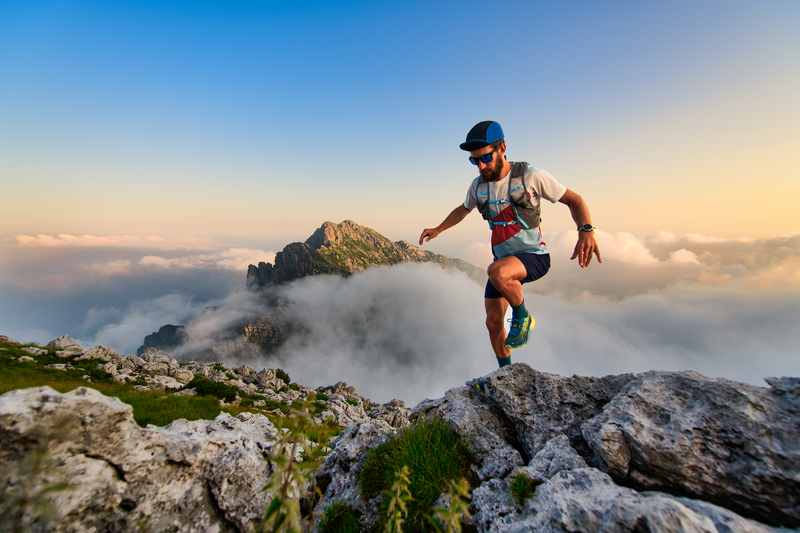
94% of researchers rate our articles as excellent or good
Learn more about the work of our research integrity team to safeguard the quality of each article we publish.
Find out more
BRIEF RESEARCH REPORT article
Front. Genet. , 06 March 2020
Sec. Genetics of Common and Rare Diseases
Volume 11 - 2020 | https://doi.org/10.3389/fgene.2020.00169
A large fraction of DNA variants impairs pre-mRNA splicing in human hereditary disorders. Crigler-Najjar syndrome (CNS) is characterized by a severe unconjugated hyperbilirubinemia caused by variants in the UGT1A1 gene. We previously reported one CNS-type II patient with two splice-site variants in trans (c.864+5G>T and c.996+2_996+5del). According to MaxEntScan, both disrupt their corresponding donor sites (c.864+5G>T: 6.99 → 2.28; c.996+2_996+5del: 5.96 → −11.02), so they were selected for subsequent functional tests. Given the unavailability of patient RNA, we constructed an UGT1A1 splicing-reporter minigene with exons 1–4 to characterize the underlying splicing anomaly. The variant c.996+2_996+5del generated two aberrant transcripts, Δ(E2) (exon 2 skipping/64%) and ▼(E2q135) (intron retention of 135-nt/36%), which lead to the loss of 18 conserved amino-acids and the gain of 45 new ones of a critical functional domain, respectively. The c.864+5G>T variant mainly produced the aberrant transcript Δ(E1q141) (141-nt deletion/70.4%) and the full-length isoform (29.6%). Δ(E1q141) would provoke the loss of 47 amino-acids of the N-terminal domain that encodes for substrate specificity. Thus, the three anomalous transcripts are likely to inactivate UGT1A1. Moreover, this patient is also homozygous for the promoter variant A(TA)7TAA that decreases UGT1A1 expression by 70%, so the full-length transcript produced by c.864+5G>T would be even more reduced (<9%), thus supporting the diagnosis of CNS-type II. Therefore, minigenes represent valuable tools for the functional and clinical classifications of genetic variants.
Unconjugated hyperbilirubinemia ranges in severity from no detectable symptoms (most Gilbert’s syndrome individuals) to severe bilirubin toxicity in Crigler-Najjar syndrome type II patients and fatal accumulation of bilirubin in Crigler-Najjar syndrome type I patients. The clinical outcome of a UGT1A1 deficiency relies on the residual activity of the UGT1A1 gene (OMIM#606433, Gene ID: 54658). In Gilbert’s syndrome patients, UGT1A1 residual activity is ∼30% of the wild type activity, whereas in Crigler-Najjar type II and type I patients it is below 10 and 0%, respectively (Arias et al., 1969; Seppen et al., 1994; Kadakol et al., 2001; Maruo et al., 2016; Wagner et al., 2018).
We have previously detailed a patient with clinically diagnosed Crigler-Najjar syndrome type II caused by multiple allelic variants in the UGT1A1 gene (Gailite et al., 2018). One of these variants was a novel deletion of four intronic nucleotides after exon 2 (NG_033238.1:g.11895_11898del, c.996+2_996+5del), potentially affecting the donor splice site. Another one was a nucleotide substitution in the +5 position of the first exon-intron splice junction (NG_033238.1:g.5884G>T, c.864+5G>T, rs777807265). Both variants were in trans and affected the 5′ splice sites; however, the absence of functional studies limited their clinical interpretations (Gailite et al., 2018). Interestingly, more than two decades ago, splice site variants were first proposed as a possible cause of unconjugated hyperbilirubinemia (Gantla et al., 1998).
