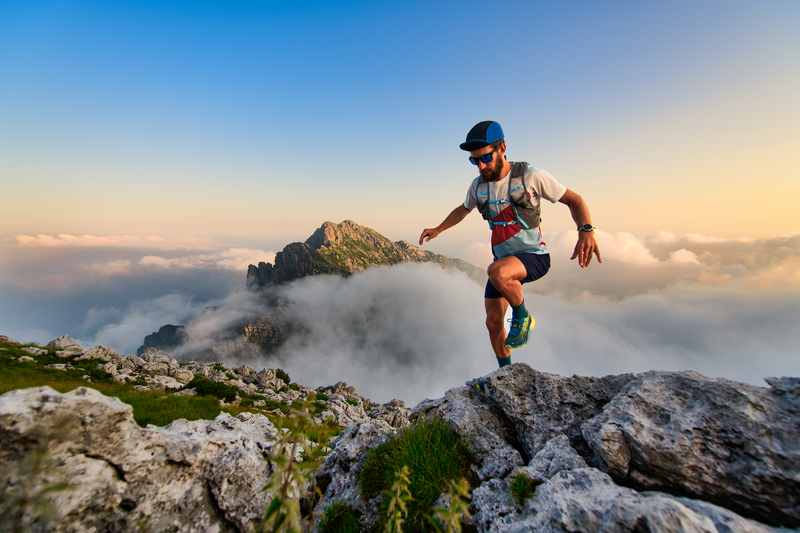
95% of researchers rate our articles as excellent or good
Learn more about the work of our research integrity team to safeguard the quality of each article we publish.
Find out more
REVIEW article
Front. Genet. , 26 September 2017
Sec. Genetics of Aging
Volume 8 - 2017 | https://doi.org/10.3389/fgene.2017.00138
This article is part of the Research Topic Proceedings of the International Conference Biomedical Innovations for Healthy Longevity View all 14 articles
Aging is characterized by functional decline of diverse organs and an increased risk for several diseases. Therefore, a high interest exists in understanding the molecular mechanisms that stimulate aging at all levels, from cells and tissues to organs and organisms, in order to develop ways to promote healthy aging. While many molecular and biochemical mechanisms are already understood in some detail, the role of changes in epigenetic regulation has only begun to be considered in recent years. The age-dependent global reduction in heterochromatin, along with site-specific changes in the patterns of DNA methylation and modification of histones, have been observed in several aging model systems. However, understanding of the precise role of such changes requires further research. In this review, we will discuss the role of epigenetic regulation in aging and indicate future research directions that will help elucidate the mechanistic details of it.
Aging is an associated with an increased risk of several morbidities such cancer, cardiovascular disease, and autoimmune disease. It is associated with the body's altered capacity to cope with stress induced by metabolism, infection, and damage to cellular macromolecules. Understanding the molecular mechanisms that advance aging will help scientists to understand why aging individuals are more prone to those diseases and why they may be less stress-resistant.
The cells in the aging body exhibit large differences to cells in the juvenile organism, ranging from changed gene expression and DNA methylation patterns (Heyn et al., 2012) to shortened telomeres (Allsopp et al., 1992) and deteriorating genome maintenance mechanisms (Gorbunova et al., 2007). At the tissue, organ, and systemic levels, cells are exposed to altered stromal milieus caused by altered secretory profiles of senescent cells (Rodier et al., 2009) and a deteriorating immune system that is not able to mount immune responses toward new antigens equal to that of a younger immune system (MacKall et al., 1996). The combination of those factors may facilitate a malignant transformation of cells and prevent the efficient recognition and clearance of transformed cells; thus, the cancer incidence is increased.
In recent years, epigenetic mechanisms have been increasingly recognized for playing important roles in health and disease. In addition, age-dependent changes in epigenetic regulation have been observed indicating that epigenetic regulation may also play an important role in aging. The following paragraphs will examine the role of epigenetic regulation in aging and conclude by highlighting some of the open questions in the field.
Aging is characterized by the functional decline of the organism and an increase in a chance of dying at any time. In order to determine molecular mechanisms that underlie aging, the field of aging research has pursued two major directions: the study of lifespan modifications, which allows us to determine effects of gene mutations on median and maximum lifespan of an organism, and the study of functional senescence, which investigates the age-related intrinsic functional decline in the functional status of cells, organs, and organ systems (Grotewiel et al., 2005). Both types of approaches have identified several mechanisms that affect aging, including telomere shortening, nutrient sensing and signaling (caloric restriction, and mutations in related signaling pathways, such as IGF1 and insulin signaling, TOR signaling that leads to increased lifespans in several model organisms (Table S1), mitochondrial dysfunction and oxidative stress (which by causing damage to macromolecules of the cell may promote aging; on the other hand, mild mitochondrial dysfunction has also been linked to lifespan extension in lower organisms through an adaptive response termed mitohormesis), deterioration of DNA repair and accumulation of DNA damage, changes in protein homeostasis leading to the accumulation and aggregation of misfolded proteins, and changes in epigenetic regulation, which will be the topic of this review (Tables S1, S2 and references within).
The exact mechanism that causes organismal aging is not yet understood. A model has been proposed suggesting that the process of aging is not a clonal phenomenon, but rather the result of increased cell heterogeneity in a tissue (Vijg and Dolle, 2002). Thus, it is possible that the functional decline of tissue is caused by various changes to the cells it is composed of and, as a result, to the functional decline of the organism. Given the high specialization of all the organs that make up an organism, it is likely that the mechanisms that contribute to the functional decline of certain tissue or cell types also differ. Cells of the intestinal epithelium, which are constantly exposed to stress, are likely more susceptible to senescence caused by activated DNA damage signaling (Wang et al., 2009), whereas T-cells that need to clonally expand in order to perform their immune function are likely more prone to senescence due to telomere shortening (Bodnar et al., 1996).
Clearly, more research is needed to get a systemic understanding of the underlying mechanisms that cause aging. Understanding the processes that drive aging at a cell and organ level is a first step and has already given researchers insights into the commonalities and differences in the aging process of different organs. In order to potentially increase the functional lifespan of humans, it will be necessary to gain a systemic understanding of the aging process, including the identification of the initial molecular changes entailed in aging.
Below, we will summarize knowledge on the molecular mechanisms that underlie cellular senescence and promote aging at the cellular level. We will also examine mechanisms that underlie immunosenescence as an example for an aging system.
Human diploid cells have a limited lifespan after which they terminally exit the cell cycle (Hayflick, 1965). This is termed cellular senescence. Cellular senescence is thought to play a role in aging, but this idea is still controversial. Several studies have shown that senescent cells accumulate in aging tissues in vivo (Herbig et al., 2006; Farr et al., 2016; Biran et al., 2017). Fibroblasts from progeria patients exhibit shortened replicative lifespans (Huang et al., 2008), suggesting that cellular senescence is linked with organismal aging. At the same time, the in vitro replicative lifespan of fibroblasts weakly correlates with donor age and the lifespan of the donor species (Smith et al., 2002; Ng et al., 2009). Assessment of 669 fibroblast cell cultures deriving from donors with different ages showed significant correlation between donor age and cell proliferative potential in females but not in males (Smith et al., 2002). The results of another study showed that telomerase activity and in vitro fibroblast cell growth were independent of donor age (Ng et al., 2009). Similarly, a study by Cristofalo et al. (1998) did not find any correlation between replicative lifespan of skin fibroblasts and donor age (Cristofalo et al., 1998).
Senescence may play a different role in different cell types during aging. Senescence typically occurs in the differentiated cells in most organs but can also be initiated in the stem cells influencing the capacity of tissues to renew and repair (Krishnamurthy et al., 2006). It is still unclear, however, whether the decrease in the functionality of stem cells that is observed with aging is the result of aging of stem cells or rather the result of changes in the niche, an environment that surrounds the cells in the aging tissue (Rando, 2006). The former was recently support by the observation that adult quiescent muscle stem cells undergo irreversible senescence in muscle tissues of old mice (Sousa-Victor et al., 2014). Thus, while senescent cells accumulate in tissues of aging individuals, the involvement of senescence in aging seems to be dependent on the affected cell types and tissues.
While senescence may promote aging and age-related pathologies, altered expression of senescence-associated genes may be sufficient to trigger carcinogenesis of human cells (Hahn et al., 1999). Hence, understanding of molecular mechanisms that underlie senescence is helpful in two ways: it will allow scientists to attempt to prolong the healthy lifespan, and potentially to develop new therapy of cancer.
To date, several mechanisms that promote senescence have been identified, which mainly involve accumulation of damage to DNA and other macromolecules of the cell (Lundblad and Szostak, 1989; Harley et al., 1990; Sohal and Weindruch, 1996; Vaziri and Benchimol, 1996; Sedelnikova et al., 2004; Seluanov et al., 2004; Sfeir and de Lange, 2012).
