- 1Department of Integrated Biological Science, Pusan National University, Busan, South Korea
- 2Department of Integrative Bioscience and Biotechnology, Sejong University, Seoul, South Korea
- 3Laboratory of Low Dose Risk Assessment, National Radiation Emergency Medical Center, Korea Institute of Radiological & Medical Sciences, Seoul, South Korea
- 4Department of Biological Sciences, Pusan National University, Busan, South Korea
Ionizing radiation (IR) is a high-energy radiation whose biological effects depend on the irradiation doses. Low-dose radiation (LDR) is delivered during medical diagnoses or by an exposure to radioactive elements and has been linked to the occurrence of chronic diseases, such as leukemia and cardiovascular diseases. Though epidemiological research is indispensable for predicting and dealing with LDR-induced abnormalities in individuals exposed to LDR, little is known about epidemiological markers of LDR exposure. Moreover, difference in the LDR-induced molecular events in each organ has been an obstacle to a thorough investigation of the LDR effects and a validation of the experimental results in in vivo models. In this review, we summarized the recent reports on LDR-induced risk of organ-specifically arranged the alterations for a comprehensive understanding of the biological effects of LDR. We suggested that LDR basically caused the accumulation of DNA damages, controlled systemic immune systems, induced oxidative damages on peripheral organs, and even benefited the viability in some organs. Furthermore, we concluded that understanding of organ-specific responses and the biological markers involved in the responses is needed to investigate the precise biological effects of LDR.
Introduction
Ionizing radiation (IR) is a high energy radiation that can change the status of intracellular nucleotides, proteins, and organic molecules by generating reactive oxygen species (ROS; Kim et al., 2019). Its effect exhibits a dose-dependently increase, and high dose radiation (HDR) has been utilized for eliminating inoperable cancer cells and keloid scars in patients (Rödel et al., 2017). Conversely, low-dose radiation (LDR) exposure occurs during clinical diagnoses – such as X-ray radiography and computed tomography (CT) – continuous nuclear work, or after nuclear accidents (Maqsudur Rashid et al., 2019). Although there are controversies regarding the definition and effects of LDR, many experimental studies consider IR under 0.5 Gy as LDR and have demonstrated that individuals exposed to LDR show significant health risks, including the occurrence of leukemia and cardiovascular diseases (Pearce et al., 2012; Siegel et al., 2017). However, the precise molecular mechanisms activated in response to LDR exposure are still elusive.
As the effects of LDR on humans are subtle and are generally represented as chronic effects, current studies have concluded the effects based on epidemiological studies in human and animal populations exposed to radiation from nuclear power plants (Boice et al., 2019). However, the results of these epidemiological studies are very limited and have identified very few epidemiological markers for further investigations (Braga-Tanaka et al., 2018). Many experimental studies have explored LDR-induced molecular markers in mouse and cell models and emphasized organ-specific sensitivities and responses in the expression patterns of these markers (Lee et al., 2006). Therefore, it is important to comprehend these studies in the context of alterations in biological events and markers in response to LDR exposure from the viewpoint of dose‐ and organ-specificity.
Although little is known about the mechanism of LDR exposure, many studies reported DNA damages, oxidative stress, and pro-inflammatory responses as major mediating events induced by an LDR exposure. Accumulations of the oxidative stresses and persistent pro-inflammatory responses cooperatively alters the cellular and mitochondrial redox balance, forms oxidized nucleotides, and impairs the DNA repair capacity, which results in DNA damages (Dąbrowska and Wiczkowski, 2017; Czarny et al., 2018; Moloney and Cotter, 2018). Previous studies showed that genes in euchromatic regions were more susceptible to both HDR‐ and LDR-induced DNA damage, double-strand break, and r-H2AX accumulation (Puck et al., 2002; Lafon-Hughes et al., 2008; Sak et al., 2015). Therefore, LDR-induced stresses convergently led to the permanent changes in the cells. Therefore, we focused on the risk of biological effects derived from the LDR exposure and suggested the phenotypic marker expressions.
In this review, we summarized the studies on LDR-induced biological effects in humans, which have been supported by various mouse and cell models. We arranged these effects according to dose‐ and organ-specificity, with descriptions based on significantly altered expressions of biological markers and related molecular mechanisms.
The Effects of LDR in Humans
The harmful effects of LDR were first reported by some epidemiological studies on individuals who were occupationally or accidently exposed to LDR. At that time, the studies warned that LDR could induce DNA damage and its related responses, which highlighted the need for investigations on LDR exposure-induced molecular changes. First, we discussed studies encompassing changes in people exposed to LDR.
A study evaluated the occurrence of mutations in hypoxanthine-phosphoribosyltransferase (HPRT) in workers engaged in the clean-up after the nuclear accident at the Chernobyl nuclear power plant (Thomas et al., 2002). Results showed that LDR (0.09–0.11 Gy) significantly increased deletion mutants in HPRT in peripheral blood cells, and that the mutation frequency declined with time following exposure. This study stressed the importance of early detection of LDR-induced DNA damage, which reduces with the passage of time. In addition, the same study found that the gene expression profile induced by 0.025 Gy was not significantly different from that induced by 0.1 Gy, which suggested a high sensitivity of humans to LDR exposures. LDR also alters gene expression through epigenetic regulation; global methylation levels were lower and chromosome aberrations higher in radiation-exposed workers than that in controls (Lee et al., 2015). Although LDR-induced ROS in humans has not been that well-studied, individuals exposed to LDR (0.004 Gy/year) showed increased H2O2 production and antioxidant expression, and their cells showed susceptibility to apoptosis (Russo et al., 2012).
Studies on people exposed to LDR after the Chernobyl nuclear power plant disaster showed that LDR > 0.01 Gy increased the expression of cytokine receptors and growth factors in blood monocytes, which suggested LDR induces the inflammatory responses (Albanese et al., 2007). The importance of LDR-induced biological aberrations was also supported by analyses of severe chronic symptoms presented by individuals exposed to the radiation. An epidemiological investigation about the effects of LDR – by European project toward low dose research toward multidisciplinary integration (DoReMi) – revealed that the risk of leukemia and solid tumors increased in response to exposure to LDR < 0.1 Gy, and that the risk of pediatric leukemia, brain tumor, and cardiovascular disease increased after CT scans with a radiation strength of 0.03–0.06 Gy (Pearce et al., 2012; Hall et al., 2017). In addition, the Chernobyl accident victims showed that enhanced CAP-Gly domain-containing linker protein 2 (CLIP2) expression derived from chromosomal mutation in 7q11.23 region (Kaiser et al., 2016). In the context of physiological malfunction, chronic LDR exposure in contaminated region resulted in increased microvessel density through stabilization of hypoxia-inducible factor-1 alpha via the activation of Ras/Raf signaling (Romanenko et al., 2012). Hormone levels, including those of thyroxin, cortisol, thromboxane B2, growth hormone, cAMP, cGMP, and 6-ketoprostaglandin F1, were reportedly altered upon LDR exposure in recovery workers working at the site of the Chernobyl accident (liquidators; Souchkevitch and Lyasko, 1997).
Although these studies highlighted the significant involvement of LDR in various symptoms, the underlying molecular mechanisms remain elusive due to the lack of studies about the biological responses and markers of LDR exposure. In the following section, we have listed and summarized the recent experimental studies on LDR exposure for a better understanding of the biological roles of the LDR-specific markers. Although the conditions of irradiation are quite different in each of these mice and cell models, most studies have reported the organ-specificity of LDR-induced biological effects. Therefore, we classified the results based on the tissues of interest with descriptions of the LDR doses used.
The Organ-Specific Effects of LDR in Cell and Mouse Models
Peripheral Blood Cells
The alterations in biological markers in blood are widely used for disease diagnosis due to high sample accessibility and variety. Therefore, many studies aimed at identifying the biological markers and studying the effects of LDR exposure used blood specimens. Collective analysis of peripheral blood cells is useful due to its availability. In an analysis of whole white blood cells, exposure to LDR < 0.5 Gy resulted in increased expression of DNA damage-inducible genes (Ishihara et al., 2016). In particular, it was found that the expression of Ku70, Ku80, and H2A histone family member X (H2AX) – but not that of γH2AX – was induced in peripheral blood cells upon prolonged LDR exposure in a rat model (Zhang et al., 2016b). This was supported by another study that demonstrated increased DNA strand breaks, oxidative base damage, and chromosomal aberrations with no changes in the amount of oxidized nucleic bases, following exposure to LDR (0.05 and 0.1 Gy) in human blood cells (Sudprasert et al., 2006). As the expression of DNA repair genes, including that of human 8-oxoguanine DNA N-glycosylase 1 (hOGG1) and X-ray repair cross complementing 1 (XRCC1), was downregulated by LDR (0.05 Gy) – which further decreased in a dose-dependent manner – LDR was suggested to enhance DNA damage and reduce DNA repair capacity in peripheral blood cells. In humoral immunity, LDR exposure decreased white blood cell and platelets counts along with the number of CD3+, CD4+, and CD8+ T cells and CD4+/CD8+ ratio in peripheral blood cells (Zhang et al., 2016b). In addition, LDR (0.3–0.7 Gy) reportedly reduced leukocyte/endothelial adhesion through inducing the expression of transforming growth factor beta (TGF-β) and interleukin 6 (IL-6), and inhibited leukocyte infiltration into inflammatory site (Roedel et al., 2002). Taken together, LDR could significantly regulate the status of peripheral blood cells by inducing DNA aberrations, suppressing activation, reducing viability, and perhaps inhibiting immunogenicity of peripheral blood cells.
Immune Cells
Having discussed the effects of LDR on whole peripheral blood cells, we focused on studies performed using sorted immune cells or cell lines. These studies present information regarding cell type-specific molecular events and signaling pathways involved in LDR responses in immune cells. In bone marrow mesenchymal stem cells (BM-MSCs), LDR (0.1 Gy) slowed the expansion of BM-MSCs and induced the differentiation of hematopoietic cells (HPCs) into myeloid progenitor cells (CD34+ CD38+ cells) through increased expression of IL-6 and decreased expression of FMS-like tyrosine kinase 3 ligand (Flt3L; Novershtern et al., 2011; Fujishiro et al., 2017). In contrast, LDR (0.1–0.2 Gy) promoted the proliferation of BM-MSCs in a dose-dependent manner (Wu et al., 2011). Further, LDR (0.025, 0.075, and 0.1 Gy) enhanced the proliferation and mobilization of HPCs into peripheral blood through increased granulocyte-colony stimulating factor (G-CSF) expression in a mouse model (Li et al., 2004). Although these results could not explain the regulation of proliferation in bone marrow stem cells, it is commonly believed that LDR enhances hematopoiesis through increased secretion of differentiating cytokines.
