- 1Department of Plant Biology, Faculty of Biological Sciences, Tarbiat Modares University (TMU), Tehran, Iran
- 2Department of Biodiversity and Conservation, Real Jardín Botánico (CSIC), Madrid, Spain
The Irano-Turanian floristic region spans a topographically complex and climatically continental territory, which has served as a source of xerophytic taxa for neighboring regions and is represented by a high percent of endemics. Yet, a comprehensive picture of the abiotic and biotic factors that have driven diversification within this biota remains to be established due to the scarcity of phylogenetic studies. Acantholimon is an important component of the subalpine steppe flora of the Irano-Turanian region, containing c. 200 cushion-forming sub-shrubby pungent-leaved species. Our recent molecular phylogenetic study has led to enlarging the circumscription of this genus to include eight mono- or oligospecific genera lacking the characteristic life-form and leaves. Using the same molecular phylogeny, here we investigate the tempo and mode of diversification as well as the biogeographic patterns in this genus, to test the hypothesis that a combination of key morphological innovations and abiotic factors is behind Acantholimon high species diversity. Molecular dating analysis indicates that Acantholimon s.l. started to diversify between the Late Miocene and the Pliocene and the biogeographic analysis points to an Eastern Iran–Afghanistan origin. Macroevolutionary models support the hypothesis that the high diversity of the genus is explained by accelerated diversification rates in two clades associated with the appearance of morphological key innovations such as a cushion life-form and pungent leaves; this would have favored the colonization of water-stressed, substrate-poor mountainous habitats along the newly uplifted IT mountains during the Mio-Pliocene. Given the apparent similarity of mountain habitats for most species of Acantholimon, we hypothesize that its current high species diversity responds to a scenario of non-adaptive radiation fueled by allopatric speciation rather than evolutionary radiation driven by ecological opportunity. Similar scenarios might underlie the high diversity of other speciose genera in the topographically complex Irano-Turanian landscape, though this remains to be tested with fine-grained distribution and climatic data.
Introduction
The disparity of organismal diversification rates (speciation minus extinction) and its causes are a hot topic in evolutionary biology (Ricklefs, 2006; Linder, 2008; Losos, 2010; Morlon et al., 2011; Pyron and Burbrink, 2013; Stadler, 2013; Morlon, 2014; Alexander et al., 2016; López-Estrada et al., 2018). Variations in the rates of speciation and extinction over time and across lineages and the factors underlying those changes are key to understanding how diversity is generated, which in turn is instrumental in designing how it can be preserved (Linder, 2005; Condamine and Hines, 2015; Sanmartín and Meseguer, 2016). Molecular phylogenies of species-rich groups are suitable models for investigating the tempo, rate and mode of diversification (Nee et al., 1992; Paradis, 1997; Pybus and Harvey, 2000; Harmon et al., 2003), and for inferring the spatial component of diversification by testing hypotheses about biogeography and migration patterns (Moore and Donoghue, 2007; Antonelli and Sanmartín, 2011; Condamine et al., 2012).
Evolutionary radiations are usually defined as involving rapid cladogenesis from a common ancestor and yielding taxon rich clades (Simpson, 1953; Ober and Heider, 2010). Most studies on radiations have focused on adaptive radiation, defined as “the evolution of ecological and phenotypic diversity within a rapidly multiplying lineage” (Schluter, 2000), a process in which ecological opportunity and key innovations are important features (Givnish, 2010; Yoder et al., 2010). While there is both empirical (Seehausen, 2006; Fior et al., 2013; Joly et al., 2013; Lagomarsino et al., 2016) and theoretical (Gavrilets and Vose, 2005) support for the occurrence of this model, which elements are central to the hypothesis of adaptive radiation remain controversial (Glor, 2010; Losos and Mahler, 2010; Givnish, 2015). For instance, in line with the view that adaptive radiation is distinct from ecological speciation, some models suggest that speciation during adaptive radiation is largely non-ecological and involves processes other than niche evolution (Grant and Grant, 2008; Rundell and Price, 2009; Glor, 2010). Other views question the focus on extraordinary diversification (Glor, 2010; Givnish, 2015), connecting with the old idea that adaptive radiation in a wider sense may be the predominant mode of biological diversification (Simpson, 1953; Stebbins, 1974). In addition, others openly advocate the role than non-adaptive radiation, i.e., involving niche conservatism, may play in radiative processes (Gittenberger, 1991; Kozak et al., 2006). Such alternative models and views on how radiations may be generated suggest that theoretical preconceptions should be minimized when examining new empirical data that involve rapid diversification in species-rich groups.
In flowering plants, there has been substantial research into the morphological, ecological (environmental), and physiological correlates of cladogenesis (Ree, 2005; Hughes and Eastwood, 2006; Lagomarsino et al., 2016; Meseguer et al., 2018). Whether abiotic factors such as climate and geography or the appearance of morphological evolutionary novelties are behind changes in a lineage's diversification trajectory is the subject of a rich recent literature (Donoghue and Edwards, 2014; Donoghue and Sanderson, 2015). Causality in correlations between key innovations and changes in diversification rates are difficult to demonstrate because evolution rarely affects a separate evolutionary trait (Donoghue and Sanderson, 2015), and often these changes appear only once in the phylogeny, preventing adequate statistical testing (Maddison and FitzJohn, 2015). Yet, the causal link between key innovation and rapid diversification is one of the major appeals of studies of radiations.
Covering one third of the surface of Eurasia, the Irano-Turanian (hereafter, IT) region is one of the largest floristic regions in the world (Takhtajan, 1986) but its limits have been debated and recently reviewed in Manafzadeh et al. (2017). It encompasses a vast topographically complex and climatically very continental territory (Djamali et al., 2012a) that includes two hotspots sensu Mittermeier et al. (2005), the Irano-Anatolian and the Mountains of Central Asia, as well as the southern and eastern parts of a third one, the Caucasus. Overall, the IT region extends from the Anatolian Plateau (excluding the southeastern Mediterranean fringe) and Levant region (up to northern part of the Sinai peninsula) on the west to the western China provinces of Gansu and western Sichuan on the east; and from southeastern Russia, Kazakhstan and Southern Mongolia on the north to the Iranian plateau (except the Iranian Gulf and Gulf of Oman), Afghanistan and the western Himalayas on the south. The IT biota, represented by a high percent of endemics, has also served as a source of xerophytic taxa for neighboring regions, in particular the Mediterranean region (Blondel et al., 2010; Manafzadeh et al., 2017), via migration corridors during dry climate episodes. Its dynamic geological history, resulting from the tectonic collision of three major plates, Eurasia, Arabia, and India, offers good opportunities for studying patterns of episodic biotic exchange between independently evolving biotas. Yet, the IT region has been scarcely studied from a biogeographical and in general an evolutionary point of view (Manafzadeh et al., 2014).
A key step in explaining the high botanical diversity of IT hotspots is demonstrating whether such diversity is the result of a rapid diversification compared to neighboring regions, and, if so, whether this is the result of a higher rate of speciation or a lower rate of extinction. The recent origin and radiation of IT species-rich groups, such as Cousinia Cass. subgen. Cousinia (c. 8.7 Mya; López-Vinyallonga et al., 2009) and Acanthophyllum C.A.Mey. s.l. (c. 11.1 Mya; Pirani et al., 2014), lends support to the idea of high diversification rates in the IT region, though old lineages with slower diversification rates also occur in this region, e.g., Haplophyllum A. Juss. (Manafzadeh et al., 2014). Yet, explicit comparisons of diversification rates between groups within and outside IT hotspots remain rare.
The IT region is characterized by a dynamic geological history, with several active tectonic lines, ongoing plate subduction (e.g., the African Plate beneath the Anatolian Plate), and several orogenic uplift events (e.g., Tibet, Caucasus). This has probably resulted in numerous opportunities for species formation, through geographic isolation (allopatry), colonization of novel environmental niches (mountain peaks and slopes), or adaptation to a more continental climate.
Acantholimon Boiss. is one of the richest angiosperm plant genera in the IT region and the second most species-rich genus in the Plumbaginaceae, with c. 200 species, most of which are geographically restricted (Mobayen, 1964; Kubitzki, 1993). All the species occur in the IT region but < 5% of them also occur in the Mediterranean region (Bunge, 1872; Mobayen, 1964; Linczevski, 1967; Kubitzki, 1993; Peng and Kamelin, 1996; Assadi, 2005, 2006; Dogan and Akaydin, 2007; Dogan et al., 2011). Acantholimon species have been traditionally recognized by their pulvinate (cushion forming) to densely-branched cespitose pungent subshrubby life-form. They occur in mountainous regions, mostly at mid- and higher elevations, growing in gravelly and stony soils or on exposed rocks. A recent molecular phylogenetic study, including c. two-thirds of the species diversity, has revealed that Acantholimon as currently defined is not monophyletic, and supports a widely circumscribed Acantholimon, with the small genus Goniolimon Boiss. (c. 20 species) as its sister group (Moharrek et al., 2017). This new definition of the genus—which we refer below as Acantholimon s.l.—includes the species-poor genera Bamiania Lincz., Bukiniczia Lincz., Chaetolimon (Bunge) Lincz., Cephalorhizum Popov & Korovin, Dictyolimon Rech.f., Gladiolimon Mobayen, Popoviolimon Lincz., and Vassilczenkoa Lincz., which differ markedly from Acantholimon s.str. in their overall morphology, in particular life-form and leaves. Moharrek et al. (2017) placed these genera, except for Gladiolimon, as early diverging lineages relative to two different clades that contained the species traditionally included within Acantholimon, hereafter Acantholimon s.str. Ancestral character state reconstruction of morphological traits revealed that the cushion life-form and pungent leaves, characteristic of Acantholimon (it is also present in the IT genus Acanthophyllum, Pirani et al., 2014), evolved independently in the two main Acantholimon s.str. clades (Moharrek et al., 2017). These authors suggested that the current high diversity of Acantholimon s.l. could be related to the evolution of these key innovations and driven by orography and climate change in the IT region. Yet, the absence of an explicit spatiotemporal framework prevented the statistical testing of this hypothesis. Because of its large species-richness in the IT region, compared to its sister genus Goniolimon, and the inclusion of species-poor and rich lineages, Acantholimon represents an ideal model system for testing the potential correlation between changes in diversification rates, morphological key innovations, and ecological opportunity in driving the high diversity of the IT region.
Here, we used the phylogenetic hypothesis of Acantholimon s.l. in Moharrek et al. (2017), c. 65% of all species, to investigate the tempo and mode of diversification and reconstruct the spatiotemporal evolution of this genus. Specifically, we aimed to test the hypothesis that key morphological innovations in life form are behind Acantholimon s.l.'s current high species diversity, and to explore the role that biogeographic events such as the colonization of new areas and abiotic factors (e.g., climate change toward aridification and continentality) played in the rapid diversification of this genus. For this, we (1) estimated the age of divergence of major lineages within Acantholimon using relaxed molecular clocks; (2) inferred point changes in diversification rates along the evolutionary history of Acantholimon over time and across clades; (3) tested for a causal relationship between transitions in life-form and leaf morphology and changes in speciation and extinction rates; and (4) reconstructed the biogeographic pathways by which this genus reached its current range. By reconstructing evolutionary patterns in the second largest genus of Plumbaginaceae, we also provide new insights on the evolution of this family.
