- Centre for Tumour Biology, Barts Cancer Institute, CRUK Centre of Excellence, Queen Mary University of London, London, United Kingdom
Whilst cross-talk between stroma and epithelium is critical for tissue development and homeostasis, aberrant paracrine stimulation can result in neoplastic transformation. Chronic stimulation of epithelial cells with paracrine Fibroblast Growth Factor 10 (FGF10) has been implicated in multiple cancers, including breast, prostate and pancreatic ductal adenocarcinoma. Here, we examine the mechanisms underlying FGF10-induced tumourigenesis and explore novel approaches to target FGF10 signaling in cancer.
Introduction
The majority of secreted FGFs signal in an autocrine or paracrine manner, by binding to FGF receptors (FGFR1-4) on the surface of target cells. Alternative splicing of the immunoglobin-like domain III in FGFR1-FGFR3 produces two variants, IIIb and IIIc, which confer different ligand binding specificities. FGF10, a member of the FGF7 subfamily, signals in a paracrine manner through activation of the IIIb splice variants of FGFR2 (FGFR2b) and FGFR1 (FGFR1b), which are predominantly expressed on the surface of cells of epithelial origin. Whilst in vitro assays have shown FGF10 to activate FGFR1b more weakly than FGFR2b, FGFR1b activation in vivo may be achieved in scenarios where extracellular FGF10 reaches high concentrations (Zhang et al., 2006; Ornitz and Itoh, 2015). It has been suggested that FGF10 may also perform intracrine roles within FGF10-producing cells, primarily through trafficking to the nucleus. Whilst the functional significance of FGF10 nuclear localization remains to be determined, disrupted nuclear localization of FGF10 has been linked to lacrimo-auriculo-denot-digital syndrome (Mikolajczak et al., 2016).
FGF10 is known to be critical for brain, lung and limb development (Sekine et al., 1999; Hajihosseini et al., 2008) and contributes to wound healing and tissue repair by promoting cell migration and proliferation (Werner et al., 1994; Volckaert et al., 2011). Given this role of FGF10 in adult tissues, it is unsurprising that aberrant signaling of FGF10 through FGFR2b, and in some instances FGFR1b, contributes to the progression of a number of human cancers.
Fgf10 in Breast Cancer
Studies in Fgf10−/− and Fgfr2b−/− mouse embryos demonstrate that FGF10-FGFR2b signaling plays a key role in mammary gland development (Mailleux et al., 2002; Veltmaat et al., 2006). Whilst FGF10 is not expressed in the luminal epithelial cells of the normal human breast duct (Grigoriadis et al., 2006), transcription of the FGF10 gene is elevated 10% of breast carcinomas (Theodorou et al., 2004) and genome-wide association studies have identified variants near the FGF10 locus as a genetic risk factor for breast cancer susceptibility (Stacey et al., 2008). Similarly, SNPs affecting FGFR2b expression have been correlated with breast cancer susceptibility (Meyer et al., 2008; Fachal and Dunning, 2015) and amplification of FGFR1, occurring in up to 12% of breast cancer cases (Cerami et al., 2012; Gao et al., 2013; Pereira et al., 2016), is correlated with poor prognosis (Reis-Filho et al., 2006; Elbauomy Elsheikh et al., 2007). A number of in vitro studies have shed light on the cellular roles of FGF10-FGFR2/1 signaling in breast cancer cell behavior (Figure 1).
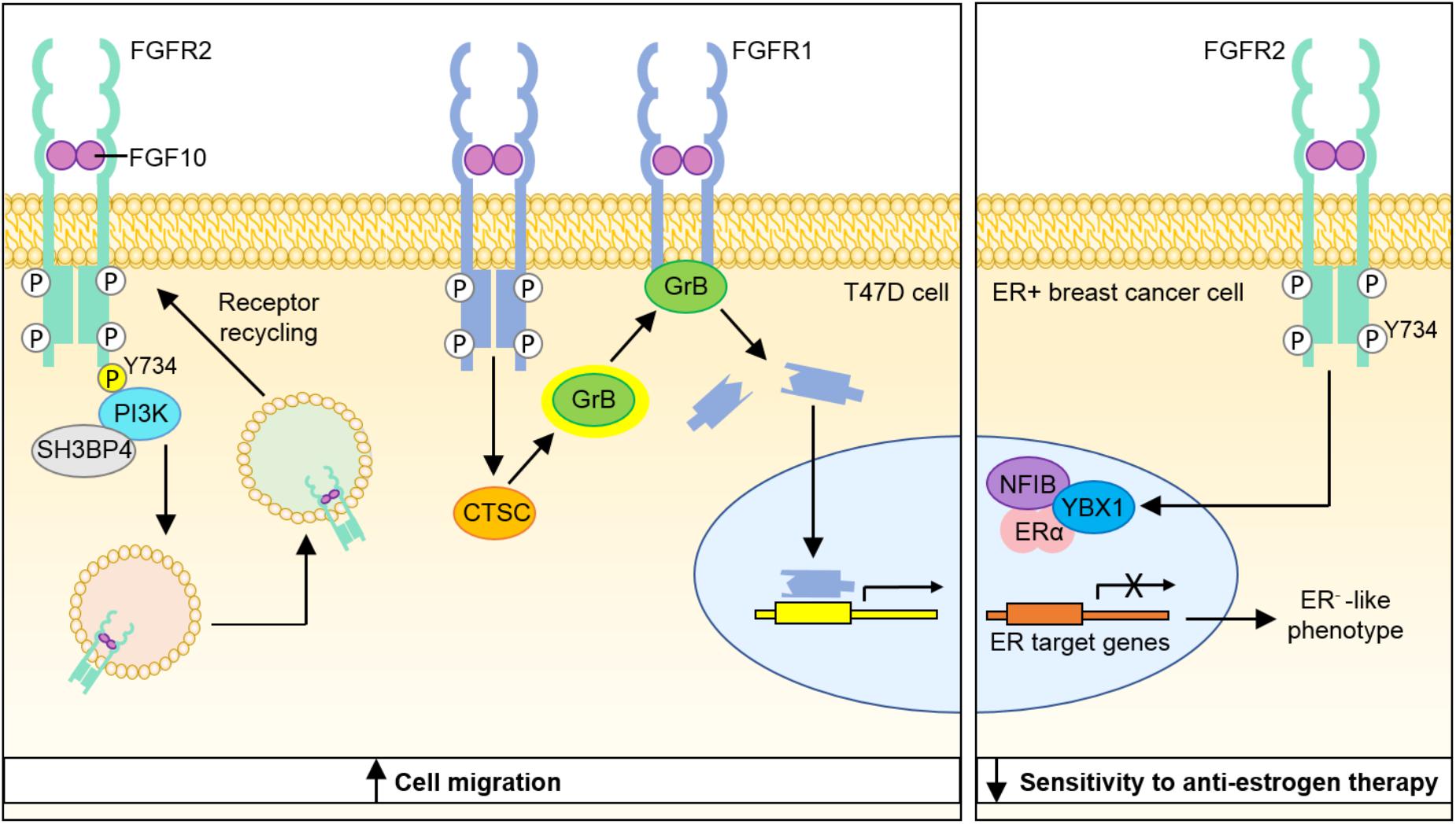
FIGURE 1. Model depicting molecular mechanisms through which FGF10-FGFR1 and FGF10-FGFR2 signaling may contribute to breast cancer progression. Binding of FGF10 to FGFR2b leads to phosphorylation of the receptor at Y734 and recruitment of PI3K and SH3BP4, which promote receptor recycling and increased cell migration. FGF10 binding to FGFR1 leads to cleavage of the receptor by granzyme B and the translocation of a 55 kDa fragment of FGFR1 to the nucleus, leading to increased cell migration. Stimulation of ER+ breast cancer cells with FGF10 enhances the interaction of NFIB and YBX1 with the ER and inhibits its transcriptional activity to produce a more ER− phenotype with lower sensitivity to anti-estrogen therapy. PI3K, phosphatidylinositide 3-kinase; SH3BP4, SH3-binding protein 4; CTSC, cathepsin C; GrB, granzyme B; NFIB, nuclear factor I B; YBX1, Y-Box Binding Protein-1; ERα, estrogen receptor α.
