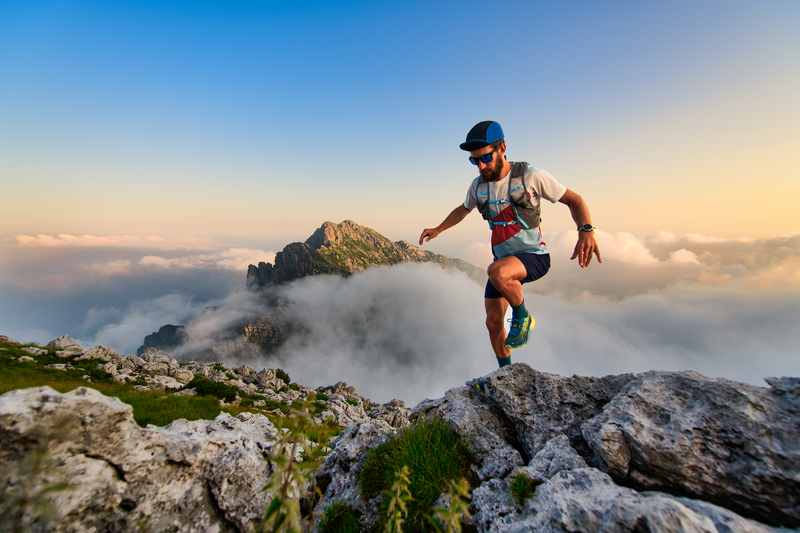
94% of researchers rate our articles as excellent or good
Learn more about the work of our research integrity team to safeguard the quality of each article we publish.
Find out more
ORIGINAL RESEARCH article
Front. Genet. , 25 September 2020
Sec. Evolutionary and Genomic Microbiology
Volume 11 - 2020 | https://doi.org/10.3389/fgene.2020.530915
This article is part of the Research Topic Genomic Characterization of Emerging Human Fungal Pathogens View all 11 articles
Candida species are important pathogens of humans and the fourth most commonly isolated pathogen from nosocomial blood stream infections. Although Candida albicans is the principle causative agent of invasive candidosis, the incidence of Candida glabrata infections has rapidly grown. The reason for this increase is not fully understood, but it is clear that the species has a higher innate tolerance to commonly administered azole antifungals, in addition to being highly tolerant to stresses especially oxidative stress. Taking the approach that using the model organism, Saccharomyces cerevisiae, with its intrinsic sensitivity to oxidative stress, we hypothesized that by expressing mediators of stress resistance from C. glabrata in S. cerevisiae, it would result in induced resistance. To test this we transformed, en-masse, the C. glabrata ORFeome library into S. cerevisiae. This resulted in 1,500 stress resistant colonies and the recovered plasmids of 118 ORFs. Sequencing of these plasmids revealed a total of 16 different C. glabrata ORFs. The recovery of genes encoding known stress protectant proteins such as GPD1, GPD2 and TRX3 was predicted and validated the integrity of the screen. Through this screen we identified a C. glabrata unique ORF that confers oxidative stress resistance. We set to characterise this gene herein, examining expression in oxidative stress sensitive strains, comet assays to measure DNA damage and synthetic genetic array analysis to identify genetic interaction maps in the presence and absence of oxidative stress.
Biological systems function in constantly changing complex environments, where they are subject to wide ranging perturbations. Their ability to adapt to these perturbations is essential for all life and understanding the mechanisms that underpin these adaptations are of fundamental biological interest. Although, Candida albicans is the principle causative agent of invasive candidosis, the incidence of Candida glabrata infection has grown rapidly over the last 20 years, and it is now responsible for approximately 25% of cases (Hajjeh et al., 2004). The reason for this increasing incidence of C. glabrata infection is not fully understood. The molecular mechanisms underpinning the response to stress is relatively well characterized in a number of model and pathogenic species (Gellon et al., 2001; Lackner et al., 2012; Boiteux and Jinks-Robertson, 2013; Miramón et al., 2013; Storr et al., 2013), but remains relatively unexplored in C. glabrata.
When engulfed by phagocytic cells pathogenic fungi are submitted to combinations of stress that cause oxidative damage, which if not repaired can result in cell death (Fang, 2004). C. glabrata is highly resistant to oxidative stress conditions, having evolved capabilities to cope with such stress (Nikolaou et al., 2009; Roetzer et al., 2011). C. glabrata can persist for long periods as a human commensal, and live cells can be isolated weeks after infection (Brieland et al., 2001; Jacobsen et al., 2010), clearly demonstrating that C. glabrata can adapt to the stresses encountered within the host. Indeed, C. glabrata has been shown to survive and multiply inside murine phagocytic cells (Kaur et al., 2007; Roetzer et al., 2008; Seider et al., 2011). When engulfed by phagocytes, C. glabrata cells are exposed to reactive oxygen species (ROS) plus cationic fluxes activated by intracellular ion currents (Fang, 2004). The mechanistic basis of C. glabrata stress resistance is not understood but genes encoding functions in cell wall biosynthesis, nutrient acquisition, metal (calcium and iron) ion homeostasis and stress response have been implicated by functional genomics (Seider et al., 2014). Previous studies have demonstrated that the C. glabrata response to in vitro exerted oxidative stress is very similar to that observed upon phagocyte engulfment, both at the level of gene expression (Kaur et al., 2007; Fukuda et al., 2013), where the up-regulation of genes encoding functions related to stress adaptation and nutrient recycling overlap substantially, and in growth kinetics (Kaloriti et al., 2012) where in both environments, approximately 20% of C. glabrata cells survive initial contact with a substantial delay occurring prior to growth re-commencing. These data sets demonstrate that in vitro oxidative stress is a realistic model of host-induced stress and the ability to survive oxidative stress is an important virulence determinant for pathogens. Understanding this network, and the role that selected components play in stress resistance, is essential to the long-term development of small molecule inhibitors.