Innate immunity plays a major role in establishing primary defense mechanisms against antigens via inducing the inflammatory response, detecting foreign cells and phagocytosis, and presenting the antigens to adaptive immune cells. Therefore, a change in innate immune cell activity has a direct effect on the regulation of systemic immune responses (Zhang and Mosser, 2008). In microglial cells, LDR < 0.5 Gy was found to reduce oxidative stress by activating superoxide dismutase (SOD) and suppressing the formation of mitochondrial permeability transition pore (mPTP; Betlazar et al., 2016). This is consistent with findings from another study that showed that LDR (0.25 and 0.5 Gy) induced glutathione expression through activation of the activator protein 1 and NF-kB pathway in macrophages (Kawakita et al., 2003). LDR <0.5 Gy reduced the expression and secretion of IL-1β, IL-6, and tumor necrosis factor α (TNF-α) in macrophages (Li et al., 2017). In addition, LDR exposure also resulted in reduced p65 and extracellular signal-regulated kinase (ERK) phosphorylation, suggesting that LDR suppresses the dispersion of macrophages. Several studies have also demonstrated the significant roles of LDR in the regulation of mast cell activation. LDR < 0.5 Gy had no cytotoxic effects on mast cells, but resulted in reduced cytokine expression (TNF-α, IL-4, and IL-13), with subsequent suppression of allergic response (Joo et al., 2015). Consistently, LDR < 0.05 Gy reduced histamine, IL-4, TNF-α, and beta-hexosaminidase expression while LDR < 0.5 Gy suppressed migration and activation of mast cells through downregulation of nuclear receptor subfamily 4 group A member 2 (Nr4A2; Joo et al., 2012; Song et al., 2019). Taken together, studies show that the exposure of innate immune cells to LDR can lead to the suppression of immunity through regulatory imbalance in the levels of inflammatory cytokines and oxidative stress.
On the other hand, investigations regarding the effects of LDR on adaptive immune cells are limited. One study demonstrated that LDR (0.05 Gy) activated T cells by increasing interferon γ (IFN-γ), IL-2 expression, and differentiation rates, even in immune-suppressive environments (Chen et al., 2011, 2014b). Heat shock protein 70 – whose expression is increased by LDR – could aid the delivery of antigenic peptides to T cells via indirect antigen presentation pathways (Multhoff et al., 2015). In B cells, LDR (0.1 Gy) reportedly induced expression of miRNAs including let7a, miR-15b, miR-16, miR-21, and miR-23b, which commonly target the lipid biosynthetic enzyme, glycerol-3-phosphate acyltransferase (GPAT; Tabe et al., 2016). As the expression of GPAT correlates with the immunogenic activity of B cells, LDR might help B cell in acquiring immunity. A transcriptome analysis confirmed LDR-induced (0.05 Gy) expression of IFN-γ, IL-4, and IL-6 (Cho et al., 2018). In the same study, LDR also induced the expression of genes involved in mRNA translation, mitochondrial function, cell cycle regulation, and cytokine induction. These studies demonstrated that LDR induced acquisition of immunity of T and B cells through regulation of both intracellular gene expression and cytokine expression. Given that these findings clearly contradict the findings of studies conducted in innate immunity cells, more strictly-controlled investigations are warranted to elucidate the roles of LDR in immunity.
Skin
The skin is the primary recipient of external radiation, and many studies have investigated molecular alterations in skin models after LDR exposure. In HaCaT cells, LDR (0.1 Gy) induced p21 expression and increased keratinocyte differentiation (Hahn et al., 2016). However, in another study, LDR < 0.1 Gy increased the differentiation of HaCaT cells, but suppressed p21 expression, remaining the disparity of LDR-induced survival changes in HaCaT cells (Son et al., 2019). In a study on the effects of LDR on the survival of HaCaT cells, 0.05 and 0.5 Gy of LDR was found to upregulate the expression of TP53, BAX, BCL-2, and caspases 2 and 6, leading to cell cycle arrest and transduction of pro-apoptotic signals (Furlong et al., 2013). LDR-induced changes were also validated by a metabolomic analysis, which showed that LDR (0.03 and 0.1 Gy) significantly altered the concentration of metabolites involved in DNA/RNA damage and repair and lipid and energy metabolism (Hu et al., 2012). Although the data are few, these studies suggested that LDR could enhance the differentiation of keratinocytes and could modulate cell proliferation through cell cycle regulation and apoptotic gene expression.
In dermal fibroblasts, the expression of collagen type I alpha 1 (COL1A1), matrix metalloproteinase-1, growth/differentiation factor 15, and Connexin 43 was found to be increased by LDR < 0.5 Gy (Glover et al., 2003; Bae et al., 2015; Sándor et al., 2015). Alterations in these molecules indicated that LDR could accelerate the remodeling of the dermal matrix. A transcriptome analysis performed in primary human skin fibroblast compared a list of the most distinctive signaling pathways responsive to LDR and HDR (Ding et al., 2005). This study showed that genes related to the regulation of extracellular matrix (ECM) were induced by both LDR and HDR while genes involved in cytoskeleton and intercellular signaling were responsive only to LDR. Given these results, LDR exposure specifically enhanced gene expression related to ECM remodeling in dermal fibroblasts without significant changes in genomic DNA.
Liver
The liver is a major organ that controls systemic metabolism and maintains homeostasis in response to external stimuli. Many studies implicated LDR as a problematic factor inducing significant changes in liver function and deregulating some hepatic metabolites involved in homeostasis. Firstly, in a mouse model, LDR (0.25 and 0.5 Gy) increased the hepatic expression and urine level of hepcidin-2 even after 168 h of irradiation (Iizuka et al., 2016). The increase in urine hepcidin levels was related with impairments in iron transport, which was consistent with the findings of another study that showed that X-rays interfered with the maintenance of iron homeostasis in a mouse model (Christiansen et al., 2007).
In addition, many studies have proposed the principal roles for LDR in glucose and lipid metabolism. LDR (0.1 and 0.5 Gy) inhibited the glycolytic pathway and pyruvate dehydrogenase to suppress glucose consumption and inhibited lipid metabolism via inactivation of peroxisome proliferator-activated receptor α and got worse liver inflammation (Bakshi et al., 2015). Although it was not clear whether LDR enhances or suppresses lipid oxidation, the alterations in hepatic lipid metabolism in response to LDR exposure were supported by some in vivo studies. Accumulative LDR exposure (total 0.02 or 0.4 Gy) significantly decreased the expression of genes involved in non-esterified fatty acid metabolism ontology in mice (Uehara et al., 2010). In mice exposed continuously to LDR, LDR-induced lipid metabolic changes were also found to be influenced by changes in miRNA (miR-21, miR-221, miR-421, miR-155, and miR-375) expression (Liang et al., 2018). In summary, the studies implied that LDR exposure induced liver specific gene alteration leading to imbalanced homeostasis with deregulated iron, glucose, and lipid metabolism in the liver.
Brain
Although the skull protects the brain from external damages, LDR does penetrate and affect the brain. Experimental studies have provided various evidences about the occurrence of brain damage in response to LDR exposure. A transcriptome analysis showed that LDR (0.1 Gy) mediated gene expression change related to DNA damage response, ion channels regulation, long-term potentiation/depression, and vascular damage in the brain tissues of mice (Lowe et al., 2009). Furthermore, LDR-induced (0.1 Gy) DNA damage was validated by increased expression of genes involved in DNA repair [protein tyrosine phosphatase non-receptor type 1 (PTPN1), PMS2, high mobility group nucleosomal binding domain 2 (HMGN2), and interferon regulatory factor 3 (IRF3); Birger et al., 2003; Furusawa and Cherukuri, 2010; Yu et al., 2015; Mojena et al., 2018]. These aberrant changes induced by LDR also led to the activation of survival signaling pathway in the brain. LDR < 0.5 Gy induced the expression of cytokine IL-8, leading to the activation of NF-κB and upregulation of NF-κB target genes [intercellular adhesion molecule 1 (ICAM-1), vascular cell adhesion molecule 1 (VCAM-1), and cyclooxygenase-2 (COX2)] in the brain (Manna and Ramesh, 2005; Veeraraghavan et al., 2011). In addition, NF-κB activated by LDR (0.01 and 0.5 Gy) reportedly increased SOD2 expression, which triggered a NF-κB-SOD2-NF-κB positive feedback cycle (Veeraraghavan et al., 2011; Bevelacqua and Mortazavi, 2018). Studies also reported that the damages induced by LDR resulted in neuronal defects. In a mouse model, LDR (0.075 Gy) decreased cAMP levels and cAMP/cGMP ratio in the neurons of the hypothalamus, which was related to the suppression of neurite growth and regeneration (Wan et al., 2001; Batty et al., 2017). An analysis of mice exposed to LDR (0.05 Gy) revealed that the expression of pre‐ and post-synaptic markers (Synapsin 1, Synaptophysin, Synapse-associated protein 97, Debrin 1, and Postsynaptic density protein 95) was significantly increased, which may cause impairments in cognition and learning (Howe et al., 2019). This is consistent with the findings of a study that showed that LDR (0.5 Gy) had a profound effect on the brain, as evidenced by precursor cell dysfunction and defects in cognition (Silasi et al., 2004). In summary, exposure of brain tissues to LDR can result in detrimental biological marker alterations mediated by oxidative stress and DNA damage, and possible impairment of learning and memory.