Furthermore, chromatin structure plays a critical role in regulation of cell and organism life span (Sedivy et al., 2008; Feser and Tyler, 2011; O'Sullivan and Karlseder, 2012). Decrease in the expression of genes encoding histones and the decrease in the number of repressive heterochromatin marks, such as methylation of DNA, and methylation of histones, including H3K9me3, H3K27me3, and H4K20me3 marks, observed in senescent cells and older organisms may suggest that aging at least in part is associated with the reduction of heterochromatin, which will be discussed in the sections that follow.
Immunosenescence is the age-dependent deterioration of the immune system; it is linked with higher morbidity and mortality in the elderly population (Ferguson et al., 1995), as the immune system protects animals against pathogens and eliminates abnormally proliferating cells. In order to avoid autoimmune responses, maturing T-cells are subject to positive selection (T-cell are activated by antigen survive) and negative selection (autoreactive T-cells are reduced in numbers) in the thymus. These selection processes are not very efficient but energy-consuming, typically resulting in the elimination of over 95% of newly generated T-cells (Surh and Sprent, 1994). Therefore, when peripheral lymphatic organs are supplied with sufficient population of T-cells, the thymus shrinks, decreasing in size by about ~3% a year until fifth decades when it starts shrinking by ~1% per year (Steinmann et al., 1985). This phenomenon, often referred to as thymic involution, occurs similarly in all species that have thymus. Thymic involution also involves changes in the thymic cellularity and architecture leading to a decrease of production of naïve T-cells per the size of the thymus (Cunningham et al., 2001).
This reduced thymic T-cell output is paralleled by repeated clonal selection and expansion of T-cells in the periphery of an organism, resulting in an increased specialization and a decreased proliferative potential of the peripheral T-cell population. Both of these changes contribute to immunosenescence (Ferguson et al., 1995). Thus, immunosenescence differs from cellular senescence in that it involves the deterioration of a system rather than simply the terminal growth arrest of a single cell. However, cellular senescence may contribute mechanistically to immunosenescence, including thymic involution as outlined in the following paragraph.
The epithelial compartment of the thymus itself seems to play a crucial role in promoting thymic involution (Ortman et al., 2002; Zhu et al., 2007; Aw et al., 2009). During thymic involution, the epithelial compartment accumulates senescent and apoptotic cells (Aw et al., 2008) resulting in a shrinkage of the epithelial compartment (Flores et al., 1999). Consequently, cellular senescence and cell death may limit the functionality and the regeneration of the aging thymus and promote its age-dependent functional decline. On the other hand, the peripheral T-cell pool that is subject to repeated clonal expansions is prone to telomere shortening (Weng et al., 1995; Rufer et al., 1999) and cellular senescence over time. In line with this, after clonal selection in vitro leads to accumulation of replicatively senescent T-cells. In addition, senescence-like T-cells also occur in vivo (Effros, 2004).
In addition to telomere shortening, changes in the expression of several transcription factors also affect thymic involution (Trebilcock and Ponnappan, 1996; Ortman et al., 2002). Moreover, alterations in epigenetic regulation have also been associated with immunosenescence. Kuwahara et al. (2014) revealed that Menin promotes histone acetylation at the Bach2 locus, thereby suppressing T-cell senescence and ultimately immunosenescence (Kuwahara et al., 2014). Additionally, our laboratory showed that immunosenescence in rats is paralleled by substantial changes in thymus and spleen transcriptome of old rats. Additionally, aging is associated with a loss of heterochromatin marks, including H3K9me3 with corresponding reduction in SUV39H1 expression and increased genomic instability in the thymus (Sidler et al., 2013). This may contribute to the induction of senescence and apoptosis in cells within the aging thymus. However, further research is necessary to identify the role of epigenetic regulation in immunosenescence.
Analysis of DNA methylation in the genomes from CD4+ T-cells from a newborn and a centenarian revealed a global loss of DNA methylation with age with differentially methylated sites identified in promoter (~10%), exonic (~10%), intronic (~45%), and intergenic (~35%) regions (Heyn et al., 2012). When Heyn et al. (2012) compared the global methylation levels in the centenarian and newborn genomes to the level in the genome of a 26-year-old, they found that the global DNA methylation level in the 26-year-old was intermediate. Thus, they proposed a model that asserts cells accumulate stepwise changes in DNA methylation throughout a lifespan.
Age-dependent decrease in global genome methylation has also been observed in other aging model organisms such as mice (Wilson et al., 1987) and rats (Vanyushin et al., 1973), although more recent studies do not always support these early findings. For example Maegawa et al. (2010) found that more cytosines became hypermethylated in older mice, as compared to those that became hypomethylated; analysis of methylation in 3,627 autosomal genes showed that 774 (21%) of them became hypermethylated, whereas only 466 (13%)—hypomethylated in 35-month vs. 3 month-old C57BL/6 mice (Maegawa et al., 2010). The inhibition of DNA methylation resulted in reduced replicative lifespan of cells (Holliday, 1986). However, the extent of global DNA methylation changes and the CpG sites affected may vary between different tissues. For example, DNA methylation levels in rat adipose tissues did not significantly change with age (Thompson et al., 2010).
While global DNA hypomethylation is observed in various tissues with age, the accumulation of domains of heterochromatin during the early steps leading to senescence has been described (Narita et al., 2003). These senescence-associated heterochromatin foci (SAHF) were originally shown to coincide with E2F target promoters. However, while pRB and p53 were demonstrated to be important for the establishment of SAHF, senescence in response to oncogene activation also involved the formation of SAHF (Ye et al., 2007). The observation that telomeres and subtelomeric regions became de-heterochromatinized in the absence of telomerase in aging mice (Benetti et al., 2007) led Zhang and Adams to propose that during aging, the heterochromatin marks get redistributed from areas of constitutive heterochromatin, such as telomeres and pericentromeres, to areas of facultative heterochromatin, such as SAHF (Zhang and Adams, 2007).
When analyzing the observed changes in DNA methylomes of human blood cells in more detail, Heyn et al. (2012) indicated DNA hypomethylation primarily occurred in CpG-poor promoters or tissue-specific promoters. It was also shown that hypomethylation is more frequent in the genomics regions enriched with polycomb proteins or permissive histone modifications (McClay et al., 2014). In contrast, DNA hypermethylation, was primarily found CpG-rich sequences. Interestingly, when we compared gene expression and DNA methylation patterns in WI38 cells throughout their lifespan, we observed a similar trend to DNA hypomethylation with some genomic sites also being hypermethylated, but also found that they rarely corresponded with differential gene expression (Sidler et al., 2014a).
These findings are further backed up by a mathematical modeling study performed on 82 available Illumina DNA methylation array data sets to test for the presence of age predictor sites in the genome (Horvath, 2013). Horvath has identified 353 differentially methylated CpG sites that predicted age very robustly, which has resulted in the proposition of an epigenetic clock model for aging. Among these 353 CpG sites 193 sites were commonly hypermethylated and 160 hypomethylated with increasing age. While the methylation status of the hypermethylated sites was quite robust across different tissues, the hypomethylated sites exhibited more tissue-specificity (Horvath, 2013). Also, while hypomethylated sites were more commonly found in CpG shores than hypermethylated sites, hypermethylated sites were over-represented near Polycomb-target genes. However, the overlap with gene expression changes associated with these age predictor sites was low, so it is not quite clear how the hypermethylation at these sites affects aging.
It was observed that DNA hypermethylation is more site-specific as compared to hypomethylation. Importance of DNA hypomethylation for triggering senescence is demonstrated by two facts. First, hypomethylation occurs in senescing cells but not in immortalized cells (Wilson and Jones, 1983; Degerman et al., 2014). Second, DNA methylation inhibition triggers growth arrest in immortal cells (Vogt et al., 1998).
As a result, the shift to hypomethylation of constitutive heterochromatin and hypermethylation of promoters in cell-cycle promoting genes, age-dependent changes in DNA methylation may trigger increased genomic instability and lead to a permanent cell-cycle arrest. Furthermore, the differential methylation of promoter CpGs may also contribute to the observed changes in senescence- and age-related gene expression profiles. The role of DNA hypomethylation and site-specific hypermethylation in aging and senescence would have to be clarified in the future research.
Post-translational modifications of the histone amino tails affect their affinity to DNA and interacting proteins, and different combinations of such modification marks may have synergistic or antagonistic effects on those affinities. This indicates a role of histone modifications in formation of active or repressive chromatin states and led Jenuwein and Allis to the formulation of the “histone code” theory (Jenuwein and Allis, 2001). Generally, acetylation and phosphorylation of histone tails are considered to be euchromatin marks and methylation of histones is enriched in heterochromatin regions (Jenuwein, 2001). However, some exceptions to this theory exist. For example, H4K20 acetylation is a repressive mark (Kaimori et al., 2016), whereas H3K4 and H3R17 methylation are activating marks. The effect of histone modification marks is mediated by bromodomain-containing proteins in the case of acetylation marks (Dhalluin et al., 1999) or by chromodomain-containing proteins in the case of methylation marks (Bannister et al., 2001).