Materials and Methods
Taxon Sampling
For the reconstruction of the temporal evolution and biogeographic patterns of the study group, we used a simplified dataset of that in Moharrek et al. (2017). The original was a combined dataset of nuclear ribosomal DNA ITS and plastid trnY-T sequences. Since no incongruence was found between the two markers, subsequent analyses were based on the concatenated nuclear-plastid matrix (2,021 bp). This dataset was trimmed to include a single accession per species covering 130 species of Acantholimon, as the ingroup, and 26 species, belonging to subfamilies Limonioideae and Plumbaginoideae, as the outgroup. The sampling encompasses the major areas of occurrence of Acantholimon in the IT region, including its main center of diversity in Iran and Afghanistan, as well as Anatolia, the southern Caucasus, Central Asia, and Pakistan. All samples except that of A. ulicinum from Greece lie within the limits of the IT region. Voucher information and GenBank accession numbers are shown in Data Sheet S1.
Divergence Time Estimation
Divergence times were inferred with BEAST v1.8.0 (Drummond et al., 2012a), ran at the CIPRES Science Gateway (http://www.phylo.org/; Miller et al., 2010) assuming a Bayesian relaxed molecular clock. Molecular rates were allowed to vary among lineages around an average value, by enforcing an uncorrelated lognormal clock of evolutionary rates. A birth–death branch process prior was used in the analysis. A general time reversible model with rate variation across sites, modeled using a gamma distribution and invariant proportion of sites (GTR + G + I), was applied to the concatenated data matrix.
There are no known macrofossils in Plumbaginaceae, which is mainly composed of herbs and subshrubs, but there is a pollen record. Pollen of Limonium Mill., Armeria Willd., Goniolimon, Acantholimon, Psylliostachys (Jaub. & Spach) Nevski, and most likely the other genera in the Limonioideae, are virtually indistinguishable. They consist of two morphs associated to an incompatibility system (Baker, 1948; Bokhari, 1972; Weber, 1981; Weber-El-Ghobary, 1987; Morretti et al., 2015) and are usually reported in palynological papers indistinctly either as Limonium or Armeria type. Using the available fossil pollen from the Upper Miocene (van Campo, 1976; Muller, 1981), Upper-Middle Miocene (Rivas-Carballo et al., 1994) or Middle Miocene (Valle et al., 1995) is thus problematic. Therefore, we calibrated our dating analysis with age estimates from a recently published time tree of angiosperms calibrated with 171 macrofossils (Magallón et al., 2015). Specifically, we calibrated the root node of our tree, the crown age of the family, using a normal distribution prior with median = 41.67 Mya and standard deviation (SD) = 10.8 Mya that covers the 95% high posterior density (HPD) credibility interval for that node in the original study (S. Magallón, pers. comm.). However, because the use of secondary calibrations has been recently criticized (Schenk, 2016), we used the Limonioideae pollen record as an additional calibration point, after performing a sensitivity analysis to explore the influence of the assignment of this fossil pollen to different nodes in our tree. Focusing conservatively on the Upper Miocene records only, we assigned the Limonium or Armeria type pollen record to the following nodes, in four alternative analyses (using exclusively this calibration): (1) the most-recent-common-ancestor (MRCA) of all Armeria, Psylliostachys, and Limonium species in our analysis, (2) the MRCA of Armeria and Psylliostachys species, (3) the MRCA of all Armeria species, and (4) the MRCA of all Limonium species in our analysis. These nodes were assigned a lognormal distribution prior with offset = 5.33 Mya, and SD = 1.15 Mya, covering the entire Upper Miocene geological interval (5.333–11.62 Mya). In doing this, we accounted for the possibility that the pollen belonged to either genus Armeria or Limonium, since these two genera are well-represented in the Iberian Peninsula where the original fossils were collected, or to a common ancestor of these two genera. Since the results of the analyses using the fossil pollen calibration point applied in four different positions were within the 95% HPD of each other (Table S1), we assigned the Limonioideae pollen record to the Armeria stem-node. We then performed the final dating analysis using two calibration points: the secondary calibration for the Plumbaginaceae crown-node and the fossil calibration for Armeria stem-node in our analysis (Data Sheet S2). The resulting chronogram (Figure 1) was used for the subsequent diversification and biogeographic analyses.
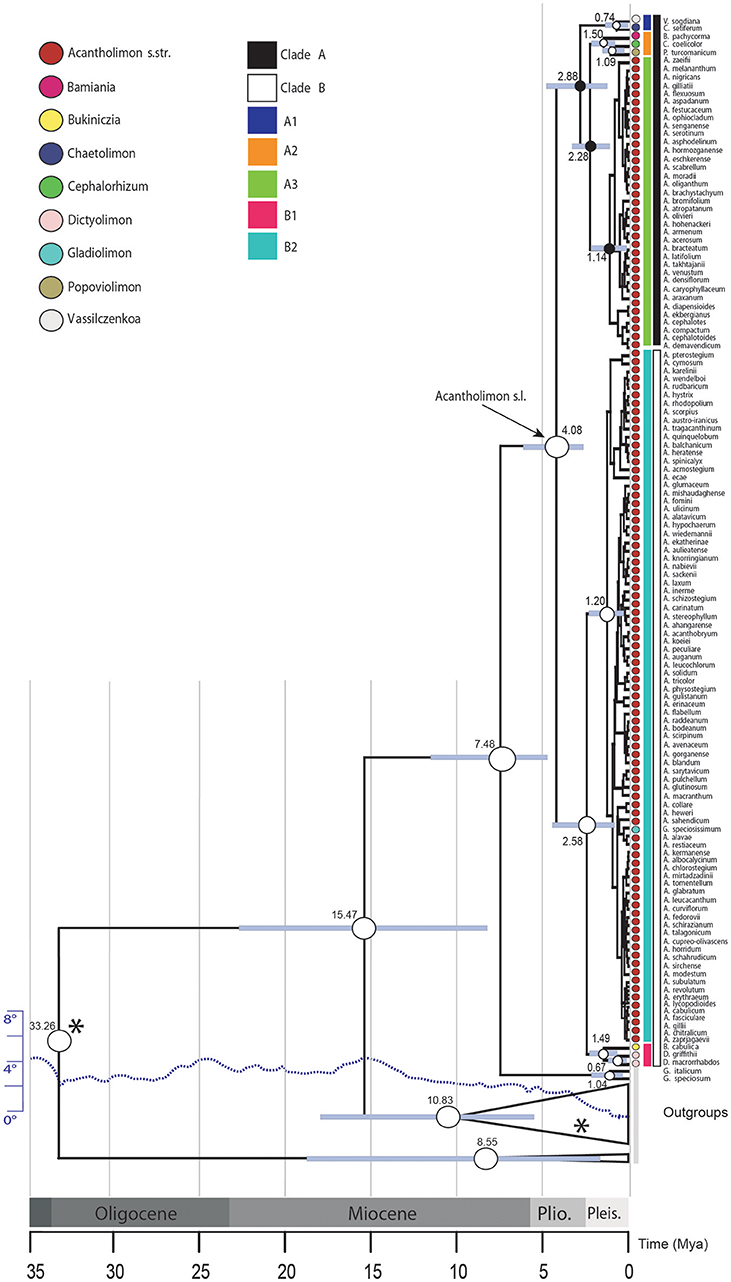
Figure 1. Bayesian inference analysis of Acantholimon s.l. based on two DNA regions (ITS and trnY-T). Chronogram represents the maximum clade credibility tree estimated in BEAST, with mean divergence dates in million years ago (Mya) shown for key nodes, some of which are labeled following Moharrek et al. (2017). Colored circles label samples according to formerly recognized genera as well as Acantholimon traditional circumscription. White dots denote strongly supported nodes (≥95% posterior probability, PP); black dots, intermediately supported nodes (85–94% PP); gray bars represent 95% highest posterior density credibility intervals for node ages. Asterisks indicate nodes used as calibration points. Dotted line, scaled on the left, indicates change of average global deep ocean temperature over time based on benthic foraminiferal oxygen-isotope records corrected to account for ice-sheet accumulation according to Hansen et al. (2008).
The MCMC chain was run in two separate analyses for 50 million generations sampling every 1000th generation. LogCombiner v1.7.5 (Drummond et al., 2012a) was used to combine the log and tree files after discarding the initial 5 million iterations of each analysis as burn-in. Tracer v1.6 (Rambaut et al., 2014) was used to assess that Effective Sample Sizes (ESS) were above 200 for optimal convergence and tree likelihood stationarity. Tracer automatically removed the first 10% of the log statistics from the combined sample, so we correspondingly discarded the first 10% of trees. A maximum clade credibility (MCC) tree was constructed in TreeAnnotator v1.7.5 (Drummond et al., 2012a) depicting the maximum sum of posterior clade probabilities (nexus file in Data Sheet S3). The MCC Tree was visualized in FigTree v1.4.1 (Drummond et al., 2012a).
Diversification Analyses
We used macroevolutionary methods implemented in R (R Development Core Team, 2018) to evaluate potential factors underlying diversification in Acantholimon. All analyses were run on the BEAST MCC tree pruned to leave only the 130 terminal taxa belonging to Acantholimon s.l., to avoid biases related to incomplete taxon sampling in the outgroup. We first visualized variation in diversification rates over time by plotting a semilogarithm lineage-through-time (LTT; Nee et al., 1992) plot with the R package ape (Paradis et al., 2004) using both the MCC tree and a random sample of 1,000 trees from the BEAST MCMC posterior distribution to account for dating uncertainty. Second, we used the whole-tree maximum likelihood methods implemented in the R package TreePar (Stadler, 2011) to detect the signal of mass extinctions and temporal shifts in the diversification rate using the MCC tree as phylogenetic hypothesis. The function “bd.shifts.optim” with the option ME = FALSE was used to estimate the time and magnitude of changes in the rate of diversification (r = speciation minus extinction) and background extinction (ε = extinction/speciation) at discrete points in time. Sampling fraction at present was set to rho = 0.65 to reflect incomplete taxon sampling, and we estimated potential rate shifts in an interval grid of every 0.1 Mya, using the option posdiv = FALSE to allow for negative diversification rates (periods of declining diversity). We compared a constant birth-death model against models with an increasing number of rate shifts by using likelihood ratio tests (LRTs) at a significant alpha level of 0.05 (Stadler, 2011). We also run analyses with the ME option set to TRUE to detect mass extinction events (MEEs), i.e., tree-wide sampling events in which a percentage of extant diversity is instantaneously removed at a point in time. This option assumes r and ε to be constant before and after the MEE but allows estimating the magnitude of the MEEs. Though in theory it is possible to estimate simultaneously tree-wide rate shifts and MEEs, in practice this leads to problems of parameter non-identifiability, since these two types of events are modeled identically in the likelihood BD framework (Stadler, 2011). We tested models with increasing number of MEEs via LRTs.