FGFR2 activation has been shown to repress the activity of the estrogen receptor (ER) regulon (Campbell T. M. et al., 2016), which has been correlated with poor prognosis in a cohort of ER+ breast cancer patients (Castro et al., 2016). Stimulation of the ER+ breast cancer cell line MCF-7 with FGF10 enhanced the interaction of the transcription factors NFIB and YBX1 with the ER, which inhibited its transcriptional activity and shunted the cells toward a more ER−, basal-like cancer phenotype with reduced estrogen dependency and lower sensitivity to anti-estrogen therapy. Treatment of ER+ breast cancer cell lines with the FGFR inhibitors AZD4547 and PD173074 sensitized the cells to the anti-estrogen tamoxifen, suggesting that targeting FGF10-FGFR2 signaling may offer a new approach to overcoming resistance to hormone-deprivation therapy in ER+ breast cancer (Campbell et al., 2018).
Nuclear localization of receptor tyrosine kinases (RTKs) has been documented for 12 RTK families (Chen and Hung, 2015) and has been correlated with poor prognosis in various cancers (Aleksic et al., 2010; Hadžisejdić et al., 2010; Traynor et al., 2013; Coleman et al., 2014). In vitro studies using breast cancer cell lines showed that FGF10 stimulation lead to the nuclear translocation of a 55 kDa C-terminal fragment of FGFR1, which in turn promoted the transcription of genes that stimulate cell migration and invasion. Cleavage of FGFR1 to yield this C-terminal fragment was found to be mediated by granzyme B activity, which was itself positively regulated by FGF10 stimulation. In 3D organotypic cell culture models, FGFR1 nuclear localization was most apparent in invading cells. Importantly, increased nuclear FGFR1 staining was also detected in tissue sections of invasive breast carcinoma (Chioni and Grose, 2012).
Analysis of FGFR2b signaling networks in vitro revealed that stimulation of FGFR2b with FGF10 promoted receptor recycling and led to an increase in breast cancer migration, whilst stimulation of FGFR2b with FGF7 resulted in receptor degradation and led to increased cell proliferation. Using quantitative proteomics to explore the mechanism underlying this functional dichotomy, it was revealed that FGF10 binding resulted in the novel phosphorylation of FGFR2b at Y734, which led to the recruitment of PI3K and SH3BP4 and targeting of the receptor to recycling endosomes. Whilst the mechanism through which FGFR2b recycling promotes cell migration is not fully understood, the role of FGFR2b-PI3K-SH3BP4 complex formation in this response to FGF10 was illustrated by experiments showing that FGF10-stimulated cell migration could be inhibited by depletion of SH3BP4 or expression of a FGFR2b-Y734F mutant (Francavilla et al., 2013).
FGF10 in Prostate Cancer
In vivo models have shown that elevated paracrine FGF10 stimulation of mouse prostate epithelial cells led to the development of adenocarcinoma, predominantly via activation of epithelial FGFR1. These lesions showed heightened levels of androgen receptor (AR), caused most likely by post-translational modifications augmenting AR stability. Following host castration, a subset of FGF10-induced prostate adenocarcinoma cells showed continued survival and proliferation, suggesting that paracrine FGF10 stimulation may contribute to the development of androgen independence in this murine model of prostate cancer (Memarzadeh et al., 2007). Later work using testicular feminized mice revealed that FGF10-induced prostate neoplasia is dependent on the expression of functional AR (Memarzadeh et al., 2011), demonstrating a role for FGF10-AR cross-talk in early prostate tumourigenesis.
AR expression has also been detected in prostate cancer-associated fibroblasts (CAFs) in vitro and siRNA-mediated depletion of AR from these stromal cells resulted in a decrease in FGF10 expression. Thus, there may exist a positive feedback loop whereby increased stromal cell AR levels promote the expression of FGF10, which then acts in a paracrine manner to elevate AR levels in prostate epithelial cells and potentially also in an autocrine manner to further elevate levels of AR in the stroma (Figure 2; Yu et al., 2013).