Oxidative stress is generated by normal cellular metabolism or produced via exogenous chemicals (such as hydrogen peroxide) and can induce several types of DNA damage including DNA base damage and the formation of apurinic/apyrimidinic (AP) sites, which are thought to be processed primarily through the base excision repair (BER) pathway (Eide et al., 1996; Swanson et al., 1999). In the BER pathway, a damaged base is removed by a specific N-glycosylase (Ntg1 and Ntg2), (Alseth et al., 1999; Meadows et al., 2003; Griffiths et al., 2009), and the resulting AP site is cleaved by an AP endonuclease. Following the processing of the 5′ terminus by deoxyribose phosphodiesterase, DNA polymerase fills in the gap and DNA ligase seals the ends together (Supplementary Figure 1). The other main DNA repair pathway is the nucleotide excision repair (NER) pathway, which generally removes bulky DNA lesions and is also implicated in the repair of oxidative stress damage (Huang et al., 1994; Boiteux and Jinks-Robertson, 2013). The damaged DNA bases are removed by introducing nicks 5′ and 3′ to the DNA damage. The 3′ incision is produced by RAD2 and the 5′ incision by the RAD1-RAD10 complex (Habraken et al., 1993). Once the oligonucleotide including the damaged DNA is removed, DNA polymerase fills in the gap and DNA ligase joins the ends (Supplementary Figure 1). Recombination is involved in the repair of single or double stranded breaks. Cells deficient in the Rad52 epistasis group of proteins are highly sensitive to the killing effects of agents that produce strand breaks (Mortensen et al., 1996; Pâques and Haber, 1999; Symington, 2002). Recombination rates are known to increase upon exposure to hydrogen peroxide, playing a role for recombination in response to oxidative induced DNA damage. There is an overlap between the different DNA repair mechanisms and DNA damage tolerance pathways in the processing of oxidative DNA damage (Giglia-Mari et al., 2011).
In the model yeast Saccharomyces cerevisiae, the removal of oxidative stress induced damage is thought to be conducted primarily through the base excision pathway (Farrugia and Balzan, 2012). The main genes involved are NTG1 and NTG2, N-glycosylase-associated apurinic/apyrimidinic lyases that recognize a wide variety of damaged pyrimidines and APN1, a major AP endonuclease in yeast, with the majority of AP sites in vivo processed via this pathway (Ramotar et al., 1991; Ildiko et al., 2000; Ishchenko et al., 2005). In general, the majority of yeast cells lacking these three genes are hyper-recombinogenic and exhibit a mutator phenotype but are not sensitive to all oxidative stress chemicals. However, the additional disruption of RAD52 confers a high degree of sensitivity to oxidative stress damage by eliminating the nucleotide excision repair pathway.
In this study, we transformed S. cerevisiae cells, which are exquisitely sensitive to oxidative stress, with a C. glabrata genomic library and isolated oxidative stress resistant clones and identified the genes that encoded mediators of oxidative stress resistance. We have identified a novel C. glabrata protein, CAGL0G06710g, designated ORI1 (Oxidative stress Resistance Increased) for this work, (Table 1) which can confer oxidative stress resistance in S. cerevisiae. ORI1 is a C. glabrata specific protein, which has no homology to proteins encoded in any other sequenced genome. We hypothesized that this gene plays a role in oxidative stress resistance in C. glabrata via comet assays and genetically interacts with genes playing a role in the base excision via SGA screens in the presence and absence of oxidative stress (H2O2) and nucleotide excision DNA damage repair pathways.
The C. glabrata ORFeome (Ho and Huvet, unpublished), was generated using the Gateway cloning system, whereby pENTRY plasmids were generated for each ORF in the C. glabrata genome and shuttled to destination vectors of interest using the LR clonase protocol, for this work the destination plasmid used was pAG423GPD-ccdB, a constitutive promoter with HIS3 selection (Ho and Haynes, 2015). The C. glabrata ORFeome was pooled and transformed en mass into S. cerevisiae strain BY4741, using the lithium acetate method (Gietz and Schiestl, 2007). Transformants were recovered from selective media plates containing 5 mM hydrogen peroxide, a concentration known to inhibit growth in S. cerevisiae. The individual colonies were isolated and grown on fresh media containing 5 mM hydrogen peroxide to remove any break through colonies. Using the QIAprep Spin miniprep kit (Qiagen), the plasmid DNA was recovered and sent for sequencing to identify the C. glabrata ORFs conferring stress tolerance.
The KanMX cassette was amplified from plasmid pFA6-kanMX4 with complementary up and down stream sequences for ORI1, (Supplementary Table 1 for primers used), using the following conditions for a 50 μl reaction; 1× Taq buffer, 0.2 μM dNTPs, 0.5 μM of each primer, 1 μl Taq-polymerase and plasmid DNA; 93°C for 3 min, with 30 cycles of 93°C for 30 s, 50°C for 30 s, 72°C for 2 min with 10 min at 72°C. The fragments were gel-purified in 0.7% agarose gels and the final deletion construct purified via ethanol precipitation.
Candida glabrata CAGL0G06710g was cloned into the pDONR221 entry vector using GATEWAY cloning techniques and were shuttled into pAG423GPD-ccdB destination vector (Addgene) using the LR clonase reaction. Destination vectors were transformed into C. glabrata via electroporation (Istel et al., 2015) and S. cerevisiae cells via the LiAc transformation method (Gietz and Schiestl, 2007). The correct transformants were selected for growth on SC-HIS media. Three independent transformants of each strain were collected.
