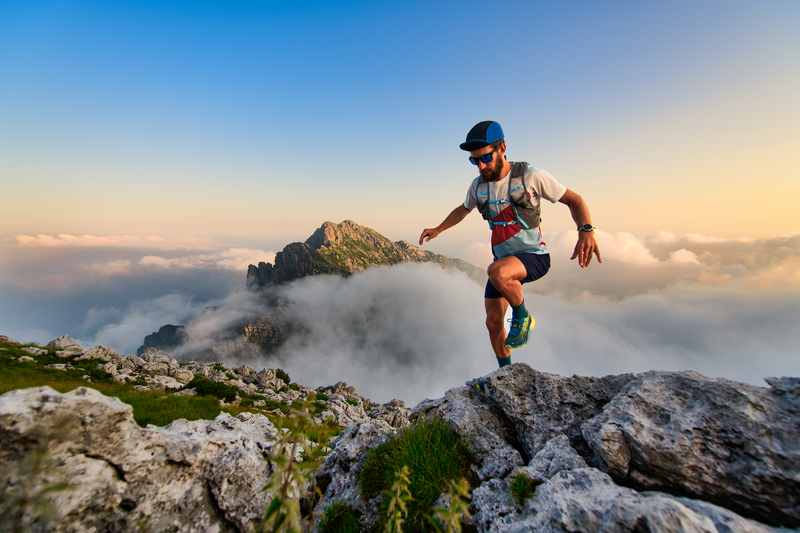
95% of researchers rate our articles as excellent or good
Learn more about the work of our research integrity team to safeguard the quality of each article we publish.
Find out more
ORIGINAL RESEARCH article
Front. Genet. , 06 August 2020
Sec. Epigenomics and Epigenetics
Volume 11 - 2020 | https://doi.org/10.3389/fgene.2020.00887
This article is part of the Research Topic Genetic and Epigenetic Regulation of Insect Development, Reproduction, and Phenotypic Plasticity View all 24 articles
Cuticle barrier efficiency in insects depends largely on cuticular lipids. To learn about the evolution of cuticle barrier function, we compared the basic properties of the cuticle inward and outward barrier function in adults of the fruit flies Drosophila suzukii and Drosophila melanogaster that live on fruits sharing a similar habitat. At low air humidity, D. suzukii flies desiccate faster than D. melanogaster flies. We observed a general trend indicating that in this respect males are less robust than females in both species. Xenobiotics penetration occurs at lower temperatures in D. suzukii than in D. melanogaster. Likewise, D. suzukii flies are more susceptible to contact insecticides than D. melanogaster flies. Thus, both the inward and outward barriers of D. suzukii are less efficient. Consistently, D. suzukii flies have less cuticular hydrocarbons (CHC) that participate as key components of the cuticle barrier. Especially, the relative amounts of branched and desaturated CHCs, known to enhance desiccation resistance, show reduced levels in D. suzukii. Moreover, the expression of snustorr (snu) that encodes an ABC transporter involved in barrier construction and CHC externalization, is strongly suppressed in D. suzukii. Hence, species-specific genetic programs regulate the quality of the lipid-based cuticle barrier in these two Drosophilae. Together, we conclude that the weaker inward and outward barriers of D. suzukii may be partly explained by differences in CHC composition and by a reduced Snu-dependent transport rate of CHCs to the surface. In turn, this suggests that snu is an ecologically adjustable and therefore relevant gene in cuticle barrier efficiency.
Fruit flies of the genus Drosophila including Drosophila, Sophophora, and Hawaiian Drosophila (O’Grady and DeSalle, 2018b) commonly live on fruits that serve as a site for feeding, mating, oviposition, and development. Usually, despite of the common macrohabitat, i.e., orchards, Drosophila flies of different species may occupy their specific microhabitats and largely avoid each other in time and space (Mitsui et al., 2006; Beltrami et al., 2012; O’Grady and DeSalle, 2018a; Plantamp et al., 2019). Some species such as Drosophila suzukii prefer, for instance, immature fruits (Lee et al., 2011; Walsh et al., 2011; Silva-Soares et al., 2017; Olazcuaga et al., 2019), while others like Drosophila melanogaster prefer rotten fruits (Versace et al., 2016).
Microhabitat choice is complex and involves along with behavior and diet preference chemo-physical environmental constraints. A major challenge in microhabitat choice is availability of water including air humidity. One strategy to cope with the water problem is to adapt the efficiency of the cuticular barrier to the specific needs. Molecular mechanisms modulating a cuticle barrier against water loss (desiccation) and accounting for adaptation to different humidity conditions have been analyzed only in a few cases. The differences in desiccation resistance between the closely related East-Australian Drosophila birchii and Drosophila serrata, for instance, rely on the composition of cuticular hydrocarbons (CHCs) that serve as a barrier at the cuticle surface (Chung et al., 2014). In D. birchii, the expression level of mFas that codes for a fatty acid synthase producing methyl-branched CHCs is suppressed compared to its expression level in D. serrata. Thus, reduced methyl-branched CHCs may explain why D. birchii is more sensitive to desiccation than D. serrata.