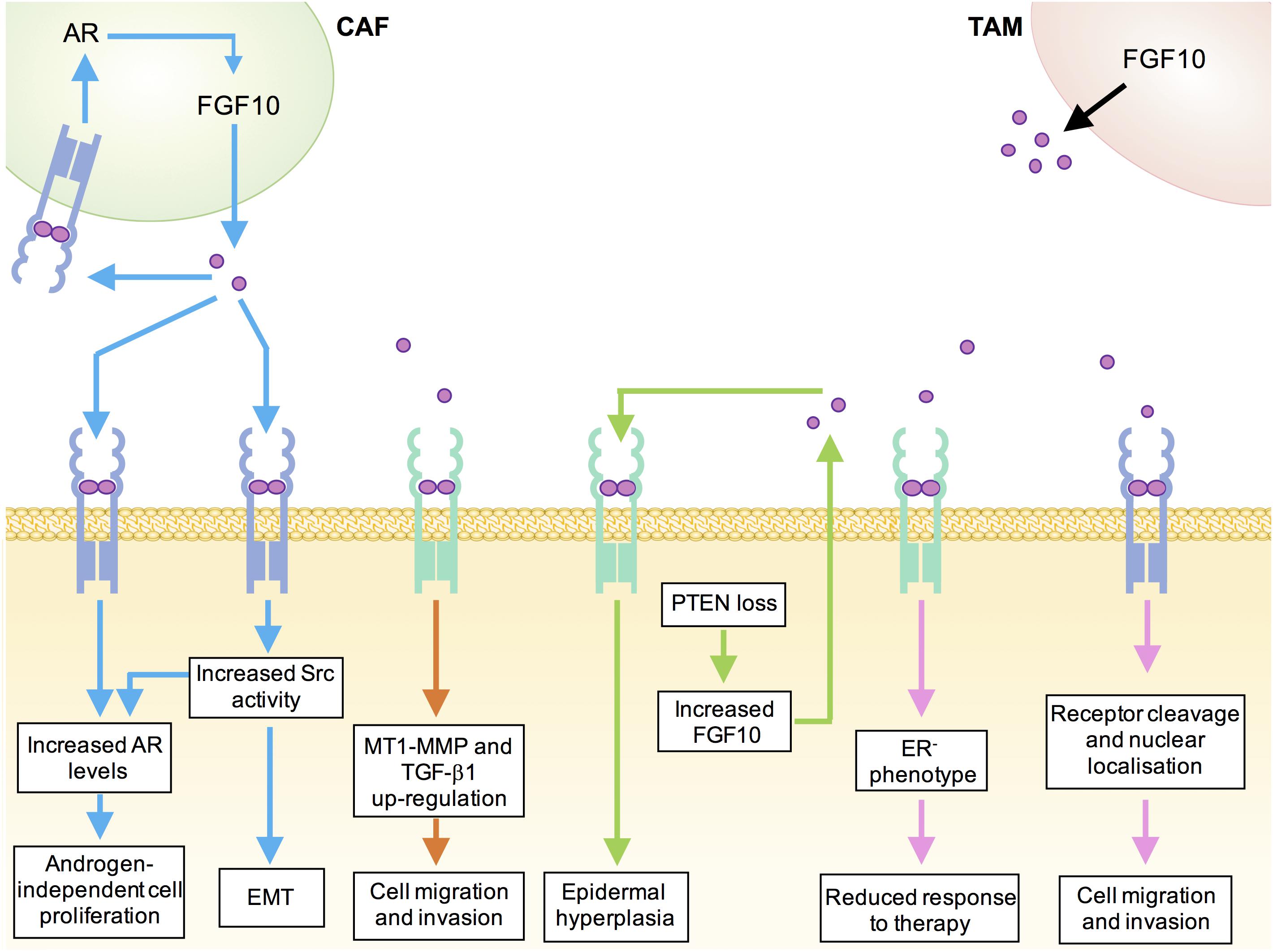
FIGURE 2. Mechanisms underlying tumor-promoting functions of FGF10 in human cancers. Blue, prostate cancer; orange, pancreatic ductal adenocarcinoma; green, cutaneous squamous cell carcinoma; pink, breast cancer; black, lung cancer; CAF, cancer-associated fibroblast; TAM, tumor-associated macrophage.
In a model of FGF10-induced prostate tumourigenesis, enhanced phosphorylation of Src-family kinases (SFKs) was detected in the adenocarcinoma lesions. Prostate epithelium from Src−/−Fyn+/− mice showed normal histology following exposure to elevated paracrine FGF10, suggesting that the tumourigenic effects of FGF10 on prostate epithelial cells are in part mediated by epithelial Src and Fyn kinases. Importantly, this work also established a link between FGF10, SFK signaling and AR levels by demonstrating that FGF10-stimulated Src−/−Fyn+/− xenografts showed downregulation of AR relative to FGF10-stimulated wild type xenografts (Cai et al., 2011). Recent in vivo studies have provided further evidence for a role of a FGF10/FGFR/Src signaling axis in prostate cancer. Ectopic expression of FGFR1, FGFR2, or Src in mouse prostate epithelial cells growing in a normal microenvironment was not sufficient to induce prostate tumourigenesis in vivo. However, paracrine FGF10 was found to synergize with FGFR1/2 over-expression to induce epithelial-mesenchymal transition and FGF10 synergized with Src overexpression to induce high-grade epithelial tumors. Importantly, inhibition of Src signaling, either by pharmacological Src kinase inhibitors or by deletion of the Src myristoylation site, inhibited paracrine FGF10-induced prostate tumourigenesis (Li et al., 2018b).
