- 1Department of Neurogenetics, Pavlov Institute of Physiology, Russian Academy of Sciences, St. Petersburg, Russia
- 2Department of Biophysical Chemistry and Molecular Oncology, Institute of Biophysics, Academy of Sciences of the Czech Republic, Brno, Czechia
- 3Department of Human and Animal Anatomy and Physiology, Herzen State Pedagogical University, St. Petersburg, Russia
- 4Department of Molecular Mechanisms of Development, Koltzov Institute of Developmental Biology, Russian Academy of Sciences, Moscow, Russia
- 5Department of Molecular Mechanisms of Biological Adaptation, Engelhardt Institute of Molecular Biology, Russian Academy of Sciences, Moscow, Russia
- 6Department of Biochemical Genetics of Animals, Institute of Molecular Genetics, Russian Academy of Sciences, Moscow, Russia
Genomic disorders, the syndromes with multiple manifestations, may occur sporadically due to unequal recombination in chromosomal regions with specific architecture. Therefore, each patient may carry an individual structural variant of DNA sequence (SV) with small insertions and deletions (INDELs) sometimes less than 10 bp. The transposable elements of the Tc1/mariner superfamily are often associated with hotspots for homologous recombination involved in human genetic disorders, such as Williams Beuren Syndromes (WBS) with LIM-kinase 1-dependent cognitive defects. The Drosophila melanogaster mutant agnts3 has unusual architecture of the agnostic locus harboring LIMK1: it is a hotspot of chromosome breaks, ectopic contacts, underreplication, and recombination. Here, we present the analysis of LIMK1-containing locus sequencing data in agnts3 and three D. melanogaster wild-type strains—Canton-S, Berlin, and Oregon-R. We found multiple strain-specific SVs, namely, single base changes and small INDEls. The specific feature of agnts3 is 28 bp A/T-rich insertion in intron 1 of LIMK1 and the insertion of mobile S-element from Tc1/mariner superfamily residing ~460 bp downstream LIMK1 3′UTR. Neither of SVs leads to amino acid substitutions in agnts3 LIMK1. However, they apparently affect the nucleosome distribution, non-canonical DNA structure formation and transcriptional factors binding. Interestingly, the overall expression of miRNAs including the biomarkers for human neurological diseases, is drastically reduced in agnts3 relative to the wild-type strains. Thus, LIMK1 DNA structure per se, as well as the pronounced changes in total miRNAs profile, probably lead to LIMK1 dysregulation and complex behavioral dysfunctions observed in agnts3 making this mutant a simple plausible Drosophila model for WBS.
Introduction
Currently, nuclear organization and 3D chromatin architecture are believed to play a main role in cognition and neuropsychiatric disorders (Medrano-Fernández and Barco, 2016).
The genomic diseases representing syndromes with multiple manifestations, occur spontaneously and sporadically as a result of contiguous deletions and duplications generated by unequal recombination in chromosomal regions with a specific architecture. Among them are the Williams Beuren syndrome, Smith-Magenis syndrome, DiGeorge syndrome (Carvalho and Lupski, 2016). These syndromes occur with a frequency of 0.7–1.0 per 1,000 live births, share neurodevelopmental phenotypes, and are detected by genome-wide segmental aneuploidy screening.
Genome-wide studies of the genomic disorders have uncovered the key role of genome architecture in the formation of structural variants (SVs). The definition of SVs overlaps with the concept of small insertions and deletions (INDELs, Mills et al., 2006). Hundreds of INDELs altering miRNA target sites of genes related to human disease pathways were identified (Bhattacharya et al., 2012). Transposable genetic elements (TEs) present an example of INDELs; upon integrating they may cause DNA insertions leading to human diseases (Ostertag and Kazazian, 2001; Mullaney et al., 2010; Carvalho and Lupski, 2016). Furthermore, miRNAs in the central nervous system are involved in epigenetic networks tuned by INDELs and TEs (Feschotte, 2008; Mattick, 2011; Morris and Mattick, 2014; Cao et al., 2016).
The DNA sequences can adopt non-B conformation, such as cruciforms, thereby affecting chromosomal structural changes in non-sequence specific manner (Hastings et al., 2009; Inagaki et al., 2009; Brázda et al., 2011; Kim and Kim, 2016). Breakpoints of gross deletions are found at the sites of non-B DNA conformation which trigger genomic rearrangements due to recombination-repair activities (Bacolla et al., 2004; Carvalho and Lupski, 2016).