Several genes involved in cuticular desiccation resistance have been identified and characterized in D. melanogaster. The ABC transporters Oskyddad (Osy) and Snustorr (Snu) and the extracellular protein Snustorr-snarlik (Snsl) are needed for the constitution of the cuticle surface comprising the envelope, the outermost cuticle layer and the CHC overlay (Moussian, 2010; Zuber et al., 2018; Wang et al., 2020). Mutations in osy, snu, and snsl cause rapid water loss and subsequent death. Likewise, RNA interference against cyp4g1 coding for a P450 oxidative decarbonylase required for CHC production enhances desiccation sensitivity (Qiu et al., 2012). It is yet unexplored whether the expression and function of these genes is under environmental control. In a genome-wide study, they were not found to be associated with CHC profile changes in wild-type D. melanogaster flies (Dembeck et al., 2015).
In this work, we sought to compare the cuticle barrier efficiency in D. melanogaster and D. suzukii in order to gain more insight in the ecology and evolution of this trait in fruit flies.
In the summer of 2018, five D. suzukii wild-type flies (2 females and 3 males) were collected from cherries from a cherry tree in a private garden in Tübingen, Germany. With these five flies, we established a D. suzukii stock, that we named “Tübingen 2018.” In 2019, we harvested several dozens of blackberries in a private garden in Tübingen; around 40 flies eclosed from these fruits and were used to set up a new line that was called “Brombeere 2019.” A third D. suzukii wild-type stock was established in 2018 starting from around 20 flies eclosed from several dozens of cherries from the city Bad Wimpfen, Germany, that is about 90 km to the north of Tübingen. The wild-type D. melanogaster stock was established from five female and five male flies collected in a wine orchard in Dijon in 2000 (“Dijon 2000”). All flies were raised in polysterol bottles containing standard corn meal food supplied with fresh baker’s yeast. A filter paper was stuck into D. suzukii culture bottles. The identical laboratory environment for both species ensures comparability of the data.
Ten male or female 5-days old flies were weighed on a micro-balance before drying for 2 h at 90°C. They were weighed for a second time after drying. The difference between the fresh and the dry weighs was used to evaluate the amount of water.
In each assay, groups of ten six to 10 days old flies were collected on ice and incubated in a petri dish containing silica (Sigma Aldrich) at 22°C. The petri dish was sealed with parafilm during the experiment. The experiment was repeated at least three times. Control flies survive for several hours in the petri dish without silica.
According to our recently published protocol (Wang et al., 2016), ten to twenty flies of each sex and species were incubated for 20 min with Eosin Y (0.5%, Sigma-Aldrich) at different temperatures. After staining, flies were washed with tap water at room temperature. Wings were cut off using micro-scissors, observed under a Leica EZ4 stereomicroscope and imaged using the in-built Leica camera and software.
Ten flies of each species were incubated in vials that contained either 0, 0.05 or 0.1 μg chlorpyriphos (stock solution 1 mg/ml acetone). Lethality was recorded during 4 h of incubation at the end of which all flies died when exposed at the highest insecticide amounts. This experiment was repeated at least three times.
To design qPCR primers, D. melanogaster, and D. suzukii transcript sequences were retrieved from flybase (flybase.org). Primers (Table 1) amplifying 100–120 bp were designed using the online primer3 software1. Total RNA was extracted (RNEasy kit, Qiagen) from five freshly eclosed male or female flies (0 to 3 h old) to prepare the cDNA template (Omniscript RT kit, Qiagen) for the qPCR reaction (FastStart Essential DNA Green Master, Roche) on a Roche Nano LightCycler. This experiment was repeated four times. Data were analyzed with the inbuilt software of Roche and Microsoft Excel. The 2–ΔΔCT method was applied to calculate the gene expression levels. The transcript levels of the reference gene RpS20 coding for ribosomal protein S20 were used to normalize gene expression.