Third, we used Bayesian Analysis of Macroevolutionary Mixture (BAMM v2.5, Rabosky, 2014) to detect heterogeneity in evolutionary rates across lineages within the phylogeny of Acantholimon s.l. This method employs MCMC and Bayes Factor comparisons and Compound Poisson Process (CPP) models to explore alternative diversification dynamic regimes, allowing for both rate-heterogeneity across lineages and variation in speciation rates over time. To account for incomplete taxon sampling, we specified a global sampling probability of 0.65. The R package BAMMtools v2.1 (Rabosky et al., 2014) was used to choose appropriate prior distributions for all parameters in the analysis, and to analyze and visualize the output, using 15% of samples as burn-in. BAMM has been questioned, in particular its initial versions, because of the unrealistic treatment of extinction in unobserved (non-sampled or extinct) lineages and flawed CPP priors (Moore et al., 2016). The first one was suggested to be a minor bias for most datasets based on simulations (Rabosky et al., 2017), but its effect on empirical cases remains to be tested. Sensitivity to the choice of the CPP prior, which informs the expected number of rate shifts, was assessed by performing multiple BAMM analyses with varying CPP priors (1, 5, and 10). We ran each analysis for 5 million generations, sampling every 5,000th generation. Since the selected CPP prior had little effect on our results, we ran the final analysis with the default prior in BAMMtools (a prior rate shift of 1) and four independent chains of 10 million generations each, sampled every 10,000th generation.
Trait-Dependent Diversification Analyses
We tested for the effect of life-form and leaf type on diversification rates using the binary-state speciation and extinction (BiSSE) algorithm (Maddison et al., 2007) implemented in the DIVERSITREE R package (FitzJohn et al., 2009; FitzJohn, 2012). Coding of morphological states for each species was based on Moharrek et al. (2017), who used the taxonomic literature and direct observations to distinguish between two predominant overall morphologies or “syndromes” within Acantholimon s.l., defined mostly on the basis of life-form and leaves. The “Acantholimon syndrome” consists of a pulvinate to densely branched caespitose subshrub bearing mostly spike-like or capitate inflorescences, with linear rigid acuminate leaves, and occurs in Acantholimon s.str. and Gladiolimon. The “Limonium syndrome” is a perennial herb with thick rootstock, rosulate, spathulate, and slightly fleshy basal leaves, and flowering stems bearing paniculate inflorescences. This syndrome occurs in the species-poor Bamiania, Bukiniczia, Cephalorhizum, Dictyolimon, and Popoviolimon, but was here ascribed also to Chaetolimon and Vassilczenkoa since, although they do not exhibit spatulate leaves, their life-form is closer to the representatives of this syndrome than to those showing Acantholimon syndrome. We compared a model with syndrome-dependent speciation and extinction rates, and asymmetrical transition rates between the Acantholimon and Limonium syndromes (full BiSSE model, six parameters), against simpler, nested models where speciation, extinction or transition rates were constrained alternatively to be equal for the two states (five parameters). We assessed the significance of model differences by LRTs. To account for uncertainty in the estimation of model parameters, a Bayesian MCMC was run for 10,000 generations using an exponential prior under the best fitting model. Chain convergence was verified using the R package coda (Plummer et al., 2006). All chains converged within the first 1,000 generations. However, to be conservative, we discarded the first 2,500 steps of every chain and concatenated the last 7,500 steps for each tree together to construct the posterior probability distributions.
Ancestral Area Reconstruction
The biogeographic history of Acantholimon and allied genera was reconstructed using Lagrange (Ree and Smith, 2008) with the C++ implementation (http://code.google.com/p/lagrange). As for diversification, we removed all outgroup taxa. Lagrange implements the maximum likelihood dispersal–extinction–cladogenesis (DEC) model (Ree et al., 2005) to estimate the most likely ancestral geographic range based on current distributions of extant lineages. The DEC model has been used broadly to study biogeographic patterns in closely related taxa with restricted geographic ranges (e.g., Fabre et al., 2013). This model assumes extinction or dispersal by contraction or expansion of the ancestral geographic range, respectively, and allows estimating the probability of ancestral areas at each node of a phylogenetic tree. The range of Acantholimon s.l. and its close relatives was divided into seven major areas. In defining the boundaries of our operational areas, we considered the current distribution patterns of Acantholimon s.l. species, i.e., geographic areas defined by the congruence in distribution of two or more species, but also the existence of significant geological features that could have acted as barriers to dispersal, or whose appearance could have resulted in the formation of new species due to vicariance (Sanmartín, 2014). For instance, area B (Levant and E Anatolia) is separated from area A (Eastern Mediterranean region and W Anatolia) by the Anatolian Diagonal, an area that is both species-rich and seems to have functioned as barrier for many organisms (Davis, 1971; Avci, 1996). The large deserts lying in the middle of the Iranian plateau, Dasht-e Kavir and Dasht-e Lut, mark the geographical boundary between areas D (W Iran) and E (E Iran and Afghanistan). This “mixed” biotic-geological criterion was adopted to maximize congruence with other biogeographic studies on animal and plant organisms endemic to this region but with slightly different ecological and distribution patterns (Sanmartín, 2003; Roquet et al., 2009; Barres et al., 2013, etc.). We did not strictly follow Takhtajan's floristic regions because in our case it did not entirely capture the fine-grained distribution patterns in Acantholimon, and because boundaries between floristic regions in Takhtajan's classification reflect current geological and climatic settings but not necessarily older history. Use of Takhtajan's subregions within the IT floristic region was also problematic because of the intractability of a DEC analysis including more than 9 areas (Landis et al., 2013). The seven areas considered were: (A) Eastern Mediterranean region and W Anatolia including Greece and western Turkey; (B) Levant and E Anatolia; (C) Caucasus region including Georgia, Azerbaijan, and Armenia; (D) W Iran including most of the Iranian Plateau except for the eastern part; (E) Eastern Iran and Afghanistan including the eastern part of the Iranian plateau and Afghanistan; (F) Central Asia defined as the Turanian or trans-Caspian region, including the republics of Turkmenistan, Uzbekistan, Tajikistan, Kirzigistan, and most of Kazakhstan; and (G) Western Himalayas. The BEAST MCC tree was used for the Lagrange analysis. A geographical range matrix was constructed, coding each species as present or absent in each of the seven geographical areas. To reduce the size of this matrix and avoid non-identifiability with optimized ranges, we constrained widespread ancestral ranges to include only geographically adjacent areas assuming that transitions between widespread non-adjacent ancestral ranges involve an additional extinction event.
Results
Estimated Divergence Time
Acantholimon s.l. diverged from its sister genus 7.48 Mya (4.91–11.72 95% HPD) and started to diversify 4.08 Mya (2.61–6.22 95% HPD). Ages of the earliest diverging lineages within Acantholimon s.l., i.e., clades A and B, were estimated to be 2.88 Mya (1.21–4.88 95% HPD) and 2.58 Mya (0.98–4.28 95% HPD), respectively (Figure 1). Within clade A, non-cushion species corresponding to the former Vassilczenkoa and Chaetolimon (A1) diverged 0.74 Mya (0.22–1.46), whereas those corresponding to Cephalorhizum, Bamiania, and Popoviolimon (clade A2) diverged 1.50 Mya (0.79–2.37). Within clade B, the lineage including non-cushion species gathered in the former genera Dictyolimon and Bukiniczia (B1) diverged 1.49 Mya (0.53–2.10). Cushion forming species—exhibiting Acantholimon syndrome—originated around 1.14 Mya (0.19–1.88; clade A3) and 1.20 Mya (0.50–2.10; clade B2), respectively (Figure 1).
Diversification Analyses
The lineage-through-time (LTT) plot shows slow diversification before c. 1.5 Mya, after which the slope of the curve (rate of lineage accumulation) increases, accelerating toward 0.1 Mya (Figure 2). Among rate-variable models, TreePar supported a one-rate shift model, within Acantholimon s.l., with a slight increase of diversification rate and a joint marked decrease in turnover at time t = 0.015 Mya (Table 1). This model showed a significantly better fit (p = 0.99) to the curve than a constant birth-death model based on LRT tests; the two-rate-shift model was not significantly better than the one-rate-shift model (p = 0.83). The model supporting one MEE showed a better fit to the data than a constant birth-death model, albeit non-significant (p = 0.90), but worse than the one-rate-shift model (p = 0.99). Such MEE removed c. 89% of extant lineages at t = 1.81 Mya (sp = 0.11, Table 1). Subsequent models allowing for a higher number of MEEs were not significant (Table 1).
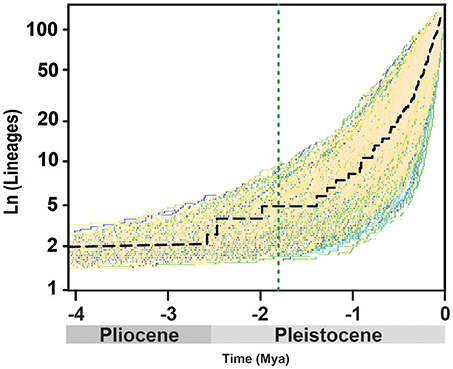
Figure 2. Accumulation of Acantholimon s.l. lineages through time, showing the maximum clade credibility tree (dash black line) and 1,000 trees from the post-burnin 95% highest posterior density distribution, representing uncertainty time estimates. The dotted green line marks a possible mass extinction event inferred by TreePar.
BAMM supported significant rate heterogeneity within Acantholimon s.l., recovering two significantly supported shifts in diversification at the crown nodes of Acantholimon s.str. species within clade A and clade B (A3, B2; Figures 1, 3A); the two most credible rate shift sets differed only in the position of the shift along the stem or crown node of Acantholimon s.str. species within clade B (B2; Figures 1, 3A). Mean diversification rates in clades A3 and B2 were inferred to be almost twice as high as in the rest of the tree (Table 2; Figure 3B). Rate-through-time plots showed that this was due to accelerated speciation rates, up to 7.6 (Figure 3A), starting c. 1 Mya (Figure 3B).
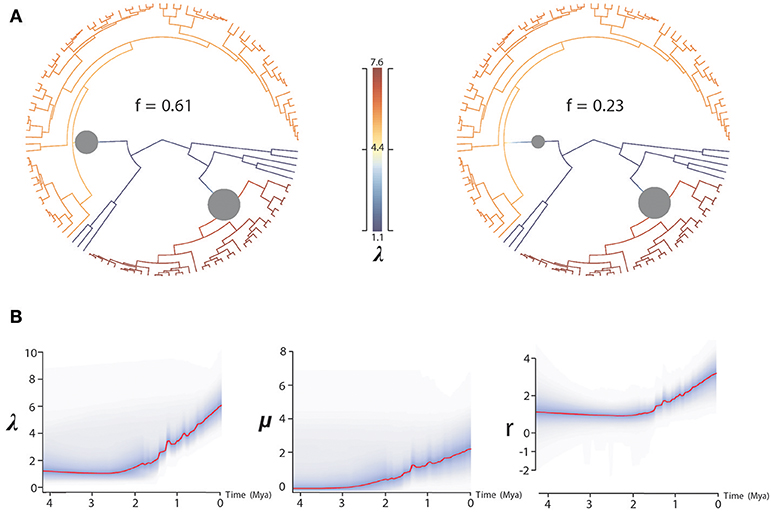
Figure 3. BAMM analysis of rate shifts in diversification within Acantholimon s.l. (A) Rate shift configurations (credible rate shift sets) with the highest posterior probability model showing two significant rate shifts in speciation (λ)—color coded by λ and marked by circles in the nodes. (B) Rates-through-time analysis of speciation (λ), extinction (μ) and net diversification (r) in Acantholimon s.l. over the last 4.08 Mya.