Therefore, a new term “disorders of genome architecture” has been introduced to highlight a new paradigm regarding the contribution of non-B DNA structures in mutagenesis and the etiology of human genetic diseases (Wells, 2007, 2009; Kumar, 2008).
In particular, the role of chromosomal architecture is emphasized by studies of Williams- Beuren Syndrome (WBS). WBS is a neurodevelopmental disorder resulted from a heterozygous de novo, recurrent deletion of 26–28 genes at 7q11.23 due to nonallelic homologous recombination between large flanking low copy repeats (LCRs), including Hsmar2 transposable element from Tc1/mariner superfamily and facilitated by SVs or CNVs of the region (Pérez Jurado, 2003; Cusco et al., 2008; Gil et al., 2013). WBS is characterized by dysmorphic features, cardiovascular pathology, hypersociability, strong fixation on faces and cognitive visuospatial deficits These symptoms are often accompanied by manifestations of attention deficit hyperactivity disorder (ADHD) in WBS patients (Hoogenraad et al., 2004; Nikitina et al., 2014b and refs. therein). Since understanding the mechanisms underlying the WBS neurocognitive profile is of significant clinical importance, for a long time researchers tried to gain insights in the genomic and environmental impacts on these de novo events and to elucidate the role of every gene from the deleted genes (Hehir-Kwa et al., 2011). This requires development of animal models having deletions or mutations of individual genes within the WBS critical region. Such animal models are valuable to evaluate novel therapeutic approaches. Therefore, the genomic structure of the region was precisely defined in primates and mice (Valero et al., 2000). These studies have shown that although the region is inverted relative to the human map, the order of the WBS genes is conserved in the mouse genome. Moreover, each gene from WBS deletion has its Drosophila ortholog, but in this case the genes are scattered over different chromosomes (Nikitina et al., 2014a,b). LIMK1 in Drosophila belongs to the genes responsible for neurocognitive phenotype. It encodes LIM kinase 1, which affects cytoskeletal dynamics by phosphorylating and inactivating cofilin, the main regulator of actin filaments (Meng et al., 2002; Hoogenraad et al., 2004).
Previously, we have described in D. melanogaster the X-chromosome agnostic locus harboring LIMK1 gene (Savvateeva-Popova et al., 2004; Nikitina et al., 2014b). The agnostic locus resides in 11AB region of the X-chromosome representing a hot spot of chromosomal breaks, ectopic contacts, underreplication in salivary gland chromosomes, and recombination (Hawley, 1980; Zhimulev et al., 1982; Xamena et al., 1985; Belyaeva et al., 1998). As a result, agnostic region is highly breakage-prone, and, hence, its length varies depending on a source—wild type strains Canton-S (CS), Oregon-R (OrR), or (Ber), presumably due to a high rate of spontaneous unequal recombination (Savvateeva-Popova et al., 2004; Medvedeva et al., 2008; Nikitina et al., 2014a). Characteristically, these strains differ in their cognitive abilities: Oregon-R shows deficits in short-term memory (STM) being normal in long-term memory (LTM) retention, on the contrary, Berlin demonstrates LTM deficits 8 days after training. STM and LTM are drastically suppressed in agnts3 relative to CS (Kaminskaya et al., 2012, 2015). Interestingly, agnts3 shows ts-lethality at all stages of development at 29°C, but not in the adults (Savvateeva-Popova et al., 2002, 2004). Adult agnts3 individuals demonstrate learning/memory defects, locomotor impairments and amyloid-like brain inclusions at normal temperature. Characteristically, heat shock treatment (HS) of agnts3 adults ameliorates these mutant manifestations. While LIMK1 protein level is increased in adult agnts3 brain at 22°C it drops down to that of the wild type at 29°C or 37°C (Medvedeva et al., 2008). This makes the mutation an appropriate model for gaining insights both into the genomic perturbances and environmental events provoking the de novo generation of WBS-linked manifestations.
Here, we report the results of LIMK1 sequencing in agnts3 and three wild-type strains that demonstrate the strain-specific SVs, namely, single base changes, small deletions, and insertions in promoter region, introns, and exons. The found INDELs may affect nucleosome formation probability (NFP) and, hence, DNA non-canonical structures formation and ectopic pairing, as well as transcription factors (TFs) and miRNAs binding. Additionally, for some reason total miRNAs expression is drastically reduced in agnts3 relative to the wild-type strains.
The data accumulated herein suggest that the mechanisms of agnts3 phenotype formation probably involve both LIMK1 DNA structural rearrangements and the expression changes of certain miRNAs regulating a wide range of neurological processes.