Splicing is a critical gene expression step whereby introns are accurately removed from the precursor mRNA and exons are consecutively joined. Alternative splicing is a highly-regulated mechanism that is responsible for transcriptome and protein diversity. Indeed, it has been estimated that about 95% of human genes undergo alternative splicing (Pan et al., 2008; Wang et al., 2008). This process is strictly controlled by ribonucleoproteins, splicing factors, and a variety of cis-regulatory elements including the 5′ and 3′ splice sites, the polypyrimidine tract, the branch point, and splicing enhancers and silencers (Cartegni et al., 2002). All of these components are potential targets of pathogenic variants that can disrupt splicing and consequently lead to disease. In fact, defective splicing is one of the most common mechanisms of genetic diseases, being responsible for ∼10% of all genetic diseases (Wang and Cooper, 2007; Fraile-Bethencourt et al., 2019b; Jaganathan et al., 2019). Despite the implementation of novel algorithms and deep learning methods, prediction of the effect of variants located outside of the canonical splice sites remains limited. Further, even for canonical splice site variants, it is impossible to precisely predict transcript outcomes. Therefore, splicing functional assays are necessary to demonstrate the splicing impact of a particular DNA variant and to evaluate its pathogenicity (Richards et al., 2015; Jaganathan et al., 2019). RT-PCR of patient RNA samples is the most direct method to test splicing. However, the main limitation of this method is the requirement of tissue expressing the gene of interest, and unfortunately this type of tissue sample is not always available. Resultantly, splicing reporter minigenes have emerged as an alternative approach to test a variant from the splicing perspective (Tammaro et al., 2014). Remarkably, minigenes of the splicing vector pSAD have been successfully used to assay more than 300 variants of the breast cancer gene BRCA2 (Acedo et al., 2015; Fraile-Bethencourt et al., 2017, 2019b).
In this study, we constructed an ad hoc UGT1A1 minigene with exons 1 to 4 (mgUGT1A1_ex1-4) with the purpose of testing the intronic variants c.864+5G>T and c.996+2_996+5del from the donor sites of exons 1 and 2, respectively.
The DNA of a patient with unconjugated hyperbilirubinemia was isolated from peripheral blood and the UGT1A1 gene was sequenced as described previously (Gailite et al., 2018). Four different genetic variants were identified. Two of them were known and had been previously characterized – NG_033238.1:g.3664A>C (c.1352A>C, rs3755319) and NG_033238.1:g.4963_4964TA[7] (c.-53_-52insTA, A(TA)7TAA, UGT1A1∗28, rs8175347). The pathogenicity of the two other variants, which were found in trans (NG_033238.1:g.11895_11898del -c.996+2_996+5del- and NG_033238.1:g.5884G>T -c.864+5G>T, rs777807265) was evaluated according to the American College of Medical Genetics guidelines (Richards et al., 2015); both variants were classified as likely pathogenic. The patient’s phenotype and molecular findings confirmed Crigler-Najjar syndrome type II. Because UGT1A1 gene expression occurs almost exclusively in the liver and gastrointestinal system and not in blood [GTEx: (GTEx Consortium et al., 2017)], we were unable to test the effect of the c.996+2_996+5del and c.864+5G>T variants on splicing in the patient due to the lack of non-blood tissue.
The study was carried out in accordance with the approved protocol of the local Ethics Review Committee. All participants (patient and parents) gave written informed consent in accordance with the Declaration of Helsinki.
In silico analysis of the wild type and mutant sequences was performed by using the MaxEntScan algorithm (MES) (Yeo and Burge, 2004) that is integrated within the bioinformatics tool Human Splicing Finder version 3.1 (HSF)1 (Desmet et al., 2009). The default threshold value of MES within HSF is 3.0 except for variant analysis where all the resultant splice site scores are reported.
Variants and transcripts were described according to the Human Genome Variation Society (HGVS) guidelines on the basis of the UGT1A1 GenBank sequence NM_00463.2. For simplification, transcripts were annotated with a shortened code, as described previously (Fraile-Bethencourt et al., 2019a; Lopez-Perolio et al., 2019): Δ (skipping or loss of exonic sequences), ▼ (inclusion of intronic sequences), E (exon), p (acceptor shift), and q (donor shift). When necessary, the exact number of skipped or retained nucleotides is indicated.
The construct mgUGT1A1_ex1-4 was assembled in three steps by overlapping extension PCR or classical restriction digestion/ligation cloning with three intermediate constructs: mgUGT1A1_EX2, mgUGT1A1_EX2-4, and mgUGT1A1_EX1-4. All the inserts were amplified from patient DNA with Phusion High Fidelity polymerase (Thermo Fisher Scientific, Waltham, MA, United States) and primers indicated in Table 1.