Heart
Atherosclerosis is one of the most concerning disease found in those exposed to IR (Barjaktarovic et al., 2013a). Although a precise molecular mechanism about this phenomenon has not been suggested yet, we summarized previous studies covering the effects of LDR on the heart tissues to find a common conclusion. Some studies insisted that LDR induced harmful effects on cardiac tissue by showing that LDR (0.04 Gy) significantly increased oxidative stress and downstream responsive protein expressions in cardiac tissues (Barjaktarovic et al., 2013a; Horot and Tkachenko, 2017; Seawright et al., 2017). Furthermore, LDR-induced oxidative stress was led to non-transient alteration in metabolism of cardiac muscles and malfunctioning (Barjaktarovic et al., 2013b). In the aspect of metabolic alteration, two studies commonly reported LDR suppressed lipid metabolism with evidence that LDR (0.28 Gy) increased cardiac expression of miRNAs leading to lipid metabolism suppression and chronic internal/external LDR exposure (0.1 mGy/day) showed reduced lipid catabolism and mitochondrial oxidation (Orekhova and Modorov, 2017; Liang et al., 2018). These oxidative stresses possibly mediated heart specific biological effects according to a study that LDR (0.2 Gy) induced DNA damage and its responsive genes expression in primary human fibroblast cell from heart (Grudzenski et al., 2010). However, completely opposite results were suggested in studies to investigate the molecular events after LDR exposure in atherosclerosis models. LDR under 0.1 Gy was delivered to several cardiovascular disease models induced by type 1 diabetes, Apolipoprotein E deficiency, and doxorubicin treatment and reduced oxidative stress and inflammation in the heart were found in the models (Zhang et al., 2011, 2016a; Mathias et al., 2015; Jiang et al., 2017). Therefore, LDR may be a threat for the cardiac tissue impairment, as well as relieve the cardiac damages through regulation of oxidative stress and pro-inflammatory responses.
Thyroid
The thyroid is one of the most sensitive organs against to LDR exposure from CT, occupational radiation exposures, or nuclear accidents (Sinnott et al., 2010). Most of the studies on the thyroid have assessed the effect of exposure to radiation originating from CT, while a few studies reported on the biological events induced by experimental LDR. LDR < 0.5 Gy could induce increase the significant risk of mediated by ROS generation and DNA damage-responsive expressions of γH2AX and p-p53 (Ser15) in the thyroid (Kanagaraj et al., 2015; Kurashige et al., 2017; Visweswaran et al., 2019).
These damages were validated by histological changes (increase in thyroid gland follicle size, nucleic size, colloid density, and induction of hemorrhage) and functional changes (reduced thyroid hormone synthesis and secretion) in mouse models (Nadol’nik et al., 2003, 2005; Pavlov et al., 2013). LDR-induced thyroid dysfunctions were also supported by the studies, demonstrating unique effects of LDR in autoimmune thyroiditis mouse models. In these studies, LDR (0.5 Gy) consistently promoted the development of thyroiditis through increased autoimmunity; these findings are similar those observed in patients exposed to radiation (Nagayama et al., 2008, 2009). Conversely, LDR < 0.1 Gy protected the thyroid from mutation-induced carcinogenesis (Kaushik et al., 2019). Taken together, LDR can easily damage the thyroid through generation of the significant DNA damages, which are determined by the total dose of radiation.
Lung
Many studies have shown that the lung ranks among the organs that are most resistant to LDR damage. LDR (0.05–0.6 Gy) did not induce significant biological damage in the lung (Sypin et al., 2003; Kim et al., 2007; Jangiam et al., 2018; Puukila et al., 2019). Although many studies opposed it through showing LDR (0.1 and 0.2 Gy) reportedly promoted cell death, inflammation, ROS generation (lipid oxidation), and DNA damage (5-hydroxymethylcytosine); and activated damage responses (p53, p38, p21, ERK1/2, NF-κB, TGF-β, etc.) in the lung, these studies unanimously agreed that certain irradiation conditions resulted in less severe changes in lung than that in the liver and the spleen (Sypin et al., 2003; Avti et al., 2005; Lee et al., 2006; Kim et al., 2007, 2015; Hong et al., 2014; Jangiam et al., 2018). Moreover, the protective roles of LDR in the lung have been demonstrated in various stress models. In these studies, LDR up to 0.5 Gy suppressed ROS generation, attenuated inflammatory responses (IL-1β, IL-5, IL-6, and TNF-α), delayed senescence, and reduced adverse effects induced by HDR (Kim et al., 2007, 2015; Hong et al., 2014; Liang et al., 2016; Velegzhaninov et al., 2018; Park et al., 2019; Son et al., 2019). Given these results, the lung may be more resistant to LDR exposure than other organs and seems to have a high threshold for LDR-induced lung specific biological marker alteration. Although the precise reason of pulmonary resistance against LDR, it is supposed to be based on the lung-specific antioxidant gene expression to modulate oxidative stress (Kim et al., 2018).
Spleen
The spleen consists of large number of pulps where peripheral blood cells get collected, activated, or removed; and molecular crosstalk between the spleen and peripheral blood cells determines the systemic immune responses. Similar to the reports on LDR-regulated oxidative stress and inflammatory signaling in peripheral blood cells, studies on the spleen also reported LDR-mediated control of tissue damage. In mouse models, LDR (0.02 and 0.2 Gy) protected the tissue from oxidative stress by increasing the synthesis and recycling of GSH via the expression of glutamate-cysteine ligase modifier subunit (GCLM), GSH synthase, and glutathione peroxidase (Lee et al., 2013). This antioxidant effect of LDR was validated in other studies where LDR < 0.1 Gy reduced following HDR-induced damages (Yoshida et al., 1993; Bannister et al., 2015). Another investigation on the effect of LDR on splenocytes demonstrated that LDR (0.02 Gy) did not induce significant damage in cells, and instead slowed the rate of clearance and turnover of damaged cells (Bannister et al., 2015). As pretreatment with LDR (0.1 Gy) even attenuated the stress-induced inflammation of the spleen, the protective roles of LDR in the spleen may be evident (Bannister et al., 2015; Son et al., 2019). However, other studies reported that LDR > 0.1 Gy significantly induced apoptosis in the spleen via inducing the expression of p53 and p21 (Lee et al., 2006; Bannister et al., 2015). A few reports on the relationship between the spleen and immune cells also demonstrated the protective effects of LDR. LDR < 0.1 Gy increased the percentage of the CD4+ T cell subpopulation while dendritic cell and macrophage counts were reduced in response to short-term exposures (Song et al., 2015). However, long term LDR exposure reduced the percentage of the CD8+ or CD28+ T cell subpopulation. Interestingly, the common findings from these studies demonstrated a high sensitivity of the spleen with respect to LDR-induced effects that are not similar to those found in other tissues, such as brain, liver, lung, and testis. These results suggest that the LDR induced both harmful and beneficial biological effects in the spleen and followed a hormesis model of LDR-induced responses with a threshold of 0.1 Gy.
Endothelium
The endothelium refers to cells that line the inner surface of blood vessels and lymphatic vessels. Endothelial cells are composed of simple, single-layered, squamous cells that have direct contact with the blood and lymph. As blood cells are closely associated with endothelial tissues, the changes in the endothelium in response to LDR exposure have been discussed here. According to the studies, LDR was found to make human umbilical vein endothelial cells (HUVECs) more sensitive to pro-inflammatory activation. LDR < 0.5 Gy could activate NF-κB signaling pathway through both increased expression and enhanced transcriptional activity (Prasad et al., 1994; Rödel et al., 2004; Cervelli et al., 2014). LDR (<0.5 Gy) promoted the formation of pro-inflammatory environments by an increase in epithelial ICAM-1 expression and an activation of the adhesion and migration of leukocytes towards inflammatory sites (Voisard et al., 2007; Cervelli et al., 2014). In addition, LDR induced the expression of pro-inflammatory cytokines (IL-8, G-CSF, and platelet-derived growth factor-BB) in epithelial cells, which resulted in a microenvironment that is highly susceptible to inflammation (Meeren et al., 1997; Schröder et al., 2019). LDR (0.1 Gy) increased the expression of endothelin 1, a pro-inflammatory and fibrotic inducer, in HUVECs (Lanza et al., 2007). Further, LDR (0.05 and 0.072 Gy) was found to induce proliferation and migration through ERK signaling in endothelial progenitor cells; this result serves as an evidence for LDR-induced endothelial inflammation (Wang et al., 2019). With the pro-inflammatory responses, LDR (<0.5 Gy) could induce double-strand breaks of DNA and activate DNA damage responses in endothelial cells supported by a microarray data (Mouw et al., 2017). Taken together, LDR is implicated as a major inductor of cellular and environmental inflammation response and DNA damages in the endothelial tissue.
Osteoblast/Clast
Regulation of the balance between osteoblasts and osteoclasts is important for bone growth and maintenance. The best microstructure for bone growth is formed through repeated formation and absorption. In adults, highly activated osteoclasts in bone tissue can induce arthritis and osteoporosis through bone absorption and inflammation (Lee et al., 2017). Studies that investigate the effects of LDR on osteoblasts and osteoclasts have assessed the alterations in differentiation, proliferation, and activity. Irradiation with LDR (<0.5 Gy) resulted in enhanced differentiation of osteoblasts (Chen et al., 2014a; Lima et al., 2017; Yang et al., 2017). It was evidenced by the activation of Wnt and NF-κB signaling transduction and the expression of differentiation markers [COL1A, alkaline phosphatase, osteocalcin (OCN), core-binding factor alpha 1, and osteoprotegerin; Xu et al., 2012; Chen et al., 2016, 2017; She et al., 2016; Deloch et al., 2018a]. In addition, LDR < 0.5 Gy was found to increase the proliferation of BM-MSCs and osteoblasts, which led to activation of osteoblasts (Chen et al., 2014a; Yang et al., 2017). With respect to osteoclast, multiple studies have reported that LDR (0.5 Gy) suppresses the differentiation into osteoclasts and bone resorbing activities, which led to enhanced bone formation and ameliorated arthritis (Deloch et al., 2018a,b). From these studies, it seems that LDR protects and promotes bone growth by regulating genes related to supporting the proliferation of osteoblast/osteoclasts.
Testis and Ovary
The testis is a reproductive organ which must be protected from mutations. Testicular tissues are highly vulnerable to damages induced by LDR exposure. LDR < 0.2 Gy increased the levels of ROS, which leads to induction of ER stress and apoptosis in testicular cells of irradiated mice (Wang et al., 2013). Long-term LDR exposure also altered the expression of miRNAs to induce apoptosis through oxidative stress in mouse testes (Liang et al., 2018). However, in the state of inflammation – in the background of type 1 diabetes – LDR < 0.05 Gy has been reported to attenuate apoptosis in mouse testicular tissues (Zhao et al., 2010). Given these results, LDR may pose a major threat to testicular tissue due to aberrant gene expression, despite its protective effects with respect to hyperinflammation.