In addition to histone tail modifications, the nucleosome density affects the level of DNA packaging as well. Therefore, DNA regions with a high density of nucleosomes are likely transcriptionally inactive while transcriptionally active DNA regions are characterized by looser packaging and a low density of nucleosomes (Boeger et al., 2003).
Both nucleosome occupancy and histone tail modifications experience changes as cells age (Table 1). A decline in nucleosome occupancy with increasing age was first demonstrated in human skin fibroblasts in which the linker regions between nucleosomes were shown to become increasingly heterogeneous in length (Ishimi et al., 1987). The global loss of histones has been hypothesized to be associated with a reduced deposition of histones, which is mediated by ASF1 in a replication-dependent and a replication-independent manner together with CAF1 and HIRA (Groth et al., 2007; Galvani et al., 2008), or by reduced expression, also regulated by ASF1 during replication (Sutton et al., 2001), or the maturation histone mRNA promoted by SLBP (Kaygun and Marzluff, 2005). The loss of function of ASF1 in yeast resulted in impaired heterochromatin formation and genomic instability (Tanae et al., 2012). The finding that ASF1 and SLBP expression decrease with age in human cells (O'Sullivan et al., 2010) and that over-expression of histones increases lifespan (Feser et al., 2010), may indicate that declining nucleosome occupancy in aging human cells similarly results in a loss of heterochromatin paired with increased genomic instability. This also likely results in changes to the gene expression profile. However, to better understand the potential role of nucleosome loss in aging, a more detailed understanding of DNA regions affected by a reduced nucleosome density and the functional consequences is needed.
Table 1. Changes in histone modification pattern and nucleosome occupancy with increasing age and senescence.
In addition to overall changes in nucleosome numbers, numerous changes in post-translational modifications of histone tails with age have been described (Table 1). However, their functional consequences are only beginning to be understood. H3K56ac promotes nucleosome assembly and transcriptional activation of histone gene expression (Kaplan et al., 2008; Williams et al., 2008). H3K9me3 is involved in heterochromatin formation in telomeric, subtelomeric, and pericentromeric regions in young cells (Benetti et al., 2007; Vaquero et al., 2007) and with the establishment of SAHF in senescing cells (Narita et al., 2003). Finally, H4K16ac is involved in telomere silencing (Kozak et al., 2010).
Aforementioned histone marks are established by various histone-modifying enzymes including histone deacetylases and acetyltransferases (Kuo and Allis, 1998), histone methyltransferases, and histone demethylases (Black et al., 2012). Thus, changes in the frequencies of different histone modifications may be associated with different expression or activity levels of histone-modifying enzymes. In line with this, the redistribution of SIRT1 from heterochromatin regions to sites of DNA damage resulted in gene expression changes resembling those in aging tissues (Oberdoerffer et al., 2008). The importance of SIRT1, a histone deacetylase, in the processes of normal cell proliferation and aging cannot be underestimated. SIRT1 deacetylates and inactivates the RelA/p65 subunit of transcription factor NF-κB known to be the main transcriptional regulator of several genes directly involved in inflammation processes associated with aging (Lawrence, 2009).
Moreover, SUV39H1 seems to play an important role in controlling the proliferative status of a cell, through alterations of the chromatin structure at centromere region during the process of cell division (Aagaard et al., 2000), the organization of the nuclear architecture (Uhlirova et al., 2010), the inhibition of cell differentiation in transgenic mice overexpressing SUV39H1 (Czvitkovich et al., 2001), and the reduction of viability along with increased genomic instability and susceptibility to cancer found in SUV39H1/2 knockout mice (Peters et al., 2001).
Furthermore, reduced SUV39H1 levels during cell senescence and aging (Sidler et al., 2013, 2014b) are associated with reduced H3K9 trimethylation in pericentric satellite regions. Since the relaxation of satellite heterochromatin has been shown to occur early during the establishment of senescence (Swanson et al., 2013), these observations may indicate that SUV39H1 plays an important role in promoting genome stability through its role in heterochromatin formation, thereby allowing for continued cell division.
Additionally, SUV39H1 is involved in regulating gene expression. For instance, SUV39H1 cooperates with E2F/RB to silence S-phase genes in terminally differentiating cells (Ait-Si-Ali et al., 2004), and contributes to transcriptional repression of the p21 promoter (Cherrier et al., 2009). During senescence, SUV39H1 is either enriched or reduced at specific promoters. Such specific recruitment of SUV39H1 by transcription factors may be essential for modulation of H3K9me3 patterns during aging (Ait-Si-Ali et al., 2004; Lomberk et al., 2012), and may in addition segregate SUV39H1 away from constitutive heterochromatin regions.
Studies of the effects of SUV39H1 mutations on lifespan are complicated as both the overexpression and the knockout lead to developmental defects (Czvitkovich et al., 2001; Peters et al., 2001). However, one study showed that mice deficient in Zmpste24 and Suv39h1 lived 11 weeks longer than mice deficient for Zmpste24 alone (Liu et al., 2013). However, the reason for the lifespan extension by Suv39h1 deficiency in this background may also be related to its role in promoting heterochromatin DNA repair (Zheng et al., 2014) rather than its role in cell proliferation, as Zmpste24 deficiency is known to lead to accumulation of damaged and unrepaired DNA (comparable to senescent cells) (Liu et al., 2005). It is quite likely that during the establishment of cellular senescence the downregulation of SUV39H1 may initially occur to promote DNA repair leading to genome destabilization due to deheterochromatinization of repetitive DNA.
To sum up, the downregulation of SUV39H1 during the establishment of senescence may promote DNA repair in heterochromatin regions but eventually results in increased genomic instability promoting cell-cycle arrest. On the other hand, the altered expression and recruitment of SUV39H1 to promoter regions of genes may also shape the senescence-associated gene expression profile and further contribute to the establishment of senescence. Further research is needed to clearly define the regulation and role of SUV39H1 during the establishment of senescence.
Both SUV39H1/2 knock-out mice and SUV39H1 transgenic mice showed severe developmental defects, while the knockout animals exhibited decreased viability, increased genomic instability, and susceptibility to tumor formation (Peters et al., 2001). The transgenic animals overexpressing SUV39H1 showed a deficiency in cell differentiation (Czvitkovich et al., 2001). Our results further support a role for the SUV39H1 downregulation in the induction of senescence (Sidler et al., 2014b). Being a histone methyltransferase, SUV39H1 expression is important for the maintenance of adequate levels of H3K9 trimethylation in order to silence pericentric repetitive DNA regions (Peters et al., 2001; Lehnertz et al., 2003) and to regulate transcriptional repression through increased H3K9me3 levels or transcriptional activation through the reduction in H3K9me3 levels.
Thus, it can be hypothesized that adequate SUV39H1 expression levels are required in order to balance genomic stability and proliferative potential. In such a model, increased SUV39H1 expression levels would protect the genome integrity and promote cell division, whereas decreased SUV39H1 expression levels would increase genomic instability and favor cell cycle arrest; however, if the SUV39H1 expression levels fall below a threshold level, the silencing of promoter regions of growth inhibitory genes may also be compromised and therefore result in tumor formation. In addition to mere differences in expression levels, the altered recruitment of SUV39H1 to specific DNA regions likely also contributes to senescence-associated changes in the distribution of H3K9me3 marks. In order to test this model for the role of SUV39H1 expression and recruitment in balancing genomic integrity and cell proliferation, it would be interesting to address the following questions.
While the role of SUV39H1 in the formation of the heterochromatin in pericentric regions is well-accepted, the patterns of SUV39H1-mediated histone H3K9 trimethylation in other DNA sequence regions are less characterized. A better understanding of which transcription factors interact with SUV39H1 and may therefore recruit it to their target promoters could help researchers to understand the effects of SUV39H1-mediated H3K9 trimethylation on gene expression. One such example is the association of SUV39H1 with RB/HDAC1 resulting in the heterochromatin formation and suppression of genes involved in cell cycle progression (Bandyopadhyay et al., 2007). Thus, a systematic analysis of the interactome of SUV39H1 and ChIP-seq determination of the target sequences of SUV39H1-mediated heterochromatin formation in dividing when compared to senescent cells will allow researchers to further dissect the role of SUV39H1 in senescence-associated changes in transcriptional regulation and the role of SUV39H1 recruitment in the establishment of senescence.