Mutant (c.996+2_996+5del) and wild type exon 2 from the patient were subcloned into the pSAD vector (Acedo et al., 2015) by overlapping extension PCR. Exons 3 and 4 were then inserted between the EcoRI and HindIII restriction sites of mgUGT1A1_ex2. Finally, mutant (c.864+5G>T) and wild type exon 1 from the patient were introduced by overlapping extension PCR, creating a chimeric exon V1-UGT1A1 exon 1. This chimeric exon joins and keeps the open reading frames of V1 exon and UGT1A1 exon 1 (codons 200 to 288): [V1]…LKGTF-[UGT1A1]_HSDHMTFLQ……NCLHQNPLSQ. The structure of the final insert was: ex1 (267 bp) – ivs1 (234 bp)//ivs1 (251 bp) – ex2 (132 bp) – ivs2 (234 bp)/EcoRI/ivs2 (449 bp) – ex3 (88 bp) – ivs3 (283 bp) – ex4 (220 bp) – ivs4 (553 bp) (Figure 1). Minigene mgUGT1A1_ex1-4 was sequenced to check the presence of the wild type or the mutant alleles. Therefore, three different minigenes were obtained: I) wild type; II) c.864+5G>T; and III) c.996+2_996+5del.
Figure 1. Insert sequence (2,717 bp) of minigene mgUGT1A1_ex1-4. Exons are indicated in uppercase and variants c.864+5G>T (intron 1) and c.996+2_996+5del (intron 2) are shown in red and underlined. The donor splice sites of exons 1 and 2 are boxed.
Approximately 105 MCF-7 cells were grown to 90% confluency in 0.5 ml of medium (Minimum Essential Medium Eagle, 10% Fetal Bovine Serum, 1% Non-Essential Amino Acid Solution, 2 mM). Cells were transfected with 1 μg of each construct and 2 μl of Lipofectamine LTX (Life Technologies, Carlsbad, CA, United States). To inhibit nonsense-mediated decay, a 4-hr incubation with cycloheximide 300 μg/ml (Sigma-Aldrich, St. Louis, MO, United States) was performed. RNA was purified with the GeneMATRIX Universal RNA Purification Kit (EURx, Gdańsk, Poland) including on-column DNase I digestion.
Retrotranscription was carried out with 400 ng of RNA and the RevertAid First Strand cDNA Synthesis Kit (Thermo Fisher Scientific) using the vector-specific primer RTPSPL3-RV (5′-TGAGGAGTGAATTGGTCGAA-3′). Samples were incubated at 42°C for 1 hr, followed by 5 min at 70°C. Then, 400 ng of cDNA (final volume of 50 μl) were amplified with SD6-PSPL3_RT-FW (5′-TCACCTGGACAACCTCAAAG-3′) and RTpSAD-RV (Patent P201231427) (full-length transcript 832 nt) using Platinum-Taq DNA polymerase (Thermo Fisher Scientific). Samples were denatured at 94°C for 2 min, followed by 35 cycles of 94°C/30 s, 60°C/30 s, and 72°C (1 min/kb), and a final extension step at 72°C for 5 min. RT-PCR products were run on 1% agarose gels and sequenced at the Macrogen facility in Madrid, Spain.
To quantify all transcripts, semiquantitative fluorescent RT-PCRs were undertaken in triplicate with primers SD6-PSPL3_RT-FW and RTpSAD-RV (FAM-labeled) and Platinum Taq DNA polymerase (Thermo Fisher Scientific) under standard conditions except that 26 cycles were herein applied (Fraile-Bethencourt et al., 2017, 2018). FAM-labeled products were run with LIZ-1200 Size Standard (Thermo Fisher Scientific) at the Macrogen facility and analyzed with Peak Scanner software V1.0 (Thermo Fisher Scientific). Only peak heights ≥ 50 RFU (Relative Fluorescence Units) were considered.
In silico analysis of the wild type and mutant sequences with MES revealed that variant c.864+5G>T severely affected the exon 1 donor site (6.99 → 2.28), while c.996+2_996+5del caused a complete disruption of the exon 2 donor site (5.96 → −11.02). Therefore, both variants were judged to have the potential to impair splicing and were consequently selected for subsequent functional analysis.
Then, the UGT1A1 minigene with exons 1 to 4 was constructed from patient DNA in several steps as indicated in Materials and Methods. Therefore, we obtained three different minigenes: wild type, c.864+5G>T and c.996+2_996+5del. Remarkably, our construct contained a chimeric fusion of the vector reporter exon V1 and UGT1A1 exon 1 that preserved its donor site (Figure 2A), so it was plausible to check variants affecting this site. Functional analysis of the wild type construct showed a stable transcript of the expected size (832 nt) and structure [V1 (28 nt)- ex1 (267 nt)- ex2 (132 nt)- ex3 (88 nt)- ex4 (220 nt)- V2 (97 nt)] (Figures 2B,C), so it was used for further testing.