Only few studies have assessed the changes induced by LDR exposure in other reproductive organs, although the changes reported were significant. LDR (0.05 Gy) protected oocytes from HDR exposure-induced DNA damage (Jacquet et al., 2008). Conversely, LDR (0.1 Gy) significantly reduced the number of follicles as both short‐ and long-term effects (Kimler et al., 2018). In addition, LDR (0.36 Gy) reduced 50% of the germ cells in ovaries of prenatal and neonatal mice (Rönnbäck, 1983). Taken together, LDR also showed hormetic effects in the ovary, while the effective threshold was further lower than that in other organs.
Conclusion
Determination of LDR exposure is an important problem for continuity of nuclear works and epidemiological analysis of nuclear accidents. However, the effects of LDR are subtle, and the absence of reliable biological markers has been obstacles. In this review, we summarized the biological markers caused by LDR in human, mouse, and cell studies for better understanding of the effects of LDR. Due to the limited studies that have investigated the changes in humans, most of the studies reviewed here were based on the findings in experimental models. The alterations of biological markers by LDR were summarized in Figure 1 and their related molecular events were listed with corresponding references in Table 1. By suggesting promising molecular markers expressed in each organ, we look forward to further studies to discover the LDR specific molecular makers based on this review.
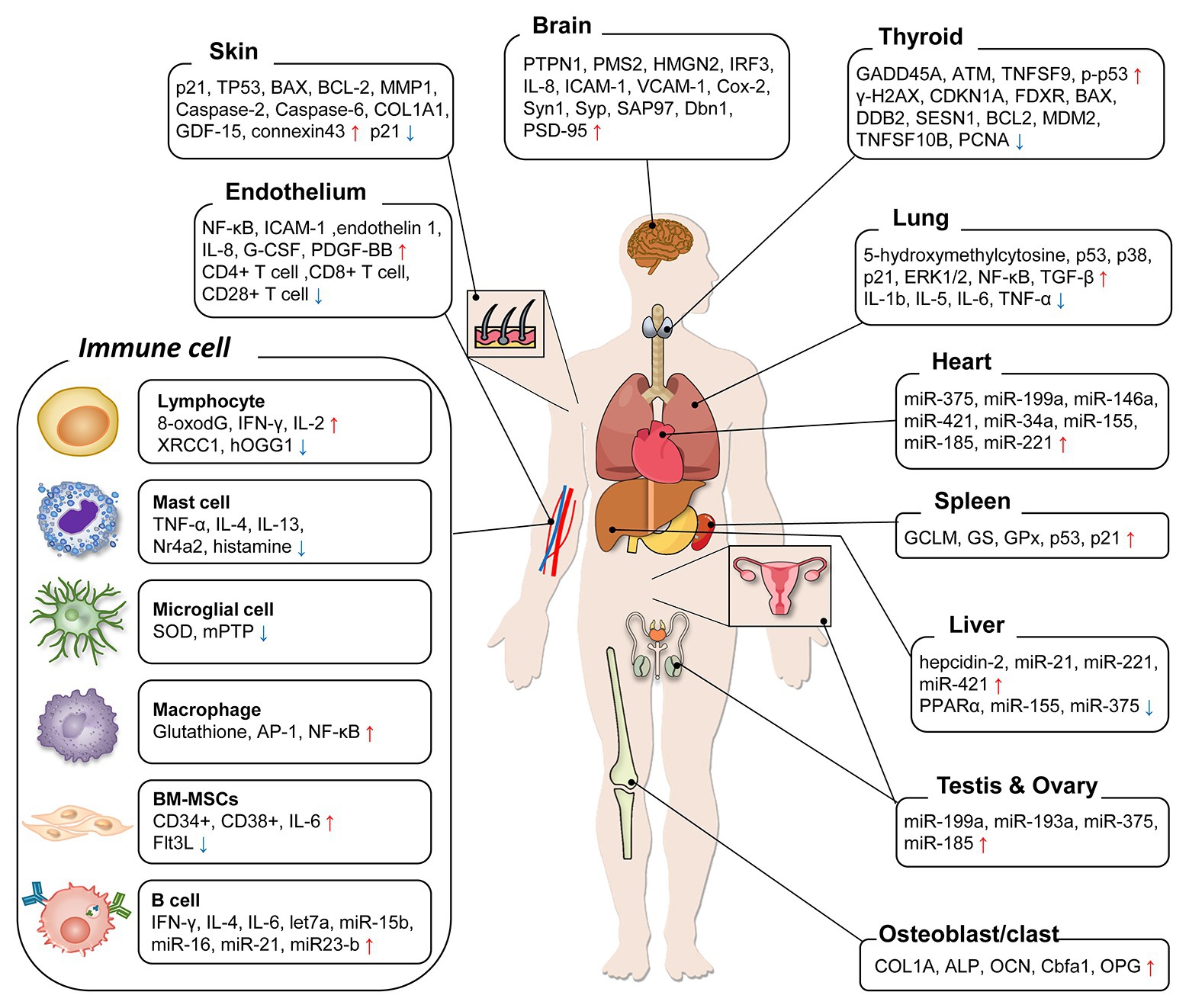
Figure 1. A diagram summarizing organ-specific biological markers by low-dose radiation (LDR) exposure. The molecular markers suggested in previous LDR studies were categorized. For each organ, the markers were enumerated following the expression change after LDR exposure and increase/decrease was marked as arrows (↑/↓).
Although previous studies suggested that there are quite a few differences of the marker expressions among organs by LDR exposure, the molecular basis about the organ-specific sensitivity against LDR exposure has not been well-studied. We observed that LDR generally suppressed innate immune system, while established pro-inflammatory environments for adaptive immune cells. In peripheral organs and brain, LDR commonly induced DNA damages and oxidative stresses, which led to systemic aberrations. Oppositely, hormetic effects of LDR were barely shown in studies about spleen, osteoblasts, and ovary. In a recent study, the concentration of radionuclides was measured in several organs of 79 cattle around the Fukushima Daiichi nuclear power plant (Fukuda et al., 2013), and the deposition of radionuclides was different depending on the organs examined. The radionuclides deposition in muscles was top ranked among the organs due to proximity to skin, but brain, which is covered by skull, showed lower deposition of radionuclides compared to most other organs. This result suggested that even with whole-body irradiation, the irradiation dose and the effect of LDR might vary depending on the location of the organ. Collectively, a convoluted understanding of the organ-specific LDR responses and sensitivity against LDR can be a promising strategy to figure out the core molecular markers.
Although many epidemiological studies about radiation accident or LDR exposure have suggested the risk ratio of diseases, including cancers and cardiovascular diseases, they only could utilize the irradiation doses as a risk factor (Kreuzer et al., 2015; Shore et al., 2017; Hauptmann et al., 2020). As the measuring was performed in various sites (colon, stomach, skin, etc.), it is difficult to interpret the epidemiological analysis precisely. In recent epidemiology studies, the introduction of associated biological markers not only enhanced the accuracy of the analysis but also enabled early diagnosis of the diseases (Sjaarda et al., 2018; Elliott et al., 2019). Furthermore, in the studies covering a stimulus and disease with organ-specific cytotoxicity, the significance of measuring organ-specific biomarkers greatly increases (Tajima et al., 2019; Lieske et al., 2020). Therefore, further epidemiological LDR research is warranted to consider the utilization of biological markers for its risk estimation and pro/retrospective analysis. After all, we hope this review can be relayed to the next generation as an inspiration for further research to find out LDR-specific molecular markers based on biological basis.
Author Contributions
ES, SL, HK, and BY: conceptualization. ES, SL, HK, and BY: writing original draft preparation. ES, SL, HK, JK, KK, HY, YJ, SS, and BY: writing review and editing. BY: supervision and project administration. All authors contributed to the article and approved the submitted version.
Funding
This work was supported by the Nuclear Safety Research Program through the Korea Foundation of Nuclear Safety (KoFONS) using the financial resource granted by the Nuclear Safety and Security Commission (NSSC) of the Republic of Korea (no. 1805019), a grant of the Korea Institute of Radiological and Medical Sciences (KIRAMS), funded by Nuclear Safety and Security Commission (NSSC) of the Republic of Korea (No. 50091-2020), and PNU-RENovation (2019-2020).
Conflict of Interest
The authors declare that the research was conducted in the absence of any commercial or financial relationships that could be construed as a potential conflict of interest.
Abbreviations
BM-MSC, Bone marrow mesenchymal stem cell;; COL1A1, Collagen type I alpha 1;; CT, Computed tomography;; ECM, Extracellular matrix;; ERK, Extracellular signal-regulated kinases;; G-CSF, Granulocyte-colony stimulating factor;; H2AX, H2A histone family member X;; HDR, High dose radiation;; HIF-1α, Hypoxia-inducible factor-1 alpha;; HPC, Hematopoietic cell;; HPRT, Hypoxanthine-phosphoribosyltransferase;; HUVECs, Human umbilical vein endothelial cells;; IFN, Interferon;; IL, Interleukin;; IR, Ionizing radiation;; LDR, Low dose radiation;; NF-kB, Nuclear factor kappa-light-chain-enhancer of activated B cells;; ROS, Reactive oxygen species;; SOD, Superoxide dismutase;; TGF-β, Transforming growth factor beta.