Materials and Methods
Fly Strains
The following D. melanogaster strains were used:
1. wild-type strain Canton-S (CS);
2. wild-type strain Berlin (Ber) isolated from the natural population of Berlin, Germany, and widely used in European behavioral studies;
3. wild-type strain Oregon-R (OrR) isolated from the natural population of Oregon, USA, and widely used over the world as a background for marker genes and balancers;
4. agnts3 mutant of the agnostic locus found identified in a screen for the X-chromosome-linked ethyl methanesulfonate (EMS) induced temperature-sensitive (ts) mutations affecting cAMP metabolism (Savvateeva and Kamyshev, 1981; Medvedeva and Savvateeva, 1991) on the background of CS. PCR mapping revealed 1.7 kb insertion ~1 kb apart from 3′-untranslated region (UTR) of the LIMK1 gene (Medvedeva et al., 2008).
All strains were kept in 160 mL vials on the standard yeast–raisin medium at 25 ± 0.5°C and a 12:12 daily illumination cycle. Five to seven days imago males were taken in sequencing experiments.
DNA Amplification and Sequencing
We have sequenced LIMK1 gene with its 5′-untranscribed region (5′-UTR) and 3′-UTR including ~200 bp upstream exon 1 and the inter-gene spacer sequence upstream CG1138 gene. For amplification and sequencing specific primers to Dmel\LIMK1 gene (FlyBase ID: FBgn0041203) were chosen using NCBI Primer-BLAST. Fly genomic DNA (5 males per sample) was prepared using DNA extraction with DEPC according to (www.MolecularCloning.com). The gene fragments were amplified by polymerase chain reaction using Long PCR Enzyme mix (Thermo Scientific®), separated by agarose gel electrophoresis and extracted from gel using QIAquick gel extraction kit (Qiagen®). DNA sequencing was performed using Big Dye v3.1 and Big Dye v1.1 reagents (Applied Biosystems®) and 3130 Genetic Analyzer. Chromatograms were evaluated using SeqScape® Software v2.6. Genomic LIMK1 sequence was used as a reference (GeneBank, http://www.ncbi.nlm.nih.gov/Genbank). The results of LIMK1 sequencing in the studied Drosophila strains are submitted to GeneBank (ID: Dlimk1_CantonS—JX987486; Dlimk1_agnosticts3—JX987487; Dlimk1_Oregon-R—JX987488; Dlimk1_Berlin—JX987489).
Heat Shock Treatment
A specially designed heat shock (HS) treatment protocol for Drosophila was used to modulate HS stress response in development and to assed its remote effects in adult 5-day-old flies (Savvateeva-Popova et al., 2008). For this, HS-treatment was applied to larvae III–pupa during formation of the central complex of the brain (critical for visual, motor, and courtship learning). The prepupae were subjected to acute heat shock in empty vials placed in a water bath for 30 min at 37°C.
Small RNA Libraries Preparation
Extract RNA reagent (Evrogen, Russia) was used for total RNA extraction from adult 5 days old males. To obtain the fraction of small RNA, ~25 μg of total RNA were separated using 15% polyacrylamide gel electrophoresis in the presence of Urea (8 M) following excision of small RNA fraction corresponding to 21–29 nts. Illumina TruSeq Small RNA prep kit (Illumina, USA) was used for mall RNA libraries preparation. Sequencing was done on an Illumina HiSeq 2000 platform.
miRNA Bioinformatic Analysis
Deep-sequencing results in ~10 million of small RNA reads for each library. Pre-processing procedure included: 3′-adapter cropping, filtration of reads by length filtration (>18 nt) and quality control (80% of nt should have ≥20 Phred quality). Filtered reads were mapped to the D. melanogaster genome (Dm3 release) by Bowtie with requiring of perfect match. The amount of mapped miRNAs reads was counted by BEDTools (v. 2.22) and mirbase annotation (r. 19) (Quinlan and Hall, 2010). Analysis of differentially expressed miRNAs was performed using edgeR (v. 3.10.2) package in R environment (v. 3.2.2) (Robinson et al., 2010).
Computer Modeling
Multiple DNA alignments were performed using SeaView software (Gouy et al., 2010). Homology-modeling of D. melanogaster LIMK1 protein kinase domain was performed using Swiss-Model software (Guex and Peitsch, 1997; Schwede et al., 2003; Arnold et al., 2006). IGF1R kinase catalytic domain (PDB ID: 1K3A_A) was used as a template for homology modeling. The protein 3D structures were visualized using VMD software (Humphrey et al., 1996).