In addition to mediating the silencing of repetitive DNA regions, SUV39H1 also plays a significant role in the regulation of telomere length (Garcia-Cao et al., 2004), and telomere nuclear architecture (Uhlirova et al., 2010). Furthermore, heterochromatin regions enriched with repressive histone marks associate with the nuclear lamina (Guelen et al., 2008) and likely contribute to the domain organization of chromosomes, with regions more distant to the nuclear lamina being more frequently transcriptionally active (Peric-Hupkes et al., 2010). Peric-Hupkes et al. (2010) showed that this domain organization changed with increasing differentiation of mouse embryonic fibroblasts, resulting in differential gene expression. Further, a study by Zhang et al. (2015) showed that upon inducing WRN deficiency in mesenchymal stem cells, the cells exhibited various aging phenotypes, including a global loss of H3K9me3 and changes in heterochromatin architecture (PMID: 25931448). The others also showed that expression of catalytically inactive SUV39H1 in wild-type stem cells resulted in a similar phenotype, further supporting a role for SUV39H1 in preventing senescence. Hence, it would be interesting to test whether the downregulation of SUV39H1 during the establishment of senescence corresponds with alterations to the nuclear architecture and chromosome domain organization. If so, further testing could show whether this results in altered gene expression patterns that might contribute to the establishment of senescence.
In order to address how gradual changes in SUV39H1 expression levels affect the cell-cycle dynamics, human diploid fibroblasts could be treated with increasing amounts of SUV39H1 targeting siRNA or transfected with increasing amounts of an SUV39H1 overexpression construct followed by analysis of cell proliferation by BrdU incorporation and senescence by the SA-beta-GAL assay. This would allow for testing of the hypotheses that have been formulated above and might help in determining the threshold of the SUV39H1 expression level that is necessary for the inhibition of cell division if such a threshold indeed exists.
Another approach is to determine how the SUV39H1 expression levels correlate with the rate of proliferation in various cell types. For this, it would be interesting to compare various cancer cell lines, stem cells, and human diploid fibroblasts at different population doublings and primary cells derived from donors of different ages.
A more detailed understanding of how SUV39H1 expression is controlled on the level of transcription and post-transcriptionally will help in studying what intra- and extra-cellular stimuli may trigger the downregulation of SUV39H1. Two studies have described an indirect role of p53 in regulating the expression of SUV39H1 by inducing the transcription of p21, which in turn represses E2F mediated transcription of SUV39H1 (Mungamuri et al., 2012; Zheng et al., 2014). In addition, miRNA-mediated post-transcriptional regulation of SUV39H1 expression has also been described (Villeneuve et al., 2010). A systematic promoter analysis of the SUV39H1 promoter region, as well as a target screen for miRNA-mediated post-transcriptional regulation, may indicate additional regulatory mechanisms that may affect the SUV39H1 expression during cell differentiation and cell proliferation. In addition, our study of the treatment of cells with chaetocin resulted in the reduction of SUV39H1 transcript levels (Sidler et al., 2014b), which was surprising because chaetocin inhibits the enzymatic activity of SUV39H1. This may therefore indicate that SUV39H1 expression is also regulated by a positive feedback mechanism. Therefore, it would be important to find out whether this is the case and how it is mediated. One possibility for such a mechanism could be that SUV39H1 may methylate one of its own transcriptional repressors and thereby inactivate it.
Several studies have described altered DNA methylomes that were characterized by extensive site-specific DNA hypomethylation alongside of some site-specific hypermethylation with increasing age (Heyn et al., 2012; Johansson et al., 2013; McClay et al., 2014). We made similar observations in senescence when compared to actively dividing cell cultures of WI38 human lung fibroblasts (Sidler et al., 2014a). While our dataset showed that there were some clusters of DNA hypo- or hyper-methylation throughout the genome, differential methylation of promoter regions rarely corresponded with a change in gene expression. In addition, the global loss of DNA methylation and downregulation of DNMT1 correlates with the observation that the majority of differentially methylated CpG sites in senescent cells are hypomethylated. However, it will be interesting to further address the role of this altered DNA methylome in senescence and whether it is a consequence of other senescence-related molecular changes or otherwise plays a causal role in the establishment of senescence. Furthermore, it would be interesting to study how certain sites are targeted for hypo- or hyper-methylation.
The downregulation of DNMT1 during senescence could indicate that DNA methylation marks are passively lost through the deficiency in maintenance through cell divisions. However, 1,849 CpG sites were significantly differentially methylated when comparing three senescent to three dividing cell cultures in our study, pointing that the loss of DNA methylation during senescence may at least in part be non-random. Thus, it would be interesting to study whether the recruitment of DNMT1 to DNA is altered during senescence. This could be achieved through the immunoprecipitation of DNMT1 followed by mass-spectrometric analysis of its interacting proteins in order to determine whether DNMT1 is associated with different binding partners during senescence. Some studies suggest a role for the existing histone modification pattern in limiting the de novo DNA methylation in a sequence-specific way (Schlesinger et al., 2007; Straussman et al., 2009; Ludwig et al., 2014). Consequently, a better understanding of the regulation of the histone modification pattern during senescence may help in answering this question.
Changes in the DNA methylation patterns do not extensively correlate with altered gene expression, but the inhibition of DNA methylation was shown to trigger cell growth arrest (Vogt et al., 1998) suggesting that DNA hypomethylation is associated with the molecular changes that inhibit cell division. This could potentially be mediated through contributing to genomic instability through the loss of heterochromatin or to the altered nuclear architecture potentially by affecting the nuclear lamina interaction and chromosomal domain organization (Guelen et al., 2008) and could be studied with similar approaches described for SUV39H1 above.
In order to dissect the role of epigenetic changes in thymic involution further, it would be interesting to separate the different cell types that occur within the thymus, dendritic cells, epithelial cells, nurse cells, macrophages, and maturing T-cells. The measurement of DNMT1 and SUV39H1 protein levels and determination of global DNA methylation and H3K9 trimethylation in the different cell types could then indicate which cells are mainly affected by the loss of heterochromatin marks and corresponding increase in genomic instability.
Furthermore, our study involved only three time points across the lifespan of the rats—1month-old animals, 4 month-old animals around the age of the onset of the thymic involution, and 18-month-old animals, which have a very small thymus (Sidler et al., 2013). The analysis of additional time points around the onset of thymic involution may help in determining cell type and the stage of thymus development in which the downregulation of SUV39H1 and DNMT1 occurs. If it occurs relatively early, this may suggest that the associated heterochromatin loss, genomic instability, and alterations in the gene expression profile may play a causal role in the induction of senescence and/or apoptosis in the affected cell types. If it occurs relatively late, it may rather be a consequence of other age-related changes within the thymus, such as DNA damage-induced downregulation of SUV39H1 in order to facilitate the repair of DNA damage within heterochromatin regions.
If the downregulation of SUV39H1 and DNMT1 does occur relatively early during thymic involution, it would be interesting to further study their role in the onset of thymic involution by creating mouse-models that specifically overexpress or repress SUV39H1 or DNMT1 in the thymus or possibly even in a specific cell type that is affected by their age-dependent downregulation (Sidler et al., 2013). The phenotype of such mouse-models would give further indications on what the specific role of the SUV39H1- and DNMT1-downregulation is during thymic involution.
On the other hand, since the proliferation of peripheral T-cells depends on antigen recognition, which is limited in the rats we studied as they were housed in a pathogen-free environment (Sidler et al., 2013), it would be interesting to study epigenetic changes in T-cell populations that are challenged with pathogens. This could be achieved either in vivo, through the repeated infection with a virus, such as CMV, followed by collection of blood samples in order to study the T-cell population that was clonally selected in response to each cycle of infection, or in vitro, by exposing virus-specific T-cells to their cognate antigen for several subcultures and studying changes in epigenetic regulation.
Considerable progress has been made in understanding regulatory pathways that are involved in aging over the recent years. Several studies have described age-dependent reduction in global and constitutive heterochromatin affecting both DNA methylation and histone modifications as well as site-specific changes in DNA methylation and histone modification patterns. A more detailed understanding of the regulation of the modifying enzymes and their recruitment during the establishment of senescence will likely pinpoint several molecular targets that are involved in the regulation of aging and may even serve as possible drug targets to promote a prolonged, healthy lifespan.
CS: Wrote the manuscript; OK: Proposed the topic, revised the manuscript; IK: Proposed the topic, wrote the manuscript.
The authors declare that the research was conducted in the absence of any commercial or financial relationships that could be construed as a potential conflict of interest.
This work was supported by graduate studentships from Alberta Cancer Foundation and Alberta Innovates Health Solutions to CS and Grants from the DoE, NSERC, and CIHR to OK and Natural Sciences and Engineering Research Council of Canada grant to IK.
