization of Extremely Drug-Resistant and Hypervirulent Acinetobacter baumannii AB030. Antibiot. 9:328. doi: 10.3390/antibiotics9060328
Skerniškytė, J., Karazijaitė, E., Deschamps, J., Krasauskas, R., Armalytė, J., Briandet, R., et al. (2019). Blp1 protein shows virulence-associated features and elicits protective immunity to Acinetobacter baumannii infection. BMC Microbiol. 19:259. doi: 10.1186/s12866-019-1615-3
Stachler, A. E., and Marchfelder, A. (2016). Gene repression in haloarchaea using the CRISPR (Clustered regularly interspaced short palindromic repeats)-Cas I-B system. J. Biol. Chem. 291, 15226–15242. doi: 10.1074/jbc.M116.724062
Sternberg, S. H., Redding, S., Jinek, M., Greene, E. C., and Doudna, J. A. (2014). DNA interrogation by the CRISPR RNA-guided endonuclease Cas9. Nature 507, 62–67. doi: 10.1038/nature13011
Subashchandrabose, S., Smith, S., DeOrnellas, V., Crepin, S., Kole, M., Zahdeh, C., et al. (2015). Acinetobacter baumannii Genes Required for Bacterial Survival during Bloodstream Infection. mSphere 1:e00013-15. doi: 10.1128/msphere.00013-15
Sun, B., Liu, H., Jiang, Y., Shao, L., Yang, S., and Chen, D. (2020). New Mutations Involved in Colistin Resistance in Acinetobacter baumannii. mSphere 5:e00895-19. doi: 10.1128/msphere.00895-19
Swarts, D. C., Jore, M. M., Westra, E. R., Zhu, Y., Janssen, J. H., Snijders, A. P., et al. (2014). DNA-guided DNA interference by a prokaryotic Argonaute. Nature 507, 258–261. doi: 10.1038/nature12971
Szczelkun, M. D., Tikhomirova, M. S., Sinkunas, T., Gasiunas, G., Karvelis, T., Pschera, P., et al. (2014). Direct observation of R-loop formation by single RNA-guided Cas9 and Cascade effector complexes. Proc. Natl. Acad. Sci. U.S.A. 111, 9798–9803. doi: 10.1073/pnas.1402597111
Tacconelli, E., Carrara, E., Savoldi, A., Harbarth, S., Mendelson, M., Monnet, D. L., et al. (2018). Discovery, research, and development of new antibiotics: the WHO priority list of antibiotic-resistant bacteria and tuberculosis. Lancet Infect. Dis. 18, 318–327. doi: 10.1016/S1473-3099(17)30753-3
Tarasava, K., Liu, R., Garst, A., and Gill, R. T. (2018). Combinatorial pathway engineering using type I-E CRISPR interference. Biotechnol. Bioeng. 115, 1878–1883. doi: 10.1002/bit.26589
Terns, M. P., and Terns, R. M. (2011). CRISPR-based adaptive immune systems. Curr. Opin. Microbiol. 14, 321–327. doi: 10.1016/j.mib.2011.03.005
Tipton, K. A., Dimitrova, D., and Rather, P. N. (2015). Phase-Variable Control of Multiple Phenotypes in Acinetobacter baumannii Strain AB5075. J. Bacteriol. 197, 2593–2599. doi: 10.1128/JB.00188-15
Tipton, K. A., Farokhyfar, M., and Rather, P. N. (2017). Multiple roles for a novel RND-type efflux system in Acinetobacter baumannii AB5075. Microbiologyopen 6:e00418. doi: 10.1002/mbo3.418
Tock, M. R., and Dryden, D. T. (2005). The biology of restriction and anti-restriction. Curr. Opin. Microbiol. 8, 466–472. doi: 10.1016/j.mib.2005.06.003
Trebosc, V., Gartenmann, S., Royet, K., Manfredi, P., Tötzl, M., Schellhorn, B., et al. (2016). A novel genome-editing platform for drug-resistant Acinetobacter baumannii reveals an AdeR-unrelated tigecycline resistance mechanism. Antimicrob. Agents Chemother. 60, 7263–7271. doi: 10.1128/AAC.01275-16
Trebosc, V., Gartenmann, S., Tötzl, M., Lucchini, V., Schellhorn, B., Pieren, M., et al. (2019). Dissecting colistin resistance mechanisms in extensively drug-resistant Acinetobacter baumannii clinical isolates. mBio 10:e01083-19.