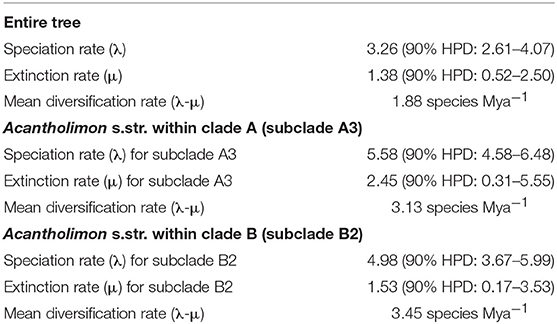
Table 2. Speciation (λ), extinction (μ) and mean diversification rates (λ-μ) for the entire tree, and for Acantholimon s.str. from BAMM.
Trait-dependent diversification models (BiSSE) suggest that the diversification rate heterogeneity detected by BAMM is an effect of morphological syndrome on diversification rates (Table S2). In particular, we detected a significant effect on speciation rates, as the “symmetric speciation” model was rejected for the MCC tree. Bayesian estimation of BiSSE parameters revealed significantly higher speciation and diversification rates for the Acantholimon syndrome than for the Limonium syndrome, as shown by the non-overlapping 95% credibility intervals obtained when analyzing the MCC tree (Figures 4A,C). No effect on extinction rates was detected (see the widely overlapping 95% credibility intervals in Figure 4B). Transition rates were not significantly different between syndromes, as indicated by overlapping Bayesian posterior densities of the estimated rate (Figure 4D).
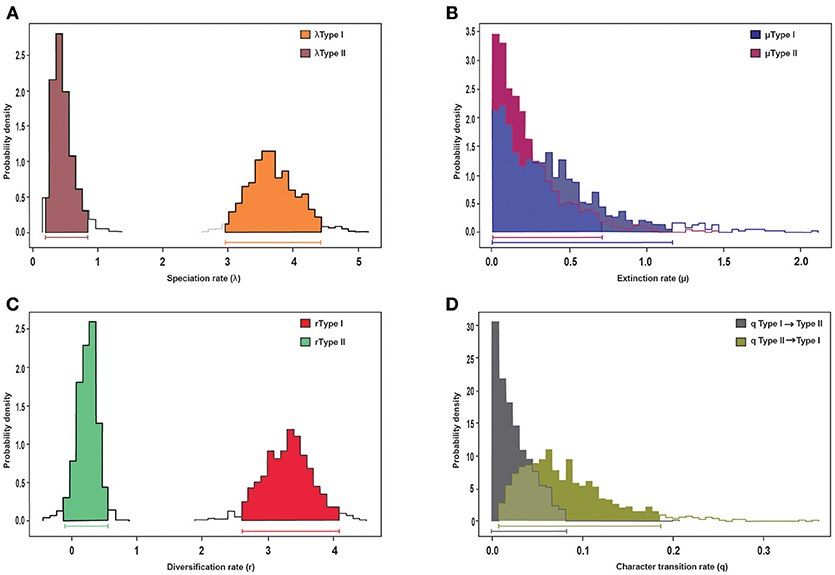
Figure 4. BiSSE analysis of diversification of the MCC tree and its association to morphological trait evolution, specifically life-form and leaves, represented by the Acantholimon (Type I) and the Limonium (Type II) syndromes. Posterior distributions of parameters obtained in the MCMC-BiSSE analysis: (A) speciation rates, (B) extinction rates, (C) diversification rates, (D) character transition rates. Horizontal bars indicate the 95% credibility interval for each parameter.
Ancestral Area Reconstruction
The eastern Iran–Afghanistan region (area E) was inferred as the most likely ancestral area for Acantholimon s.l., as well as for the two main lineages (clades A and B; Figure 5; Table S3). Many species were also reconstructed as having originated within this region. The ancestral area for the MRCA of Acantholimon s.str. species within clade B (subclade B2) was widespread in Western Iran and Eastern Iran–Afghanistan (areas DE), implying a dispersal event to the west. Within clade A, the ancestral area of the MRCA of Acantholimon s.str. species (subclade A3) was inferred to be widespread in Iran–Afghanistan and Central Asia (DEF). The other regions (A to C, and G) seem to have been colonized by later dispersal events westwards and eastwards, followed by peripatric speciation, or rarely vicariance (e.g., between C and D; Figure 5).
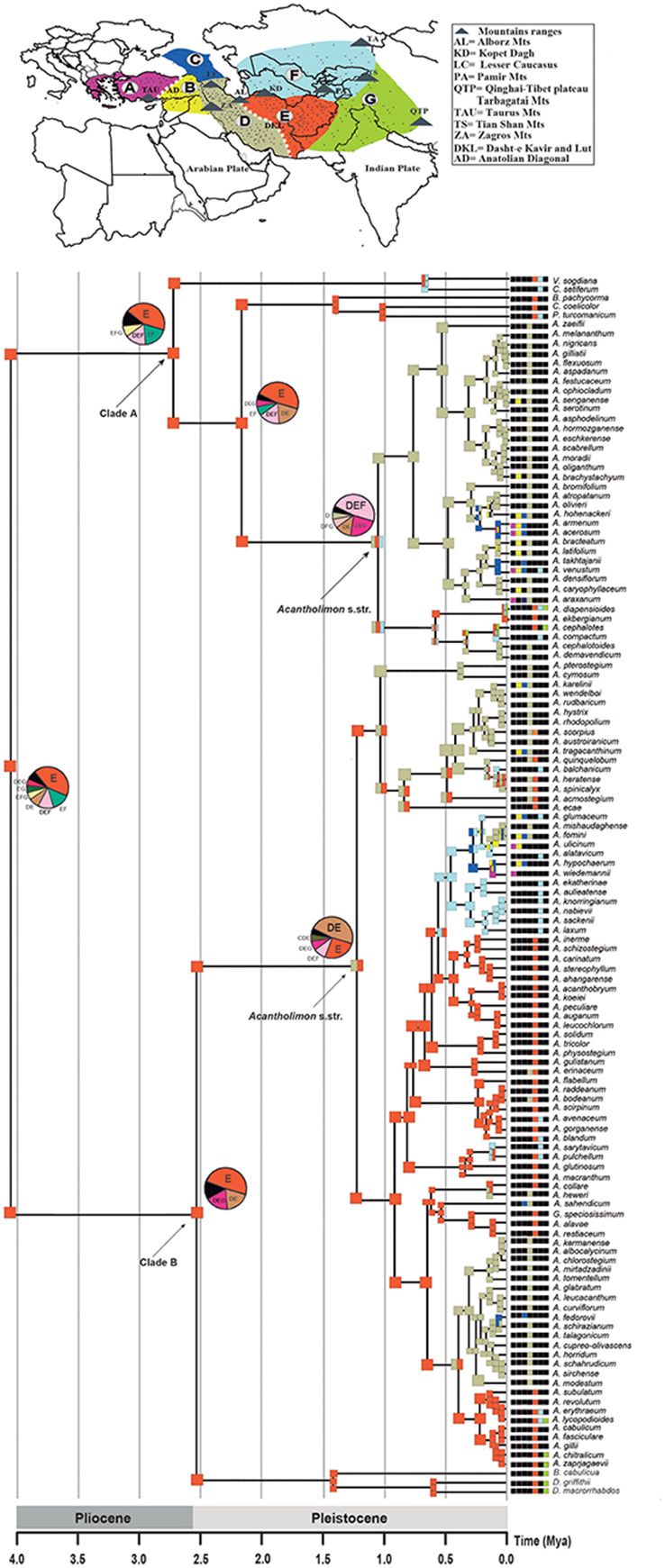
Figure 5. Ancestral Area Reconstruction of Acantholimon s.l. estimated in LAGRANGE using the seven operational areas shown in the map above. Current species distribution across the seven areas is indicated by color-coded squares next to the terminals; black squares indicate absence in the corresponding area. Ancestral areas and pie charts at nodes represent alternative range probabilities; black slices in pie charts indicate equivocal range probabilities. Scale depicts geological period time scale. An outline of Acantholimon distribution is provided by dots representing specimen locations obtained from GBIF.org (7th October 2015). GBIF Occurrence Available online at: (http://doi.org/10.15468/dl.aeqcxf).
Discussion
Parallel Diversification Rate Shifts and Key Innovations Explain Diversity Patterns Within Acantholimon
The uneven distribution of species diversity across clades and regions is a candent topic in evolutionary biology (Wiens, 2017). Species-rich clades can be explained by either their age of origin, i.e., older clades have more time to accumulate lineages (McPeek and Brown, 2007), or by events of rapid diversification (evolutionary radiations). Our analyses using several diversification rate methods support the hypothesis that Acantholimon s.l. species diversity resulted from two parallel events of accelerated speciation rates in subclades A3 and B2 (Figure 3A), which were linked to the acquisition of morphological novelties in habit and leaf form. Clade heterogeneity-aware method BAMM identified these two events, with only slight uncertainty on the timing along the stem/crown node of clade B2, and linked them to an increase in speciation rates (Figure 3). Trait-dependent diversification method BiSSE supported an association between diversification rates and morphological evolution: the (independent) acquisition of the Acantholimon syndrome, i.e., the joint occurrence of cushion life-form and linear rigid pungent leaves, was associated to higher speciation rates, with no significant effect on extinction rates, compared to the Limonium syndrome.
Time-dependent diversification models implemented in TreePar supported one rate shift toward higher diversification rates at 0.015 Mya, and a potential mass extinction event removing more than 90% of extant diversity at 1.81 Mya, which predates the clade-dependent rate shifts detected by BAMM (Figure 2, Table 1). However, we recommend caution in interpreting these results since it has been shown that methods such as TreePar exhibit a high Type II error in the case of rate heterogeneity due to clade-specific shifts (Laurent et al., 2015). Thus, sister-clades exhibiting different diversification dynamics such as in the case of Acantholimon s.str. species (A3, B2) and their species-poor relatives (A1, A2, B1) (Figure 1) could have misled the TreePar results. Yet, we cannot reject the possibility that high extinction rates in Acantholimon before the divergence of the major clades could have contributed to the observed diversification pattern.
Diversification rates estimated in Acantholimon (up to r = 3.45 species Myr−1; Table 2, Figure 3B) are comparable to those in other lineages proposed as examples of rapid radiations (e.g., Pelargonium L'Hér., Bakker et al., 2005; Lupinus L., Hughes and Eastwood, 2006; Drummond et al., 2012b; Astragalus L., Scherson et al., 2008; Castilleja Mutis ex L. f., Tank and Olmstead, 2008, 2009; Indigofera L., Schrire et al., 2009; Dianthus L.,Valente et al., 2010; Tragopogon L., Bell et al., 2012; Lobelioideae, Lagomarsino et al., 2016). These radiations have generally been attributed to ecological opportunity and/or evolutionary innovation, but relatively few studies have identified multiple diversification rate shifts coinciding with the evolution of the same derived traits (Drummond et al., 2012b). Our analyses with BAMM and BiSSE indicate that the newly arisen Acantholimon syndrome facilitated two parallel events of accelerated speciation rates in clades A3 and B2, suggesting that these morphological novelties in life form and leaf shape provided a competitive advantage for species in those lineages.