The Supplementary Material for this article can be found online at: http://journal.frontiersin.org/article/10.3389/fgene.2017.00138/full#supplementary-material
Aagaard, L., Schmid, M., Warburton, P., and Jenuwein, T. (2000). Mitotic phosphorylation of SUV39H1, a novel component of active centromeres, coincides with transient accumulation at mammalian centromeres. J. Cell Sci. 113(Pt. 5), 817–829.
Ait-Si-Ali, S., Guasconi, V., Fritsch, L., Yahi, H., Sekhri, R., Naguibneva, I., et al. (2004). A Suv39h-dependent mechanism for silencing S-phase genes in differentiating but not in cycling cells. EMBO J. 23, 605–615. doi: 10.1038/sj.emboj.7600074
Allsopp, R. C., Vaziri, H., Patterson, C., Goldstein, S., Younglai, E. V., Futcher, A. B., et al. (1992). Telomere length predicts replicative capacity of human fibroblasts. Proc. Natl. Acad. Sci. U.S.A. 89, 10114–10118. doi: 10.1073/pnas.89.21.10114
Aw, D., Silva, A. B., Maddick, M., von Zglinicki, T., and Palmer, D. B. (2008). Architectural changes in the thymus of aging mice. Aging Cell 7, 158–167. doi: 10.1111/j.1474-9726.2007.00365.x
Aw, D., Taylor-Brown, F., Cooper, K., and Palmer, D. B. (2009). Phenotypical and morphological changes in the thymic microenvironment from ageing mice. Biogerontology 10, 311–322. doi: 10.1007/s10522-008-9182-2
Bandyopadhyay, D., Curry, J. L., Lin, Q., Richards, H. W., Chen, D., Hornsby, P. J., et al. (2007). Dynamic assembly of chromatin complexes during cellular senescence: implications for the growth arrest of human melanocytic nevi. Aging Cell 6, 577–591. doi: 10.1111/j.1474-9726.2007.00308.x
Bannister, A. J., Zegerman, P., Partridge, J. F., Miska, E. A., Thomas, J. O., Allshire, R. C., et al. (2001). Selective recognition of methylated lysine 9 on histone H3 by the HP1 chromo domain. Nature 410, 120–124. doi: 10.1038/35065138
Benetti, R., Garcia-Cao, M., and Blasco, M. A. (2007). Telomere length regulates the epigenetic status of mammalian telomeres and subtelomeres. Nat. Genet. 39, 243–250. doi: 10.1038/ng1952
Biran, A., Zada, L., Abou Karam, P., Vadai, E., Roitman, L., Ovadya, Y., et al. (2017). Quantitative identification of senescent cells in aging and disease. Aging Cell 16, 661–671. doi: 10.1111/acel.12592
Black, J. C., Van Rechem, C., and Whetstine, J. R. (2012). Histone lysine methylation dynamics: establishment, regulation, and biological impact. Mol. Cell 48, 491–507. doi: 10.1016/j.molcel.2012.11.006
Bodnar, A. G., Kim, N. W., Effros, R. B., and Chiu, C. P. (1996). Mechanism of telomerase induction during T cell activation. Exp. Cell Res. 228, 58–64. doi: 10.1006/excr.1996.0299
Boeger, H., Griesenbeck, J., Strattan, J. S., and Kornberg, R. D. (2003). Nucleosomes unfold completely at a transcriptionally active promoter. Mol. Cell 11, 1587–1598. doi: 10.1016/S1097-2765(03)00231-4
Bracken, A. P., Kleine-Kohlbrecher, D., Dietrich, N., Pasini, D., Gargiulo, G., Beekman, C., et al. (2007). The Polycomb group proteins bind throughout the INK4A-ARF locus and are disassociated in senescent cells. Genes Dev. 21, 525–530. doi: 10.1101/gad.415507
Braig, M., Lee, S., Loddenkemper, C., Rudolph, C., Peters, A. H., Schlegelberger, B., et al. (2005). Oncogene-induced senescence as an initial barrier in lymphoma development. Nature 436, 660–665. doi: 10.1038/nature03841
Cherrier, T., Suzanne, S., Redel, L., Calao, M., Marban, C., Samah, B., et al. (2009). p21(WAF1) gene promoter is epigenetically silenced by CTIP2 and SUV39H1. Oncogene 28, 3380–3389. doi: 10.1038/onc.2009.193
Cristofalo, V. J., Allen, R. G., Pignolo, R. J., Martin, B. G., and Beck, J. C. (1998). Relationship between donor age and the replicative lifespan of human cells in culture: a reevaluation. Proc. Natl. Acad. Sci. U.S.A. 95, 10614–10619. doi: 10.1073/pnas.95.18.10614
Cunningham, C. P., Kimpton, W. G., Holder, J. E., and Cahill, R. N. (2001). Thymic export in aged sheep: a continuous role for the thymus throughout pre- and postnatal life. Eur. J. Immunol. 31, 802–811. doi: 10.1002/1521-4141(200103)31:3<802::AID-IMMU802>3.0.CO;2-P
Czvitkovich, S., Sauer, S., Peters, A. H., Deiner, E., Wolf, A., Laible, G., et al. (2001). Over-expression of the SUV39H1 histone methyltransferase induces altered proliferation and differentiation in transgenic mice. Mech. Dev. 107, 141–153. doi: 10.1016/S0925-4773(01)00464-6
d'Adda di Fagagna, F., Reaper, P. M., Clay-Farrace, L., Fiegler, H., Carr, P., Von Zglinicki, T., et al. (2003). A DNA damage checkpoint response in telomere-initiated senescence. Nature 426, 194–198. doi: 10.1038/nature02118
Dang, W., Steffen, K. K., Perry, R., Dorsey, J. A., Johnson, F. B., Shilatifard, A., et al. (2009). Histone H4 lysine 16 acetylation regulates cellular lifespan. Nature 459, 802–807. doi: 10.1038/nature08085
Degerman, S., Landfors, M., Siwicki, J. K., Revie, J., Borssen, M., Evelonn, E., et al. (2014). Immortalization of T-cells is accompanied by gradual changes in CpG methylation resulting in a profile resembling a subset of T-cell leukemias. Neoplasia 16, 606–615. doi: 10.1016/j.neo.2014.07.001
Dhalluin, C., Carlson, J. E., Zeng, L., He, C., Aggarwal, A. K., and Zhou, M. M. (1999). Structure and ligand of a histone acetyltransferase bromodomain. Nature 399, 491–496. doi: 10.1038/20974
Dhawan, S., Tschen, S.-I., and Bhushan, A. (2009). Bmi-1 regulates the Ink4a/Arf locus to control pancreatic β-cell proliferation. Genes Dev. 23, 906–911. doi: 10.1101/gad.1742609
Di Micco, R., Sulli, G., Dobreva, M., Liontos, M., Botrugno, O. A., Gargiulo, G., et al. (2011). Interplay between oncogene-induced DNA damage response and heterochromatin in senescence and cancer. Nat. Cell Biol. 13, 292–302. doi: 10.1038/ncb2170
Effros, R. B. (2004). From Hayflick to Walford: the role of T cell replicative senescence in human aging. Exp. Gerontol. 39, 885–890. doi: 10.1016/j.exger.2004.03.004
Farr, J. N., Fraser, D. G., Wang, H., Jaehn, K., Ogrodnik, M. B., Weivoda, M. M., et al. (2016). Identification of senescent cells in the bone microenvironment. J. Bone Miner. Res. 31, 1920–1929. doi: 10.1002/jbmr.2892
Ferguson, F. G., Wikby, A., Maxson, P., Olsson, J., and Johansson, B. (1995). Immune parameters in a longitudinal study of a very old population of Swedish people: a comparison between survivors and nonsurvivors. J. Gerontol. A Biol. Sci. Med. Sci. 50, B378–B382. doi: 10.1093/gerona/50A.6.B378
Feser, J., and Tyler, J. (2011). Chromatin structure as a mediator of aging. FEBS Lett. 585, 2041–2048. doi: 10.1016/j.febslet.2010.11.016
Feser, J., Truong, D., Das, C., Carson, J. J., Kieft, J., Harkness, T., et al. (2010). Elevated histone expression promotes life span extension. Mol. Cell 39, 724–735. doi: 10.1016/j.molcel.2010.08.015
Flores, K. G., Li, J., Sempowski, G. D., Haynes, B. F., and Hale, L. P. (1999). Analysis of the human thymic perivascular space during aging. J. Clin. Invest. 104, 1031–1039. doi: 10.1172/JCI7558
Galvani, A., Courbeyrette, R., Agez, M., Ochsenbein, F., Mann, C., and Thuret, J. Y. (2008). In vivo study of the nucleosome assembly functions of ASF1 histone chaperones in human cells. Mol. Cell. Biol. 28, 3672–3685. doi: 10.1128/MCB.00510-07