Tucker, A. T., Nowicki, E. M., Boll, J. M., Knauf, G. A., Burdis, N. C., Trent, M. S., et al. (2014). Defining gene-phenotype relationships in Acinetobacter baumannii through one-step chromosomal gene inactivation. mBio 5:e01313–1327. doi: 10.1128/mBio.01313-14
Van Opijnen, T., and Camilli, A. (2013). Transposon insertion sequencing: a new tool for systems-level analysis of microorganisms. Nat. Rev. Microbiol. 11, 435–442. doi: 10.1038/nrmicro3033
Van Zyl, W. F., Dicks, L. M. T., and Deane, S. M. (2019). Development of a novel selection/counter-selection system for chromosomal gene integrations and deletions in lactic acid bacteria. BMC Mol. Biol. 20:10. doi: 10.1186/s12867-019-0127-x
Wang, N., Ozer, E. A., Mandel, M. J., and Hauser, A. R. (2014). Genome-wide identification of Acinetobacter baumannii genes necessary for persistence in the lung. mBio 5:e01163–1177. doi: 10.1128/mBio.01163-14
Wang, T., Li, Y., Li, J., Zhang, D., Cai, N., Zhao, G., et al. (2019). An update of the suicide plasmid-mediated genome editing system in Corynebacterium glutamicum. Microb. Biotechnol. 12, 907–919. doi: 10.1111/1751-7915.13444
Wang, Y., Wang, Z., Chen, Y., Hua, X., Yu, Y., and Correspondence, J. (2019). A Highly Efficient CRISPR-Cas9-based genome engineering platform in Acinetobacter baumannii to understand the H 2 O 2-Sensing Mechanism of OxyR. Cell Chem. Biol. 26, 1732–1742. doi: 10.1016/j.chembiol.2019.09.003
Wang, Y., Wang, S., Chen, W., Song, L., Zhang, Y., Shen, Z., et al. (2018). CRISPRCas9 and CRISPR-assisted cytidine deaminase enable precise and efficient genome editing in Klebsiella pneumoniae. Appl. Environ. Microbiol. 84, 1834–1852. doi: 10.1128/AEM.01834-18
Williams, C. L., Neu, H. M., Alamneh, Y. A., Reddinger, R. M., Jacobs, A. C., Singh, S., et al. (2020). Characterization of Acinetobacter baumannii copper resistance reveals a role in virulence. Front. Microbiol. 11:16. doi: 10.3389/fmicb.2020.00016
Xie, Z., Okinaga, T., Qi, F., Zhang, Z., and Merritt, J. (2011). Cloning-independent and counterselectable markerless mutagenesis system in Streptococcus mutans. Appl. Environ. Microbiol. 77, 8025–8033. doi: 10.1128/AEM.06362-11
Xu, C., Bilya, S. R., and Xu, W. (2019). adeABC efflux gene in Acinetobacter baumannii. New Microbes New Infect. 30:100549. doi: 10.1016/j.nmni.2019.100549
Xu, Q., Chen, T., Yan, B., Zhang, L., Pi, B., Yang, Y., et al. (2019). Dual Role of gnaA in antibiotic resistance and virulence in Acinetobacter baumannii. Antimicrob. Agents Chemother. 63, e694–e619. doi: 10.1128/AAC.00694-19
Xue, C., Zhu, Y., Zhang, X., Shin, Y.-K., and Sashital, D. G. (2017). Real-time observation of target search by the CRISPR surveillance complex cascade. Cell Rep. 21, 3717–3727. doi: 10.1016/j.celrep.2017.11.110
Yang, J., Jiang, W., and Yang, S. (2009). mazF as a counter-selectable marker for unmarked genetic modification of Pichia pastoris. FEMS Yeast Res. 9, 600–609. doi: 10.1111/j.1567-1364.2009.00503.x
Yang-Yi Tan, S., Lin Chua, S., Liu, Y., Høiby, N., Percival Andersen, L., Givskov, M., et al. (2013). Comparative genomic analysis of rapid evolution of an extreme-drug-resistant Acinetobacter baumannii clone. Genome Biol. Evol. 5, 807–813. doi: 10.1093/gbe/evt047
Zarrilli, R., Pournaras, S., Giannouli, M., and Tsakris, A. (2013). Global evolution of multidrug-resistant Acinetobacter baumannii clonal lineages. Int. J. Antimicrob. Agents 41, 11–19. doi: 10.1016/j.ijantimicag.2012.09.008
Zhang, J., Zong, W., Hong, W., Zhang, Z. T., and Wang, Y. (2018). Exploiting endogenous CRISPR-Cas system for multiplex genome editing in Clostridium tyrobutyricum and engineer the strain for high-level butanol production. Metab. Eng. 47, 49–59. doi: 10.1016/j.ymben.2018.03.007
Zhang, X. Z., Yan, X., Cui, Z. L., Hong, Q., and Li, S. P. (2006). mazF, a novel counter-selectable marker for unmarked chromosomal manipulation in Bacillus subtilis. Nucleic Acids Res. 34:e71. doi: 10.1093/nar/gkl358
Keywords: multi-drug resistance, gene deletions, genetic complementation, selection marker, counter-selection marker, non-clinical antibiotics
Citation: Sykes EME, Deo S and Kumar A (2020) Recent Advances in Genetic Tools for Acinetobacter baumannii. Front. Genet. 11:601380. doi: 10.3389/fgene.2020.601380
Received: 31 August 2020; Accepted: 30 November 2020;
Published: 22 December 2020.
Edited by:
Natalia Polouliakh, Sony Computer Science Laboratories, JapanReviewed by:
Brock Aaron Arivett, Middle Tennessee State University, United StatesBalaji Veeraraghavan, Christian Medical College and Hospital, India
Copyright © 2020 Sykes, Deo and Kumar. This is an open-access article distributed under the terms of the Creative Commons Attribution License (CC BY). The use, distribution or reproduction in other forums is permitted, provided the original author(s) and the copyright owner(s) are credited and that the original publication in this journal is cited, in accordance with accepted academic practice. No use, distribution or reproduction is permitted which does not comply with these terms.
*Correspondence: Ayush Kumar, QXl1c2guS3VtYXJAdW1hbml0b2JhLmNh