Figure 2. Splicing functional assays of minigene mgUGT1A1_ex1-4. (A) Structure of the construct. Splicing reactions in eukaryotic cells are represented by broken lines; specific vector exon primers are shown as black arrows in V1 and V2. (B) Agarose gel electrophoresis of RT-PCR products (in triplicate) of the wild type and mutant minigenes: 1, c.996+2_996+5del; 2, c.864+5G>T. The 1-kb DNA plus ladder is shown on the left. (C) Fragment analysis of FAM-RT-PCR products of the wild type and mutant minigenes experiments. Transcripts are shown as blue peaks while the LIZ1200 size standard is shown as orange peaks. Below is shown an electropherogram where the wild type and mutant ones have been overlaid. In this case aberrant transcripts are shown as red peaks. (D) Sequences of aberrant transcripts. (E) Diagrams of splicing events in the full-length and anomalous transcripts.
Then, both mutant minigenes were transfected and the RNA was purified and retrotranscribed. RNA assays of variant c.864+5G>T showed two transcripts (Figures 2B,C) that were subsequently sequenced (Figure 2D). The main transcript was the aberrant isoform Δ(E1q141) (70.4 ± 0.8%), generated using an alternative donor site of exon 1 (MES = 5.88), 141 nt upstream (Figures 2D,E). This variant also produced the full-length transcript in a reasonable proportion (29.6 ± 0.8%). It was surmised that Δ(E1q141) would keep the reading frame (r.724_864del) that would lead to the loss of 47 amino acids (p.Val242_Gln288del) of the N-terminal domain that encodes for substrate specificity of UGT1A1. The overall conservation of the UGT1A1 protein in vertebrates is 30.2% (161 out of 533 amino acids; Supplementary Figure S1). Particularly, 12 amino acids (25.5%) of the interval Val242_Gln288 are strictly conserved in vertebrates and 25 in mammals (Figure 3A), suggesting a relevant role in UGT1A1 function.
Figure 3. Amino acid conservation of the deleted sequences of the anomalous transcripts (A) Δ(E1q141) and (B) Δ(E2). Protein sequences were aligned with the Align tool of the Uniprot database (https://www.uniprot.org/align/). The alignment file was visualized with SnapGene Viewer version 4.3.11. The conserved residues are highlighted and the colored bars above the protein sequence show the degree of conservation where a red bar indicates the maximum level of conservation (100%). Organisms: Hsap, Homo sapiens; Mmul, macaque (Macaca mulatta); Mmus, mouse (Mus musculus); Btar, cow (Bos Taurus); Fcat, cat (Felis catus); Ggal, chicken (Gallus gallus); Acar, Anole lizard (Anolis carolinensis); Trub, fugu (Takifugu rubripes).
In contrast, c.996+2_996+5del produced two aberrant transcripts to substantial degrees: exon 2 skipping [Δ(E2)] (r.865_996del; 64.0 ± 0.4%) and a 135-nt insertion of intron 2 [▼(E2q135)] (r.996_997ins996+5_996+139; 36.0 ± 0.4%) by alternative use of a cryptic intronic 5′ splice site (MES = 3.43). It was established that both transcripts, Δ(E2) and ▼(E2q135), would keep the reading frame [p.Glu289_Thr332del and p.Thr332_Val333ins(45), respectively]. Whereas Δ(E2) was predicted to delete 44 amino acids, ▼(E2q135) was speculated to insert 45 new amino acids with no similarities with any known human protein. In both cases, the resultant impact on protein function remains to be established. In any case, 18 out of the 44 deleted residues of exon 2 are conserved in vertebrates (36 in mammals), suggesting that they are essential for an adequate protein function (Figure 3B).
We previously identified two putative variants that affected the canonical donor splice sites of UGT1A1 exons 1 and 2 (Gailite et al., 2018). Both variants were predicted by MES to disrupt their respective splice site. Therefore, splicing assays were highly recommended to prove their impact on splicing. The UGT1A1 gene is almost exclusively expressed in liver cells; however, patient RNA was not available. Alternatively, splicing reporter minigenes constitute a valuable tool for the characterization of splicing outcomes and have been judged as comparable with expression studies performed using patient liver cells (Gantla et al., 1998).
The UGT1A1 gene generates several protein isoforms where the amino acid sequence from exon 1 is responsible for substrate specificity (e.g., bilirubin binding) and exons 2–5 are shared with other UGT1A isoforms (Girard et al., 2007). Additionally, UGT1A1 isoforms can undergo alternative splicing by using an alternative exon 5, accounting for 27 different mature mRNA species (Jones et al., 2012). UGT1A1 transforms lipophilic molecules (mainly unconjugated bilirubin) into soluble metabolites, enabling them to be excreted from the body. Decreased or zero activity of the protein leads to the accumulation of bilirubin, resulting in unconjugated hyperbilirubinemia syndromes such as Crigler-Najjar syndrome type II.