What could have been those advantages? We can hypothesize that dry continental environmental conditions, poor soils, and herbivory exerted strong selection pressures on Acantholimon s.l. lineages. Cushion life-form is a classic example of a convergent character that has repeatedly favored the colonization of cold and dry environments (Boucher et al., 2016). Not all species in the two speciose lineages (A3 and B2) are cushion-forming, but those that are not form densely branched subshrubs. Though there are some species living below 1,000 m in the Mediterranean parts of Anatolia (Dogan and Akaydin, 2007), most Acantholimon species occur in mountainous habitats, where cushion (or densely cespitose) life-forms are suitable to thrive. Only 14 (8.53%) of the 164 Acantholimon species recognized in the Flora Iranica include herbarium records below 1,000 m of elevation (Rechinger and Schiman-Czeika, 1974). And for 13 of those species with records below 1,000 m, there are additional records well above such elevation, even up to 3,000 m. The highest specimen recorded for this genus in Flora Iranica is at 4,650, 4,800 m in the Flora of China (Peng and Kamelin, 1996; note that Flora Iranica covers not only Iran but also Afghanistan and adjacent territories of Turkmenistan, Pakistan and Iraq).
The other morphological character associated to the parallel acceleration in diversification rates is leaf shape. Flattened leaves with an expanded limb occur in most of the genera merged under Acantholimon based on molecular data (Moharrek et al., 2017), i.e., Bamiania, Bukiniczia, Cephalorhizum, Dictyolimon, and Popoviolimon, as well as, profusely, in the outgroups, e.g., Goniolimon and Limonium. In contrast, those species included in the two highly diversified lineages (clades A3 and B2, Figure 1) typically show linear rigid acuminate leaves, representing a minimal leaf surface that reduces water loss, and whose pungency defends the plant against large herbivores. Nine of those 14 species with records below 1,000 m in Flora Iranica belong in sect. Tragacanthina Bunge. This was characterized by some degree of seasonal leaf dimorphism consisting of non-rigid spring leaves—a season when evapotranspiration is lower—and rigid summer leaves. This example suggests that rigid acuminate leaves may have been selected for extreme dry continental climates and that only Acantholimon species with relatively mild climate during part of the year exhibit non-rigid leaves. It is likely that the joint occurrence of these two characters—cushion life-form and rigid acuminate leaves—, with important functions for survival in extreme continental environments helped Acantholimon to become one of the dominant genera along vast extensions of the IT region. Interestingly, a strikingly similar morphological syndrome including life-form and leaves is exhibited by the genus Acanthophyllum, another large IT genus occurring in similar habitats, but belonging to another family, Caryophyllaceae (Pirani et al., 2014).
But, is the Acantholimon syndrome a key innovation? This term is subjected to controversy; possibly in part because of the recurring comparison with the most paradigmatic example, i.e., the pharyngeal jaw apparatus of cichlid fishes (Liem, 1973; Givnish, 2015). This anatomical structure triggered the invasion of a wide range of adaptive zones based on its maximum versatility. In contrast, the two morphological traits acquired independently in Acantholimon seem to lack the potential adaptive versatility of the pharyngeal jaw apparatus, but could have facilitated the colonization of vast territories of seemingly homogeneous continental high elevation environments and promoted a rapid subsequent diversification. It is in this sense that key innovation may be applied to the cushion life-form and pungent leaves in Acantholimon although alternatively a new term could be needed (Losos and Mahler, 2010).
Biogeographic History of Acantholimon
What was the climatic and geological framework within which Acantholimon evolved? Molecular dating analysis suggests that Acantholimon s.l. started to diversify sometime between the Late Miocene and Pliocene, whereas the onset of differentiation within the two lineages that constitute Acantholimon s.str. (clades A3 and B2) is dated in the Pleistocene (Figure 1). These dates are much younger than the tectonic and climatic events that shaped the current environmental conditions of the Irano-Turanian region. Climatically, the history of the region is shaped by both aridification and cooling. The Pleistocene glacial episodes took place over an already existing global gradual cooling trend that had started in the Oligocene (Zachos et al., 2008) and an aridification in central continental Asia that started by the early Miocene (Guo et al., 2002; Miao et al., 2012). Two major tectonic events shaped the topography and climate of IT region: the collisions of the Indian and Eurasian plates, started in the early Eocene (Yin, 2010; Smit et al., 2013), and that of the Arabian and Eurasian plates, started in the late Oligocene (Mouthereau et al., 2012). These two collisions triggered the uplifts of mountain ranges (Taurus, Caucasus, Zagros, Alborz, Kopet Dagh, Tian Shan, Pamir) and plateaus (Iranian, Anatolian, Qinghai-Tibetan) in the region, in a rather asynchronic and discontinuous fashion over the last 20 Ma (Li et al., 1996; Koçyigit et al., 2001; Wu et al., 2001; Meulenkamp and Sissingh, 2003; Popov et al., 2004; Guest et al., 2007; Buslov et al., 2008; Hatzfeld and Molnar, 2010; Mosar et al., 2010; Djamali et al., 2012b; Mouthereau et al., 2012; Favre et al., 2015). The uplift of these mountain ranges and plateau regions caused an increasing climate cooling and aridification through the formation of rain shadows on a large scale (Manafzadeh et al., 2017). It is in this context of increasing aridity and cooling in the IT region where diversification and expansion of Acantholimon species could have found favorable conditions over the last two million years. The Pleistocene date for the accelerated diversification events found in Acantholimon (Figure 1) contrasts with the older, Miocene, radiation reported in other speciose IT genera such as Cousinia (López-Vinyallonga et al., 2009; Djamali et al., 2012b). In this genus, Pleistocene climatic oscillations were inferred to have caused only moderately negative effects on preexisting diversification. By contrast, in Acantholimon, the altitudinal distribution of its cold-adapted xerophytic species, together with the inferred tempo of diversification, is consistent with an allopatric speciation scenario, in which Pleistocene climatic oscillations actively promoted diversification: range contraction and differentiation in isolation in the highest peaks during interglacial periods vs. range expansion and migration during glacial periods likely contributed to the divergence of gene pools and eventually to allopatric speciation.
The biogeographic analysis suggests that the origin of Acantholimon s.l. is most likely eastern Iran–Afghanistan; an area that may have acted as a “species pump” (Figure 5). This possibility is consistent with the fact that Iran and Afghanistan are the major centers of the taxonomic diversity of the genus (Kubitzki, 1993). The origin of the two major clades A and B is inferred to be eastern Iran–Afghanistan and migrations from this region appear to have occurred mainly following several lines: (1) westwards into W Iran and then northwards to the Caucasus, Anatolia and Eastern Mediterranean regions, (2) eastwards into the northern parts of Pakistan (Baluchistan) and the western Himalayas (India), (3) northwards into Central Asia and the Pamir mountains and then westwards to the Caucausus and Anatolia (Figure 5). A westward colonization of the Mediterranean region from IT lineages has been also described in other IT groups such as Haplophyllum (Manafzadeh et al., 2014). However, our phylogenetic results in Acantholimon indicate that most species from the same floristic region do not form monophyletic groups (Figure 5). The Anatolian and eastern Mediterranean representatives of the genus do not all cluster together but are embedded within clades that primarily include species from the inferred origin of the genus, Iran and Afghanistan (area E), and species that occur in more than one floristic region (Figure 5). This suggests that multiple westward invasions of the western regions from the core of the IT region took place during the evolution of the genus. A similar lack of monophyly is found for species occurring north and east of Iran and Afghanistan (areas F, G) although several central Asian species seem to have a single origin (A. glumaceum to A. laxum in clade B; Figure 5). Our results suggest that the IT region acted both as a cradle for new species, via high speciation rates, and as a museum, preserving older lineages that began to radiate outside this region. Additionally, one could ask about the relative importance of colonization vs. in situ diversification in assembling the IT biota (e.g., Xing and Ree, 2017). The high number of endemics in Acantholimon concentrated in an area inferred as the origin of the genus indicates a major contribution of in situ diversification.
Non-adaptive Radiation?
The elements and limits of the classical adaptive radiation model (Schluter, 2000; Glor, 2010; Givnish, 2015) and its relative importance compared to other models of evolutionary radiations (Simões et al., 2016) have been much debated. Niche modeling and niche overlap studies of every Acantholimon species are needed to determine the interactions between environmental factors and the fast diversification and associated key innovations documented in this genus. However, available data suggest that speciation in the IT Acantholimon was not accompanied by niche differentiation. A large amount of information on habitats of Acantholimon species recorded in floras, herbarium records, regional works (Linczevski, 1967; Rechinger and Schiman-Czeika, 1974; Peng and Kamelin, 1996; Assadi, 2005; Dogan and Akaydin, 2007) and personal observations consistently depict a rather uniform habitat: stony mountain slopes or summit areas with poorly developed soils, often dry, sometimes directly on rocky outcrops both limestone and granite. These environments where Acantholimon species occur are frequent and even dominant in the IT region, and host also other species-rich genera, such as Acanthophyllum, Astragalus, Cousinia, Haplophyllum, Onobrychis Mill. (Podlech and Maassoumi, 2003; Ranjbar and Karamian, 2003; López-Vinyallonga et al., 2009; Djamali et al., 2012b; Manafzadeh et al., 2014; Pirani et al., 2014; Amirahmadi et al., 2016).
If such a lack of niche differentiation is confirmed in Acantholimon species, a non-adaptive model, i.e., involving niche conservatism (Gittenberger, 1991; Kozak et al., 2006; Rundell and Price, 2009), would be the best explanation for its diversification patterns. Specifically, such a scenario could approach a geographic radiation model sensu Simões et al. (2016) and Givnish (2015), which states that geographic speciation in “extensive cordilleras, archipelagoes, lake systems or submarine outcrops dissected by multiple natural barriers to gene flow and species dispersal” can also result in explosive diversification.
In sum, rapid diversification in Acantholimon was probably facilitated by colonization of available mountainous environments enabled by key innovations, and by climatic-driven Pleistocene range shifts. Further work is needed to assess the role that niche differentiation vs. allopatry have played in its evolutionary radiation and the contribution of those elements to the assembly of the entire IT flora.
Data Availability Statement
The xml file used for the molecular dating in BEAST and the resulting phylogenetic tree in nexus file format are published as Data Sheets S1 and S3, respectively, in the Supplementary Material.
Author Contributions
FM, GNF, and IS conceived and designed this study. FM made field collections and molecular data compilation with contributions from SK-O on both aspects. FM and IS analyzed the data with help from GNF. FM and GNF wrote the manuscript with important contributions from IS. All authors contributed to manuscript revision, read, and approved the submitted version.
Funding
This study was supported by grants from the Spanish Ministry of Economy and Competitivity—CGL2013-49097-C2-1-P and CGL2017-88500-P (AEI/FEDER, EU) to GNF, CGL2015-67849-P (MINECO/FEDER) to IS—; and a Systematics Research Fund (SRF) from the Systematics Association to FM. FM was also funded by the research council of the Tarbiat Modares University, through a Ph.D. student fellowship.
Conflict of Interest Statement
The authors declare that the research was conducted in the absence of any commercial or financial relationships that could be construed as a potential conflict of interest.
Acknowledgments
We are grateful to the curators of the herbaria cited in Data Sheet S1, to colleagues who provided samples such as Juli Caujapé, Andreas Fleischmann, Allan Forrest, Hajar Hassanpour, Charo Noya, Robabeh Shahi and Ernst Vitek, and to Susana Magallón for providing an unpublished estimated date for Plumbaginaceae. We also acknowledge support of the publication fee by the CSIC Open Access Publication Support Initiative through its Unit of Information Resources for Research (URICI).