Whilst these studies in mouse models of prostate cancer have revealed potential novel roles for FGF10 in androgen signaling, it is important to note that elevated FGF10 has not been detected in human prostate cancer tissue (Abate-Shen and Shen, 2007; Eiro et al., 2017) and levels of FGF10 in the normal adult prostate are extremely low, compared to those of FGF7 (Ropiquet et al., 2000). Transfection of malignant prostate tumor cells with FGFR2b cDNA has been shown to reduce the growth rate of the derived tumors (Feng et al., 1997) and restoration of FGFR2b expression in castration-resistant prostate cancer cells increased sensitivity to chemotherapeutic agents (Shoji et al., 2014). Further investigation is therefore required to fully elucidate the complex roles of FGF10-FGFR2b signaling in prostate tumourigenesis.
FGF10 in Pancreatic Cancer
Whilst FGF10 expression is not detected in the normal adult human pancreas (Ishiwata et al., 1998), FGF10 is required for the proliferation of pancreatic epithelial progenitor cells and FGF10−/− mouse embryos show pancreatic hypoplasia, arrested pancreatic epithelial branching and an absence of islet cells (Bhushan et al., 2001). Expression of FGFR2 and FGFR1 are up-regulated in approximately 25% of pancreatic adenocarcinoma (PDAC) cases and elevated stromal FGF10 expression coupled with high cancer cell FGFR2b expression has been correlated with poor prognosis (Cerami et al., 2012; Gao et al., 2013; Bailey et al., 2016). In vitro studies using FGFR2b-expressing pancreatic cancer cell lines revealed that FGF10 stimulation promoted cancer cell migration and invasion through the up-regulation of MT1-MMP and TGF-β1 (Figure 2; Nomura et al., 2008).
More recent work aiming to identify diagnostic and predictive markers for pancreatic cancer found that FGF10 levels were elevated in the sera of untreated patients with PDAC compared to healthy controls and found that in combination with a panel of 4 other cytokine markers, FGF10 could be used as a diagnostic biomarker for PDAC (Torres et al., 2014).
FGF10 in Stomach Cancer
During development, FGF10 signaling plays a key role in stomach morphogenesis, through regulating gastric gland formation and maintaining an epithelial progenitor cell niche (Nyeng et al., 2007). In a cohort of 961 gastric cancers from the United Kingdom, Korea and China, FGFR2 amplification was detected in 4.2–7.4% of cases and was associated with lymph node metastasis and poor overall survival (Su et al., 2014). FGF10 amplification has also been detected in 3% of gastric cancers (Ooi et al., 2015) and immunohistochemical analysis of 178 gastric adenocarcinoma samples revealed that FGF10 levels are correlated with poor prognosis (Sun et al., 2015). Interrogation of most recent cBioPortal data suggests this could be an underestimate, with FGF10 amplifications reported in 5.7% of stomach adenocarcinoma cases (Cerami et al., 2012; Gao et al., 2013).
FGF10 in Skin Cancer
Whilst local elevation of FGF22, FGF7, and FGF10 are required for efficient healing of skin lesions (Braun et al., 2004), sustained elevation of FGF10 has also been implicated in cutaneous squamous cell carcinoma (SCC). Epidermal deletion of the tumor suppressor Pten produces a model of cutaneous squamous cell carcinoma in mice (Suzuki et al., 2003). The epidermis of these mice showed elevated keratinocyte expression of FGF10, with no change in levels of FGF7, FGF2, and FGF1. This increase in FGF10 was not accompanied by any Fgf10 transcriptional changes and was found to be dependent on the elevated mTORC1 signaling resulting from loss of the negative regulator, PTEN. The specific contribution of FGF10 to carcinogenesis was demonstrated by induction of constitutive epidermal FGF10 expression, which produced epidermal hyperplasia and spontaneous papillomas in all mice by 3 weeks of age. Crucially, it was shown that genetic ablation of Fgfr2 prevented hyperplasia in PTEN-deficient epidermis, suggesting that epidermal tumourigenesis induced by PTEN loss is mediated by an up-regulation of FGF10-FGFR2 autocrine signaling. Low levels of PTEN accompanied by elevated FGF10 have been observed in a panel of clinical SCC samples, highlighting the potential importance of this mechanism in the human disease (Hertzler-Schaefer et al., 2014).
In contrast to these data implicating FGF10 in skin carcinogenesis, previous work has suggested that FGF10-FGFR2b signaling may perform tumor-suppressive functions in the skin. Mice lacking epidermal Fgfr2b showed increased sensitivity to chemical carcinogens and 10% of animals surviving into adulthood developed spontaneous papillomas. Epidermal Fgfr2b ablation induced several changes in gene expression in the skin, including downregulation of Serpin a3b, a potential tumor suppressor (Grose et al., 2007).
FGF10 in Lung Cancer
Whilst FGF10 is crucial for branching morphogenesis in the developing lung (Sekine et al., 1999), induction of FGF10 over-expression in the respiratory epithelial cells of adult mice has been shown to cause multifocal pulmonary adenomas (Clark et al., 2001). A recent study designed to identify genomic variations in cell-free DNA in small cell lung cancer patients identified FGF10 amplification in 37.5% and of patients tested and FGFR1 amplification in 25% of cases (Du et al., 2018). Analysis of 1144 lung cancer tumors comprising lung adenocarcinomas and lung squamous cell carcinomas revealed FGF10 amplifications in 8.7% and FGFR1 amplifications in 9.3% of cases (Campbell J. D. et al., 2016).
The role of FGF10 in lung cancer initiation and progression remains poorly understood. However, emerging evidence suggests that FGF10 secreted from tumor-associated macrophages may play a role in promoting lung tumourigenesis (Figure 2). Tumour-associated macrophages (TAMs) are macrophages that have been co-opted by the tumor microenvironment to promote the growth and invasion of cancer cells (Noy and Pollard, 2014). Recent work showed that induction of FGF9 overexpression in the lungs of transgenic mice resulted in the development of adenocarcinoma-like nodules that are infiltrated with an immune response consisting mostly of macrophages. Expression analysis revealed that the TAMs from these transgenic mice expressed significantly higher levels of FGF2, FGF10, and FGFR2 than macrophages from wild-type mice. It has been suggested that activation of an FGF10-FGFR2 pathway may underlie the transition to FGF9-independent tumor growth, which has been observed in previous studies using this lung cancer model (Hegab et al., 2018). Expression profiling of TAMs in other pre-clinical cancer models will reveal the clinical significance of these early findings.