Therefore, we designed and constructed a UGT1A1 minigene with exons 1–4 as both variants had the potential to affect the splicing of exons 1 and 2 but not exon 5. Remarkably, the main limitations of minigenes are the first and last exons of any gene because the splicing vector (pSAD) contains a reporter vector exon V1 which launches the splicing reaction and a vector exon V2 which finishes it (Figure 2A). We had previously constructed a minigene with a fusion of the last exon of the BRCA2 gene (exon 27) and V2 (Acedo et al., 2015). Here, we report a chimeric V1-exon 1 that was generated by overlapping extension PCR. Moreover, this construct was fully functional and induced a full-length transcript of the expected size and sequence (Figures 2B,C). Likewise, the mutant constructs (c.864+5G>T and c.996+2_996+5del) yielded clean splicing patterns (Figure 2C), where three aberrant transcripts [Δ(E1q141), Δ(E2), and ▼(E2q135)] were identified with high resolution and sensitivity by fluorescent fragment analysis (Fraile-Bethencourt et al., 2019a). Thus, c.864+5G>T induced a deletion of 141 nt of exon 1, similar to previously reported variant c.864+1G>C (Gantla et al., 1998), but also a significant proportion of the full-length transcript (29.6%) (Figures 2B–E). Although in some cases splicing does not differ among different cell lines (Sanz et al., 2010; Sharma et al., 2014), these splicing outcomes have been obtained in MCF-7 cells (human breast adenocarcinoma) and should be further confirmed in liver cell lines.
Analysis of the aberrant transcripts suggested that active protein would not be formed. Δ(E1q141) was found to entail the deletion of 47 amino acids of the UGT1A1 N-terminal domain that encodes for substrate specificity (exon 1, 288 amino acids) (Kadakol et al., 2000). Hence, protein functionality would likely be altered. In contrast, the splicing tests of c.996+2_996+5del revealed two abnormal transcripts [Δ(E2) and ▼(E2q135)] (Figures 2B–E). Both RNA isoforms were found to keep the reading frame. The common exons 2 to 5 of the UGT1A1 genes encode for the domain that binds the donor substrate UDP-glucuronic acid (Kadakol et al., 2000). Moreover, exon 2 skipping [Δ(E2)] was predicted to result in the loss of 18 amino acids conserved in vertebrates (Figure 3). Consequently, it is plausible that either the loss of exon 2 codons or the insertion of 45 new amino acids [▼(E2q135)] would impair UGT1A1 function; however, our interpretation of these in-frame transcripts should be approached with a degree of caution. Interestingly, a missense variant at the conserved Gly 308 (p.Gly308Glu) has been reported as pathogenic at the UGT1A1 mutation database2, thus supporting the relevance of this protein region.
For Crigler-Najjar syndrome type II, the functional activity of UGT1A1 is below 10%. Our Crigler-Najjar syndrome type II patient with 29.6% expression of the canonical transcript from one allele (c.864+5G>T) does not appear to fulfill this criterion. Also family segregation data from our previous study suggested that each of the variants separately did not reduce UGT1A1 enzyme activity enough to cause Crigler-Najjar syndrome type II (Gailite et al., 2018).
However, there are two possible explanations as to why in vivo expression could be even more reduced. First, this patient is homozygous for the UGT1A1 promoter A(TA)7TAA variant which leads to 70% reduction in UGT1A1 expression. This may affect and strengthen the deleterious effect of variants located in the coding part of the gene (Kadakol et al., 2001). Secondly, homo- and heterodimerization of isoforms may have an impact on UGT1A1 activity, where the amount of wild type protein is reduced by dimerization with aberrant isoforms (Ghosh et al., 2001). This dominant-negative effect would account for some Crigler-Najjar syndrome type II patients with only one pathogenic allelic variant (Suzuki et al., 2014). Yet, this effect was not observed in the parents of the patient.
According to the American College of Medical Genetics guidelines (Richards et al., 2015), our functional studies now allow both variants to be classified as pathogenic as they fulfill the following criteria: PS3 (well-established in vitro or in vivo functional studies supportive of a damaging effect on the gene or gene product), PM2 (absent from controls (or at extremely low frequency if recessive) in Exome Sequencing Project, 1000 Genomes, or ExAC), PM3 (for recessive disorders, detected in trans with a pathogenic variant), PP3 [multiple lines of computational evidence support a deleterious effect on the gene or gene product (conservation, evolutionary, splicing impact, etc.)], and PP4 (patient’s phenotype or family history is highly specific for a disease with a single genetic etiology).