Supplementary Material
The Supplementary Material for this article can be found online at: https://www.frontiersin.org/articles/10.3389/fgene.2018.00698/full#supplementary-material
Data Sheet S1. Voucher information: species name, locality, collector(s), voucher (herbarium), GenBank accession numbers for nrDNA ITS and trnY-T, respectively.
Data Sheet S2. XML file used for molecular phylogenetic dating of the complete dataset comprising 130 species from Acantholimon s.l. and 26 outgroup taxa, using the two-calibration point strategy (see main article).
Data Sheet S3. Maximum clade credibility (MCC) tree showing mean estimates and 95% high-posterior-density (HPD) credibility intervals obtained from the BEAST analysis of the molecular dataset comprising 130 species from Acantholimon s.l. and 26 outgroups taxa, using the two-calibration point strategy (see Table S1). The tree is the same as the one shown in Figure 1.
References
Alexander, H. K., Lambert, A., and Stadler, T. (2016). Quantifying age-dependent extinction from species phylogenies. Syst. Biol. 65, 35–50. doi: 10.1093/sysbio/syv065
Amirahmadi, A., Kazempour-Osaloo, S., Kaveh, A., Maassoumi, A. A, and Naderi, R. (2016). The phylogeny and new classification of the genus Onobrychis (Fabaceae-Hedysareae): evidence from molecular data. Plant Syst. Evol. 302, 1445–1456. doi: 10.1007/s00606-016-1343-1
Antonelli, A., and Sanmartín, I. (2011). Mass extinction, gradual cooling, or rapid radiation? Reconstructing the spatiotemporal evolution of the ancient angiosperm genus Hedyosmum (Chloranthaceae) using empirical and simulated approaches. Syst. Biol. 60, 596–615. doi: 10.1093/sysbio/syr062
Assadi, M. (2005). “Plumbaginaceae,” in Flora of Iran. Vol. 51, eds M. Assadi, Z. Jamzad, and A. A. Maassoumi (Tehran: Research Institute of Forests and Rangelands), 47–204.
Assadi, M. (2006). Distribution patterns of the genus Acantholimon (Plumbaginaceae) in Iran. Iranian J. Bot. 12, 114–120.
Avci, M. (1996). The floristic regions of Turkey and a geographical approach for Anatolian Diagonal. Rev. Dept. Geogr. Univ. Istanbul 3, 59–91.
Baker, H. G. (1948). Dimorphism and monomorphism in the Plumbaginaceae: I. A survey of the family. Ann. Bot. 12, 207–219.
Bakker, F. T., Marais, E. M., Culham, A., and Gibby, M. (2005). “Nested radiations in Cape Pelargonium,” in Plant Species-Level Systematics: New Approaches on Pattern and Process, eds F. T. Bakker, L. W. Chatrou, B. Gravendeel, and P. Pelser (Ruggell, Liechtenstein: Gantner Verlag), 75–100.
Barres, L., Sanmartín, I., Anderson, C. L., Susanna, A., Buerki, S., Galbany-Casals, M., et al. (2013). Reconstructing the evolution and biogeographic history of tribe Cardueae (Compositae). Am. J. Bot. 100, 867–882. doi: 10.3732/ajb.1200058
Bell, C. D., Mavrodiev, E. V., Soltis, P. S., Calaminus, A. K., Albach, D. C., Cellinese, N., et al. (2012). Rapid diversification of Tragopogon and ecological associates in Eurasia. J. Evol. Biol. 25, 2470–2480. doi: 10.1111/j.1420-9101.2012.02616.x
Blondel, J., Aronson, J., Bodiou, J.-Y., and Boeuf, G. (2010). The Mediterranean Region: Biological Diversity in Space and Time. Oxford: Oxford University Press.
Bokhari, M. H. (1972). A brief review of stigma and pollen types in Acantholimon and Limonium. Notes Roy. Bot. Gard. Edinburgh 32, 79–84.
Boucher, F. C., Lavergne, S., Basile, M., Choler, P., and Aubert, S. (2016). Evolution and biogeography of the cushion life form in angiosperms. Persp. Plant Ecol. Evol. Syst. 20, 22–31. doi: 10.1016/j.ppees.2016.03.002
Bunge, A. (1872). Die Gattung Acantholimon Boiss. Mém. Acad. Imp. Sci. St. Pétersbourg Sér. 7, 1–72.
Buslov, M. M., Kokh, D. A., and De Grave, J. (2008). Mesozoic-Cenozoic tectonics and geodynamics of Altai, Tien Shan, and Northern Kazakhstan, from apatite fission-track data. Russ. Geol. Geophys. 49, 648–654. doi: 10.1016/j.rgg.2008.01.006
Condamine, F. L., and Hines, H. M. (2015). Historical species losses in bumblebee evolution. Biol. Lett. 11:20141049. doi: 10.1098/rsbl.2014.1049
Condamine, F. L., Sperling, F. A. H., Wahlberg, N., Rasplus, J.-Y., and Kergoat, G. J. (2012). What causes latitudinal gradients in species diversity? Evolutionary processes and ecological constraints on swallowtail biodiversity. Ecol. Lett. 15, 267–277. doi: 10.1111/j.1461-0248.2011.01737.x
Davis, P. H. (1971). “Distribution patterns in Anatolia with particular reference to endemism,” in Plant Life of South-West Asia, eds P. H. Davis, P. C. Harper, and I. C. Hedge (dinburgh: Botanical Society of Edinburgh), 15–27.
Djamali, M., Baumel, A., Brewer, S., Jackson, S. T., Kadereit, J. W., López-Vinyallonga, S., et al. (2012b). Ecological implications of Cousinia Cass. (Asteraceae) persistence through the last two glacial—interglacial cycles in the continental Middle East for the Irano-Turanian flora. Rev. Palaeobot. Palynol. 172, 10–20. doi: 10.1016/j.revpalbo.2012.01.005
Djamali, M., Brewer, S., Breckle, S. W., and Jackson, S. T. (2012a). Climatic determinism in phytogeographic regionalization: a test from the Irano-Turanian region, SW and Central Asia. Flora 207, 237–249. doi: 10.1016/j.flora.2012.01.009
Dogan, H. M., Dogan, M., Akaydin, G., and Celep, F. (2011). Mapping and analysing the diversity of the genus Acantholimon taxa in Turkey by geographic information systems (GIS). Turkish J. Bot. 35, 91–110. doi: 10.3906/bot-0910-193
Dogan, M., and Akaydin, G. (2007). Synopsis of Turkish Acantholimon Boiss. (Plumbaginaceae). Bot. J. Linn. Soc. 154, 397–419. doi: 10.1111/j.1095-8339.2007.00663.x
Donoghue, M. J., and Edwards, E. J. (2014). Biome shifts and niche evolution in plants. Ann. Rev. Ecol. Evol. Syst. 45, 547–542. doi: 10.1146/annurev-ecolsys-120213-091905
Donoghue, M. J., and Sanderson, M. J. (2015). Confluence, synnovation, and depauperons in plant diversification. New Phytol. 207, 260–274. doi: 10.1111/nph.13367
Drummond, A. J., Suchard, M. A., Xie, D., and Rambaut, A. (2012a). Bayesian phylogenetics with BEAUti and the BEAST 1.7. Mol. Biol. Evol. 29, 1969–1973. doi: 10.1093/molbev/mss075
Drummond, C. S., Eastwood, R. J., Miotto, S. T., and Hughes, C. E. (2012b). Multiple continental radiations and correlates of diversification in Lupinus (Leguminosae): testing for key innovation with incomplete taxon sampling. Syst. Biol. 61, 443–460. doi: 10.1093/sysbio/syr126
Fabre, P. H., Pagès, M., Musser, G. G., Fitriana, Y. S., Fjeldså, J., Jennings, A., et al. (2013). A new genus of rodent from Wallacea (Rodentia: Muridae: Murinae: Rattini), and its implication for biogeography and Indo-Pacific Rattini systematics. Zool. J. Linn. Soc. 169, 408–447. doi: 10.1111/zoj.12061
Favre, A., Päckert, M., Pauls, S. U., Jähnig, S. C., Uhl, D., Michalak, I., et al. (2015). The role of the uplift of the Qinghai-Tibetan Plateau for the evolution of Tibetan biotas. Biol. Rev. 90, 236–253. doi: 10.1111/brv.12107
Fior, S., Li, M., Oxelman, B., Viola, R., Hodges, S. A., Ometto, L., et al. (2013). Spatiotemporal reconstruction of the Aquilegia rapid radiation through next-generation sequencing of rapidly evolving cpDNA regions. New Phytol. 198, 579–592. doi: 10.1111/nph.12163
FitzJohn, R. G. (2012). Diversitree: comparative phylogenetic analyses of diversification in R. Methods Ecol. Evol. 3, 1084–1092. doi: 10.1111/j.2041-210X.2012.00234.x
FitzJohn, R. G., Maddison, W. P., and Otto, S. P. (2009). Estimating trait-dependent speciation and extinction rates from incompletely resolved phylogenies. Syst. Biol. 58, 595–611. doi: 10.1093/sysbio/syp067
Gavrilets, S., and Vose, A. (2005). Dynamic patterns of adaptive radiation. Proc. Natl. Acad. Sci. U.S.A. 102, 18040–18045. doi: 10.1073/pnas.0506330102
Givnish, T. J. (2015). Adaptive radiation versus ‘radiation' and ‘explosive diversification': why conceptual distinctions are fundamental to understanding evolution. New Phytol. 207, 297–303. doi: 10.1111/nph.13482
Glor, R. E. (2010). Phylogenetic insights on adaptive radiation. Ann. Rev. Ecol. Evol. Syst. 41, 251–270. doi: 10.1146/annurev.ecolsys.39.110707.173447
Grant, P. R., and Grant, B. R. (2008). How and Why Species Multiply—The Radiation of Darwin's Finches. Princeton, NJ: Princeton University Press.
Guest, B., Guest, A., and Axen, G. (2007). Late tertiary tectonic evolution of northern Iran: a case for simple crustal folding. Global Planet. Change 58, 435–453. doi: 10.1016/j.gloplacha.2007.02.014
Guo, Z. T., Ruddiman, W. F., Hao, Q. Z., Wu, H. B., Qiao, Y. S., Zhu, R. X., et al. (2002). Onset of Asian desertification by 22 Myr ago inferred from loess deposits in China. Nature 416, 159–163. doi: 10.1038/416159a
Hansen, J., Sato, M., Kharecha, P., Beerling, D., Berner, R., Masson-Delmotte, V., et al. (2008). Target atmospheric CO2: where should humanity aim? Open Atmos. Sci. J. 2:217–231. doi: 10.2174/1874282300802010217
Harmon, L. J., Schulte, J. A., Larson, A., and Losos, J. B. (2003). Tempo and mode of evolutionary radiation in iguanian lizards. Science 301, 961–964. doi: 10.1126/science.1084786
Hatzfeld, D., and Molnar, P. (2010). Comparisons of the kinematics and deep structures of the Zagros and Himalaya and of the Iranian and Tibetan plateaus and geodynamic implications. Rev. Geophys. 48:304. doi: 10.1029/2009RG000304
Hughes, C., and Eastwood, R. (2006). Island radiation on a continental scale: exceptional rates of plant diversification after uplift of the Andes. Proc. Natl. Acad. Sci. U.S.A. 103, 10334–10339. doi: 10.1073/pnas.0601928103
Joly, S., Heenan, P. B., and Lockhart, P. J. (2013). Species radiation by niche shifts in New Zealand's rockcresses (Pachycladon, Brassicaceae). Syst. Biol. 63, 192–202. doi: 10.1093/sysbio/syt104
Koçyigit, A., Yilmaz, A., Adamia, S., and Kuloshvili, S. (2001). Neotectonics of East Anatolian Plateau (Turkey) and Lesser Caucasus: implication for transition from thrusting to strike-slip faulting. Geodin. Acta 14, 177–195. doi: 10.1080/09853111.2001.11432443
Kozak, K. H., Weisrock, D. W., and Larson, A. (2006). Rapid lineage accumulation in a non-adaptive radiation: phylogenetic analysis of diversification rates in eastern North American woodland salamanders (Plethodontidae: Plethodon). Proc. R. Soc. B 273, 539–546. doi: 10.1098/rspb.2005.3326
Kubitzki, K. (1993). “Plumbaginaceae,” in The Families and Genera of Vascular Plants. Vol. 2, eds K. Kubitzki, J. G. Rohwer, and V. Bittrich (Berlin: Springer), 523–530.