Garcia-Cao, M., O'Sullivan, R., Peters, A. H., Jenuwein, T., and Blasco, M. A. (2004). Epigenetic regulation of telomere length in mammalian cells by the Suv39h1 and Suv39h2 histone methyltransferases. Nat. Genet. 36, 94–99. doi: 10.1038/ng1278
Gorbunova, V., Seluanov, A., Mao, Z., and Hine, C. (2007). Changes in DNA repair during aging. Nucleic Acids Res. 35, 7466–7474. doi: 10.1093/nar/gkm756
Grotewiel, M. S., Martin, I., Bhandari, P., and Cook-Wiens, E. (2005). Functional senescence in Drosophila melanogaster. Ageing Res. Rev. 4, 372–397. doi: 10.1016/j.arr.2005.04.001
Groth, A., Corpet, A., Cook, A. J., Roche, D., Bartek, J., Lukas, J., et al. (2007). Regulation of replication fork progression through histone supply and demand. Science 318, 1928–1931. doi: 10.1126/science.1148992
Guelen, L., Pagie, L., Brasset, E., Meuleman, W., Faza, M. B., Talhout, W., et al. (2008). Domain organization of human chromosomes revealed by mapping of nuclear lamina interactions. Nature 453, 948–951. doi: 10.1038/nature06947
Hahn, W. C., Counter, C. M., Lundberg, A. S., Beijersbergen, R. L., Brooks, M. W., and Weinberg, R. A. (1999). Creation of human tumour cells with defined genetic elements. Nature 400, 464–468. doi: 10.1038/22780
Harley, C. B., Futcher, A. B., and Greider, C. W. (1990). Telomeres shorten during ageing of human fibroblasts. Nature 345, 458–460. doi: 10.1038/345458a0
Hayflick, L. (1965). The limited in vitro lifetime of human diploid cell strains. Exp. Cell Res. 37, 614–636. doi: 10.1016/0014-4827(65)90211-9
Herbig, U., Ferreira, M., Condel, L., Carey, D., and Sedivy, J. M. (2006). Cellular senescence in aging primates. Science 311:1257. doi: 10.1126/science.1122446
Heyn, H., Li, N., Ferreira, H. J., Moran, S., Pisano, D. G., Gomez, A., et al. (2012). Distinct DNA methylomes of newborns and centenarians. Proc. Natl. Acad. Sci. U.S.A. 109, 10522–10527. doi: 10.1073/pnas.1120658109
Holliday, R. (1986). Strong effects of 5-azacytidine on the in vitro lifespan of human diploid fibroblasts. Exp. Cell Res. 166, 543–552. doi: 10.1016/0014-4827(86)90499-4
Horvath, S. (2013). DNA methylation age of human tissues and cell types. Genome Biol. 14:R115. doi: 10.1186/gb-2013-14-10-r115
Huang, J. C., Yan, L. Y., Lei, Z. L., Miao, Y. L., Shi, L. H., Yang, J. W., et al. (2007). Changes in histone acetylation during postovulatory aging of mouse oocyte. Biol. Reprod. 77, 666–670. doi: 10.1095/biolreprod.107.062703
Huang, S., Risques, R. A., Martin, G. M., Rabinovitch, P. S., and Oshima, J. (2008). Accelerated telomere shortening and replicative senescence in human fibroblasts overexpressing mutant and wild-type lamin A. Exp. Cell Res. 314, 82–91. doi: 10.1016/j.yexcr.2007.08.004
Ishimi, Y., Kojima, M., Takeuchi, F., Miyamoto, T., Yamada, M., and Hanaoka, F. (1987). Changes in chromatin structure during aging of human skin fibroblasts. Exp. Cell Res. 169, 458–467. doi: 10.1016/0014-4827(87)90206-0
Jenuwein, T. (2001). Re-SET-ting heterochromatin by histone methyltransferases. Trends Cell Biol. 11, 266–273. doi: 10.1016/S0962-8924(01)02001-3
Jenuwein, T., and Allis, C. D. (2001). Translating the histone code. Science 293, 1074–1080. doi: 10.1126/science.1063127
Johansson, A., Enroth, S., and Gyllensten, U. (2013). Continuous aging of the human DNA methylome throughout the human lifespan. PLoS ONE 8:e67378. doi: 10.1371/journal.pone.0067378
Kaimori, J. Y., Maehara, K., Hayashi-Takanaka, Y., Harada, A., Fukuda, M., Yamamoto, S., et al. (2016). Histone H4 lysine 20 acetylation is associated with gene repression in human cells. Sci. Rep. 6:24318. doi: 10.1038/srep24318
Kaplan, T., Liu, C. L., Erkmann, J. A., Holik, J., Grunstein, M., Kaufman, P. D., et al. (2008). Cell cycle- and chaperone-mediated regulation of H3K56ac incorporation in yeast. PLoS Genet. 4:e1000270. doi: 10.1371/journal.pgen.1000270
Kawakami, K., Nakamura, A., Ishigami, A., Goto, S., and Takahashi, R. (2009). Age-related difference of site-specific histone modifications in rat liver. Biogerontology 10, 415–421. doi: 10.1007/s10522-008-9176-0
Kaygun, H., and Marzluff, W. F. (2005). Regulated degradation of replication-dependent histone mRNAs requires both ATR and Upf1. Nat. Struct. Mol. Biol. 12, 794–800. doi: 10.1038/nsmb972
Kozak, M. L., Chavez, A., Dang, W., Berger, S. L., Ashok, A., Guo, X., et al. (2010). Inactivation of the Sas2 histone acetyltransferase delays senescence driven by telomere dysfunction. EMBO J. 29, 158–170. doi: 10.1038/emboj.2009.314
Krishnamurthy, J., Ramsey, M. R., Ligon, K. L., Torrice, C., Koh, A., Bonner-Weir, S., et al. (2006). p16INK4a induces an age-dependent decline in islet regenerative potential. Nature 443, 453–457. doi: 10.1038/nature05092
Kuo, M. H., and Allis, C. D. (1998). Roles of histone acetyltransferases and deacetylases in gene regulation. Bioessays 20, 615–626. doi: 10.1002/(SICI)1521-1878(199808)20:8<615::AID-BIES4>3.0.CO;2-H
Kuwahara, M., Suzuki, J., Tofukuji, S., Yamada, T., Kanoh, M., Matsumoto, A., et al. (2014). The Menin-Bach2 axis is critical for regulating CD4 T-cell senescence and cytokine homeostasis. Nat. Commun. 5:3555. doi: 10.1038/ncomms4555
Larson, K., Yan, S. J., Tsurumi, A., Liu, J., Zhou, J., Gaur, K., et al. (2012). Heterochromatin formation promotes longevity and represses ribosomal RNA synthesis. PLoS Genet. 8:e1002473. doi: 10.1371/journal.pgen.1002473
Lawrence, T. (2009). The nuclear factor NF-kappaB pathway in inflammation. Cold Spring Harb. Perspect. Biol. 1:a001651. doi: 10.1101/cshperspect.a001651
Lehnertz, B., Ueda, Y., Derijck, A. A., Braunschweig, U., Perez-Burgos, L., Kubicek, S., et al. (2003). Suv39h-mediated histone H3 lysine 9 methylation directs DNA methylation to major satellite repeats at pericentric heterochromatin. Curr. Biol. 13, 1192–1200. doi: 10.1016/S0960-9822(03)00432-9
Liu, B., Wang, J., Chan, K. M., Tjia, W. M., Deng, W., Guan, X., et al. (2005). Genomic instability in laminopathy-based premature aging. Nat. Med. 11, 780–785. doi: 10.1038/nm1266
Liu, B., Wang, Z., Zhang, L., Ghosh, S., Zheng, H., and Zhou, Z. (2013). Depleting the methyltransferase Suv39h1 improves DNA repair and extends lifespan in a progeria mouse model. Nat. Commun. 4:1868. doi: 10.1038/ncomms2885
Lomberk, G., Mathison, A. J., Grzenda, A., Seo, S., DeMars, C. J., Rizvi, S., et al. (2012). Sequence-specific recruitment of heterochromatin protein 1 via interaction with Kruppel-like factor 11, a human transcription factor involved in tumor suppression and metabolic diseases. J. Biol. Chem. 287, 13026–13039. doi: 10.1074/jbc.M112.342634
Ludwig, G., Nejman, D., Hecht, M., Orlanski, S., Abu-Remaileh, M., Yanuka, O., et al. (2014). Aberrant DNA Methylation in ES Cells. PLoS ONE 9:e96090. doi: 10.1371/journal.pone.0096090
Lundblad, V., and Szostak, J. W. (1989). A mutant with a defect in telomere elongation leads to senescence in yeast. Cell 57, 633–643. doi: 10.1016/0092-8674(89)90132-3
MacKall, C. L., Bare, C. V., Granger, L. A., Sharrow, S. O., Titus, J. A., and Gress, R. E. (1996). Thymic-independent T cell regeneration occurs via antigen-driven expansion of peripheral T cells resulting in a repertoire that is limited in diversity and prone to skewing. J. Immunol. 156, 4609–4616.