In conclusion, we have shown that functional assays utilizing minigenes are very useful for checking the splicing impacts of potential spliceogenic DNA variants. Such assays are absolutely essential for the clinical interpretation of this type of variants. Indeed, they allowed the molecular confirmation of Crigler-Najjar syndrome type II diagnosis in our patient. Furthermore, although the splicing vector pSAD was initially developed to study the breast cancer genes BRCA1 and BRCA2 (Acedo et al., 2015; Fraile-Bethencourt et al., 2017), it has also been demonstrated to be a powerful tool to test other disease genes such as SERPINA1 (Lara et al., 2014), CHD7 (Villate et al., 2018), and UGT1A1, as well as others currently under investigation3.
All datasets generated for this study are included in the article/Supplementary Material.
MK proposed the concept of the study and involved in the patient’s clinical characterization. MK, DR, and LG performed the patient’s genetic analysis and the family segregation analysis. LG and EV designed the functional study. AV-P, LS-M, and EV involved in construction of the minigenes and all steps of the functional study. EV and LG prepared the manuscript. All authors critically reviewed the manuscript.
The Scientific Laboratory of Molecular Genetics was funded by internal grants from the Riga Stradins University. EV was supported by grants from the Spanish Ministry of Science, Innovation and Universities, Plan Nacional de I + D + I 2013-2016, ISCIII (PI17/00227) co-funded by FEDER from Regional Development European Funds (European Union), and grant CSI242P18 (actuación cofinanciada P.O. FEDER 2014-2020 de Castilla y León) from the Consejería de Educación, Junta de Castilla y León. AV-P was supported by a predoctoral fellowship from the Consejería de Educación, Junta de Castilla y León (2018–2022). LS-M was supported by a predoctoral fellowship from the Spanish Association Against Cancer (AECC), Junta Provincial de Valladolid (2019–2023).
The authors declare that the research was conducted in the absence of any commercial or financial relationships that could be construed as a potential conflict of interest.
The Supplementary Material for this article can be found online at: https://www.frontiersin.org/articles/10.3389/fgene.2020.00169/full#supplementary-material
Acedo, A., Hernández-Moro, C., Curiel-García, Á, Díez-Gómez, B., and Velasco, E. A. (2015). Functional classification of BRCA2 DNA variants by splicing assays in a large minigene with 9 exons. Hum. Mutat. 36, 210–221. doi: 10.1002/humu.22725
Arias, I. M., Gartner, L. M., Cohen, M., Ezzer, J., Ben, and Levi, A. J. (1969). Chronic nonhemolytic unconjugated hyperbilirubinemia with glucuronyl transferase deficiency. Am. J. Med. 47, 395–409. doi: 10.1016/0002-9343(69)90224-1
Cartegni, L., Chew, S. L., and Krainer, A. R. (2002). Listening to silence and understanding nonsense: exonic mutations that affect splicing. Nat. Rev. Genet. 3, 285–298. doi: 10.1038/nrg775
Desmet, F.-O. O., Hamroun, D., Lalande, M., Collod-Béroud, G., Claustres, M., Béroud, C., et al. (2009). Human splicing finder: an online bioinformatics tool to predict splicing signals. Nucleic Acids Res. 37:e67. doi: 10.1093/nar/gkp215
Fraile-Bethencourt, E., Díez-Gómez, B., Velásquez-Zapata, V., Acedo, A., Sanz, D. J., and Velasco, E. A. (2017). Functional classification of DNA variants by hybrid minigenes: identification of 30 spliceogenic variants of BRCA2 exons 17 and 18. PLoS Genet. 13:e1006691. doi: 10.1371/journal.pgen.1006691
Fraile-Bethencourt, E., Valenzuela-Palomo, A., Díez-Gómez, B., Acedo, A., and Velasco, E. A. (2018). Identification of eight spliceogenic variants in BRCA2 Exon 16 by minigene assays. Front. Genet. 9:188. doi: 10.3389/fgene.2018.00188
Fraile-Bethencourt, E., Valenzuela-Palomo, A., Díez-Gómez, B., Caloca, M. J., Gómez-Barrero, S., and Velasco, E. A. (2019a). Minigene splicing assays identify 12 spliceogenic variants of BRCA2 Exons 14 and 15. Front. Genet. 10:503. doi: 10.3389/fgene.2019.00503
Fraile-Bethencourt, E., Valenzuela-Palomo, A., Díez-Gómez, B., Goina, E., Acedo, A., Buratti, E., et al. (2019b). Mis-splicing in breast cancer: identification of pathogenic BRCA2 variants by systematic minigene assays. J. Pathol. 248, 409–420. doi: 10.1002/path.5268