Lagomarsino, L. P., Condamine, F. L., Antonelli, A., Mulch, A., and Davis, C. C. (2016). The abiotic and biotic drivers of rapid diversification in Andean bellflowers (Campanulaceae). New Phytol. 210, 1430–1442. doi: 10.1111/nph.13920
Landis, M. J., Matzke, N. J., Moore, B. R., and Huelsenbeck, J. P. (2013). Bayesian analysis of biogeography when the number of areas is large. Syst. Biol. 62, 789–804. doi: 10.1093/sysbio/syt040
Laurent, S., Robinson-Rechavi, M., and Salamin, N. (2015). Detecting patterns of species diversification in the presence of both rate shifts and mass extinctions. BMC Evol. Biol. 15:157 doi: 10.1186/s12862-015-0432-z
Li, J., Fang, X., Ma, H., Zhu, J., Pan, B., and Chen, H. (1996). Geomorphological and environmental evolution in the upper reaches of the Yellow River during the late Cenozoic. Sci. China Earth Sci. 39, 380–390.
Liem, K. F. (1973). Evolutionary strategies and morphological innovations: cichlid pharyngeal jaws. Syst. Zool. 22, 425–441. doi: 10.2307/2412950
Linczevski, I. A. (1967). “Plumbaginaceae,” in Flora of the USSR. Vol. 18, eds B. K. Shishkin and E. G. Bobrov (Jerusalem/Washington: Israel Program for Scientific Translations, Smithsonian Institution and the National Science Foundation), 216–348.
Linder, H. P. (2005). Evolution of diversity. The Cape Flora. Trends Plant Sci. 10, 536–541. doi: 10.1016/j.tplants.2005.09.006
Linder, H. P. (2008). Plant species radiations: where, when, why? Phil. Trans. R. Soc. B 363, 3097–3105. doi: 10.1098/rstb.2008.0075
López-Estrada, E. K., Sanmartín, I., García-París, M., and Zaldívar-Riverón, A. (2018). High extinction rates and non-adaptive radiation explains patterns of low diversity and extreme morphological disparity in North American blister beetles (Coleoptera, Meloidae). Mol. Phylogenet. Evol. 130, 156–168. doi: 10.1016/j.ympev.2018.09.014
López-Vinyallonga, S., Mehregan, I., Garcia-Jacas, N., Tscherneva, O., Susanna, A., and Kadereit, J. W. (2009). Phylogeny and evolution of the Arctium–Cousinia complex (Compositae, Cardueae-Caruinae). Taxon 58, 153–171.
Losos, J. B. (2010). Adaptive radiation, ecological opportunity, and evolutionary determinism. Am. Nat. 175, 623–639. doi: 10.1086/652433
Losos, J. B., and Mahler, D. L. (2010). “Adaptive radiation: the interaction of ecological opportunity, adaptation, and speciation,” in Evolution Since Darwin: The First 150 Years, eds M. A. Bell, D. J. Futuyma, W. F. Eanes, and J. S. Levinton (Sunderland, MA: Sinauer), 381–420.
Maddison, W. P., and FitzJohn, R. G. (2015). The unsolved challenge to phylogenetic correlation tests for categorical characters. Syst. Biol. 64, 127–136. doi: 10.1093/sysbio/syu070
Maddison, W. P., Midford, P. E., and Otto, S. P. (2007). Estimating a binary character's effect on speciation and extinction. Syst. Biol. 56, 701–710. doi: 10.1080/10635150701607033
Magallón, S., Gómez-Acevedo, S., Sánchez-Reyes, L. L., and Hernández-Hernández, T. (2015). A metacalibrated time-tree documents the early rise of flowering plant phylogenetic diversity. New Phytol. 207, 437–453. doi: 10.1111/nph.13264
Manafzadeh, S., Salvo, G., and Conti, E. (2014). A tale of migrations from east to west: the Irano-Turanian floristic region as a source of Mediterranean xerophytes. J. Biogeogr. 41, 366–379. doi: 10.1111/jbi.12185
Manafzadeh, S., Staedler, Y. M., and Conti, E. (2017). Visions of the past and dreams of the future in the Orient: the Irano-Turanian region from classical botany to evolutionary studies. Biol. Rev. 92, 1365–1388. doi: 10.1111/brv.12287
McPeek, M. A., and Brown, J. M. (2007). Clade age and not diversification rate explains species richness among animal taxa. Am. Nat. 169, E97–E106. doi: 10.1086/512135
Meseguer, A. S., Lobo, J. M., Cornuault, J., Beerling, D., Ruhfel, B. R., Davis, C. C., et al. (2018). Reconstructing deep-time palaeoclimate legacies in the clusioid Malpighiales unveils their role in the evolution and extinction of the boreotropical flora. Global Ecol. Biogeogr. 27, 616–628. doi: 10.1111/geb.12724
Meulenkamp, J. E., and Sissingh, W. (2003). Tertiary palaeogeography and tectonostratigraphic evolution of the Northern and Southern Peri-Tethys platforms and the intermediate domains of the African–Eurasian convergent plate boundary zone. Palaeogeogr. Palaeoclim. Palaeoecol. 196, 209–228. doi: 10.1016/S0031-0182(03)00319-5
Miao, Y.-F., Herrmann, M., Wu, F.-L., Yan, X.-L., and Yang, S.-L. (2012). What controlled mid-late Miocene long-term aridification in Central Asia?—global cooling or Tibetan Plateau uplift: a review. Earth-Science Rev. 112, 155–172. doi: 10.1016/j.earscirev.2012.02.003
Miller, M. A., Pfeiffer, W., and Schwartz, T. (2010). “Creating the CIPRES Science Gateway for inference of large phylogenetic trees,” in Proceedings of the Gateway Computing Environments Workshop (GCE), November 14, 2010 (New Orleans, LA), 1–8.
Mittermeier, R. A., Gil, P. R., Hoffman, M., Pilgrim, J., Brooks, T., Mittermeier, C. G., et al. (2005). Hotspots Revisited: Earth's Biologically Richest and Most Endangered Terrestrial Ecoregions. Washington, DC: Conservation International.
Mobayen, S. (1964). Revision Taxonomique du Genre Acantholimon. Dissertation thesis. Université de Montpellier.
Moharrek, F., Kazempour-Osaloo, S., Assadi, M., and Feliner, G. N. (2017). Molecular phylogenetic evidence for a wide circumscription of a characteristic Irano-Turanian element: Acantholimon (Plumbaginaceae: Limonioideae). Bot. J. Linn. Soc. 184, 366–386. doi: 10.1093/botlinnean/box033
Moore, B. R., and Donoghue, M. J. (2007). Correlates of diversification in the plant clade Dipsacales: geographic movement and evolutionary innovations. Am. Nat. 170, S28–S55. doi: 10.1086/519460
Moore, B. R., Höhna, S., May, M. R., Rannala, B., and Huelsenbeck, J. P. (2016). Critically evaluating the theory and performance of Bayesian analysis of macroevolutionary mixtures. Proc. Natl. Acad. Sci. U.S.A. 113, 9569–9574. doi: 10.1073/pnas.1518659113
Morlon, H. (2014). Phylogenetic approaches for studying diversification. Ecol. Lett. 17, 508–525. doi: 10.1111/ele.12251
Morlon, H., Parsons, T. L., and Plotkin, J. B. (2011). Reconciling molecular phylogenies with the fossil record. Proc. Natl. Acad. Sci. U.S.A. 108, 16327–16332. doi: 10.1073/pnas.1102543108
Morretti, F., Puppi, G., Giuliani, C., and Conti, F. (2015). Heterostyly in Goniolimon italicum (Plumbaginaceae), endemic to Abruzzo (central Apennines, Italy). Anales Jard. Bot. Madrid 72:e014. doi: 10.3989/ajbm.2365
Mosar, J., Kangarli, T., Bochud, M., Glasmacher, U. A., Rast, A., Brunet, M. F., et al. (2010). Cenozoic-Recent tectonics and uplift in the Greater Caucasus: a perspective from Azerbaijan. Geol. Soc. Lond. Spec. Public 340, 261–280. doi: 10.1144/SP340.12
Mouthereau, F., Lacombe, O., and Vergés, J. (2012). Building the Zagros collisional orogen: timing, strain distribution and the dynamics of Arabia/Eurasia plate convergence. Tectonophysics 532–535, 27–60. doi: 10.1016/j.tecto.2012.01.022
Muller, J. (1981). Fossil pollen records of extant angiosperms. Bot. Rev. 47, 1–142. doi: 10.1007/BF02860537
Nee, S., Mooers, A. O., and Harvey, P. H. (1992). Tempo and mode of evolution revealed from molecular phylogenies. Proc. Natl. Acad. Sci. U.S.A. 89, 8322–8326. doi: 10.1073/pnas.89.17.8322
Ober, K. A., and Heider, T. N. (2010). Phylogenetic diversification patterns and divergence times in ground beetles (Coleoptera: Carabidae: Harpalinae). BMC Evol. Biol. 10:262. doi: 10.1186/1471-2148-10-262
Paradis, E. (1997). Assessing temporal variations in diversification rates from phylogenies: estimation and hypothesis testing. Proc. R. Soc. B 264, 1141–1147. doi: 10.1098/rspb.1997.0158
Paradis, E., Claude, J., and Strimmer, K. (2004). APE: analyses of phylogenetics and evolution in R language. Bioinformatics 20, 289–290. doi: 10.1093/bioinformatics/btg412
Peng, Z.-X., and Kamelin, R. V. (1996). “Plumbaginaceae,” in Flora of China. Vol. 15, eds Z. Y. Wu and P. H. Raven (St. Louis: Missouri Botanical Garden Press), 190–204.
Pirani, A., Zarre, S., Pfeil, B. E., Bertrand, Y. J., Assadi, M., and Oxelman, B. (2014). Molecular phylogeny of Acanthophyllum (Caryophyllaceae: Caryophylleae), with emphasis on infrageneric classification. Taxon 63, 592–607. doi: 10.12705/633.39
Plummer, M., Best, N., Cowles, K., and Vines, K. (2006). CODA: convergence diagnosis and output analysis for MCMC. R News 6, 7–11.