Aliquots of 106cells/ml C. glabrata and S. cerevisiae cells were collected by centrifugation and mixed with 1.5% low melting point agarose (w/v) in S buffer containing 2 mg/ml zymolyase (20T), 80 μl of this mixture was spread over a slide coated in 0.5% normal melting point agarose (w/v) and covered with a coverslip and incubated for 20 min at 30°C for cell wall enzymatic degradation, following which the cover slips were removed. All remaining steps were performed at 4°C. The slides were incubated in lysis solution (30 mM NaOK, 1M NaCl, 0.05% lauryl sarcosine (w/v), 50 mM EDTA, 10 mM Tris–HCl, pH10) for 20 min in order to lyse spheroplasts. The slides were then washed three times for 20 min each in electrophoresis buffer (30 mM NaOH, 10 mM Tris–HCl, pH10) to remove the lysis solution. The slides were then electrophoresis in the same buffer for 10 min at 0.7V/cm. After electrophoresis, the slides were incubated in neutralization buffer (10 mM Tris–HCl, pH7.4) for 10 min, followed by 10 min in 76 and 96% ethanol, respectively. The slides were then stained with ethidium bromide (10 μg/ml) and visualized using a Leica microsystem DM fluorescence microscope. Each condition was repeated in triplicate with 20 representative images of each slide acquired. The images were analyzed using Comet Score software.
Synthetic Genetic Array (SGA) screens were performed in triplicate with double spotting within each triplicate. The S. cerevisiae deletion mutant library was arrayed using a Singer RoToR HDA (Singer Instruments). For the genome-wide synthetic lethal SGA screens (SL-SGA) the MATα query strain Y70921 was transformed with the C. glabrata gene of interest, ORI1. The resulting query strain was mated with the S. cerevisiae MATa deletion mutant array library and SGA methodology was used (Hin et al., 2001; Measday et al., 2005). For the screens in the presence of stress, the last plate of the screen (double mutant plates) was then spotted onto media containing oxidative stress (2 mM H2O2) and incubated for 24 h at 30°C. To identify deletion mutants that showed growth defects due to the expression of the C. glabrata genes, all screens were performed in triplicate. Growth was scored for three main criteria, slow growth (SS), lethality (SL or suppression S, improved growth) after 24 h on the final DMA plates. For verification, any potential genetic interactions had to be identified in a minimum of two out of the three replicated in any screen.
Dot assays were performed by spotting 5 μl of 10-fold serial dilutions (OD600 = 0.1, 0.01, 0.001, 0.0001) onto specified media, and sealed plates were incubated at 30°C for 24 h. All dot assay experiments were repeated in triplicate using three different isolates of each strain.
Saccharomyces cerevisiae is exquisitely sensitive to oxidative stress. We hypothesized that expression of effectors of C. glabrata stress resistance in S. cerevisiae would lead to increased oxidative stress resistance. We transformed, en-bloc, our recently constructed C. glabrata partial ORFeome (∼3,000 genes) into S. cerevisiae strain BY4741 and screened the transformants on media containing the oxidative stress agent, hydrogen peroxide (5 mM). From this we identified 1,500 stress resistant colonies, which were then individually regrown on hydrogen peroxide containing media to remove break-out colonies. The plasmids were recovered from each of these and their C. glabrata ORFS identified by sequencing. A total of 16 C. glabrata ORFs were isolated (Table 1). Recovery of genes encoding known stress protectant proteins e.g., GPD1, GPD2, and TRX3 (Albertyn et al., 1994; Trotter and Grant, 2005; Tkach et al., 2012) were predicted and validated the integrity of the screen. In addition, we identified a number of genes encoding proteins with general roles in transcription/translation, epigenetic modification and endosomal sorting e.g., BRX1, CET1, HAT1, RPB7, SNT309, and VPS28 whose over-expression are likely to have pleiotropic effects and hence deciphering a specific role in stress resistance would be extremely difficult.
Finally, we isolated four C. glabrata specific ORFs, encoding proteins of unknown function, CAGL0G06710g, CAGL0E06094g, CAGL0H04059g, and CAGL0A00649g (Table 1). We have taken ORF CAGL0G6710g forward for further investigation and for the purpose of this work refer to it as ORI1 (Oxidative Stress Resistance Increased). It was isolated in 34/85 of the stress resistant mutants.
To confirm that the expression of ORI1 conferred oxidative stress protection in C. glabrata, following its identification in the S. cerevisiae screen, we generated an ORI1 deletion strain and over-expression strain in C. glabrata wild-type strain CBS138. This also confirmed that the gene is not essential for viability. The strains were then screened on oxidative (2 mM hydrogen peroxide), osmotic (1.5 M sodium chloride) and combinatorial stress plates [oxidative (H2O2) and osmotic (NaCl)] (Figure 1). The C. glabrataΔori1 mutants are sensitive to combinatorial stress, whereas the ORI1 over-expressing strains had an increased combinatorial stress tolerance but most striking was the increased tolerance to oxidative stress. This demonstrated a potential role for ORI1 in mediating C. glabrata stress resistance.
Figure 1. Spot plate assay of ORI1 on stress media. C. glabrata wild-type, Ori1 (CAGL0G06710g) deletion and overexpression strains. Strains were serially diluted and 5 μl spots were placed onto minimal media containing either oxidative stress (2 mM H2O2), Osmotic stress (0.5 M NaCl) and combinatorial stress (2 mM H2O2 and 0.5 M NaCl). The plates were then incubated for 24 h at 37°C. Each spot assay was performed in triplicate.