Gailite, L., Rots, D., Pukite, I., Cernevska, G., and Kreile, M. (2018). Case report: multiple UGT1A1 gene variants in a patient with Crigler-Najjar syndrome. BMC Pediatr. 18:317. doi: 10.1186/s12887-018-1285-6
Gantla, S., Bakker, C. T. M., Deocharan, B., Thummala, N. R., Zweiner, J., Sinaasappel, M., et al. (1998). Splice-site mutations: a novel genetic mechanism of Crigler-Najjar syndrome type 1. Am. J. Hum. Genet. 62, 585–592. doi: 10.1086/301756
Ghosh, S. S., Sappal, B. S., Kalpana, G. V., Lee, S. W., Chowdhury, J. R., and Chowdhury, N. R. (2001). Homodimerization of human bilirubin-uridine-diphosphoglucuronate glucuronosyltransferase-1 (UGT1A1) and its functional implications. J. Biol. Chem. 276, 42108–42115. doi: 10.1074/jbc.M106742200
Girard, H., Lévesque, E., Bellemare, J., Journault, K., Caillier, B., and Guillemette, C. (2007). Genetic diversity at the UGT1 locus is amplified by a novel 3′ alternative splicing mechanism leading to nine additional UGT1A proteins that act as regulators of glucuronidation activity. Pharmacogenet. Genomics 17, 1077–1089. doi: 10.1097/FPC.0b013e3282f1f118
GTEx Consortium, Laboratory Data Analysis &Coordinating Center (Ldacc)—Analysis Working Group, Statistical Methods groups—Analysis Working Group, Enhancing GTEx (eGTEx) groups, Nih Common Fund, and Nih/Nci, (2017). Genetic effects on gene expression across human tissues. Nature 550, 204–213. doi: 10.1038/nature24277
Jaganathan, K., Kyriazopoulou Panagiotopoulou, S., McRae, J. F., Darbandi, S. F., Knowles, D., Li, Y. I., et al. (2019). Predicting splicing from primary sequence with deep learning. Cell 176, 535.e24–548.e24. doi: 10.1016/j.cell.2018.12.015
Jones, N. R., Sun, D., Freeman, W. M., and Lazarus, P. (2012). Quantification of hepatic UDP glucuronosyltransferase 1A splice variant expression and correlation of UDP glucuronosyltransferase 1A1 variant expression with glucuronidation activity. J. Pharmacol. Exp. Ther. 342, 720–729. doi: 10.1124/jpet.112.192658
Kadakol, A., Ghosh, S. S., Sappal, B. S., Sharma, G., Chowdhury, J. R., and Chowdhury, N. R. (2000). Genetic lesions of bilirubin uridine-diphosphoglucuronate glucuronosyltransferase (UGT1A1) causing crigler-najjar and gilbert syndromes: correlation of genotype to phenotype. Hum. Mutat. 16, 297–306. doi: 10.1002/1098-1004(200010)16:4<297::aid-humu2>3.0.co;2-z
Kadakol, A., Sappal, B. S., Ghosh, S. S., Lowenheim, M., Chowdhury, A., Chowdhury, S., et al. (2001). Interaction of coding region mutations and the Gilbert-type promoter abnormality of the UGT1A1 gene causes moderate degrees of unconjugated hyperbilirubinaemia and may lead to neonatal kernicterus [4]. J. Med. Genet. 38, 244–249. doi: 10.1136/jmg.38.4.244
Lara, B., Martínez, M. T., Blanco, I., Hernández-Moro, C., Velasco, E. A., Ferrarotti, I., et al. (2014). Severe alpha-1 antitrypsin deficiency in composite heterozygotes inheriting a new splicing mutation QOMadrid. Respir. Res. 15:125. doi: 10.1186/s12931-014-0125-y
Lopez-Perolio, I., Leman, R., Behar, R., Lattimore, V., Pearson, J. F., Castéra, L., et al. (2019). Alternative splicing and ACMG-AMP-2015-based classification of PALB2 genetic variants: an ENIGMA report. J. Med. Genet. 56, 453–460. doi: 10.1136/jmedgenet-2018-105834
Maruo, Y., Nakahara, S., Yanagi, T., Nomura, A., Mimura, Y., Matsui, K., et al. (2016). Genotype of UGT1A1 and phenotype correlation between Crigler-Najjar syndrome type II and Gilbert syndrome. J. Gastroenterol. Hepatol. 31, 403–408. doi: 10.1111/jgh.13071
Pan, Q., Shai, O., Lee, L. J., Frey, B. J., and Blencowe, B. J. (2008). Deep surveying of alternative splicing complexity in the human transcriptome by high-throughput sequencing. Nat. Genet. 40, 1413–1415. doi: 10.1038/ng.259
Richards, S., Aziz, N., Bale, S., Bick, D., Das, S., Gastier-Foster, J., et al. (2015). Standards and guidelines for the interpretation of sequence variants: a joint consensus recommendation of the American College of medical genetics and genomics and the association for molecular pathology. Genet. Med. 17, 405–424. doi: 10.1038/gim.2015.30