Maegawa, S., Hinkal, G., Kim, H. S., Shen, L., Zhang, L., Zhang, J., et al. (2010). Widespread and tissue specific age-related DNA methylation changes in mice. Genome Res. 20, 332–340. doi: 10.1101/gr.096826.109
McClay, J. L., Aberg, K. A., Clark, S. L., Nerella, S., Kumar, G., Xie, L. Y., et al. (2014). A methylome-wide study of aging using massively parallel sequencing of the methyl-CpG-enriched genomic fraction from blood in over 700 subjects. Hum. Mol. Genet. 23, 1175–1185. doi: 10.1093/hmg/ddt511
Michishita, E., McCord, R. A., Berber, E., Kioi, M., Padilla-Nash, H., Damian, M., et al. (2008). SIRT6 is a histone H3 lysine 9 deacetylase that modulates telomeric chromatin. Nature 452, 492–496. doi: 10.1038/nature06736
Mostoslavsky, R., Chua, K. F., Lombard, D. B., Pang, W. W., Fischer, M. R., Gellon, L., et al. (2006). Genomic instability and aging-like phenotype in the absence of mammalian SIRT6. Cell 124, 315–329. doi: 10.1016/j.cell.2005.11.044
Mungamuri, S. K., Benson, E. K., Wang, S., Gu, W., Lee, S. W., and Aaronson, S. A. (2012). p53-mediated heterochromatin reorganization regulates its cell fate decisions. Nat. Struct. Mol. Biol. 19, 478–484. doi: 10.1038/nsmb.2271
Narita, M., Nunez, S., Heard, E., Lin, A. W., Hearn, S. A., Spector, D. L., et al. (2003). Rb-mediated heterochromatin formation and silencing of E2F target genes during cellular senescence. Cell 113, 703–716. doi: 10.1016/S0092-8674(03)00401-X
Ng, M. H., Aminuddin, B. S., Hamizah, S., Lynette, C., Mazlyzam, A. L., and Ruszymah, B. H. (2009). Correlation of donor age and telomerase activity with in vitro cell growth and replicative potential for dermal fibroblasts and keratinocytes. J. Tissue Viability 18, 109–116. doi: 10.1016/j.jtv.2009.06.003
Oberdoerffer, P., Michan, S., McVay, M., Mostoslavsky, R., Vann, J., Park, S. K., et al. (2008). SIRT1 redistribution on chromatin promotes genomic stability but alters gene expression during aging. Cell 135, 907–918. doi: 10.1016/j.cell.2008.10.025
Ortman, C. L., Dittmar, K. A., Witte, P. L., and Le, P. T. (2002). Molecular characterization of the mouse involuted thymus: aberrations in expression of transcription regulators in thymocyte and epithelial compartments. Int. Immunol. 14, 813–822. doi: 10.1093/intimm/dxf042
O'Sullivan, R. J., and Karlseder, J. (2012). The great unravelling: chromatin as a modulator of the aging process. Trends Biochem. Sci. 37, 466–476. doi: 10.1016/j.tibs.2012.08.001
O'Sullivan, R. J., Kubicek, S., Schreiber, S. L., and Karlseder, J. (2010). Reduced histone biosynthesis and chromatin changes arising from a damage signal at telomeres. Nat. Struct. Mol. Biol. 17, 1218–1225. doi: 10.1038/nsmb.1897
Peleg, S., Sananbenesi, F., Zovoilis, A., Burkhardt, S., Bahari-Javan, S., Agis-Balboa, R. C., et al. (2010). Altered histone acetylation is associated with age-dependent memory impairment in mice. Science 328, 753–756. doi: 10.1126/science.1186088
Peric-Hupkes, D., Meuleman, W., Pagie, L., Bruggeman, S. W., Solovei, I., Brugman, W., et al. (2010). Molecular maps of the reorganization of genome-nuclear lamina interactions during differentiation. Mol. Cell 38, 603–613. doi: 10.1016/j.molcel.2010.03.016
Peters, A. H., O'Carroll, D., Scherthan, H., Mechtler, K., Sauer, S., Schofer, C., et al. (2001). Loss of the Suv39h histone methyltransferases impairs mammalian heterochromatin and genome stability. Cell 107, 323–337. doi: 10.1016/S0092-8674(01)00542-6
Pina, B., and Suau, P. (1985). Core histone variants and ubiquitinated histones 2A and 2B of rat cerebral cortex neurons. Biochem. Biophys. Res. Commun. 133, 505–510.
Pina, B., Martinez, P., and Suau, P. (1988). Differential acetylation of core histones in rat cerebral cortex neurons during development and aging. Eur. J. Biochem. 174, 311–315.
Rando, T. A. (2006). Stem cells, ageing and the quest for immortality. Nature 441, 1080–1086. doi: 10.1038/nature04958
Rodier, F., Coppe, J. P., Patil, C. K., Hoeijmakers, W. A., Munoz, D. P., Raza, S. R., et al. (2009). Persistent DNA damage signalling triggers senescence-associated inflammatory cytokine secretion. Nat. Cell Biol. 11, 973–979. doi: 10.1038/ncb1909
Rogakou, E. P., and Sekeri-Pataryas, K. E. (1999). Histone variants of H2A and H3 families are regulated during in vitro aging in the same manner as during differentiation. Exp. Gerontol. 34, 741–754.
Rufer, N., Brummendorf, T. H., Kolvraa, S., Bischoff, C., Christensen, K., Wadsworth, L., et al. (1999). Telomere fluorescence measurements in granulocytes and T lymphocyte subsets point to a high turnover of hematopoietic stem cells and memory T cells in early childhood. J. Exp. Med. 190, 157–167. doi: 10.1084/jem.190.2.157
Ryan, J. M., and Cristofalo, V. J. (1972). Histone acetylation during aging of human cells in culture. Biochem. Biophys. Res. Commun. 48, 735–742.
Sarg, B., Koutzamani, E., Helliger, W., Rundquist, I., and Lindner, H. H. (2002). Postsynthetic trimethylation of histone H4 at lysine 20 in mammalian tissues is associated with aging. J. Biol. Chem. 277, 39195–39201. doi: 10.1074/jbc.M205166200
Scaffidi, P., and Misteli, T. (2006). Lamin A-dependent nuclear defects in human aging. Science 312, 1059–1063. doi: 10.1126/science.1127168
Schlesinger, Y., Straussman, R., Keshet, I., Farkash, S., Hecht, M., Zimmerman, J., et al. (2007). Polycomb-mediated methylation on Lys27 of histone H3 pre-marks genes for de novo methylation in cancer. Nat. Genet. 39, 232–236. doi: 10.1038/ng1950
Sedelnikova, O. A., Horikawa, I., Zimonjic, D. B., Popescu, N. C., Bonner, W. M., and Barrett, J. C. (2004). Senescing human cells and ageing mice accumulate DNA lesions with unrepairable double-strand breaks. Nat. Cell Biol. 6, 168–170. doi: 10.1038/ncb1095
Sedivy, J. M., Banumathy, G., and Adams, P. D. (2008). Aging by epigenetics–a consequence of chromatin damage? Exp. Cell Res. 314, 1909–1917. doi: 10.1016/j.yexcr.2008.02.023
Seluanov, A., Mittelman, D., Pereira-Smith, O. M., Wilson, J. H., and Gorbunova, V. (2004). DNA end joining becomes less efficient and more error-prone during cellular senescence. Proc. Natl. Acad. Sci. U.S.A. 101, 7624–7629. doi: 10.1073/pnas.0400726101
Sfeir, A., and de Lange, T. (2012). Removal of shelterin reveals the telomere end-protection problem. Science 336, 593–597. doi: 10.1126/science.1218498
Sidler, C., Woycicki, R., Ilnytskyy, Y., Metz, G., Kovalchuk, I., and Kovalchuk, O. (2013). Immunosenescence is associated with altered gene expression and epigenetic regulation in primary and secondary immune organs. Front. Genet. 4:211. doi: 10.3389/fgene.2013.00211
Sidler, C., Woycicki, R., Kovalchuk, I., and Kovalchuk, O. (2014a). WI-38 senescence is associated with global and site-specific hypomethylation. Aging 6, 564–574. doi: 10.18632/aging.100679
Sidler, C., Woycicki, R., Li, D., Wang, B., Kovalchuk, I., and Kovalchuk, O. (2014b). A role for SUV39H1-mediated H3K9 trimethylation in the control of genome stability and senescence in WI38 human diploid lung fibroblasts. Aging 6, 545–563. doi: 10.18632/aging.100678
Smith, J. R., Venable, S., Roberts, T. W., Metter, E. J., Monticone, R., and Schneider, E. L. (2002). Relationship between in vivo age and in vitro aging: assessment of 669 cell cultures derived from members of the Baltimore longitudinal study of aging. J. Gerontol. A Biol. Sci. Med. Sci. 57, B239–B246. doi: 10.1093/gerona/57.6.B239
Sohal, R. S., and Weindruch, R. (1996). Oxidative stress, caloric restriction, and aging. Science 273, 59–63. doi: 10.1126/science.273.5271.59
Sousa-Victor, P., Gutarra, S., Garcia-Prat, L., Rodriguez-Ubreva, J., Ortet, L., Ruiz-Bonilla, V., et al. (2014). Geriatric muscle stem cells switch reversible quiescence into senescence. Nature 506, 316–321. doi: 10.1038/nature13013
Steinmann, G. G., Klaus, B., and Muller-Hermelink, H. K. (1985). The involution of the ageing human thymic epithelium is independent of puberty. a morphometric study. Scand. J. Immunol. 22, 563–575. doi: 10.1111/j.1365-3083.1985.tb01916.x
Straussman, R., Nejman, D., Roberts, D., Steinfeld, I., Blum, B., Benvenisty, N., et al. (2009). Developmental programming of CpG island methylation profiles in the human genome. Nat. Struct. Mol. Biol. 16, 564–571. doi: 10.1038/nsmb.1594
Surh, C. D., and Sprent, J. (1994). T-cell apoptosis detected in situ during positive and negative selection in the thymus. Nature 372, 100–103. doi: 10.1038/372100a0
Sutton, A., Bucaria, J., Osley, M. A., and Sternglanz, R. (2001). Yeast ASF1 protein is required for cell cycle regulation of histone gene transcription. Genetics 158, 587–596.