Following from the spot assay, we utilized Comet assays as an uncomplicated and sensitive method to detect DNA damage at the level of individual cells. The term “comet” refers to the pattern of DNA migration through the electrophoresis gel after exposure to a DNA damaging agent, in this study, hydrogen peroxide. The intensity of the comet tail relative to the head reflects the number of DNA strand breaks. The basis for this is that loops containing a break lose their supercoiling and become free to extend toward the anode during electrophoresis. We examined C. glabrata and S. cerevisiae wild-type strains and ones over-expressing ORI1 (Figure 2). As can be clearly seen the over-expression on ORI1 offers protection from the DNA damage induced by the oxidative stressor H2O2 at a range of different concentrations when compared to the level of damage experienced by the wild-type cells due to the shortened tail lengths observed. This effect was most pronounced in the S. cerevisiae cells overexpressing ORI1. This may be due to the fact that S. cerevisiae is sensitive to oxidative stress. Control cells (i.e., no exposure to hydrogen peroxide) were also observed to display comet-like features, this is likely due to initial DNA damage present, replication forks or damage induced by the handling of cells during preparation of comets. The use of this methodology is interesting, as the combination of yeast cells, either pathogenic or non-pathogenic and the comet assay is an extremely accessible technique for genotoxicity testing.
Figure 2. Comet assay on C. glabrata and S. cerevisiae wild-type and ORI1 overexpressing strains. Comet assays were performed on (A) S. cerevisiae wild-type cells, (B) C. glabrata wild-type cells, and (C) S. cerevisiae cells overexpressing ORI1 in the presence of no stress (No Txt in figure), 5 mM H2O2 and 10 mM H2O2. DNA damage was measured in 100 comets for each strain time for each condition and analysis with Comet Assay V4.0. (D) The DNA damage is represented as a mean ratio of tail length to head intensity allowing for positional variations in intensity. 100 comets were scored per experiment for each concentration.
Synthetic Genetic Array screens enable the construction of double mutants on a genome wide scale and as such this trend for large scale genetic screens reveal a wealth of genetic interaction information on biological systems. Therefore we performed unbiased SGA screens on ORI1 in the S. cerevisiae background in order to determine what genes it genetically interacts in both the presence and absence of oxidative stress (2 mM hydrogen peroxide). Interactions were identified by slow growth (synthetic sick) or death [synthetic lethality (SL)]. These genetic interactions arise from mechanistically distinct genetic origins, as the interactions are occurring between members of the same pathway. Using the principle that an increased amount of an exogenously expressed substrate may cause a fitness defect in a mutant background, this methodology has proven successful in identification of downstream targets as well as targets of specific proteolytic pathways (Costanzo et al., 2010). Therefore using this methodology can identify genetic interactions previously unknown especially when exogenous genes from yeast with no high throughput screening systems can be expressed in a model system such as S. cerevisiae. A SL or synthetic sick genetic interaction is defined as the combination of two genes, each individually viable, that cause a fitness defect not predicted from the multiplicative effect of combining the single mutations (either deletions or over-expression). Classically, this type of genetic interaction is that the cell cannot tolerate the disruption of a shared function of the two genes, implying that the encoded proteins work in parallel to one another. Conversely, SL genetic interaction profiles can predict genes that function in the same pathway or protein complex.
In an attempt to identify the genetic interactors of ORI1 in C. glabrata, we performed genome-wide SGA screens. In brief the S. cerevisiae query strain containing C. glabrata ORI1 was mated to the yeast deletion mutant arrays and the SDL methodology was used to incorporate the plasmid into the deletion mutants. Growth of the deletion mutants containing the plasmids was scored for sickness or lethality. We identified 14 synthetic lethal interactions (Supplementary Table 2) including alo1 (ALO1 is a mitochondrial membrane protein that catalyses the last reaction in biosynthesis of the antioxidant D-ascorbate). This allowed us to hypothesize that ORI1 may play a role in protection from oxidative damage. In addition to ALO1, there were also genetic interactions with genes that play a specific role in the DNA damage and repair pathways namely CRT10, NTG1, RAD52, and ECM11 (Supplementary Table 2), these genes identified in both the screens in the absence and presence of oxidative stress. The SGA screens also highlight the different genetic interactors involved in the presence of oxidative stress (Figure 3 and Supplementary Table 3). The synthetic lethal interactions (Supplementary Table 3) remained the same under both conditions indicating that the cells may be primed to encounter a stress.
Figure 3. Genetic interaction network maps of ORI1 in the presence or absence of oxidative stress. Genome wide synthetic interaction SGA screens were performed using the query strain that was transformed with ORI1 (CAGL0G06710g) from C. glabrata. Genes are represented by nodes that are color-codes according to the S. cerevisiae database (SGD) cellular roles (www.yeastgenome.org). The interactions are represented by edges. All screens were performed in triplicate with double spotting on each plate. Deletion mutants that displayed a synthetic sick or synthetic lethal interaction are detailed in Supplementary Table 1. (A) Genetic interaction map of ORI1 in the absence of stress. (B) Genetic interaction map of ORI1 in the presence of oxidative stress (2 mM H2O2). It can be seen that the presence of oxidative stress affects the genes that ORI1 genetically interacts with.