To extract CHs, 6-h or 5-day old flies were frozen for 5 min at −20°C, prior to the extraction procedure. For wing analysis, wings were cut off using micro-scissors. Each pair of wings was immersed for 10 min at room temperature in vials containing 20 μl of hexane. For whole fly extraction, each individual was immersed under similar conditions in 30 μl of hexane. In all cases, the solution also contained 3.33 ng/μl of C26 (n-hexacosane) and 3.33 ng/μl of C30 (n-triacontane) as internal standards. After removing the wings or the fly bodies, the extracts were stored at −20°C until analysis. All extracts were analyzed using a Varian CP3380 gas chromatograph fitted with a flame ionization detector, a CP Sil 5CB column (25 m × 0.25 mm internal diameter; 0.1 m film thickness; Agilent), and a split–splitless injector (60 ml/min split-flow; valve opening 30 s after injection) with helium as carrier gas (velocity = 50 cm/s at 120°C). The temperature program began at 120°C, ramping at 10°C/min to 140°C, then ramping at 2 C/min to 290°C, and holding for 10 min. The chemical identity of the CHCs was checked using gas chromatography-mass spectrometry system equipped with a CP Sil 5CB column (Everaerts et al., 2010). The amount (ng/insect) of each component was calculated based on the readings obtained from the internal standards. For the sake of clarity we only show the sum (in ng) of desaturated CHs (ΣDesat), the sum of linear saturated CHs (ΣLin), and the sum of methyl-branched CHs (ΣBr) and their respective percentages calculated based on the overall CHs sum (ΣCHCs).
To learn about ecological similarities and differences between D. suzukii and D. melanogaster, we first compared their desiccation resistance (Figure 1). D. suzukii males were dead within 4 h after exposing them to dry conditions, while most D. melanogaster males survived until 5 h of exposition to dryness. They died between 6 and 8 h under this condition. D. suzukii females started to die after 5 h of exposure to dryness and after 10 h, their lethality reached 90%. The D. melanogaster females reached the 90% lethality rate after 15 h under the same condition. In conclusion, D. suzukii and D. melanogaster females live longer under dry conditions (<10% air humidity) than males. However, both D. suzukii males and females are more sensitive to dryness than D. melanogaster males and females.
Figure 1. D. suzukii and D. melanogaster flies were incubated at low air humidity (<10%). Survival of flies was recorded until all flies had died. Females and males were tested separately as it is known that females are generally more robust than males. The TL50 values are 3–4 h for D. suzukii males, 6–7 h for D. suzukii females, 9–10 h for D. melanogaster males, and 13–14 h for D. melanogaster females. Following a log-rank test, the p-values for the differences between males and between females of both species were calculated; they are <0.0001 for both comparisons.
Body mass and body water content are reported to be positively correlated to desiccation resistance (Ferveur et al., 2018). We determined these two parameters in D. suzukii and D. melanogaster males and females (Table 2). D. suzukii males are 58% heavier than D. melanogaster males, while D. suzukii females are 75% heavier than D. melanogaster females. These differences are statistically significant (see Table 2). Water represents 76% and 82% of the D. suzukii female and male body mass, respectively, while in D. melanogaster females and males it represents 80% and 87%, respectively. These differences have a low statistical significance (see Table 2). Thus, D. suzukii flies weight more than D. melanogaster flies, but, by trend, contain proportionally less water.
Our results suggest that the outward barrier is less efficient in D. suzukii flies than in D. melanogaster flies. Using an Eosin Y incubation assay (Wang et al., 2016, 2017), we tested whether the inward barrier function also differs between D. suzukii and D. melanogaster (Figure 2). In particular, we measured the permeability of the wing cuticle, given that this flat organ allows to produce an unambiguous scoring under light microscopy. The Eosin Y penetration temperature in wings lies between 37 and 40°C in both D. suzukii sexes, whereas its range is 55 to 60°C in both D. melanogaster male and female flies. Thus, the inward barrier of the D. suzukii wing cuticle is more permeable than the D. melanogaster wing cuticle.
Figure 2. The inward barrier efficiency was tested in an Eosin Y penetration assay. As a read out, we choose to score dye penetration into the wing tissue in dependence of the incubation temperature. Here, we show the results for the male wings. Dye penetration into the wing of D. suzukii flies started at 40°C (A), while Eosin Y penetrated the D. melanogaster wing at 55°C (B). Penetration temperature was similar in males and females in both species. Female wings are shown in Supplementary Figure S1. It is worth mentioning that both strains were captured in the wild and are not isogenized; hence, variation of staining intensity and wing size is expected.
To further evaluate the inward barrier efficiency, we also compared the sensitivity of D. suzukii and D. melanogaster to the contact insecticide Chlorpyrifos (Figure 3). In general, D. suzukii flies are more susceptible to Chlorpyrifos than D. melanogaster flies. We also observed that females of both species were more resistant against Chlorpyrifos than males. This finding is consistent with the assumption that the inward barrier is weaker in D. suzukii than in D. melanogaster.
Figure 3. Groups of flies were exposed to three different amounts of chlorpyrifos for 4 h. Survival was recorded every hour. At least 30 flies were tested in each experiment.