Swanson, E. C., Manning, B., Zhang, H., and Lawrence, J. B. (2013). Higher-order unfolding of satellite heterochromatin is a consistent and early event in cell senescence. J. Cell Biol. 203, 929–942. doi: 10.1083/jcb.201306073
Tanae, K., Horiuchi, T., Matsuo, Y., Katayama, S., and Kawamukai, M. (2012). Histone chaperone Asf1 plays an essential role in maintaining genomic stability in fission yeast. PLoS ONE 7:e30472. doi: 10.1371/journal.pone.0030472
Thompson, R. F., Atzmon, G., Gheorghe, C., Liang, H. Q., Lowes, C., Greally, J. M., et al. (2010). Tissue-specific dysregulation of DNA methylation in aging. Aging Cell 9, 506–518. doi: 10.1111/j.1474-9726.2010.00577.x
Trebilcock, G. U., and Ponnappan, U. (1996). Evidence for lowered induction of nuclear factor kappa B in activated human T lymphocytes during aging. Gerontology 42, 137–146. doi: 10.1159/000213785
Uhlirova, R., Horakova, A. H., Galiova, G., Legartova, S., Matula, P., Fojtova, M., et al. (2010). SUV39h- and A-type lamin-dependent telomere nuclear rearrangement. J. Cell. Biochem. 109, 915–926. doi: 10.1002/jcb.22466
Vanyushin, B. F., Nemirovsky, L. E., Klimenko, V. V., Vasiliev, V. K., and Belozersky, A. N. (1973). The 5-methylcytosine in DNA of rats. tissue and age specificity and the changes induced by hydrocortisone and other agents. Gerontologia 19, 138–152. doi: 10.1159/000211967
Vaquero, A., Scher, M., Erdjument-Bromage, H., Tempst, P., Serrano, L., and Reinberg, D. (2007). SIRT1 regulates the histone methyl-transferase SUV39H1 during heterochromatin formation. Nature 450, 440–444. doi: 10.1038/nature06268
Vaziri, H., and Benchimol, S. (1996). From telomere loss to p53 induction and activation of a DNA-damage pathway at senescence: the telomere loss/DNA damage model of cell aging. Exp. Gerontol. 31, 295–301. doi: 10.1016/0531-5565(95)02025-X
Vijg, J., and Dolle, M. E. (2002). Large genome rearrangements as a primary cause of aging. Mech. Ageing Dev. 123, 907–915. doi: 10.1016/S0047-6374(02)00028-3
Villeneuve, L. M., Kato, M., Reddy, M. A., Wang, M., Lanting, L., and Natarajan, R. (2010). Enhanced levels of microRNA-125b in vascular smooth muscle cells of diabetic db/db mice lead to increased inflammatory gene expression by targeting the histone methyltransferase Suv39h1. Diabetes 59, 2904–2915. doi: 10.2337/db10-0208
Vogt, M., Haggblom, C., Yeargin, J., Christiansen-Weber, T., and Haas, M. (1998). Independent induction of senescence by p16INK4a and p21CIP1 in spontaneously immortalized human fibroblasts. Cell Growth Differ. 9, 139–146.
Wang, C., Jurk, D., Maddick, M., Nelson, G., Martin-Ruiz, C., and von Zglinicki, T. (2009). DNA damage response and cellular senescence in tissues of aging mice. Aging Cell 8, 311–323. doi: 10.1111/j.1474-9726.2009.00481.x
Weng, N. P., Levine, B. L., June, C. H., and Hodes, R. J. (1995). Human naive and memory T lymphocytes differ in telomeric length and replicative potential. Proc. Natl. Acad. Sci. U.S.A. 92, 11091–11094. doi: 10.1073/pnas.92.24.11091
Williams, S. K., Truong, D., and Tyler, J. K. (2008). Acetylation in the globular core of histone H3 on lysine-56 promotes chromatin disassembly during transcriptional activation. Proc. Natl. Acad. Sci. U.S.A. 105, 9000–9005. doi: 10.1073/pnas.0800057105
Wilson, V. L., and Jones, P. A. (1983). DNA methylation decreases in aging but not in immortal cells. Science 220, 1055–1057. doi: 10.1126/science.6844925
Wilson, V. L., Smith, R. A., Ma, S., and Cutler, R. G. (1987). Genomic 5-methyldeoxycytidine decreases with age. J. Biol. Chem. 262, 9948–9951.
Ye, X., Zerlanko, B., Zhang, R., Somaiah, N., Lipinski, M., Salomoni, P., et al. (2007). Definition of pRB- and p53-dependent and -independent steps in HIRA/ASF1a-mediated formation of senescence-associated heterochromatin foci. Mol. Cell. Biol. 27, 2452–2465. doi: 10.1128/MCB.01592-06
Zhang, R., and Adams, P. D. (2007). Heterochromatin and its relationship to cell senescence and cancer therapy. Cell Cycle 6, 784–789. doi: 10.4161/cc.6.7.4079
Zhang, W., Li, J., Suzuki, K., Qu, J., Wang, P., Zhou, J., et al. (2015). Aging stem cells. A Werner syndrome stem cell model unveils heterochromatin alterations as a driver of human aging. Science 348, 1160–1163. doi: 10.1126/science.aaa1356
Zheng, H., Chen, L., Pledger, W. J., Fang, J., and Chen, J. (2014). p53 promotes repair of heterochromatin DNA by regulating JMJD2b and SUV39H1 expression. Oncogene 33, 734–744. doi: 10.1038/onc.2013.6
Keywords: aging, cell senescence, epigenetic regulation, DNA methylation, heterochromatin, histone modifications
Citation: Sidler C, Kovalchuk O and Kovalchuk I (2017) Epigenetic Regulation of Cellular Senescence and Aging. Front. Genet. 8:138. doi: 10.3389/fgene.2017.00138
Received: 07 May 2017; Accepted: 14 September 2017;
Published: 26 September 2017.
Edited by:
Elena G. Pasyukova, Institute of Molecular Genetics of Russian Academy of Sciences, RussiaReviewed by:
Kaoru Tominaga, Jichi Medical University, JapanCopyright © 2017 Sidler, Kovalchuk and Kovalchuk. This is an open-access article distributed under the terms of the Creative Commons Attribution License (CC BY). The use, distribution or reproduction in other forums is permitted, provided the original author(s) or licensor are credited and that the original publication in this journal is cited, in accordance with accepted academic practice. No use, distribution or reproduction is permitted which does not comply with these terms.
*Correspondence: Igor Kovalchuk, aWdvci5rb3ZhbGNodWtAdWxldGguY2E=
Disclaimer: All claims expressed in this article are solely those of the authors and do not necessarily represent those of their affiliated organizations, or those of the publisher, the editors and the reviewers. Any product that may be evaluated in this article or claim that may be made by its manufacturer is not guaranteed or endorsed by the publisher.
Research integrity at Frontiers
Learn more about the work of our research integrity team to safeguard the quality of each article we publish.