Following the identification of the genetic interactions with genes that play a key role in the BER and NER pathways (Supplementary Figure 1), we obtained the deletion strains from the lab of Prof Jinks-Robertson (Swanson et al., 1999) in the S. cerevisiae background, where the main elements of each pathway were deleted. Using these backgrounds we were able to transform ORI1 to determine if the overexpression of this gene can compensate for the removal of these pathways (Figure 4A). As can be observed in media with no treatment (i.e., no hydrogen peroxide added), many of the mutants already exhibit a growth defect, due to the loss of different components of the DNA repair pathways which in turn was exacerbated upon exposure to hydrogen peroxide (Figures 4B,C). However, upon the addition of ORI1, into the different deletion strains we can observe some recovery of the sickness phenotype notably in the quadruple deletion strain, Δntg1Δntg2Δapn1Δrad1, on minimal media and media with increasing amounts of hydrogen peroxide as an agent of inducing oxidative stress damage. There is no improvement in the fitness of triple knockout, Δntg1Δntg2Δapn1, upon the introduction of ORI1 indicating that the exogenous gene may be hindering the other DNA damage pathways compensating for the partial lack of ntg1, ntg2 and apn1. The mutant background with the most significant improvement upon exposure to hydrogen peroxide when transformed with ORI1, was the quadruplicate knockout, Δntg1Δntg2Δapn1Δrad1, which is generally highly sensitive to oxidative stress and has had the nucleotide excision repair pathway deleted.
Figure 4. Screening of DNA pathway deletion mutants overexpressing ORI1 on oxidative stress media. A series of S. cerevisiae knock-out strains for different elements of the DNA damage repair pathways were screened on media with (A) no stress, (B) 2 mM H2O2 and (C) 10 mM H2O2. The different deletion mutants were 1: Δntg1 ntg2 apn1 (BER pathway); 2: Δntg1 ntg2 apn1 rad1 (NER pathway), 3: Δntg1 ntg2 apn1 rad52 (Recombination pathway); 4: Δntg1 ntg2 rad1 and 5: Δntg1 ntg2 apn1 rev3 (BER pathway). Plates were spotted with 10 fold serial dilutions of cells and incubated at 30°C for 24 h. Wild-type cells were used as a control.
The natural environments for C. glabrata and S. cerevisiae are significantly different. C. glabrata is a commensal of mucosal surfaces where it encounters phagocytic cells from the immune system and competition from other microbes for nutrients. These environments have selected for evolution of its high resistance to numerous stress conditions such as drug exposure, temperature shifts, osmotic and oxidative stresses. C. glabrata has evolved the capabilities to cope with oxidative stress and can proliferate inside phagocytes when engulfed, currently the mechanism to suppress reactive oxygen species production by phagocytes is unknown. The strong resistance to oxidative stress in C. glabrata is believed to be mediated through the functions of a catalase (CgCta1), two superoxide dismutases (CgSod1 and CgSod2) as well as the glutathione and thioredoxin detoxification systems (Cuéllar-Cruz et al., 2008). Our current knowledge of the oxidative stress response (OSR) in C. glabrata has increased significantly in the last decade with the characterization of the core elements as mentioned above in addition to key transcription factors Yap1, Skn7 and the general stress response regulators Msn2 and Msn4 and the newly identified Ada2 (Yu et al., 2018). However, this current understanding of the OSR in C. glabrata is far from comprehensive with additional genetic components and molecular mechanisms that remain to be explored.
Knowing that S. cerevisiae is intrinsically sensitive to oxidative stress (Farrugia and Balzan, 2012), we utilized this trait as a model to identify oxidative stress resistant genes in C. glabrata. Due to their relative closeness, the need for codon optimization was nullified, allowing for the transformation of the C. glabrata ORFeome en masse into the S. cerevisiae background. The identification of the four novel C. glabrata isolates that have no sequence or synteny homology to any S. cerevisiae genes, highlighted potential ORFs that are conferring these increased levels of oxidative stress resistance to this human fungal pathogen. We confirmed the phenotype of one C. glabrata specific ORF on CAGL0G06710g (ORI1) as it resulted in the strongest phenotype on increasing levels of oxidative stress exposure. Following the comet assays which showed that the expression of ORI1 in S. cerevisiae were offering a level of protection to DNA damage, this lead us to hypothesize that these C. glabrata specific genes may also play a role in DNA damage/repair pathways. DNA damage repair pathways in yeast have been extensively studied (Boiteux and Jinks-Robertson, 2013), however, this does not explain the resistance to DNA damaging agents from oxidative stress in the human fungal pathogen C. glabrata. Our unbiased ORFeome screen, which resulted in the identification of C. glabrata specific genes may add to this fungal pathogens story. These genes are unique to the genome of C. glabrata, potentially having evolved as the fungi carved out its niche as a pathogen.
Utilizing the SGA methodology to systematically screen the yeast deletion mutant array we sought to identify genetic interactions of ORI1. While phenotypic analysis of mutants can provide an important tool to define gene function, the effect of gene under- and over-expression when assessed globally demonstrates high levels of genetic redundancy in the yeast genome. This redundancy has resulted in the powerful approach of SGA screens to study gene function by identifying genetic relationships between genes. This is based on the knowledge that combinations of endogenous and exogenous genes that reduce cell fitness can pinpoint a shared function. With the aid of SGA screening we were able to show that ORI1 genetically interacts with the main genes involved in DNA repair (NTG1, NTG2 and APN1; p-value 7.99E-05, see Supplementary Table 3) in both the presence and absence of oxidative stress. In the presence of stress, (i.e., oxidative stress), we observed an overrepresentation of genes playing a role in base-excision repair (p-value 0.0002993), oxidation-reduction processing (p-value 0.001902).