The function of both the outward and inward barriers relies on the presence of surface CHCs. To test whether barrier differences between D. suzukii and D. melanogaster are reflected by the CHC composition, we performed GC/MS analysis of surface lipids of the whole body and of dissected wings (Figure 4). Our results are consistent with studies reporting CHC profiles in D. suzukii (Snellings et al., 2018) and D. melanogaster (Everaerts et al., 2010). On average, 1115 ng CHCs per male and 1404 ng CHCs per female were extracted from the body surface of D. suzukii. The body surface extracts of each D. melanogaster male contains 1506 ng CHCs on average, while each female D. melanogaster has 2095 ng CHCs on its body surface. The desaturation rates of D. suzukii male and female body CHCs are 63.1% and 59.1%, respectively. The desaturation rates of D. melanogaster male and female body CHCs are 59.3% and 71.1%, respectively. Around 6.5% of body CHCs on D. suzukii males and 10.4% on D. suzukii females are methylated. The methylation rates on D. melanogaster male and female body CHCs are 21.3% and 19.4%, respectively. We conclude that both the total amounts and the composition of body CHCs considerably differ between D. suzukii and D. melanogaster. In a nutshell, D. melanogater males and females have more branched, desaturated and total, but less linear CHCs than their D. suzukii counterparts. The CHC relative compositions of dissected D. suzukii and D. melanogaster wings are similar to the respective whole body CHC relative compositions.
Figure 4. Amounts (in ng) and composition of wings (left panels) and whole body (right) CHCs in individual D. melanogaster (top) and D. suzukii (bottom) were determined by gas chromatography and mass spectrometry. The amounts of sum linear (ΣLin), sum branched (ΣBr) and sum desaturated (ΣDesat) CHCs were calculated based on the overall sum of CHCs (ΣCHCs). See Supplementary Figure S2 for all detected CHCs and Table 3 for the statistical analysis of these data.
Table 3. p-values of the Wilcoxon test on differences of the wing and whole body total (CHCs), desaturated (Desat), branched (Br), and linear (Lin) CHC amounts between D. suzukii and D. melanogaster males and females presented in Figure 4.
To learn about the molecular mechanisms underlying CHC composition in D. suzukii and D. melanogaster, we monitored the expression levels of genes that code for enzymes involved in CHC formation (Figure 5). We used quantitative real-time PCR (qPCR), to record the expression levels of fas1, fas2, fas3, cyp4g1, farO, acc, desat1, and app transcripts in newly hatched flies. Compared to D. melanogaster females, the expression of genes encoding the first key enzymes of fatty acid synthesis FAS1 and ACC were low in D. suzukii females. However, these genes showed similar expression levels in D. melanogaster and D. suzukii males. Likewise, the expression of farO was similar in D. melanogaster and D. suzukii males and females. While fas3 expression was higher in D. suzukii males than in D. melanogaster males, it did not differ between D. melanogaster and D. suzukii females. The expression of the gene coding for the terminal CHC producing enzyme Cyp4g1 was lower in D. suzukii males as compared to D. melanogaster males whereas it did not differ between females of the two species. In both sexes, app transcript levels were higher in D. suzukii, while the expression levels of both fas2 and desat1 were around 100 times higher in D. suzukii than in D. melanogaster.
Figure 5. The expression of genes coding for enzymes and proteins involved in lipid (CHC) biosynthesis and transport was monitored by qPCR in females (A) and males (B) of D. suzukii and D. melanogaster. For all transcripts except for FAS2 and desat1, the D. melanogaster expression levels were set to 1. For FAS2, the expression level of the D. melanogaster transcript was set to 0.01, and the expression level of the D. melanogaster desat1 transcript was set to 0.1. The p-values following a Student’s t-test are 0.04 (female; f) and 0.33 (male; m) for FAS1, 0.29 (f) and 0.004 (m) for FAS3, 0.88 (f) and 0.004 (m) for Cyp4G1, 0.05 (f) and 0.048 (m) for FarO, 0.007 (f) and 0.04 (m) for ACC, 0.002 (f) and 0.046 (m) for app, 0.098 (f) and 0.49 (m) for snsl, 0.03 (f) and 0.003 (m) for snu, 0.047 (f) and 0.006 (m) for osy, 0.02 (f) and 0.0007 (m) for FAS2 and 0.009 (f) and 0.009 (m) for desat1 expression differences.
We also examined the expression levels of oskyddad (osy), snustorr (snu), and snustorr snarlik (snsl), all three coding for proteins required for the construction of the cuticle barrier (Figure 5). The expression of snsl did not differ significantly in both sexes of the two species. Levels of osy transcripts were higher in D. suzukii females and males than in D. melanogaster. However, snu expression was dramatically reduced in both D. suzukii females and males compared to same-sex D. melanogaster flies. We obtained identical results when we compared the expression levels of snu in both D. suzukii and D. melanogaster female and male dissected wing buds (Supplementary Figure S3).