Therapeutic Approaches Targeting FGF10-FGFR2 Signaling
In light of mounting evidence supporting a role for aberrant FGF10-FGFR2b signaling in tumourigenesis, FGFR2b has become an attractive therapeutic target. Whilst a number of pan-FGFR inhibitors have entered the clinic (Clayton et al., 2017), the development FGFR2-specific inhibitors is hindered by the structural similarity of the kinase domains of FGFR1-3 (Belov and Mohammadi, 2013). For this reason, ATP-mimetic inhibitors of the FGFR2b isoform are currently unavailable. However, the development of monocloncal antibodies targeting FGFR2b may provide an opportunity to target this isoform specifically. Bemarituzumab (2018) (FPA144) is an anti-FGFR2b humanized monoclonal antibody currently in Phase I clinical trials as a monotherapy for FGFR2b-amplified gastric cancers. Bemarituzumab prevents the binding of FGF10, FGF7 and FGF22 to the FGFR2b and is reported to also promote antibody-dependent cell-mediated cytotoxicity through the recruitment of natural killer cells to the tumor (Bemarituzumab (FPA144) | Gastric Cancer | Five Prime Therapeutics).
FGF10 signal transduction requires recruitment of the myristoylated scaffold protein FRS2α to the activated receptor, and recent pre-clinical work has highlighted the potential of targeting FRS2α myristoylation in paracrine FGF10-induced tumourigenesis. Primary mouse prostate cells transduced with FRS2α(wt) or FRS2α(G2A), a mutant that cannot be myristolyated, were mixed with mouse urogenital sinus mesenchyme (UGSM) cells expressing either FGF10 or GFP as a control. The cells were implanted sub-renally into SCID mice. Xenografts derived from FRS2α(wt) prostate cells mixed with FGF10-UGSM showed adenocarcinoma, whilst xenografts of FRS2α(G2A) cells with FGF10-UGSM cells showed normal prostate tubules. In vitro work showed that FRS2α myristolylation can be targeted pharmacologically by treating with the myristoyl-coA analog B13 (Li et al., 2018a).
Recent evidence has demonstrated that a FGF10/FGFR/Src signaling axis may contribute to prostate tumourigenesis via a mechanism that is dependent on Src activity. Inhibition of Src myristoylation, and therefore membrane localization, has been suggested as a viable therapeutic strategy for these prostate cancer subtypes. In vitro studies showed that loss of Src myristoylaton had a significant inhibitory effect on FGF10-induced oncogenic signaling in comparison with a kinase-dead Src mutant (Li et al., 2018b). These data have prompted efforts to develop an N-myristoyltransferase inhibitor as a means to therapeutically target the FGF10/FGFR/Src signaling axis in cancer (French et al., 2004; Thinon et al., 2014; Kim et al., 2017).
Conclusion and Future Perspectives
The receptors for FGF10, FGFR2, and FGFR1 have been implicated in several human cancers, including pancreatic ductal adenocarcinoma and gastric cancer. However, since FGFR2 and FGFR1 are activated by a number of FGF family members, it has been difficult to attribute the tumor-promoting effects of these receptors to binding of a specific ligand. Recent data have begun to shed light on the role of FGF10 in these cancers, demonstrating that paracrine FGF10 synergizes with FGFR1/2 over-expression to induce epithelial-mesenchymal transition in a pre-clinical model of prostate cancer. The development of isoform-selective pharmacological tools will clarify the role of FGF10-FGFR2b/1b signaling in different cancer types and will allow the potential of FGF10 as a therapeutic target to be explored.
Author Contributions
Both authors listed have made a substantial, direct and intellectual contribution to the work, and approved it for publication.
Funding
This work was supported by a Cancer Research UK Centre Grant to Barts Cancer Institute (C16420/A18066).
Conflict of Interest Statement
The authors declare that the research was conducted in the absence of any commercial or financial relationships that could be construed as a potential conflict of interest.
References
Abate-Shen, C., and Shen, M. M. (2007). FGF signaling in prostate tumorigenesis—new insights into epithelial-stromal interactions. Cancer Cell 12, 495–497. doi: 10.1016/j.ccr.2007.11.021
Aleksic, T., Chitnis, M. M., Perestenko, O. V., Gao, S., Thomas, P. H., Turner, G. D., et al. (2010). Type 1 insulin-like growth factor receptor translocates to the nucleus of human tumor cells. Cancer Res. 70, 6412–6419. doi: 10.1158/0008-5472.CAN-10-0052
Bailey, P., Chang, D. K., Nones, K., Johns, A. L., Patch, A.-M., Gingras, M.-C., et al. (2016). Genomic analyses identify molecular subtypes of pancreatic cancer. Nature 531, 47–52. doi: 10.1038/nature16965
Belov, A. A., and Mohammadi, M. (2013). Molecular mechanisms of fibroblast growth factor signaling in physiology and pathology. Cold Spring Harb. Perspect. Biol. 5:a015958. doi: 10.1101/cshperspect.a015958
Bemarituzumab (2018). (FPA144) | Gastric Cancer | Five Prime Therapeutics. Available at: http://www.fiveprime.com/pipeline/fpa144 [accessed May 23, 2018]
Bhushan, A., Itoh, N., Kato, S., Thiery, J. P., Czernichow, P., Bellusci, S., et al. (2001). Fgf10 is essential for maintaining the proliferative capacity of epithelial progenitor cells during early pancreatic organogenesis. Development 128,5109–5117.