References
Albanese, J., Martens, K., Karanitsa, L. V., Schreyer, S. K., and Dainiak, N. (2007). Multivariate analysis of low-dose radiation-associated changes in cytokine gene expression profiles using microarray technology. Exp. Hematol. 35(Suppl. 1), 47–54. doi: 10.1016/j.exphem.2007.01.012
Avti, P. K., Pathak, C. M., Kumar, S., Kaushik, G., Kaushik, T., Farooque, A., et al. (2005). Low dose gamma-irradiation differentially modulates antioxidant defense in liver and lungs of Balb/c mice. Int. J. Radiat. Biol. 81, 901–910. doi: 10.1080/09553000600567996
Bae, S., Kim, K., Cha, H. J., Choi, Y., Shin, S. H., An, I. -S., et al. (2015). Low-dose γ-irradiation induces dual radio-adaptive responses depending on the post-irradiation time by altering microRNA expression profiles in normal human dermal fibroblasts. Int. J. Mol. Med. 35, 227–237. doi: 10.3892/ijmm.2014.1994
Bakshi, M. V., Azimzadeh, O., Barjaktarovic, Z., Kempf, S. J., Merl-Pham, J., Hauck, S. M., et al. (2015). Total body exposure to low-dose ionizing radiation induces long-term alterations to the liver proteome of neonatally exposed mice. J. Proteome Res. 14, 366–373. doi: 10.1021/pr500890n
Bannister, L. A., Serran, M. L., and Mantha, R. R. (2015). Low-dose gamma radiation does not induce an adaptive response for micronucleus induction in mouse splenocytes. Radiat. Res. 184, 533–544. doi: 10.1667/RR14102.1
Barjaktarovic, Z., Anastasov, N., Azimzadeh, O., Sriharshan, A., Sarioglu, H., Ueffing, M., et al. (2013a). Integrative proteomic and microRNA analysis of primary human coronary artery endothelial cells exposed to low-dose gamma radiation. Radiat. Environ. Biophys. 52, 87–98. doi: 10.1007/s00411-012-0439-4
Barjaktarovic, Z., Shyla, A., Azimzadeh, O., Schulz, S., Haagen, J., Dörr, W., et al. (2013b). Ionising radiation induces persistent alterations in the cardiac mitochondrial function of C57BL/6 mice 40 weeks after local heart exposure. Radiother. Oncol. 106, 404–410. doi: 10.1016/j.radonc.2013.01.017
Batty, N. J., Fenrich, K. K., and Fouad, K. (2017). The role of cAMP and its downstream targets in neurite growth in the adult nervous system. Neurosci. Lett. 652, 56–63. doi: 10.1016/j.neulet.2016.12.033
Betlazar, C., Middleton, R. J., Banati, R. B., and Liu, G. -J. (2016). The impact of high and low dose ionising radiation on the central nervous system. Redox Biol. 9, 144–156. doi: 10.1016/j.redox.2016.08.002
Bevelacqua, J. J., and Mortazavi, S. M. J. (2018). Alzheimer’s disease: possible mechanisms behind neurohormesis induced by exposure to low doses of ionizing radiation. J. Biomed. Phy. Eng. 8, 153–156. doi: 10.31661/JBPE.V8I2.919
Birger, Y., West, K. L., Postnikov, Y. V., Lim, J. -H., Furusawa, T., Wagner, J. P., et al. (2003). Chromosomal protein HMGN1 enhances the rate of DNA repair in chromatin. EMBO J. 22, 1665–1675. doi: 10.1093/emboj/cdg142
Boice, J. D., Held, K. D., and Shore, R. E. (2019). Radiation epidemiology and health effects following low-level radiation exposure. J. Radiol. Prot. 39, S14–S27. doi: 10.1088/1361-6498/ab2f3d
Braga-Tanaka, I. 3rd, Tanaka, S., Kohda, A., Takai, D., Nakamura, S., Ono, T., et al. (2018). Experimental studies on the biological effects of chronic low dose-rate radiation exposure in mice: overview of the studies at the institute for environmental sciences. Int. J. Radiat. Biol. 94, 423–433. doi: 10.1080/09553002.2018.1451048
Cervelli, T., Panetta, D., Navarra, T., Andreassi, M. G., Basta, G., Galli, A., et al. (2014). Effects of single and fractionated low-dose irradiation on vascular endothelial cells. Atherosclerosis 235, 510–518. doi: 10.1016/j.atherosclerosis.2014.05.932
Chen, Y., Chen, X., Li, Y., Zhang, H., Xie, Y., Zhang, X., et al. (2011). Early effects of low dose C ion or X-ray irradiation on peripheral blood lymphocytes of patients with alimentary tract cancer. Dose-Response 9, 356–368. doi: 10.2203/dose-response.10-015.Chen
Chen, M., Dong, Q. R., Huang, Q., She, C., and Xu, W. (2017). 0.5 Gy X-ray radiation promotes osteoblast differentiation by Wnt/β-catenin signaling. Zhonghua Yi Xue Za Zhi 97, 1820–1825. doi: 10.3760/cma.j.issn.0376-2491.2017.23.013
Chen, M., Dong, Q. R., Huang, Q., Xu, W., and She, C. (2016). Effects of 0.5 Gy X-ray radiation on the profile of gene expression in MC3T3-E1 osteoblasts. Zhonghua Yi Xue Za Zhi 96, 2659–2664. doi: 10.3760/cma.j.issn.0376-2491.2016.33.013
Chen, M., Huang, Q., Xu, W., She, C., Xie, Z. -G., Mao, Y. -T., et al. (2014a). Low-dose X-ray irradiation promotes osteoblast proliferation, differentiation and fracture healing. PLoS One 9:e104016. doi: 10.1371/journal.pone.0104016
Chen, Y., Wang, C., He, M., Zhang, H., and Chen, X. (2014b). Effect of low dose heavy ion irradiation on subset percentage and cytokines expression of peripheral blood lymphocytes in patients with pancreatic cancer. Zhonghua Zhong Liu Za Zhi 36, 435–439.
Cho, S. -J., Kang, H., Hong, E. -H., Kim, J. Y., and Nam, S. Y. (2018). Transcriptome analysis of low-dose ionizing radiation-impacted genes in CD4+ T-cells undergoing activation and regulation of their expression of select cytokines. J. Immunotoxicol. 15, 137–146. doi: 10.1080/1547691X.2018.1521484
Christiansen, H., Sheikh, N., Saile, B., Reuter, F., Rave-Fränk, M., Hermann, R. M., et al. (2007). X-irradiation in rat liver: consequent upregulation of hepcidin and downregulation of hemojuvelin and ferroportin-1 gene expression. Radiology 242, 189–197. doi: 10.1148/radiol.2421060083
Czarny, P., Wigner, P., Galecki, P., and Sliwinski, T. (2018). The interplay between inflammation, oxidative stress, DNA damage, DNA repair and mitochondrial dysfunction in depression. Prog. Neuro-Psychopharmacol. Biol. Psychiatry 80, 309–321. doi: 10.1016/j.pnpbp.2017.06.036
Dąbrowska, N., and Wiczkowski, A. (2017). Analytics of oxidative stress markers in the early diagnosis of oxygen DNA damage. Adv. Clin. Exp. Med. 26, 155–166. doi: 10.17219/acem/43272
Deloch, L., Derer, A., Hueber, A. J., Herrmann, M., Schett, G. A., Wölfelschneider, J., et al. (2018a). Low-dose radiotherapy ameliorates advanced arthritis in hTNF-α tg mice by particularly positively impacting on bone metabolism. Front. Immunol. 9:1834. doi: 10.3389/fimmu.2018.01834
Deloch, L., Rückert, M., Fietkau, R., Frey, B., and Gaipl, U. S. (2018b). Low-dose radiotherapy has no harmful effects on key cells of healthy non-inflamed joints. Int. J. Mol. Sci. 19:3197. doi: 10.3390/ijms19103197
Ding, L. -H., Shingyoji, M., Chen, F., Hwang, J. -J., Burma, S., Lee, C., et al. (2005). Gene expression profiles of normal human fibroblasts after exposure to ionizing radiation: a comparative study of low and high doses. Radiat. Res. 164, 17–26. doi: 10.1667/rr3354
Elliott, H. R., Sharp, G. C., Relton, C. L., and Lawlor, D. A. (2019). Epigenetics and gestational diabetes: a review of epigenetic epidemiology studies and their use to explore epigenetic mediation and improve prediction. Diabetologia 62, 2171–2178. doi: 10.1007/s00125-019-05011-8
Fujishiro, A., Miura, Y., Iwasa, M., Fujii, S., Sugino, N., Andoh, A., et al. (2017). Effects of acute exposure to low-dose radiation on the characteristics of human bone marrow mesenchymal stromal/stem cells. Inflamm. Regen. 37:19. doi: 10.1186/s41232-017-0049-2
Fukuda, T., Kino, Y., Abe, Y., Yamashiro, H., Kuwahara, Y., Nihei, H., et al. (2013). Distribution of artificial radionuclides in abandoned cattle in the evacuation zone of the Fukushima Daiichi nuclear power plant. PLoS One 8:e54312. doi: 10.1371/journal.pone.0054312
Furlong, H., Mothersill, C., Lyng, F. M., and Howe, O. (2013). Apoptosis is signalled early by low doses of ionising radiation in a radiation-induced bystander effect. Mutat. Res. 741–742, 35–43. doi: 10.1016/j.mrfmmm.2013.02.001
Furusawa, T., and Cherukuri, S. (2010). Developmental function of HMGN proteins. Biochim. Biophys. Acta 1799, 69–73. doi: 10.1016/j.bbagrm.2009.11.011
Glover, D., Little, J. B., Lavin, M. F., and Gueven, N. (2003). Low dose ionizing radiation-induced activation of connexin 43 expression. Int. J. Radiat. Biol. 79, 955–964. doi: 10.1080/09553000310001632895
Grudzenski, S., Raths, A., Conrad, S., Rübe, C. E., and Löbrich, M. (2010). Inducible response required for repair of low-dose radiation damage in human fibroblasts. Proc. Natl. Acad. Sci. U. S. A. 107, 14205–14210. doi: 10.1073/pnas.1002213107
Hahn, H. J., Youn, H. J., Cha, H. J., Kim, K., An, S., and Ahn, K. J. (2016). Single low-dose radiation induced regulation of keratinocyte differentiation in calcium-induced HaCaT cells. Ann. Dermatol. 28, 433–437. doi: 10.5021/ad.2016.28.4.433
Hall, J., Jeggo, P. A., West, C., Gomolka, M., Quintens, R., Badie, C., et al. (2017). Ionizing radiation biomarkers in epidemiological studies—an update. Mutat. Res. 771, 59–84. doi: 10.1016/j.mrrev.2017.01.001
Hauptmann, M., Daniels, R. D., Cardis, E., Cullings, H. M., Kendall, G., Laurier, D., et al. (2020). Epidemiological studies of low-dose ionizing radiation and cancer: summary bias assessment and meta-analysis. J. Natl. Cancer Inst. Monogr. 2020, 188–200. doi: 10.1093/jncimonographs/lgaa010
Hong, G. U., Kim, N. G., and Ro, J. Y. (2014). Expression of airway remodeling proteins in mast cell activated by TGF-β released in OVA-induced allergic responses and their inhibition by low-dose irradiation or 8-oxo-dG. Radiat. Res. 181, 425–438. doi: 10.1667/RR13547.1
Horot, I. V., and Tkachenko, M. M. (2017). Peculiarities of ultrastructural organization and metabolism of reactive forms of oxygen and nitrogen in a cardiovascular system for permanent effects of ionizing radiation in low doses. Probl. Radiac. Med. Radiobiol. 22, 184–201. doi: 10.33145/2304-8336-2017-22-184-201
Howe, A., Kiffer, F., Alexander, T. C., Sridharan, V., Wang, J., Ntagwabira, F., et al. (2019). Long-term changes in cognition and physiology after low-dose 16O irradiation. Int. J. Mol. Sci. 20:188. doi: 10.3390/ijms20010188
Hu, Z. -P., Kim, Y. -M., Sowa, M. B., Robinson, R. J., Gao, X., Metz, T. O., et al. (2012). Metabolomic response of human skin tissue to low dose ionizing radiation. Mol. BioSyst. 8, 1979–1986. doi: 10.1039/c2mb25061f
Iizuka, D., Yoshioka, S., Kawai, H., Okazaki, E., Kiriyama, K., Izumi, S., et al. (2016). Hepcidin-2 in mouse urine as a candidate radiation-responsive molecule. J. Radiat. Res. 57, 142–149. doi: 10.1093/jrr/rrv098
Ishihara, H., Tanaka, I., Yakumaru, H., Tanaka, M., Yokochi, K., Fukutsu, K., et al. (2016). Quantification of damage due to low-dose radiation exposure in mice: construction and application of a biodosimetric model using mRNA indicators in circulating white blood cells. J. Radiat. Res. 57, 25–34. doi: 10.1093/jrr/rrv066
Jacquet, P., Buset, J., Neefs, M., and Vankerkom, J. (2008). Studies on the adaptive response in mouse female germ cells X-irradiated in vitro at two different stages of maturation. In Vivo 22, 179–186.