Sanz, D. J., Acedo, A., Infante, M., Durán, M., Pérez-Cabornero, L., Esteban-Cardeñosa, E., et al. (2010). A high proportion of DNA variants of BRCA1 and BRCA2 is associated with aberrant splicing in breast/ovarian cancer patients. Clin. Cancer Res. 16, 1957–1967. doi: 10.1158/1078-0432.CCR-09-2564
Seppen, J., Bosma, P. J., Goldhoorn, B. G., Bakker, C. T., Chowdhury, J. R., Chowdhury, N. R., et al. (1994). Discrimination between Crigler-Najjar type I and II by expression of mutant bilirubin uridine diphosphate-glucuronosyltransferase. J. Clin. Invest. 94, 2385–2391. doi: 10.1172/JCI117604
Sharma, N., Sosnay, P. R., Ramalho, A. S., Douville, C., Franca, A., Gottschalk, L. B., et al. (2014). Experimental assessment of splicing variants using expression minigenes and comparison with in silico predictions. Hum. Mutat. 35, 1249–1259. doi: 10.1002/humu.22624
Suzuki, M., Hirata, M., Takagi, M., Watanabe, T., Iguchi, T., Koiwai, K., et al. (2014). Truncated UDP-glucuronosyltransferase (UGT) from a Crigler-Najjar syndrome type II patient colocalizes with intact UGT in the endoplasmic reticulum. J. Hum. Genet. 59, 158–162. doi: 10.1038/jhg.2013.138
Tammaro, C., Raponi, M., Wilson, D., and Baralle, D. (2014). BRCA1 Exon 11, a CERES (Composite Regulatory Element of Splicing) element involved in splice regulation. Int. J. Mol. Sci. 15, 13045–13059. doi: 10.3390/ijms150713045
Villate, O., Ibarluzea, N., Fraile-Bethencourt, E., Valenzuela, A., Velasco, E. A., Grozeva, D., et al. (2018). Functional analyses of a novel splice variant in the CHD7 gene, found by next generation sequencing, confirm its pathogenicity in a spanish patient and diagnose him with CHARGE syndrome. Front. Genet. 9:7. doi: 10.3389/fgene.2018.00007
Wagner, K.-H., Shiels, R. G., Lang, C. A., Seyed Khoei, N., and Bulmer, A. C. (2018). Diagnostic criteria and contributors to Gilbert’s syndrome. Crit. Rev. Clin. Lab. Sci. 55, 129–139. doi: 10.1080/10408363.2018.1428526
Wang, E. T., Sandberg, R., Luo, S., Khrebtukova, I., Zhang, L., Mayr, C., et al. (2008). Alternative isoform regulation in human tissue transcriptomes. Nature 456, 470–476. doi: 10.1038/nature07509
Wang, G.-S., and Cooper, T. A. (2007). Splicing in disease: disruption of the splicing code and the decoding machinery. Nat. Rev. Genet. 8, 749–761. doi: 10.1038/nrg2164
Keywords: UGT1A1, unconjugated hyperbilirubinemia, Crigler-Najjar, aberrant splicing, minigene, splice site
Citation: Gailite L, Valenzuela-Palomo A, Sanoguera-Miralles L, Rots D, Kreile M and Velasco EA (2020) UGT1A1 Variants c.864+5G>T and c.996+2_996+5del of a Crigler-Najjar Patient Induce Aberrant Splicing in Minigene Assays. Front. Genet. 11:169. doi: 10.3389/fgene.2020.00169
Received: 14 November 2019; Accepted: 12 February 2020;
Published: 06 March 2020.
Edited by:
Andrew Landstrom, Duke University, United StatesReviewed by:
Andrés Fernando Muro, International Centre for Genetic Engineering and Biotechnology, ItalyCopyright © 2020 Gailite, Valenzuela-Palomo, Sanoguera-Miralles, Rots, Kreile and Velasco. This is an open-access article distributed under the terms of the Creative Commons Attribution License (CC BY). The use, distribution or reproduction in other forums is permitted, provided the original author(s) and the copyright owner(s) are credited and that the original publication in this journal is cited, in accordance with accepted academic practice. No use, distribution or reproduction is permitted which does not comply with these terms.
*Correspondence: Linda Gailite, bGluZGEuZ2FpbGl0ZUByc3UubHY=; Eladio A. Velasco, ZWF2ZWxzYW1AaWJnbS51dmEuZXM=
†These authors have contributed equally to this work
Disclaimer: All claims expressed in this article are solely those of the authors and do not necessarily represent those of their affiliated organizations, or those of the publisher, the editors and the reviewers. Any product that may be evaluated in this article or claim that may be made by its manufacturer is not guaranteed or endorsed by the publisher.
Research integrity at Frontiers
Learn more about the work of our research integrity team to safeguard the quality of each article we publish.