Braun, S., auf dem Keller, U., Steiling, H., and Werner, S. (2004). Fibroblast growth factors in epithelial repair and cytoprotection. Philos. Trans. R. Soc. Lond. B Biol. Sci. 359, 753–757. doi: 10.1098/rstb.2004.1464
Cai, H., Smith, D. A., Memarzadeh, S., Lowell, C. A., Cooper, J. A., and Witte, O. N. (2011). Differential transformation capacity of Src family kinases during the initiation of prostate cancer. Proc. Natl. Acad. Sci. U.S.A. 108, 6579–6584. doi: 10.1073/pnas.1103904108
Campbell, J. D., Alexandrov, A., Kim, J., Wala, J., Berger, A. H., Pedamallu, C. S., et al. (2016). Distinct patterns of somatic genome alterations in lung adenocarcinomas and squamous cell carcinomas. Nat. Genet. 48, 607–616. doi: 10.1038/ng.3564
Campbell, T. M., Castro, M. A. A., de Santiago, I., Fletcher, M. N. C., Halim, S., Prathalingam, R., et al. (2016). FGFR2 risk SNPs confer breast cancer risk by augmenting oestrogen responsiveness. Carcinogenesis 37, 741–750. doi: 10.1093/carcin/bgw065
Campbell, T. M., Castro, M. A. A., de Oliveira, K. G., Ponder, B. A. J., and Meyer, K. B. (2018). ERα binding by transcription factors NFIB and YBX1 enables FGFR2 signaling to modulate estrogen responsiveness in breast cancer. Cancer Res. 78, 410–421. doi: 10.1158/0008-5472.CAN-17-1153
Castro, M. A. A., de Santiago, I., Campbell, T. M., Vaughn, C., Hickey, T. E., Ross, E., et al. (2016). Regulators of genetic risk of breast cancer identified by integrative network analysis. Nat. Genet. 48, 12–21. doi: 10.1038/ng.3458
Cerami, E., Gao, J., Dogrusoz, U., Gross, B. E., Sumer, S. O., Aksoy, B. A., et al. (2012). The cBio cancer genomics portal: an open platform for exploring multidimensional cancer genomics data. Cancer Discov. 2, 401–404. doi: 10.1158/2159-8290.CD-12-0095
Chen, M.-K., and Hung, M.-C. (2015). Proteolytic cleavage, trafficking, and functions of nuclear receptor tyrosine kinases. FEBS J. 282, 3693–3721. doi: 10.1111/febs.13342
Chioni, A.-M., and Grose, R. (2012). FGFR1 cleavage and nuclear translocation regulates breast cancer cell behavior. J. Cell Biol. 197, 801–817. doi: 10.1083/jcb.201108077
Clark, J. C., Tichelaar, J. W., Wert, S. E., Itoh, N., Perl, A.-K. T., Stahlman, M. T., et al. (2001). FGF-10 disrupts lung morphogenesis and causes pulmonary adenomas in vivo. Am. J. Physiol. Cell. Mol. Physiol. 280, L705–L715. doi: 10.1152/ajplung.2001.280.4.L705
Clayton, N. S., Wilson, A. S., Laurent, E. P., Grose, R. P., and Carter, E. P. (2017). Fibroblast growth factor-mediated crosstalk in cancer etiology and treatment. Dev. Dyn. 246, 493–501. doi: 10.1002/dvdy.24514
Coleman, S. J., Chioni, A.-M., Ghallab, M., Anderson, R. K., Lemoine, N. R., Kocher, H. M., et al. (2014). Nuclear translocation of FGFR1 and FGF2 in pancreatic stellate cells facilitates pancreatic cancer cell invasion. EMBO Mol. Med. 6, 467–481. doi: 10.1002/emmm.201302698
Du, M., Thompson, J., Fisher, H., Zhang, P., Huang, C.-C., and Wang, L. (2018). Genomic alterations of plasma cell-free DNAs in small cell lung cancer and their clinical relevance. Lung Cancer 120, 113–121. doi: 10.1016/j.lungcan.2018.04.008
Eiro, N., Fernandez-Gomez, J., Sacristán, R., Fernandez-Garcia, B., Lobo, B., Gonzalez-Suarez, J., et al. (2017). Stromal factors involved in human prostate cancer development, progression and castration resistance. J. Cancer Res. Clin. Oncol. 143, 351–359. doi: 10.1007/s00432-016-2284-3
Elbauomy Elsheikh, S., Green, A. R., Lambros, M. B., Turner, N. C., Grainge, M. J., Powe, D., et al. (2007). FGFR1 amplification in breast carcinomas: a chromogenic in situhybridisation analysis. Breast Cancer Res. 9:R23. doi: 10.1186/bcr1665
Fachal, L., and Dunning, A. M. (2015). From candidate gene studies to GWAS and post-GWAS analyses in breast cancer. Curr. Opin. Genet. Dev. 30, 32–41. doi: 10.1016/j.gde.2015.01.004
Feng, S., Wang, F., Matsubara, A., Kan, M., and McKeehan, W. L. (1997). Fibroblast growth factor receptor 2 limits and receptor 1 accelerates tumorigenicity of prostate epithelial cells. Cancer Res. 57, 5369–5378.