Jangiam, W., Udomtanakunchai, C., Reungpatthanaphong, P., Tungjai, M., Honikel, L., Gordon, C. R., et al. (2018). Late effects of low-dose radiation on the bone marrow, lung, and testis collected from the same exposed BALB/cJ mice. Dose-Response 16:1559325818815031. doi: 10.1177/1559325818815031
Jiang, X., Hong, Y., Zhao, D., Meng, X., Zhao, L., Du, Y., et al. (2017). Low dose radiation prevents doxorubicin-induced cardiotoxicity. Oncotarget 9, 332–345. doi: 10.18632/oncotarget.23013
Joo, H. M., Kang, S. J., Nam, S. Y., Yang, K. H., Kim, C. S., Lee, I. K., et al. (2015). The inhibitory effects of low-dose ionizing radiation in IgE-mediated allergic responses. PLoS One 10:e0136394. doi: 10.1371/journal.pone.0136394
Joo, H. M., Nam, S. Y., Yang, K. H., Kim, C. S., Jin, Y. W., and Kim, J. Y. (2012). The effects of low-dose ionizing radiation in the activated rat basophilic leukemia (RBL-2H3) mast cells. J. Biol. Chem. 287, 27789–27795. doi: 10.1074/jbc.M112.378497
Kaiser, J. C., Meckbach, R., Eidemüller, M., Selmansberger, M., Unger, K., Shpak, V., et al. (2016). Integration of a radiation biomarker into modeling of thyroid carcinogenesis and post-Chernobyl risk assessment. Carcinogenesis 37, 1152–1160. doi: 10.1093/carcin/bgw102
Kanagaraj, K., Abdul Syed Basheerudeen, S., Tamizh Selvan, G., Jose, M. T., Ozhimuthu, A., Panneer Selvam, S., et al. (2015). Assessment of dose and DNA damages in individuals exposed to low dose and low dose rate ionizing radiations during computed tomography imaging. Mutat. Res. Genet. Toxicol. Environ. Mutagen. 789–790, 1–6. doi: 10.1016/j.mrgentox.2015.05.008
Kaushik, N., Kim, M. -J., Kaushik, N. K., Myung, J. K., Choi, M. -Y., Kang, J. -H., et al. (2019). Low dose radiation regulates BRAF-induced thyroid cellular dysfunction and transformation. Cell Commun. Signal 17:12. doi: 10.1186/s12964-019-0322-x
Kawakita, Y., Ikekita, M., Kurozumi, R., and Kojima, S. (2003). Increase of intracellular glutathione by low-dose gamma-ray irradiation is mediated by transcription factor AP-1 in RAW 264.7 cells. Biol. Pharm. Bull. 26, 19–23. doi: 10.1248/bpb.26.19
Kim, C. S., Kim, J. -M., Nam, S. Y., Yang, K. H., Jeong, M., Kim, H. S., et al. (2007). Low-dose of ionizing radiation enhances cell proliferation via transient ERK1/2 and p38 activation in normal human lung fibroblasts. J. Radiat. Res. 48, 407–415. doi: 10.1269/jrr.07032
Kim, J. M., Kim, H. G., and Son, C. G. (2018). Tissue-specific profiling of oxidative stress-associated transcriptome in a healthy mouse model. Int. J. Mol. Sci. 19:3174. doi: 10.3390/ijms19103174
Kim, W., Lee, S., Seo, D., Kim, D., Kim, K., Kim, E., et al. (2019). Cellular stress responses in radiotherapy. Cell 8:1105. doi: 10.3390/cells8091105
Kim, J. S., Son, Y., Bae, M. J., Lee, S. S., Park, S. H., Lee, H. J., et al. (2015). Continuous exposure to low-dose-rate gamma irradiation reduces airway inflammation in ovalbumin-induced asthma. PLoS One 10:e0143403. doi: 10.1371/journal.pone.0143403
Kimler, B. F., Briley, S. M., Johnson, B. W., Armstrong, A. G., Jasti, S., and Duncan, F. E. (2018). Radiation-induced ovarian follicle loss occurs without overt stromal changes. Reproduction 155, 553–562. doi: 10.1530/REP-18-0089
Kreuzer, M., Auvinen, A., Cardis, E., Hall, J., Jourdain, J. R., Laurier, D., et al. (2015). Low-dose ionising radiation and cardiovascular diseases-strategies for molecular epidemiological studies in Europe. Mutat. Res. Rev. Mutat. Res. 764, 90–100. doi: 10.1016/j.mrrev.2015.03.002
Kurashige, T., Shimamura, M., and Nagayama, Y. (2017). N-acetyl-L-cysteine protects thyroid cells against DNA damage induced by external and internal irradiation. Radiat. Environ. Biophys. 56, 405–412. doi: 10.1007/s00411-017-0711-8
Lafon-Hughes, L., Di Tomaso, M. V., Méndez-Acuña, L., and Martínez-López, W. (2008). Chromatin-remodelling mechanisms in cancer. Mutat. Res. 658, 191–214. doi: 10.1016/j.mrrev.2008.01.008
Lanza, V., Fadda, P., Iannone, C., and Negri, R. (2007). Low-dose ionizing radiation stimulates transcription and production of endothelin by human vein endothelial cells. Radiat. Res. 168, 193–198. doi: 10.1667/RR0780.1
Lee, W. -C., Guntur, A. R., Long, F., and Rosen, C. J. (2017). Energy metabolism of the osteoblast: implications for osteoporosis. Endocr. Rev. 38, 255–266. doi: 10.1210/er.2017-00064
Lee, Y., Kim, Y. J., Choi, Y. J., Lee, J. W., Lee, S., Cho, Y. H., et al. (2015). Radiation-induced changes in DNA methylation and their relationship to chromosome aberrations in nuclear power plant workers. Int. J. Radiat. Biol. 91, 142–149. doi: 10.3109/09553002.2015.969847
Lee, E. K., Kim, J. A., Kim, J. S., Park, S. J., Heo, K., Yang, K. M., et al. (2013). Activation of de novo GSH synthesis pathway in mouse spleen after long term low-dose γ-ray irradiation. Free Radic. Res. 47, 89–94. doi: 10.3109/10715762.2012.747678
Lee, W. -J., Majumder, Z. R., Jeoung, D. -I., Lee, H. -J., Kim, S. -H., Bae, S., et al. (2006). Organ-specific gene expressions in C57BL/6 mice after exposure to low-dose radiation. Radiat. Res. 165, 562–569. doi: 10.1667/RR3549.1
Li, W., Wang, G., Cui, J., Xue, L., and Cai, L. (2004). Low-dose radiation (LDR) induces hematopoietic hormesis: LDR-induced mobilization of hematopoietic progenitor cells into peripheral blood circulation. Exp. Hematol. 32, 1088–1096. doi: 10.1016/j.exphem.2004.07.015
Li, J., Yao, Z. -Y., She, C., Li, J., Ten, B., Liu, C., et al. (2017). Effects of low-dose X-ray irradiation on activated macrophages and their possible signal pathways. PLoS One 12:e0185854. doi: 10.1371/journal.pone.0185854
Liang, X., Gu, J., Yu, D., Wang, G., Zhou, L., Zhang, X., et al. (2016). Low-dose radiation induces cell proliferation in human embryonic lung fibroblasts but not in lung cancer cells: importance of erk1/2 and akt signaling pathways. Dose-Response 14:1559325815622174. doi: 10.1177/1559325815622174
Liang, X., Zheng, S., Cui, J., Yu, D., Yang, G., Zhou, L., et al. (2018). Alterations of microRNA expression in the liver, heart, and testis of mice upon exposure to repeated low-dose radiation. Dose-Response 16:1559325818799561. doi: 10.1177/1559325818799561
Lieske, J. C., Kashani, K., Kellum, J., Koyner, J., Mehta, R., and Parikh, C. R. (2020). Use of biomarkers to detect and manage acute kidney injury: has progress stalled? Clin. Chem. 66, 271–276. doi: 10.1093/clinchem/hvz026
Lima, F., Swift, J. M., Greene, E. S., Allen, M. R., Cunningham, D. A., Braby, L. A., et al. (2017). Exposure to low-dose X-ray radiation alters bone progenitor cells and bone microarchitecture. Radiat. Res. 188, 433–442. doi: 10.1667/RR14414.1
Lowe, X. R., Bhattacharya, S., Marchetti, F., and Wyrobek, A. J. (2009). Early brain response to low-dose radiation exposure involves molecular networks and pathways associated with cognitive functions, advanced aging and Alzheimer’s disease. Radiat. Res. 171, 53–65. doi: 10.1667/rr1389.1
Manna, S. K., and Ramesh, G. T. (2005). Interleukin-8 induces nuclear transcription factor-kappaB through a TRAF6-dependent pathway. J. Biol. Chem. 280, 7010–7021. doi: 10.1074/jbc.M410994200
Maqsudur Rashid, A., Ramalingam, L., Al-Jawadi, A., Moustaid-Moussa, N., and Moussa, H. (2019). Low dose radiation, inflammation, cancer and chemoprevention. Int. J. Radiat. Biol. 95, 506–515. doi: 10.1080/09553002.2018.1484194
Mathias, D., Mitchel, R. E. J., Barclay, M., Wyatt, H., Bugden, M., Priest, N. D., et al. (2015). Low-dose irradiation affects expression of inflammatory markers in the heart of ApoE -/- mice. PLoS One 10:e0119661. doi: 10.1371/journal.pone.0119661
Meeren, A. V., Bertho, J. M., Vandamme, M., and Gaugler, M. H. (1997). Ionizing radiation enhances IL-6 and IL-8 production by human endothelial cells. Mediat. Inflamm. 6, 185–193. doi: 10.1080/09629359791677
Mojena, M., Pimentel-Santillana, M., Povo-Retana, A., Fernández-García, V., González-Ramos, S., Rada, P., et al. (2018). Protection against gamma-radiation injury by protein tyrosine phosphatase 1B. Redox Biol. 17, 213–223. doi: 10.1016/j.redox.2018.04.018
Moloney, J. N., and Cotter, T. G. (2018). ROS signalling in the biology of cancer. Semin. Cell Dev. Biol. 80, 50–64. doi: 10.1016/j.semcdb.2017.05.023
Mouw, K. W., Goldberg, M. S., Konstantinopoulos, P. A., and D’Andrea, A. D. (2017). DNA damage and repair biomarkers of immunotherapy response. Cancer Discov. 7, 675–693. doi: 10.1158/2159-8290.CD-17-0226
Multhoff, G., Pockley, A. G., Schmid, T. E., and Schilling, D. (2015). The role of heat shock protein 70 (Hsp70) in radiation-induced immunomodulation. Cancer Lett. 368, 179–184. doi: 10.1016/j.canlet.2015.02.013
Nadol’nik, L. I., Kardash, N. A., Martynchik, D. I., Kravchuk, R. I., Netsetskaia, Z. V., Basinskiĭ, V. A., et al. (2005). The effect of one-time external low‐ and high-dose gamma-irradiation on rat thyroid structure at the acute and remote periods. Radiats. Biol. Radioecol. 45, 433–441.