The search for TF binding sites was performed using Mapper software (Marinescu et al., 2005). Additionally, the search for TF binding sites within polymorphic LIMK1 regions was fulfilled with the help of TFSEARCH (Heinemeyer et al., 1998) and ConSite (Sandelin et al., 2004) computer resources. The search for miRNA binding sites was done using TargetScanFly resource (Ruby et al., 2007) and Segal Lab miRNA prediction tool (Kertesz et al., 2007).
The nucleosome prediction, palindrome analysis, transcription factors (TF) and miRNAs binding site search was performed for strain-specific LIMK1 and adjacent sequences (from –223 to 8,264 bp relative to 0 bp, a LIMK1 A/C/E transcription start site). In the case of polymorphic nucleotides the strain-specific variants were chosen. Nucleosome prediction was made using NuPoP software (Xi et al., 2010).
DNA palindrome analysis and the search for potential DNA hairpins was performed using DNA analyzer server (Brázda et al., 2016) (Jan Kolomazník, Jiří Lýsek, Václav Brázda. DNA analyzer. Available at: http://bioinformatics.ibp.cz). The following parameter sets was used: size of palindrome: 6–500 bp, spacer: 0–1,000 bp, mismatches: 0–3.
Results
Comparative Analysis of Interstrain LIMK1 Polymorphisms
D. melanogaster LIMK1 (FBgn0041203) contains seven alternatively spliced exons, forming five different mRNA transcripts (Figure 1). mRNA A, C and E isoforms have common transcription start site (TSS) (0 bp), as well as translation start site (TlSS) (1,754 bp) within exon (1) D/F TSS (2510/2845 bp) and TlSS (2611/2906 bp) are within exon (2) Transcription termination sites (TTS) for different isoforms are the following: 6,767 (E), 7,248 bp (A/F), 7,808 bp (C/D) within exon 7. All protein isoforms have the same translation termination sites (TlTS) (6,254). C transcript has maximal length (5,627 bp) and F has minimal one (4,187 bp).
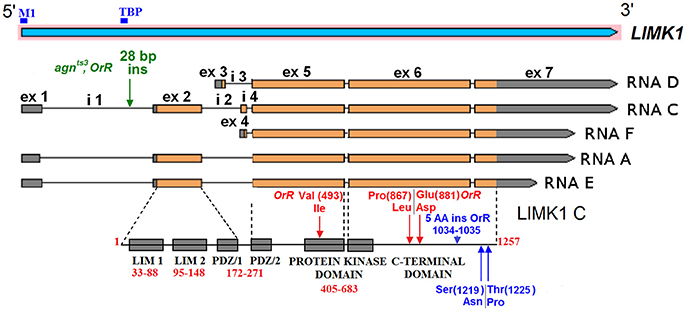
Figure 1. The structure of D. melanogaster LIMK1. The positions of exons and introns are given in accordance to FlyBase data (http://flybase.org/). The protein domain borders and the mutant amino acid residues are shown for LIMK1 C isoform. ex—exon, i—intron. Binging sites of two transcription factors: TATA-box-binding protein (TBP) and Forkhead box M1 (M1) are shown by blue bars.
We have found strain-specific INDELs in LIMK1 sequence in the wild-type strains (CS, OrR, Ber) and in agnts3 (Table S1). The sequence alterations can be subdivided into several classes according to their position and functional properties: (1) mutations in promoter region; (2) mutations in introns; (3) silent mutations in exons; (4) mutations that change amino acid (AA) sequence (Tables S1, S2). Ber sequence is the most similar to the published genomic LIMK1 sequence. About 20 single-nucleotide substitutions, insertions and deletions in intron 1 are common to agnts3 and OrR and also occur in CS strain. The most prominent of them is A/T rich 28 bp insertion. T(785)/G polymorphism occurs only in agnts3 LIMK1, but its functional effect is unknown.
The gene region from exon 5 to exon 6 encodes the major part of LIMK1 protein, from the middle of PDZ domain to C-terminal domain except ~100 terminal AA residues. In this region, multiple single base polymorphisms in CS sequence and the corresponding base substitutions in OrR are observed. The majority are silent, but five of them lead to the AA polymorphisms in CS and substitutions in OrR, respectively: Val(493)Ile, Pro(867)Leu, Glu(881)Asp. (Here and thereafter: the residue numbers are given for C isoform of reference protein sequence). Val(493)Ile substitution resides in the side loop beyond the catalytic site of the LIMK1 protein kinase domain (Figure 2). CS LIMK1 also contains two polymorphic residues within C-terminal part: Ser(1219)Asn, and Thr(1225)Pro. OrR contains five additional AA in C-terminal part (1034-1035 AA: Gly Thr Ile Val Asn) due to 15 bp insertion in exon 6 and this is a strain-specific feature. Exons 5 and 6 are similar in all protein isoforms. All substitutions in A—F isoforms do not lead to ORF shift. agnts3 and Ber exons 5 and 6 closely resemble those of genomic sequence.