Francavilla, C., Rigbolt, K. T. G., Emdal, K. B., Carraro, G., Vernet, E., Bekker-Jensen, D. B., et al. (2013). Functional proteomics defines the molecular switch underlying FGF receptor trafficking and cellular outputs. Mol. Cell 51, 707–722. doi: 10.1016/j.molcel.2013.08.002
French, K. J., Zhuang, Y., Schrecengost, R. S., Copper, J. E., Xia, Z., and Smith, C. D. (2004). Cyclohexyl-octahydro-pyrrolo[1,2-a]pyrazine-based inhibitors of human N-myristoyltransferase-1. J. Pharmacol. Exp. Ther. 309, 340–347. doi: 10.1124/jpet.103.061572
Gao, J., Aksoy, B. A., Dogrusoz, U., Dresdner, G., Gross, B., Sumer, S. O., et al. (2013). Integrative analysis of complex cancer genomics and clinical profiles using the cBioportal. Sci. Signal. 6:pl1. doi: 10.1126/scisignal.2004088
Grigoriadis, A., Mackay, A., Reis-Filho, J. S., Steele, D., Iseli, C., Stevenson, B. J., et al. (2006). Establishment of the epithelial-specific transcriptome of normal and malignant human breast cells based on MPSS and array expression data. Breast Cancer Res. 8:R56. doi: 10.1186/bcr1604
Grose, R., Fantl, V., Werner, S., Chioni, A.-M., Jarosz, M., Rudling, R., et al. (2007). The role of fibroblast growth factor receptor 2b in skin homeostasis and cancer development. EMBO J. 26, 1268–1278. doi: 10.1038/sj.emboj.7601583
Hadžisejdić, I., Mustać, E., Jonjić, N., Petković, M., and Grahovac, B. (2010). Nuclear EGFR in ductal invasive breast cancer: correlation with cyclin-D1 and prognosis. Mod. Pathol. 23, 392–403. doi: 10.1038/modpathol.2009.166
Hajihosseini, M. K., De Langhe, S., Lana-Elola, E., Morrison, H., Sparshott, N., Kelly, R., et al. (2008). Localization and fate of Fgf10-expressing cells in the adult mouse brain implicate Fgf10 in control of neurogenesis. Mol. Cell. Neurosci. 37, 857–868. doi: 10.1016/j.mcn.2008.01.008
Hegab, A. E., Ozaki, M., Kagawa, S., Hamamoto, J., Yasuda, H., Naoki, K., et al. (2018). Tumor associated macrophages support the growth of FGF9-induced lung adenocarcinoma by multiple mechanisms. Lung Cancer 119, 25–35. doi: 10.1016/j.lungcan.2018.02.015
Hertzler-Schaefer, K., Mathew, G., Somani, A.-K., Tholpady, S., Kadakia, M. P., Chen, Y., et al. (2014). Pten loss induces autocrine FGF signaling to promote skin tumorigenesis. Cell Rep. 6, 818–826. doi: 10.1016/j.celrep.2014.01.045
Ishiwata, T., Friess, H., Büchler, M. W., Lopez, M. E., and Korc, M. (1998). Characterization of keratinocyte growth factor and receptor expression in human pancreatic cancer. Am. J. Pathol. 153, 213–222. doi: 10.1016/S0002-9440(10)65562-9
Kim, S., Alsaidan, O. A., Goodwin, O., Li, Q., Sulejmani, E., Han, Z., et al. (2017). Blocking myristoylation of Src inhibits its kinase activity and suppresses prostate cancer progression. Cancer Res. 77, 6950–6962. doi: 10.1158/0008-5472.CAN-17-0981
Li, Q., Alsaidan, O. A., Ma, Y., Kim, S., Liu, J., Albers, T., et al. (2018a). Pharmacologically targeting the myristoylation of the scaffold protein FRS2α inhibits FGF/FGFR-mediated oncogenic signaling and tumor progression. J. Biol. Chem. 293, 6434–6448. doi: 10.1074/jbc.RA117.000940
Li, Q., Ingram, L., Kim, S., Beharry, Z., Cooper, J. A., and Cai, H. (2018b). Paracrine fibroblast growth factor initiates oncogenic synergy with epithelial FGFR/Src transformation in prostate tumor progression. Neoplasia 20, 233–243. doi: 10.1016/j.neo.2018.01.006
Mailleux, A. A., Spencer-Dene, B., Dillon, C., Ndiaye, D., Savona-Baron, C., Itoh, N., et al. (2002). Role of FGF10/FGFR2b signaling during mammary gland development in the mouse embryo. Development 129, 53–60.
Memarzadeh, S., Cai, H., Janzen, D. M., Xin, L., Lukacs, R., Riedinger, M., et al. (2011). Role of autonomous androgen receptor signaling in prostate cancer initiation is dichotomous and depends on the oncogenic signal. Proc. Natl. Acad. Sci. U.S.A. 108, 7962–7967. doi: 10.1073/pnas.1105243108
Memarzadeh, S., Xin, L., Mulholland, D. J., Mansukhani, A., Wu, H., Teitell, M. A., et al. (2007). Enhanced paracrine FGF10 expression promotes formation of multifocal prostate adenocarcinoma and an increase in epithelial androgen receptor. Cancer Cell 12, 572–585. doi: 10.1016/j.ccr.2007.11.002
Meyer, K. B., Maia, A.-T., O’Reilly, M., Teschendorff, A. E., Chin, S.-F., Caldas, C., et al. (2008). Allele-specific up-regulation of FGFR2 increases susceptibility to breast cancer. PLoS Biol. 6:e108. doi: 10.1371/journal.pbio.0060108
Mikolajczak, M., Goodman, T., and Hajihosseini, M. K. (2016). Interrogation of a lacrimo-auriculo-dento-digital syndrome protein reveals novel modes of fibroblast growth factor 10 (FGF10) function. Biochem. J. 473, 4593–4607. doi: 10.1042/BCJ20160441
Nomura, S., Yoshitomi, H., Takano, S., Shida, T., Kobayashi, S., Ohtsuka, M., et al. (2008). FGF10/FGFR2 signal induces cell migration and invasion in pancreatic cancer. Br. J. Cancer 99, 305–313. doi: 10.1038/sj.bjc.6604473
Noy, R., and Pollard, J. W. (2014). Review tumor-associated macrophages: from mechanisms to therapy. Immunity 41, 49–61. doi: 10.1016/j.immuni.2014.06.010
Nyeng, P., Norgaard, G. A., Kobberup, S., and Jensen, J. (2007). FGF10 signaling controls stomach morphogenesis. Dev. Biol. 303, 295–310. doi: 10.1016/j.ydbio.2006.11.017
Ooi, A., Oyama, T., Nakamura, R., Tajiri, R., Ikeda, H., Fushida, S., et al. (2015). Semi-comprehensive analysis of gene amplification in gastric cancers using multiplex ligation-dependent probe amplification and fluorescence in situ hybridization. Mod. Pathol. 28, 861–871. doi: 10.1038/modpathol.2015.33