To gain insight into the role of ORI1 in DNA damage repair in response to oxidative stress exposure, we obtained a series of deletion mutants with specific pathways deleted in the S. cerevisiae background (Supplementary Figure 1 and Figure 4). The removal of oxidative stress damage from yeast DNA is believed to be primarily through the base excision repair pathway. In general, cells lacking Ntg1, Ntg2, and Apn1 are not shown to be sensitive to hydrogen peroxide but upon the additional disruption of RAD52 there is an increased sensitivity to such oxidative stress elements. These mutants were previously determined to have a growth defects, therefore through the addition of ORI1, we were able to circumvent this defect in the presence of oxidative stress. We observed that the quadruplicate deletion strain Δntg1Δntg2Δapn1Δrad1 had increased resistance to increasing hydrogen peroxide upon the addition of ORI1. This quadruplicate deletion strain is defective in the nucleotide excision repair pathway and base excision repair pathway, and is therefore sensitive to hydrogen peroxide. With both of these pathways defective, the DNA damage tolerance pathways (recombination and translesion synthesis) must deal with exposure to oxidative stress and the resulting DNA damage. This also suggests that BER and NER are competing pathways in C. glabrata.
Currently, we are GFP-tagging ORI1 to determine where it localizes to in the cell in the presence and absence of oxidative stress and if this can be correlated to its genetic interactions with NTG1, NTG2, APN1 and RAD52. In addition, with the GFP-tagged strains we are building the protein interaction networks in the presence of oxidative stress in both C. glabrata and S. cerevisiae. Notwithstanding, the specific role Ori1 plays in oxidative stress resistance, it is essential for this resistance and therefore has potential as a therapeutic target being further investigated.
All datasets generated for this study are included in the article/Supplementary Material.
H-lH and YC performed the initial screen to identify C. glabrata isolates. YC and JU performed comet assays. JU, YC, and VA performed SGA screens. JU, H-lH, and KH initial concept. JU, YC, VA, and H-lH data analysis. JU wrote the manuscript. All authors contributed to the article and approved the submitted version.
This work was funded by grants to KH BB/F00513X/1 and BB/F005210/2 from the BBSRC. JU is based at the MRC CMM at the University of Exeter (MR/N006364/2).
The authors declare that the research was conducted in the absence of any commercial or financial relationships that could be construed as a potential conflict of interest.
We thank Prof. Sue Jink-Robertson and her group for sharing strains used in this work. We thank the Candida Research Community for their continued support to continue with this research following the death of KH.
The Supplementary Material for this article can be found online at: https://www.frontiersin.org/articles/10.3389/fgene.2020.530915/full#supplementary-material
Supplementary Figure 1 | Schematic of DNA repair pathways. The “X” represents DNA damage as either a base that is recognize and removed by with the base excision repair pathway (BER) or the nucleotide excision repair pathway (NER). In recombination pathway the polymerase is blocked thus blocking replication and allowing for recombination to occur. (Modified from Swanson et al., 1999).
Supplementary Table 1 | Primers used in this work for the generation of ORI1 deletion strains and ORF amplification for plasmid construction.
Supplementary Table 2 | Analysis of SGA screen with ORI11 (CAGL0G06710g) as the gene of interest.
Supplementary Table 3 | Analysis of the SGA screenings with ORI1 (CAGL0G06710g) in the presence and absence of oxidative stress in the form of hydrogen peroxide.
Albertyn, J., Hohmann, S., Thevelein, J. M., and Prior, B. A. (1994). GPD1, which encodes glycerol-3-phosphate dehydrogenase, is essential for growth under osmotic stress in saccharomyces cerevisiae, and its expression is regulated by the high-osmolarity glycerol response pathway. Mol. Cell. Biol. 14, 4135–4144. doi: 10.1128/mcb.14.6.4135
Alseth, I., Eide, L., Pirovano, M., Rognes, T., Seeberg, E., and Bjørås, M. (1999). The Saccharomyces cerevisiae homologues of endonuclease III from Escherichia Coli, Ntg1 and Ntg2, are both required for efficient repair of spontaneous and induced oxidative DNA damage in yeast. Mol. Cell. Biol. 19, 3779–3787. doi: 10.1128/mcb.19.5.3779
Boiteux, S., and Sue Jinks-Robertson, S. (2013). DNA repair mechanisms and the bypass of DNA damage in Saccharomyces cerevisiae. Genetics 193, 1025–1064. doi: 10.1534/genetics.112.145219
Brieland, J., Essig, D., Jackson, C., Doyle, F., Loebenberg, D., Menzel, F., et al. (2001). Comparison of pathogenesis and host immune responses to Candida glabrata and Candida albicans in systemically infected immunocompetent mice. Infect. Immun. 69, 5046–5055. doi: 10.1128/IAI.69.8.5046
Costanzo, M., Baryshnikova, A., Bellay, J., Kim, Y., Spear, E. D., Sevier, C. S., et al. (2010). The genetic landscape of a cell. Science 327, 425–431. doi: 10.1126/science.1180823
Cuéllar-Cruz, M., Briones-Martin-del-Campo, M., and Cañas-Villamar, I. (2008). High resistance to oxidative stress in the fungal pathogen Candida glabrata is mediated by a single catalase, Cta1p, and is controlled by the transcription factors Yap1p, Skn7p, Msn2p, and Msn4p. Eukaryot. Cell 7, 814–825. doi: 10.1128/EC.00011-08
Eide, L., Bjørås, M., Pirovano, M., Alseth, I., Berdal, K. G., and Seeberg, E. (1996). Base excision of oxidative purine and pyrimidine DNA damage in Saccharomyces cerevisiae by a DNA glycosylase with sequence similarity to endonuclease III from Escherichia Coli. Proc. Natl. Acad. Sci. U.S.A. 93, 10735–10740. doi: 10.1073/pnas.93.20.10735