Podlech, D., and Maassoumi, A. A. (2003). New species of Astragalus L. (Fabaceae) from Iran, mainly of sects. Incani and Malacothrix. Feddes Rep. 114, 320–349. doi: 10.1002/fedr.200311002
Popov, S. V., Rögl, F., Rozanov, A. Y., Steininger, F. F., Shcherba, I. G., Kovac, M., et al. (2004). Lithological-Paleogeographic maps of Paratethys, 10 maps Late Eocene to Pliocene. Courier Forschungsinstitut Senckenberg, Band 250. Stuttgart: E. Schweizerbart.
Pybus, O. G., and Harvey, P. H. (2000). Testing macro–evolutionary models using incomplete molecular phylogenies. Proc. R. Soc. B 267, 2267–2272. doi: 10.1098/rspb.2000.1278
Pyron, A. R. M., and Burbrink, F. T. (2013). Phylogenetic estimates of speciation and extinction rates for testing ecological and evolutionary hypotheses. Trends Ecol. Evol. 28, 729–736. doi: 10.1016/j.tree.2013.09.007
R Development Core Team (2018). R: A Language and Environment for Statistical Computing. Vienna: R Foundation for Statistical Computing. Available online at: http://www.R-project.org/
Rabosky, D. L. (2014). Automatic detection of key innovations, rate shifts, and diversity-dependence on phylogenetic trees. PLoS ONE 9:e89543. doi: 10.1371/journal.pone.0089543
Rabosky, D. L., Grundler, M., Anderson, C., Title, P., Shi, J. J., Brown, J. W., et al. (2014). BAMM tools: an R package for the analysis of evolutionary dynamics on phylogenetic trees. Methods Ecol. Evol. 5, 701–707. doi: 10.1111/2041-210X.12199
Rabosky, D. L., Mitchell, J. S., and Chang, J. (2017). Is BAMM flawed? Theoretical and practical concerns in the analysis of multi-rate diversification models. Syst. Biol. 66, 477–498. doi: 10.1093/sysbio/syx037
Rambaut, A., Suchard, M. A., Xie, D., and Drummond, A. J. (2014). Tracer, Version 1.6. Available online at: http://beast.bio.ed.ac.uk/Tracer
Ranjbar, M., and Karamian, R. (2003). Some remarks on the genus Astragalus sect. Incani DC. in Iran. Bot. J. Linn. Soc. 143, 443–447. doi: 10.1111/j.1095-8339.2003.00215.x
Rechinger, K. H., and Schiman-Czeika, H. (1974). “Plumbaginaceae,” in Flora Iranica. Vol. 108, ed K. H. Rechinger (Graz: Akademische Druck-u), 1–158.
Ree, R. H. (2005). Phylogeny and the evolution of floral diversity in Pedicularis (Orobanchaceae). Int. J. Plant Sci. 166, 595–613. doi: 10.1086/430191
Ree, R. H., Moore, B. R., Webb, C. O., and Donoghue, M. J. (2005). A likelihood framework for inferring the evolution of geographic range on phylogenetic trees. Evolution 59, 2299–2311. doi: 10.1554/05-172.1
Ree, R. H., and Smith, S. A. (2008). Maximum likelihood inference of geographic range evolution by dispersal, local extinction, and cladogenesis. Syst. Biol. 57, 4–14. doi: 10.1080/10635150701883881
Ricklefs, R. E. (2006). Evolutionary diversification and the origin of the diversity-environment relationship. Ecology 87 (Suppl. 7), S3–S13. doi: 10.1890/0012-9658(2006)87[3:EDATOO]2.0.CO;2
Rivas-Carballo, M. R., Alonso-Gavilán, G., Valle, M. F., and Civis, J. (1994). Miocene palynology of the central sector of the Duero basin (Spain) in relation to palaeogeography and palaeoenvironment. Rev. Palaeobot. Palynol. 82, 251–264.
Roquet, C., Sanmartín, I., Garcia-Jacas, N., Sáez, L., Susanna, A., Wikström, N., et al. (2009). Reconstructing the history of Campanulaceae with a Bayesian approach to molecular dating and dispersal–vicariance analyses. Mol. Phyl. Evol. 52, 575–587. doi: 10.1016/j.ympev.2009.05.014
Rundell, R. J., and Price, T. D. (2009). Adaptive radiation, nonadaptive radiation, ecological speciation and nonecological speciation. Trends Ecol. Evol. 24, 394–399. doi: 10.1016/j.tree.2009.02.007
Sanmartín, I. (2003). Dispersal vs. vicariance in the Mediterranean: historical biogeography of the Palearctic Pachydeminae (Coleoptera, Scarabaeoidea). J. Biogeogr. 30, 1883–1897. doi: 10.1046/j.0305-0270.2003.00982.x
Sanmartín, I. (2014). “Biogeography,” in The Tree of Life, eds P. Vargas and R. Zardoya (Sunderland, MA: Sinauer Associates, Inc.), 555–576.
Sanmartín, I., and Meseguer, A. S. (2016). Extinction in phylogenetics and biogeography: from timetrees to patterns of biotic assemblage. Front. Genet. 7:35. doi: 10.3389/fgene.2016.00035
Schenk, J. J. (2016). Consequences of secondary calibrations on divergence time estimates. PLoS ONE 11:e0148228. doi: 10.1371/journal.pone.0148228
Scherson, R. A., Vidal, R., and Sanderson, M. J. (2008). Phylogeny, biogeography, and rates of diversification of New World Astragalus (Leguminosae) with an emphasis on South American radiations. Am. J. Bot. 95, 1030–1039. doi: 10.3732/ajb.0800017
Schrire, B. D., Lavin, M., Barker, N. P., and Forest, F. (2009). Phylogeny of the tribe Indigoferae (Leguminosae-Papilionoideae): geographically structured more in succulent-rich and temperate settings than in grass-rich environments. Am. J. Bot. 96, 816–852. doi: 10.3732/ajb.0800185
Seehausen, O. (2006). African cichlid fish: a model system in adaptive radiation research. Proc. R. Soc. B 273, 1987–1998. doi: 10.1098/rspb.2006.3539
Simões, M., Breitkreuz, L., Alvarado, M., Baca, S., Cooper, J. C., Heins, L., et al. (2016). The evolving theory of evolutionary radiations. Trends Ecol. Evol. 31, 27–34. doi: 10.1016/j.tree.2015.10.007
Smit, J. H. W., Cloetingh, S. A. P. L., Burov, E., Tesauro, M., Sokoutis, D., and Kaban, M. (2013). Interference of lithospheric folding in western Central Asia by simultaneous Indian and Arabian plate indentation. Tectonophysics, 602, 176–193. doi: 10.1016/j.tecto.2012.10.032
Stadler, T. (2011). Mammalian phylogeny reveals recent diversification rate shifts. Proc. Natl. Acad. Sci. U.S.A. 108, 6187–6192. doi: 10.1073/pnas.1016876108
Stadler, T. (2013). Recovering speciation and extinction dynamics based on phylogenies. J. Evol. Biol. 26, 1203–1219. doi: 10.1111/jeb.12139
Stebbins, G. L. (1974). Flowering Plants: Evolution Above the Species Level. Cambridge, MA: The Belknap Press, Harvard University Press.
Tank, D. C., and Olmstead, R. G. (2008). From annuals to perennials, phylogeny of subtribe Castillejinae (Orobanchaceae). Am. J. Bot. 95, 608–625. doi: 10.3732/ajb.2007346
Tank, D. C., and Olmstead, R. G. (2009). The evolutionary origin of a second radiation of annual Castilleja (Orobanchaceae) species in South America: the role of long distance dispersal and allopolyploidy. Am. J. Bot. 96, 1907–1921. doi: 10.3732/ajb.0800416
Valente, L. M., Savolainen, V., and Vargas, P. (2010). Unparalleled rates of species diversification in Europe. Proc. R. Soc. B 277, 1489–1496. doi: 10.1098/rspb.2009.2163
Valle, M. F., Gavilán, G. A., and Rivas-Carballo, M. R. (1995). Analyse palynologique préliminaire du Miocene dans le NE de la dépression du Duero (Aire de Belorado, Burgos, Espana). Geobios 28, 407–412. doi: 10.1016/S0016-6995(95)80017-4
van Campo, É. (1976). La Flore Sporopollinique du Gisement Miocène Terminal de Venta del Moro (Espagne). Dissertation thesis. Montpellier.
Weber, M. O. (1981). Pollen diversity and identification in some Plumbaginaceae. Pollen Spores 23, 321–348.
Weber-El-Ghobary, M. O. (1987). Pollen morphology in the genus Psylliostachys (Plumbaginaceae) and its taxonomic significance. Pollen Spores 29, 131–150.
Wiens, J. J. (2017). What explains patterns of biodiversity across the Tree of Life? New research is revealing the causes of the dramatic variation in species numbers across branches of the Tree of Life. BioEssays 39:1600128. doi: 10.1002/bies.201600128
Wu, Y., Cui, Z., Liu, G., Ge, D., Yin, J., Xu, Q., et al. (2001). Quaternary geomorphological evolution of the Kunlun Pass areas and uplift of the Qinghai-Xizang (Tibet) plateau. Geomorphology 36, 203–216. doi: 10.1016/S0169-555X(00)00057-X
Xing, Y., and Ree, R. H. (2017). Uplift-driven diversification in the Hengduan Mountains, a temperate biodiversity hotspot. Proc. Natl. Acad. Sci. U.S.A. 114, E3444–E3451. doi: 10.1073/pnas.1616063114
Yin, A. (2010). Cenozoic tectonic evolution of Asia: a preliminary synthesis. Tectonophysics, 488, 293–325. doi: 10.1016/j.tecto.2009.06.002
Yoder, J. B., Clancey, E., Des Roches, S., Eastman, J. M., Gentry, L., Godsoe, W., et al. (2010). Ecological opportunity and the origin of adaptive radiations. J. Evol. Biol. 23, 1581–1596. doi: 10.1111/j.1420-9101.2010.02029.x
Keywords: Acantholimon, allopatric speciation, ancestral area reconstruction, extinction, Irano-Turanian, key innovations, mountain habitats, rapid diversification
Citation: Moharrek F, Sanmartín I, Kazempour-Osaloo S and Nieto Feliner G (2019) Morphological Innovations and Vast Extensions of Mountain Habitats Triggered Rapid Diversification Within the Species-Rich Irano-Turanian Genus Acantholimon (Plumbaginaceae). Front. Genet. 9:698. doi: 10.3389/fgene.2018.00698
Received: 05 July 2018; Accepted: 12 December 2018;
Published: 21 January 2019.
Edited by:
Jordi López-Pujol, Spanish National Research Council (CSIC), SpainReviewed by:
Yaowu Xing, Xishuangbanna Tropical Botanical Garden (CAS), ChinaStefanie M. Ickert-Bond, University of Alaska Fairbanks, United States
Sara Manafzadeh, Universität Wien, Austria
Copyright © 2019 Moharrek, Sanmartín, Kazempour-Osaloo and Nieto Feliner. This is an open-access article distributed under the terms of the Creative Commons Attribution License (CC BY). The use, distribution or reproduction in other forums is permitted, provided the original author(s) and the copyright owner(s) are credited and that the original publication in this journal is cited, in accordance with accepted academic practice. No use, distribution or reproduction is permitted which does not comply with these terms.
*Correspondence: Gonzalo Nieto Feliner, bmlldG9AcmpiLmNzaWMuZXM=