Initially, snu was characterized in D. melanogaster as a key gene involved in barrier efficiency in a genetic approach in the laboratory (Zuber et al., 2018; Wang et al., 2020). Indeed, mutations in snu are lethal underlining its role in barrier function. Here, the quantitative variation of its expression correlating with cuticle barrier efficiency in D. suzukii and D. melanogaster suggests that it is also important in nature. To underpin this notion, we repeated the analysis of its expression changing two parameters. First, in order to rule out line-specific gene expression, we quantified snu transcript levels in two additional D. suzukii wild-type lines (see section “Materials and Methods”). Second, to exclude primer-specific effects, we designed a new set of D. suzukii snu-specific primers for these experiments (Table 1). The expression of snu was significantly reduced by more than 80% in D. suzukii compared to D. melanogaster in these experiments (Supplementary Figure S3).
Overall, the expression of some key genes coding for proteins and enzymes involved in lipid-based barrier construction and function show a substantial variation between D. suzukii and D. melanogaster. Especially, the expression divergence of snu is intriguing as its transcript levels have been shown to correlate with barrier efficiency in D. melanogaster (Wang et al., 2020).
Desiccation resistance is conveyed by a combination of the behavioral repertoire and physiological and physical properties of the organism. In case of incipient drought, an animal, for instance, may respond by taking up and storing more water and initiate fortification of its barrier against evaporation. These responses and their amplitude depend, of course, on the genetic constitution of the organism. Hypothetically, the micro-environment of an insect has a decisive impact on the quality of a responsive trait and the expression dynamics of the underlying genes. Under identical conditions, the response may vary at several levels. In dimorphic species, a difference in this regard may prevail between sexes. Response variation is probably more accentuated between species than within species.
In our inter-species comparative approach, we found that D. suzukii is more sensitive to low air humidity than D. melanogaster. This is in agreement with a work published by Terhzaz et al. (2018). Since all flies were reared under similar laboratory conditions, we can rule out possible experimental bias for this observation. Indeed, both species were shown to be responsive to environmental cues. D. suzukii appears in two morphs, i.e., the summer and the winter morph with distinct ecological traits. The winter morph, for instance, is more robust at low humidity conditions than the summer morph (Toxopeus et al., 2016; Fanning et al., 2019). D. melanogaster as a cosmopolitan species also shows geographical variation (Rajpurohit et al., 2017; Dong et al., 2019). We believe that there are two physiological explanations for the inter-species differences. The first one implies that the difference in water shortage tolerance between these two species relies on total body water content. Indeed, we found that the overall body water content in D. suzukii is lower than in D. melanogaster. However, the water-content hypothesis is not very plausible given that D. suzukii males which contain relatively more water than females are less robust than females at lower air humidity. The second physiological explanation underscores that the water loss rate through the three major routes respiration, excretion or cuticular transpiration may be higher or faster in D. suzukii than in D. melanogaster. For a number of different Drosophila species including D. melanogaster (D. suzukii was not included in that study), it was proposed that especially cuticular transpiration accounts for the highest water loss rate, whereas excretion (<6%), and respiration (<10%) are rather negligible in this regard (Gibbs et al., 2003).
According to the assumption of Gibbs et al. (2003), we think that a weaker outward barrier in D. suzukii may explain the observed difference in desiccation resistance between these species. Indeed, we found that D. suzukii flies have less CHCs on their surface than D. melanogaster flies suggesting that the CHC-based barrier is weaker in D. suzukii. However, Gibbs et al. (2003) reported that the quality of the cuticle barrier does not seem to depend on the CHC amounts in the Drosophila species studied. On the other hand, several recent works suggested that reduced CHC levels do cause enhanced desiccation (Qiu et al., 2012). Gibbs et al. (2003) also argued that the CHC melting temperature Tm that depends on CHC composition did not correlate with the water loss rate in their work. This is, however, in conflict with more recent findings. Higher proportions of desaturated CHCs confer increased desiccation resistance to D. melanogaster flies in experimentally selected lines (Ferveur et al., 2018). In another study, Chung et al. (2014) demonstrated that higher amounts of branched CHCs in D. serrata (29%) entail a higher desiccation resistance compared to D. birchii (3%). In analogy, our study reveals that D. suzukii has proportionally less branched CHCs (6% in males and 13% in females of the total amounts of CHCs) than D. melanogaster (23% in males and 19% in females of the total amounts of CHCs). We conclude that these traits may contribute to the difference in desiccation resistance observed between D. suzukii and D. melanogaster.