Nadol’nik, L. I., Netsetskaia, Z. V., and Vinogradov, V. V. (2003). Effects of single-dose external gamma irradiation on rat thyroid status as observed during the year. Radiats. Biol. Radioecol. 43, 65–70.
Nagayama, Y., Ichikawa, T., Saitoh, O., and Abiru, N. (2009). Induction of late-onset spontaneous autoimmune thyroiditis by a single low-dose irradiation in thyroiditis-prone non-obese diabetic-H2h4 mice. J. Radiat. Res. 50, 573–577. doi: 10.1269/jrr.09067
Nagayama, Y., Kaminoda, K., Mizutori, Y., Saitoh, O., and Abiru, N. (2008). Exacerbation of autoimmune thyroiditis by a single low dose of whole-body irradiation in non-obese diabetic-H2h4 mice. Int. J. Radiat. Biol. 84, 761–769. doi: 10.1080/09553000802345910
Novershtern, N., Subramanian, A., Lawton, L. N., Mak, R. H., Haining, W. N., McConkey, M. E., et al. (2011). Densely interconnected transcriptional circuits control cell states in human hematopoiesis. Cell 144, 296–309. doi: 10.1016/j.cell.2011.01.004
Orekhova, N. A., and Modorov, M. V. (2017). East urals radioactive trace: dose-dependent functional-metabolic effects in the myocardium of the pygmy wood mouse (Apodemus uralensis) taking into account population size. J. Environ. Radioact. 175–176, 15–24. doi: 10.1016/j.jenvrad.2017.04.005
Park, G., Son, B., Kang, J., Lee, S., Jeon, J., Kim, J. -H., et al. (2019). LDR-induced miR-30a and miR-30b target the PAI-1 pathway to control adverse effects of NSCLC radiotherapy. Mol. Ther. 27, 342–354. doi: 10.1016/j.ymthe.2018.10.015
Pavlov, A. V., Ermakova, O. V., Korableva, T. V., and Raskosha, O. V. (2013). Morphometric analysis of follicular structure of the thyroid gland after chronic low-dose gamma-irradiation. Morfologiia 143, 43–46.
Pearce, M. S., Salotti, J. A., Little, M. P., McHugh, K., Lee, C., Kim, K. P., et al. (2012). Radiation exposure from CT scans in childhood and subsequent risk of leukaemia and brain tumours: a retrospective cohort study. Lancet 380, 499–505. doi: 10.1016/S0140-6736(12)60815-0
Prasad, A. V., Mohan, N., Chandrasekar, B., and Meltz, M. L. (1994). Activation of nuclear factor kappa B in human lymphoblastoid cells by low-dose ionizing radiation. Radiat. Res. 138, 367–372.
Puck, T. T., Johnson, R., Webb, P., Cui, H., Valdez, J. G., and Crissman, H. (2002). Mutagenesis and repair by low doses of alpha radiation in mammalian cells. Proc. Natl. Acad. Sci. U. S. A. 99, 12220–12223. doi: 10.1073/pnas.152433699
Puukila, S., Muise, S., McEvoy, J., Bouchier, T., Hooker, A. M., Boreham, D. R., et al. (2019). Acute pulmonary and splenic response in an in vivo model of whole-body low-dose X-radiation exposure. Int. J. Radiat. Biol. 95, 1072–1084. doi: 10.1080/09553002.2019.1625459
Rödel, F., Fournier, C., Wiedemann, J., Merz, F., Gaipl, U. S., Frey, B., et al. (2017). Basics of radiation biology when treating hyperproliferative benign diseases. Front. Immunol. 8:519. doi: 10.3389/fimmu.2017.00519
Rödel, F., Hantschel, M., Hildebrandt, G., Schultze-Mosgau, S., Rödel, C., Herrmann, M., et al. (2004). Dose-dependent biphasic induction and transcriptional activity of nuclear factor kappa B (NF-kappaB) in EA.hy.926 endothelial cells after low-dose X-irradiation. Int. J. Radiat. Biol. 80, 115–123. doi: 10.1080/09553000310001654701
Roedel, F., Kley, N., Beuscher, H. U., Hildebrandt, G., Keilholz, L., Kern, P., et al. (2002). Anti-inflammatory effect of low-dose X-irradiation and the involvement of a TGF-beta1-induced down-regulation of leukocyte/endothelial cell adhesion. Int. J. Radiat. Biol. 78, 711–719. doi: 10.1080/09553000210137671
Romanenko, A. M., Ruiz-Saurí, A., Morell-Quadreny, L., Valencia, G., Vozianov, A. F., and Llombart-Bosch, A. (2012). Microvessel density is high in clear-cell renal cell carcinomas of Ukrainian patients exposed to chronic persistent low-dose ionizing radiation after the Chernobyl accident. Virchows Arch. 460, 611–619. doi: 10.1007/s00428-012-1243-x
Rönnbäck, C. (1983). Effects on foetal ovaries after protracted, external gamma irradiation as compared with those from internal depositions. Acta Radiol. Oncol. 22, 465–471. doi: 10.3109/02841868309135972
Russo, G. L., Tedesco, I., Russo, M., Cioppa, A., Andreassi, M. G., and Picano, E. (2012). Cellular adaptive response to chronic radiation exposure in interventional cardiologists. Eur. Heart J. 33, 408–414. doi: 10.1093/eurheartj/ehr263
Sak, A., Kübler, D., Bannik, K., Groneberg, M., and Stuschke, M. (2015). Dependence of radiation-induced H2AX phosphorylation on histone methylation: evidence from the chromatin immunoprecipitation assay. Int. J. Radiat. Biol. 91, 346–353. doi: 10.3109/09553002.2015.997895
Sándor, N., Schilling-Tóth, B., Kis, E., Benedek, A., Lumniczky, K., Sáfrány, G., et al. (2015). Growth differentiation factor-15 (GDF-15) is a potential marker of radiation response and radiation sensitivity. Mutat. Res. Genet. Toxicol. Environ. Mutagen. 793, 142–149. doi: 10.1016/j.mrgentox.2015.06.009
Schröder, S., Juerß, D., Kriesen, S., Manda, K., and Hildebrandt, G. (2019). Immunomodulatory properties of low-dose ionizing radiation on human endothelial cells. Int. J. Radiat. Biol. 95, 23–32. doi: 10.1080/09553002.2018.1486515
Seawright, J. W., Samman, Y., Sridharan, V., Mao, X. W., Cao, M., Singh, P., et al. (2017). Effects of low-dose rate γ-irradiation combined with simulated microgravity on markers of oxidative stress, DNA methylation potential, and remodeling in the mouse heart. PLoS One 12:e0180594. doi: 10.1371/journal.pone.0180594
She, C., Shi, G. -L., Xu, W., Zhou, X. -Z., Li, J., Tian, Y., et al. (2016). Effect of low-dose X-ray irradiation and Ti particles on the osseointegration of prosthetic. J. Orthop. Res. 34, 1688–1696. doi: 10.1002/jor.23179
Shore, R., Walsh, L., Azizova, T., and Rühm, W. (2017). Risk of solid cancer in low dose-rate radiation epidemiological studies and the dose-rate effectiveness factor. Int. J. Radiat. Biol. 93, 1064–1078. doi: 10.1080/09553002.2017.1319090
Siegel, J. A., Pennington, C. W., and Sacks, B. (2017). Subjecting radiologic imaging to the linear no-threshold hypothesis: a non sequitur of non-trivial proportion. J. Nucl. Med. 58, 1–6. doi: 10.2967/jnumed.116.180182
Silasi, G., Diaz-Heijtz, R., Besplug, J., Rodriguez-Juarez, R., Titov, V., Kolb, B., et al. (2004). Selective brain responses to acute and chronic low-dose X-ray irradiation in males and females. Biochem. Biophys. Res. Commun. 325, 1223–1235. doi: 10.1016/j.bbrc.2004.10.166
Sinnott, B., Ron, E., and Schneider, A. B. (2010). Exposing the thyroid to radiation: a review of its current extent, risks, and implications. Endocr. Rev. 31, 756–773. doi: 10.1210/er.2010-0003
Sjaarda, J., Gerstein, H., Chong, M., Yusuf, S., Meyre, D., Anand, S. S., et al. (2018). Blood CSF1 and CXCL12 as causal mediators of coronary artery disease. J. Am. Coll. Cardiol. 72, 300–310. doi: 10.1016/j.jacc.2018.04.067
Son, B., Lee, S., Kim, H., Kang, H., Kim, J., Youn, H., et al. (2019). Low dose radiation attenuates inflammation and promotes wound healing in a mouse burn model. J. Dermatol. Sci. 96, 81–89. doi: 10.1016/j.jdermsci.2019.10.004
Song, C. -H., Joo, H. M., Han, S. H., Kim, J. -I., Nam, S. Y., and Kim, J. Y. (2019). Low-dose ionizing radiation attenuates mast cell migration through suppression of monocyte chemoattractant protein-1 (MCP-1) expression by Nr4a2. Int. J. Radiat. Biol. 95, 1498–1506. doi: 10.1080/09553002.2019.1642535
Song, K. -H., Kim, M. -H., Kang, S. -M., Jung, S. -Y., Ahn, J., Woo, H. -J., et al. (2015). Analysis of immune cell populations and cytokine profiles in murine splenocytes exposed to whole-body low-dose irradiation. Int. J. Radiat. Biol. 91, 795–803. doi: 10.3109/09553002.2015.1068461
Souchkevitch, G., and Lyasko, L. (1997). Investigation of the impact of radiation dose on hormones, biologically active metabolites and immunoglobulins in Chernobyl accident recovery workers. Stem Cells 15(Suppl. 2), 151–154. doi: 10.1002/stem.5530150722
Sudprasert, W., Navasumrit, P., and Ruchirawat, M. (2006). Effects of low-dose gamma radiation on DNA damage, chromosomal aberration and expression of repair genes in human blood cells. Int. J. Hyg. Environ. Health 209, 503–511. doi: 10.1016/j.ijheh.2006.06.004
Sypin, V. D., Osipov, A. N., Elakov, A. L., Pomerantseva, M. D., Zaichkina, S. I., Rozanova, O. M., et al. (2003). Estimation of genetic effects of chronic exposure to low-dose rate gamma-radiation by cytogenetic methods and DNA-comet assay. Radiats. Biol. Radioecol. 43, 156–160.