Fang, F. C. (2004). Antimicrobial reactive oxygen and nitrogen species: concepts and controversies. Nat. Rev. Microbiol. 2, 820–832. doi: 10.1038/nrmicro1004
Farrugia, G., and Balzan, R. (2012). Oxidative stress and programmed cell death in yeast. Front. Oncol. 2:64. doi: 10.3389/fonc.2012.00064
Fukuda, Y., Tsai, H. F., Myers, T. G., and Bennett, J. E. (2013). Transcriptional profiling of Candida glabrata during phagocytosis by neutrophils and in the infected mouse spleen. Infect. Immun. 81, 1325–1333. doi: 10.1128/IAI.00851-12
Gellon, L., Barbey, R., Auffret Van der Kemp, P., Thomas, D., and Boiteux, S. (2001). Synergism between base excision repair, mediated by the DNA glycosylases Ntg1 and Ntg2, and nucleotide excision repair in the removal of oxidatively damaged DNA bases in Saccharomyces cerevisiae. Mol. Genet. Genomics 265, 1087–1096. doi: 10.1007/s004380100507
Gietz, R. D., and Schiestl, R. H. (2007). High-efficiency yeast transformation using the LiAc/SS carrier DNA/PEG method. Nat. Protoc. 2, 31–34. doi: 10.1038/nprot.2007.13
Giglia-Mari, G., Zotter, A., and Vermeulen, W. (2011). DNA damage response. Cold Spring Harb. Perspect. Biol. 3, 1–19. doi: 10.1101/cshperspect.a000745
Griffiths, L. M., Swartzlander, D., Meadows, K. L., Wilkinson, K. D., Corbett, A. H., and Doetsch, P. W. (2009). Dynamic compartmentalization of base excision repair proteins in response to nuclear and mitochondrial oxidative stress. Mol. Cell. Biol. 29, 794–807. doi: 10.1128/mcb.01357-1358
Habraken, Y., Sung, P., Prakash, L., and Prakash, S. (1993). Yeast excision repair gene RAD2 encodes a single-stranded DNA endonuclease. Nat. Lett. 30, 365–368.
Hajjeh, R. A., Sofair, A. N., Harrison, L. H., Lyon, G. M., Arthington-skaggs, B. A., Mirza, S. A., et al. (2004). Incidence of bloodstream infections due to candida species and in vitro susceptibilities of isolates collected from 1998 to 2000 in a population-based active surveillance program. J. Clin. Microbiol. 42, 1519–1527. doi: 10.1128/JCM.42.4.1519
Hin, A., Tong, Y., Evangelista, M., Parsons, A. B., Xu, H., Bader, G. B., et al. (2001). Systematic genetic analysis with ordered arrays of yeast deletion mutants. Science 294, 2364–2369.
Ho, H.-l., and Haynes, K. (2015). Candida glabrata: new tools and technologies-expanding the toolkit. FEMS Yeast Res. 15:fov066. doi: 10.1093/femsyr/fov066
Huang, J. C., Hsu, D. S., Kazantsev, A., and Sancar, A. (1994). Substrate spectrum of human excinuclease: repair of abasic sites, methylated bases, mismatches, and bulky adducts. Proc. Natl. Acad. Sci. U.S.A. 91, 12213–12217. doi: 10.1073/pnas.91.25.12213
Ildiko, U., Haracska, L., Johnson, R. E., Prakash, S., and Prakash, L. (2000). Apurinic endonuclease activity of yeast Apn2 protein. J. Biol. Chem. 275, 22427–22434. doi: 10.1074/jbc.M002845200
Ishchenko, A. A., Yang, X., Ramotar, D., and Saparbaev, M. (2005). The 3′->5′ exonuclease of Apn1 provides an alternative pathway to repair 7,8-Dihydro-8-oxodeoxyguanosine in Saccharomyces cerevisiae. Mol. Cell. Biol. 25, 6380–6390. doi: 10.1128/mcb.25.15.6380-6390.2005
Istel, F., Schwarzmuller, T., Tscherner, M., and Kuchler, K. (2015). Genetic transformation of Candida glabrata by electroporation. Bio Protoc. 5:e1528. doi: 10.21769/bioprotoc.1528
Jacobsen, I. D., Brunke, S., Seider, K., Schwarzmüller, T., Firon, A., D’Enfért, C., et al. (2010). Candida glabrata persistence in mice does not depend on host immunosuppression and is unaffected by fungal amino acid auxotrophy. Infect. Immun. 78, 1066–1077. doi: 10.1128/IAI.01244-1249
Kaloriti, D., Tillmann, A., Cook, E., Jacobsen, M., You, T., Lenardon, M., et al. (2012). Combinatorial stresses kill pathogenic candida species. Med. Mycol. 50, 699–709. doi: 10.3109/13693786.2012.672770
Kaur, R., Ma, B., and Cormack, B. P. (2007). A family of glycosylphosphatidylinositol-linked aspartyl proteases is required for virulence of Candida glabrata. Proc. Natl. Acad. Sci. U.S.A. 104, 7628–7633. doi: 10.1073/pnas.0611195104
Lackner, D. H., Schmidt, M. W., Wu, S., Wolf, D., and Bähler, J. (2012). Regulation of transcriptome, translation, and proteome in response to environmental stress in fission yeast. Genome Biol. 13:R25. doi: 10.1186/gb-2012-13-4-r25
Meadows, K. L., Song, B., and Doetsch, P. W. (2003). Characterization of AP lyase activities of Saccharomyces cerevisiae Ntg1p and Ntg2p: implications for biological function. Nucleic Acids Res. 31, 5560–5567. doi: 10.1093/nar/gkg749
Measday, V., Baetz, K., Guzzo, J., Yuen, K., Kwok, T., Sheikh, B., et al. (2005). Systematic yeast synthetic lethal and synthetic dosage lethal screens identify genes required for chromosome segregation. Proc. Natl. Acad. Sci. U.S.A. 102, 13956–13961. doi: 10.1073/pnas.0503504102
Miramón, P., Kasper, L., and Hube, B. (2013). Thriving within the host: Candida Spp. interactions with phagocytic cells. Med. Microbiol. Immunol. 202, 183–195. doi: 10.1007/s00430-013-0288-z
Mortensen, U. H., Bendixen, C., Sunjevaric, I., and Rothstein, R. (1996). DNA strand annealing is promoted by the yeast RaD52 protein. Proc. Natl. Acad. Sci. U.S.A. 93, 10729–10734. doi: 10.1073/pnas.93.20.10729
Nikolaou, E., Agrafioti, I., Stumpf, M., Quinn, J., Stansfield, I., and Brown, A. J. (2009). Phylogenetic diversity of stress signalling pathways in fungi. BMC Evol. Biol. 9:44. doi: 10.1186/1471-2148-9-44
Pâques, F., and Haber, J. E. (1999). Multiple pathways of recombination induced by double-strand breaks in Saccharomyces cerevisiae. Microbiol. Mol. Biol. Rev. 63, 349–404.