Remarkably, the CHC profiles in D. suzukii and D. melanogaster did not correlate with expression profiles of genes coding for lipid synthesis or modification enzymes. For instance, FASN2 that codes for a microsomal fatty acid synthase catalyzing the formation of methyl-branched fatty acids, is expressed at higher levels in D. suzukii than in D. melanogaster, while the relative amounts of branched CHCs is reduced in D. suzukii. Moreover, the expression of Desat1 that codes for a fatty acid desaturase responsible for fatty acid desaturation is enhanced in D. suzukii although the relative amounts of desaturated CHCs are lower in this species. Either the gene expression levels do not reflect the enzyme levels, or the catalytic activity of enzymes in D. suzukii is lower than in D. melanogaster. Alternatively, the limiting process of CHC amounts may not be their production but their externalization and deposition on the cuticle. Consistent with this interpretation, we found that the expression of snu coding for an ABC transporter involved in barrier construction and function (Zuber et al., 2018; Wang et al., 2020) is reduced in D. suzukii compared to D. melanogaster. Thus, it is possible that less CHCs accumulate on the cuticle of D. suzukii because of reduced Snu activity that should be tested in biochemical experiments. This interpretation is in line with our recent findings that in D. melanogaster flies with low snu expression in the wing have decreased CHC levels and compromised wing cuticle barrier function (Wang et al., 2020). Possibly, the expression divergence between D. melanogaster and D. suzukii relies on a species-specific regulatory network and involves more target genes than snu alone (Wittkopp, 2007). One such target gene may be osy, also coding for an ABC transporter needed for cuticle barrier efficiency and acting independently from Snu (Wang et al., 2020). The expression of osy is, in contrast to snu, slightly but significantly upregulated in D. suzukii compared to D. melanogaster. Obviously, this upregulation is not sufficient to equate the cuticle barrier efficiency in D. suzukii with the one in D. melanogaster. Admittedly, this is a compelling but simplified scenario as we do neglect an important point. The experiments presented in this work were done in a constant laboratory environment. Thus, we did not analyze the gene x environment (GxE) interaction in our desiccation assay. In other words, in nature, a complex, changing environment, gene expression variation may be different (Gibson, 2008; Hodgins-Davis and Townsend, 2009). For instance, the barrier of D. suzukii flies might be more robust under natural conditions than in the laboratory. Whether these differences may account for lifestyle differences between D. suzukii and D. melanogaster remains to be investigated.
To what extent can we apply our view on the situation of the outward barrier in D. suzukii and D. melanogaster to the situation of the inward barrier? Our experiments showed that the weaker outward barrier function of D. suzukii flies is paralleled by their weaker inward barrier function. Xenobiotics including both the inert dye Eosin Y and the insecticide Chlorpyrifos did penetrate the cuticle of D. suzukii more efficiently than in D. melanogaster. Thus, in a simple scenario, the CHC composition that defines the outward barrier function, would also be responsible for the inward barrier function in both species.
We would like to share two concluding remarks with the reader. First, we are aware that some differences in barrier efficiency between D. suzukii and D. melanogaster may be due to some geographical differences between the cities of Tübingen (Germany) and Dijon (France), where the respective strains studied here were captured. Indeed, barrier efficiency varies among geographically separated D. melanogaster populations (Dong et al., 2019). D. suzukii populations also display genomic variations (Tait et al., 2017) that may have an impact on their desiccation tolerance. However, the difference of desiccation sensitivity between these two species is similar to that reported in a previous study indicating that D. melanogaster is more resistant under low air humidity than D. suzukii (Terhzaz et al., 2018). This confirms that inter-specific differences are higher than possible geographical intraspecific differences.
Second, here we focussed on the cuticle as a potential site of water loss during desiccation. Recently, Terhzaz et al. (2018) showed that differences in a neuroendocrine control of water loss through the Malpighian tubules, i.e., excretory water loss in D. suzukii and D. melanogaster could also explain differences in desiccation resistance. Hence, as mentioned above, besides cuticular transpiration control, other mechanisms of water homeostasis may account for performance differences between Drosophila species adapted to environments with variable hygrometry. Continuing work in this direction to gather the complexity of desiccation resistance or sensitivity may ultimately serve to design strategies for successful Drosophila pest management.
The raw data supporting the conclusions of this article will be made available by the authors, without undue reservation, to any qualified researcher.
YW, J-PF, YY, JY, WT, NG, J-FF, and BM performed experiments and analyzed data. J-FF and BM designed and supervised experiments. J-FF revised the manuscript. BM wrote and revised the manuscript.
This work was supported by a grant to BM by the German Research Foundation (DFG, MO1714/9-1) and a grant to YY by the National Science Foundation of China (NSFC, 31761133021).
The authors declare that the research was conducted in the absence of any commercial or financial relationships that could be construed as a potential conflict of interest.
The Supplementary Material for this article can be found online at: https://www.frontiersin.org/articles/10.3389/fgene.2020.00887/full#supplementary-material
FIGURE S1 | As in males shown in Figure 2, Eosin Y penetrates the wings of D. suzukii females at lower temperatures as the wings of D. melanogaster females.
FIGURE S2 | Here we show the array of CHCs in weight (ng) and percentage (%) as determined in wings and whole bodies of D. suzukii and D. melanogaster flies.