Tabe, Y., Hatanaka, Y., Nakashiro, M., Sekihara, K., Yamamoto, S., Matsushita, H., et al. (2016). Integrative genomic and proteomic analyses identifies glycerol-3-phosphate acyltransferase as a target of low-dose ionizing radiation in EBV infected-B cells. Int. J. Radiat. Biol. 92, 24–34. doi: 10.3109/09553002.2015.1106021
Tajima, S., Yamamoto, N., and Masuda, S. (2019). Clinical prospects of biomarkers for the early detection and/or prediction of organ injury associated with pharmacotherapy. Biochem. Pharmacol. 170:113664. doi: 10.1016/j.bcp.2019.113664
Thomas, C. B., Nelson, D. O., Pleshanov, P., and Jones, I. M. (2002). Induction and decline of HPRT mutants and deletions following a low dose radiation exposure at Chernobyl. Mutat. Res. 499, 177–187. doi: 10.1016/s0027-5107(01)00272-x
Uehara, Y., Ito, Y., Taki, K., Nenoi, M., Ichinohe, K., Nakamura, S., et al. (2010). Gene expression profiles in mouse liver after long-term low-dose-rate irradiation with gamma rays. Radiat. Res. 174, 611–617. doi: 10.1667/RR2195.1
Veeraraghavan, J., Natarajan, M., Herman, T. S., and Aravindan, N. (2011). Low-dose γ-radiation-induced oxidative stress response in mouse brain and gut: regulation by NFκB-MnSOD cross-signaling. Mutat. Res. 718, 44–55. doi: 10.1016/j.mrgentox.2010.10.006
Velegzhaninov, I. O., Ermakova, A. V., and Klokov, D. Y. (2018). Low dose ionizing irradiation suppresses cellular senescence in normal human fibroblasts. Int. J. Radiat. Biol. 94, 825–828. doi: 10.1080/09553002.2018.1492167
Visweswaran, S., Joseph, S., Vinay Hedge, S., Annalakshmi, O., Jose, M. T., and Perumal, V. (2019). DNA damage and gene expression changes in patients exposed to low-dose X-radiation during neuro-interventional radiology procedures. Mutat. Res. 844, 54–61. doi: 10.1016/j.mrgentox.2019.05.011
Voisard, R., Wiegmann, D., Baur, R., Hombach, V., and Kamenz, J. (2007). Low-dose irradiation stimulates TNF-alpha-induced ICAM-1 mRNA expression in human coronary vascular cells. Med. Sci. Monit. 13, BR107–BR111.
Wan, H., Gong, S. L., and Liu, S. Z. (2001). Effects of low dose radiation on signal transduction of neurons in mouse hypothalamus. Biomed. Environ. Sci. 14, 248–255.
Wang, Z. -C., Wang, J. -F., Li, Y. -B., Guo, C. -X., Liu, Y., Fang, F., et al. (2013). Involvement of endoplasmic reticulum stress in apoptosis of testicular cells induced by low-dose radiation. J. Huazhong Univ. Sci. Technolog. Med. Sci. 33, 551–558. doi: 10.1007/s11596-013-1157-0
Wang, P., Zhang, H., Li, Z., Liu, X., Jin, Y., Lei, M., et al. (2019). Low-dose radiation promotes the proliferation and migration of AGE-treated endothelial progenitor cells derived from bone marrow via activating SDF-1/CXCR4/ERK signaling pathway. Radiat. Res. 191, 518–526. doi: 10.1667/RR15200.1
Wu, B., Wei, Y., Liu, F. -Q., Zhang, Q., Wang, C. -B., and Bai, H. (2011). Biological effects of low dose X-irradiation on human bone marrow mesenchymal stem cells. Zhongguo Shi Yan Xue Ye Xue Za Zhi 19, 1214–1217.
Xu, W., Xu, L., Chen, M., Mao, Y. T., Xie, Z. G., Wu, S. L., et al. (2012). The effects of low dose X-irradiation on osteoblastic MC3T3-E1 cells in vitro. BMC Musculoskelet. Disord. 13:94. doi: 10.1186/1471-2474-13-94
Yang, L., Liu, Z., Chen, C., Cong, X., Li, Z., Zhao, S., et al. (2017). Low-dose radiation modulates human mesenchymal stem cell proliferation through regulating CDK and Rb. Am. J. Transl. Res. 9, 1914–1921.
Yoshida, N., Imada, H., Kunugita, N., and Norimura, T. (1993). Low dose radiation-induced adaptive survival response in mouse spleen T-lymphocytes in vivo. J. Radiat. Res. 34, 269–276. doi: 10.1269/jrr.34.269
Yu, Q., Katlinskaya, Y. V., Carbone, C. J., Zhao, B., Katlinski, K. V., Zheng, H., et al. (2015). DNA-damage-induced type I interferon promotes senescence and inhibits stem cell function. Cell Rep. 11, 785–797. doi: 10.1016/j.celrep.2015.03.069
Zhang, J., He, Y., Shen, X., Jiang, D., Wang, Q., Liu, Q., et al. (2016b). γ-H2AX responds to DNA damage induced by long-term exposure to combined low-dose-rate neutron and γ-ray radiation. Mutat. Res. Genet. Toxicol. Environ. Mutagen. 795, 36–40. doi: 10.1016/j.mrgentox.2015.11.004
Zhang, C., Jin, S., Guo, W., Li, C., Li, X., Rane, M. J., et al. (2011). Attenuation of diabetes-induced cardiac inflammation and pathological remodeling by low-dose radiation. Radiat. Res. 175, 307–321. doi: 10.1667/RR1950.1
Zhang, F., Lin, X., Yu, L., Li, W., Qian, D., Cheng, P., et al. (2016a). Low-dose radiation prevents type 1 diabetes-induced cardiomyopathy via activation of AKT mediated anti-apoptotic and anti-oxidant effects. J. Cell. Mol. Med. 20, 1352–1366. doi: 10.1111/jcmm.12823
Zhang, X., and Mosser, D. M. (2008). Macrophage activation by endogenous danger signals. J. Pathol. 214, 161–178. doi: 10.1002/path.2284
Keywords: low-dose radiation, human, animal model, organ-specificity, biological marker
Citation: Shin E, Lee S, Kang H, Kim J, Kim K, Youn H, Jin YW, Seo S and Youn B (2020) Organ-Specific Effects of Low Dose Radiation Exposure: A Comprehensive Review. Front. Genet. 11:566244. doi: 10.3389/fgene.2020.566244
Edited by:
Giovanni Cenci, Sapienza University of Rome, ItalyReviewed by:
Daniel Vaiman, Institut National de la Santé et de la Recherche Médicale (INSERM), FranceIgor Kovalchuk, University of Lethbridge, Canada
Copyright © 2020 Shin, Lee, Kang, Kim, Kim, Youn, Jin, Seo and Youn. This is an open-access article distributed under the terms of the Creative Commons Attribution License (CC BY). The use, distribution or reproduction in other forums is permitted, provided the original author(s) and the copyright owner(s) are credited and that the original publication in this journal is cited, in accordance with accepted academic practice. No use, distribution or reproduction is permitted which does not comply with these terms.
*Correspondence: BuHyun Youn, Ymh5b3VuNzJAcHVzYW4uYWMua3I=
†These authors have contributed equally to this work