Ornitz, D. M., and Itoh, N. (2015). The fibroblast growth factor signaling pathway. WIREs Dev. Biol. 4, 215–266. doi: 10.1002/wdev.176
Pereira, B., Chin, S.-F., Rueda, O. M., Vollan, H.-K. M., Provenzano, E., Bardwell, H. A., et al. (2016). The somatic mutation profiles of 2,433 breast cancers refines their genomic and transcriptomic landscapes. Nat. Commun. 7:11479. doi: 10.1038/ncomms11479
Reis-Filho, J. S., Simpson, P. T., Turner, N. C., Lambros, M. B., Jones, C., Mackay, A., et al. (2006). FGFR1 emerges as a potential therapeutic target for lobular breast carcinomas. Clin. Cancer Res. 12, 6652–6662. doi: 10.1158/1078-0432.CCR-06-1164
Ropiquet, F., Giri, D., Kwabi-Addo, B., Schmidt, K., and Ittmann, M. (2000). FGF-10 is expressed at low levels in the human prostate. Prostate 44, 334–338. doi: 10.1002/1097-0045(20000901)44:4<334::AID-PROS11>3.0.CO;2-G
Sekine, K., Ohuchi, H., Fujiwara, M., Yamasaki, M., Yoshizawa, T., Sato, T., et al. (1999). Fgf10 is essential for limb and lung formation. Nat. Genet. 21, 138–141. doi: 10.1038/5096
Shoji, K., Teishima, J., Hayashi, T., Ohara, S., Mckeehan, M. L., and Matsubara, A. (2014). Restoration of fibroblast growth factor receptor 2IIIb enhances the chemosensitivity of human prostate cancer cells. Oncol. Rep. 32, 65–70. doi: 10.3892/or.2014.3200
Stacey, S. N., Manolescu, A., Sulem, P., Thorlacius, S., Gudjonsson, S. A., Jonsson, G. F., et al. (2008). Common variants on chromosome 5p12 confer susceptibility to estrogen receptor–positive breast cancer. Nat. Genet. 40, 703–706. doi: 10.1038/ng.131
Su, X., Zhan, P., Gavine, P. R., Morgan, S., Womack, C., Ni, X., et al. (2014). FGFR2 amplification has prognostic significance in gastric cancer: results from a large international multicentre study. Br. J. Cancer 110, 967–975. doi: 10.1038/bjc.2013.802
Sun, Q., Lin, P., Zhang, J., Li, X., Yang, L., Huang, J., et al. (2015). Expression of fibroblast growth factor 10 is correlated with poor prognosis in gastric adenocarcinoma. Tohoku J. Exp. Med. 236, 311–318. doi: 10.1620/tjem.236.311
Suzuki, A., Itami, S., Ohishi, M., Hamada, K., Inoue, T., Komazawa, N., et al. (2003). Keratinocyte-specific Pten deficiency results in epidermal hyperplasia, accelerated hair follicle morphogenesis and tumor formation. Cancer Res. 63, 674–681.
Theodorou, V., Boer, M., Weigelt, B., Jonkers, J., van der Valk, M., and Hilkens, J. (2004). Fgf10 is an oncogene activated by MMTV insertional mutagenesis in mouse mammary tumors and overexpressed in a subset of human breast carcinomas. Oncogene 23, 6047–6055. doi: 10.1038/sj.onc.1207816
Thinon, E., Serwa, R. A., Broncel, M., Brannigan, J. A., Brassat, U., Wright, M. H., et al. (2014). Global profiling of co- and post-translationally N-myristoylated proteomes in human cells. Nat. Commun. 5:4919. doi: 10.1038/ncomms5919
Torres, C., Perales, S., Alejandre, M. J., Iglesias, J., Palomino, R. J., Martin, M., et al. (2014). Serum cytokine profile in patients with pancreatic cancer. Pancreas 43, 1042–1049. doi: 10.1097/MPA.0000000000000155
Traynor, A. M., Weigel, T. L., Oettel, K. R., Yang, D. T., Zhang, C., Kim, K., et al. (2013). Nuclear EGFR protein expression predicts poor survival in early stage non-small cell lung cancer. Lung Cancer 81, 138–141. doi: 10.1016/j.lungcan.2013.03.020
Veltmaat, J. M., Relaix, F., Le, L. T., Kratochwil, K., Sala, F. G., van Veelen, W., et al. (2006). Gli3-mediated somitic Fgf10 expression gradients are required for the induction and patterning of mammary epithelium along theembryonic axes. Development 133, 2325–2335. doi: 10.1242/dev.02394
Volckaert, T., Dill, E., Campbell, A., Tiozzo, C., Majka, S., Bellusci, S., et al. (2011). Parabronchial smooth muscle constitutes an airway epithelial stem cell niche in the mouse lung after injury. J. Clin. Invest. 121, 4409–4419. doi: 10.1172/JCI58097
Werner, S., Smola, H., Liao, X., Longaker, M. T., Krieg, T., Hofschneider, P. H., et al. (1994). The function of KGF in morphogenesis of epithelium and reepithelialization of wounds. Science 266, 819–822. doi: 10.1126/science.7973639
Yu, S., Xia, S., Yang, D., Wang, K., Yeh, S., Gao, Z., et al. (2013). Androgen receptor in human prostate cancer-associated fibroblasts promotes prostate cancer epithelial cell growth and invasion. Med. Oncol. 30:674. doi: 10.1007/s12032-013-0674-9
Keywords: FGF10, FGFR2, FGFR2b, FGFR1, FGFR1b, cancer
Citation: Clayton NS and Grose RP (2018) Emerging Roles of Fibroblast Growth Factor 10 in Cancer. Front. Genet. 9:499. doi: 10.3389/fgene.2018.00499
Received: 22 August 2018; Accepted: 05 October 2018;
Published: 24 October 2018.
Edited by:
Mohammad K. Hajihosseini, University of East Anglia, United KingdomReviewed by:
Agamemnon E. Grigoriadis, King’s College London, United KingdomMichael Grusch, Medizinische Universität Wien, Austria
Fen Wang, Texas A&M University, United States
Copyright © 2018 Clayton and Grose. This is an open-access article distributed under the terms of the Creative Commons Attribution License (CC BY). The use, distribution or reproduction in other forums is permitted, provided the original author(s) and the copyright owner(s) are credited and that the original publication in this journal is cited, in accordance with accepted academic practice. No use, distribution or reproduction is permitted which does not comply with these terms.
*Correspondence: Richard P. Grose, ci5wLmdyb3NlQHFtdWwuYWMudWs=