FIGURE S3 | (A) As in the whole body shown in Figure 5, snu expression is reduced also in D. suzukii wings of males and females compared to D. melanogaster. (B) The expression of snu is reduced in three different stocks of D. suzukii flies (males and females mixed) compared to D. melanogaster flies. The p-values after a Student’s t-test are 0.0004 for the expression differences of snu between female wings and 0.02 for the expression differences between male wings shown in (A). The p-values for the expression differences of snu between D. melanogaster and the different D. suzukii lines are 0.013 (Tübingen 2018), 0.009 (Brombeere), and 0.008 (Bad Wimpfen) shown in (B).
Beltrami, M., Medina-Munoz, M. C., Del Pino, F., Ferveur, J. F., and Godoy-Herrera, R. (2012). Chemical cues influence pupation behavior of Drosophila simulans and Drosophila buzzatii in nature and in the laboratory. PLoS One 7:e39393. doi: 10.1371/journal.pone.0039393
Chung, H., Loehlin, D. W., Dufour, H. D., Vaccarro, K., Millar, J. G., and Carroll, S. B. (2014). A single gene affects both ecological divergence and mate choice in Drosophila. Science 343, 1148–1151. doi: 10.1126/science.1249998
Dembeck, L. M., Boroczky, K., Huang, W., Schal, C., Anholt, R. R., and Mackay, T. F. (2015). Genetic architecture of natural variation in cuticular hydrocarbon composition in Drosophila melanogaster. eLife 4:e09861.
Dong, W., Dobler, R., Dowling, D. K., and Moussian, B. (2019). The cuticle inward barrier in Drosophila melanogaster is shaped by mitochondrial and nuclear genotypes and a sex-specific effect of diet. PeerJ 7:e7802. doi: 10.7717/peerj.7802
Everaerts, C., Farine, J. P., Cobb, M., and Ferveur, J. F. (2010). Drosophila cuticular hydrocarbons revisited: mating status alters cuticular profiles. PLoS One 5:e9607. doi: 10.1371/journal.pone.0009607
Fanning, P. D., Johnson, A. E., Luttinen, B. E., Espeland, E. M., Jahn, N. T., and Isaacs, R. (2019). Behavioral and physiological resistance to desiccation in spotted wing Drosophila (Diptera: Drosophilidae). Environ. Entomol. 48, 792–798. doi: 10.1093/ee/nvz070
Ferveur, J. F., Cortot, J., Rihani, K., Cobb, M., and Everaerts, C. (2018). Desiccation resistance: effect of cuticular hydrocarbons and water content in Drosophila melanogaster adults. PeerJ 6:e4318. doi: 10.7717/peerj.4318
Gibbs, A. G., Fukuzato, F., and Matzkin, L. M. (2003). Evolution of water conservation mechanisms in Drosophila. J. Exp. Biol. 206, 1183–1192. doi: 10.1242/jeb.00233
Gibson, G. (2008). The environmental contribution to gene expression profiles. Nat. Rev. Genet. 9, 575–581. doi: 10.1038/nrg2383
Hodgins-Davis, A., and Townsend, J. P. (2009). Evolving gene expression: from G to E to GxE. Trends Ecol. Evol. 24, 649–658. doi: 10.1016/j.tree.2009.06.011
Lee, J. C., Bruck, D. J., Curry, H., Edwards, D., Haviland, D. R., Van Steenwyk, R. A., et al. (2011). The susceptibility of small fruits and cherries to the spotted-wing Drosophila, Drosophila suzukii. Pest Manag. Sci. 67, 1358–1367. doi: 10.1002/ps.2225
Mitsui, H., Takahashi, K. H., and Kimura, M. T. (2006). Spatial distributions and clutch sizes of Drosophila species ovipositing on cherry fruits of different stages. Population Ecol. 48, 233–237. doi: 10.1007/s10144-006-0260-5
Moussian, B. (2010). Recent advances in understanding mechanisms of insect cuticle differentiation. Insect. Biochem. Mol. Biol. 40, 363–375. doi: 10.1016/j.ibmb.2010.03.003
O’Grady, P., and DeSalle, R. (2018a). Hawaiian Drosophila as an evolutionary model clade: days of future past. Bioessays 40:e1700246.
Olazcuaga, L., Rode, N. O., Foucaud, J., Facon, B., Ravigne, V., Ausset, A., et al. (2019). Oviposition preference and larval performance of Drosophila suzukii (Diptera: Drosophilidae), spotted-wing Drosophila: effects of fruit identity and composition. Environ. Entomol. 48, 867–881. doi: 10.1093/ee/nvz062
Plantamp, C., Henri, H., Andrieux, T., Regis, C., Mialdea, G., Dray, S., et al. (2019). Phenotypic plasticity in the invasive pest Drosophila suzukii: activity rhythms and gene expression in response to temperature. J. Exp. Biol. 222:jeb199398. doi: 10.1242/jeb.199398
Qiu, Y., Tittiger, C., Wicker-Thomas, C., Le Goff, G., Young, S., Wajnberg, E., et al. (2012). An insect-specific P450 oxidative decarbonylase for cuticular hydrocarbon biosynthesis. Proc. Natl. Acad. Sci. U.S.A. 109, 14858–14863. doi: 10.1073/pnas.1208650109
Rajpurohit, S., Hanus, R., Vrkoslav, V., Behrman, E. L., Bergland, A. O., Petrov, D., et al. (2017). Adaptive dynamics of cuticular hydrocarbons in Drosophila. J. Evol. Biol. 30, 66–80.