Ramotar, D., Popoff, S. C., Gralla, E. B., and Demple, B. (1991). Cellular role of yeast apn1 apurinic endonuclease/3′-diesterase: repair of oxidative and alkylation DNA damage and control of spontaneous mutation. Mol. Cell. Biol. 11, 4537–4544. doi: 10.1128/mcb.11.9.4537
Roetzer, A., Gregori, C., Jennings, A. M., Quintin, J., Ferrandon, D., Butler, G., et al. (2008). Candida glabrata environmental stress response involves Saccharomyces cerevisiae Msn2/4 orthologous transcription factors. Mol. Microbiol. 69, 603–620. doi: 10.1111/j.1365-2958.2008.06301.x
Roetzer, A., Klopf, E., Gratz, N., Marcet-Houben, M., Hiller, E., Rupp, S., et al. (2011). Regulation of candida glabrata oxidative stress resistance is adapted to host environment. FEBS Lett. 585, 319–327. doi: 10.1016/j.febslet.2010.12.006
Seider, K., Brunke, S., Schild, L., Jablonowski, N., Wilson, D., Majer, O., et al. (2011). The facultative intracellular pathogen Candida glabrata subverts macrophage cytokine production and phagolysosome maturation. J. Immunol. 187, 3072–3086. doi: 10.4049/jimmunol.1003730
Seider, K., Gerwien, F., Kasper, L., Allert, S., Brunke, S., Jablonowski, N., et al. (2014). Immune evasion, stress resistance, and efficient nutrient acquisition are crucial for intracellular survival of Candida glabrata within macrophages. Eukaryot. Cell 13, 170–183. doi: 10.1128/EC.00262-13
Storr, S. J., Woolston, C. M., Zhang, Y., and Martin, S. G. (2013). Redox environment, free radical, and oxidative DNA damage. Antioxid. Redox Signal. 18, 2399–2408. doi: 10.1089/ars.2012.4920
Swanson, R. L., Morey, N. J., Doetsch, P. W., and Jinks-Robertson, S. (1999). Overlapping specificities of base excision repair, nucleotide excision repair, recombination, and translesion synthesis pathways for DNA base damage in saccharomyces cerevisiae. Mol. Cell Biol. 19, 2929–2935. doi: 10.1128/mcb.19.4.2929
Symington, L. S. (2002). Role of RAD52 epistasis Group genes in homoogous recombinaiton and double-strand break repair. Microbiol. Mol. Biol. Rev. 66, 630–670. doi: 10.1128/MMBR.66.4.630
Tkach, J. M., Yimit, A., Lee, A. Y., Riffle, M., Costanzo, M., Jaschob, D., et al. (2012). Dissecting DNA damage response pathways by analysing protein localization and abundance changes during DNA replication stress. Nat. Cell Biol. 14, 966–976. doi: 10.1038/ncb2549
Trotter, E. W., and Grant, G. W. (2005). Overlapping roles of the cytoplasmic and mitochondrial redox regulatory systems in the yeast. Eukaryot. Cell 4, 392–400. doi: 10.1128/EC.4.2.392
Keywords: Candida glabrata, oxidative stress, SGA, human fungal pathogens, comet assay (Single cell gel electrophoresis)
Citation: Usher J, Chaudhari Y, Attah V, Ho H-l and Haynes K (2020) Functional Characterization of a Novel Oxidative Stress Protection Protein in the Pathogenic Yeast Candida glabrata. Front. Genet. 11:530915. doi: 10.3389/fgene.2020.530915
Received: 30 January 2020; Accepted: 27 August 2020;
Published: 25 September 2020.
Edited by:
Christina A. Cuomo, Broad Institute, United StatesReviewed by:
Sascha Brunke, Leibniz Institute for Natural Product Research and Infection Biology, GermanyCopyright © 2020 Usher, Chaudhari, Attah, Ho and Haynes. This is an open-access article distributed under the terms of the Creative Commons Attribution License (CC BY). The use, distribution or reproduction in other forums is permitted, provided the original author(s) and the copyright owner(s) are credited and that the original publication in this journal is cited, in accordance with accepted academic practice. No use, distribution or reproduction is permitted which does not comply with these terms.
*Correspondence: Jane Usher, ai51c2hlckBleGV0ZXIuYWMudWs=
†Deceased
Disclaimer: All claims expressed in this article are solely those of the authors and do not necessarily represent those of their affiliated organizations, or those of the publisher, the editors and the reviewers. Any product that may be evaluated in this article or claim that may be made by its manufacturer is not guaranteed or endorsed by the publisher.
Research integrity at Frontiers
Learn more about the work of our research integrity team to safeguard the quality of each article we publish.