Silva-Soares, N. F., Nogueira-Alves, A., Beldade, P., and Mirth, C. K. (2017). Adaptation to new nutritional environments: larval performance, foraging decisions, and adult oviposition choices in Drosophila suzukii. BMC Ecol. 17:21. doi: 10.1186/s12898-017-0131-2
Snellings, Y., Herrera, B., Wildemann, B., Beelen, M., Zwarts, L., Wenseleers, T., et al. (2018). The role of cuticular hydrocarbons in mate recognition in Drosophila suzukii. Sci. Rep. 8:4996.
Tait, G., Vezzulli, S., Sassu, F., Antonini, G., Biondi, A., Baser, N., et al. (2017). Genetic variability in Italian populations of Drosophila suzukii. BMC Genet. 18:87. doi: 10.1186/s12863-017-0558-7
Terhzaz, S., Alford, L., Yeoh, J. G., Marley, R., Dornan, A. J., Dow, J. A., et al. (2018). Renal neuroendocrine control of desiccation and cold tolerance by Drosophila suzukii. Pest Manag. Sci. 74, 800–810.
Toxopeus, J., Jakobs, R., Ferguson, L. V., Gariepy, T. D., and Sinclair, B. J. (2016). Reproductive arrest and stress resistance in winter-acclimated Drosophila suzukii. J. Insect. Physiol. 89, 37–51.
Versace, E., Eriksson, A., Rocchi, F., Castellan, I., Sgado, P., and Haase, A. (2016). Physiological and behavioral responses in Drosophila melanogaster to odorants present at different plant maturation stages. Physiol. Behav. 163, 322–331.
Walsh, D. B., Bolda, M. P., Goodhue, R. E., Dreves, A. J., Lee, J. C., Bruck, D. J., et al. (2011). Drosophila suzukii (Diptera: Drosophilidae): invasive pest of ripening soft fruit expanding its geographic range and damage potential. J. Intergr. Pest Manag. 2, G1–G7.
Wang, Y., Carballo, R. G., and Moussian, B. (2017). Double cuticle barrier in two global pests, the whitefly Trialeurodes vaporariorum and the bedbug Cimex lectularius. J. Exp. Biol. 220, 1396–1399.
Wang, Y., Norum, M., Oehl, K., Yang, Y., Zuber, R., Yang, J., et al. (2020). Dysfunction of Oskyddad causes Harlequin-type ichthyosis-like defects in Drosophila melanogaster. PLoS Genet. 16:e1008363. doi: 10.1371/journal.pgen.1008363
Wang, Y., Yu, Z., Zhang, J., and Moussian, B. (2016). Regionalization of surface lipids in insects. Proc. Biol. Sci. 283:20152994.
Wittkopp, P. J. (2007). Variable gene expression in eukaryotes: a network perspective. J. Exp. Biol. 210, 1567–1575.
Keywords: insect, cuticle, Drosophila, barrier, lipid
Citation: Wang Y, Farine J-P, Yang Y, Yang J, Tang W, Gehring N, Ferveur J-F and Moussian B (2020) Transcriptional Control of Quality Differences in the Lipid-Based Cuticle Barrier in Drosophila suzukii and Drosophila melanogaster. Front. Genet. 11:887. doi: 10.3389/fgene.2020.00887
Received: 27 March 2020; Accepted: 20 July 2020;
Published: 06 August 2020.
Edited by:
Zhongxia Wu, Henan University, ChinaReviewed by:
Amy Tsurumi, Massachusetts General Hospital and Harvard Medical School, United StatesCopyright © 2020 Wang, Farine, Yang, Yang, Tang, Gehring, Ferveur and Moussian. This is an open-access article distributed under the terms of the Creative Commons Attribution License (CC BY). The use, distribution or reproduction in other forums is permitted, provided the original author(s) and the copyright owner(s) are credited and that the original publication in this journal is cited, in accordance with accepted academic practice. No use, distribution or reproduction is permitted which does not comply with these terms.
*Correspondence: Bernard Moussian, YmVybmFyZC5tb3Vzc2lhbkB1bmktdHVlYmluZ2VuLmRl
Disclaimer: All claims expressed in this article are solely those of the authors and do not necessarily represent those of their affiliated organizations, or those of the publisher, the editors and the reviewers. Any product that may be evaluated in this article or claim that may be made by its manufacturer is not guaranteed or endorsed by the publisher.
Research integrity at Frontiers
Learn more about the work of our research integrity team to safeguard the quality of each article we publish.