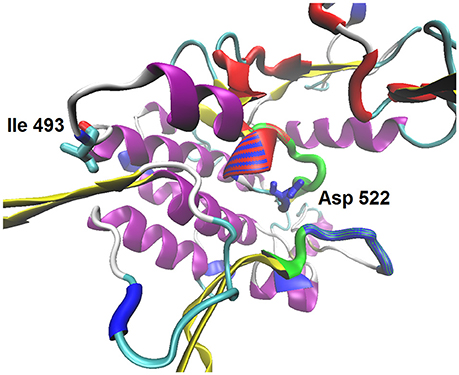
Figure 2. The model structure of D. melanogaster LIMK1 protein kinase domain. The color scheme: ATP-binding site is shown by red, the substrate binding site—by blue, the activation loop—by green; protein secondary structure: alpha-helixes are shown by magenta, beta-sheets—by yellow. Ile 493—the polymorphic residue at the external loop; Asp 522—the catalytic residue within the active side.
The insertion of S-element (1,734 bp) from ancient Tc1/mariner superfamily was found in agnts (456 bp downstream C and D LIMK1 TTS). S-LIMK1 sequence is nearly identical to that of S-AGO2{}[S-1008] detected in the second intron of the argonaute-2 gene (FlyBase ID: FBti0020119).
Transcription Factor Binding Sites Search
Several possible transcription factor (TF) binding sites are found within LIMK1 polymorphic regions (Table 1). 5′-UTR contains the binding sites for Hb and CF2-II within 100 bp upstream TSS. Substitutions A(–71)T and C(9)T are found in Hb and M1 sites of agnts3 and OrR sequences. TATA box precedes the 28 bp A/T–rich insertion. In addition to possible TF binding sites in LIMK1 polymorphic regions, several sequences with a high degree of similarity to TF binding sites (E < 1) were found in LIMK1 and S-LIMK1 sequences (Figure S1). Most of LIMK1 TF sites are shared by all strains, but some are variable. S-LIMK1 insertion creates additional 13 binding sites, such as Foxa2, Foxd3, Foxq1, and NFYA. Also, it promotes an appearance of additional br_Z1 binding sites in agnts3.
LIMK1 Nucleosome Distribution
Poly(A/T) tracts, among them A and A/T rich pentamers being the most frequent, are believed to disfavor nucleosome formation (Field et al., 2008). Computational analysis of NFP was performed for LIMK1 strain-specific sequences with additional 5′–end 2,000 bp to exclude terminal effects. Positions of presumptive nucleosome start/end and the nucleosome-free regions (NFRs) appeared to be the same in all strains (Figure 3A). NFRs tend to be associated with the functional sites of LIMK1: D TSS (2), mRNA E, A/F, and C/D TTS (3), (4), and (5) respectively. The A/T rich 28 bp insertion within NFR(1) increases its length, thereby preventing nucleosome formation. There are a few inter-strain differences in NFP, e.g., for 5′-UTR (2) containing Forkhead box M1 TF binding site it is higher in agnts3, OrR, and Ber than in CS (Figure 3B).
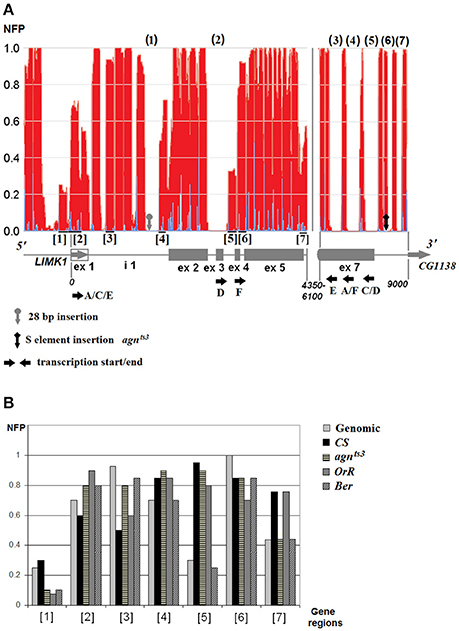
Figure 3. The nucleosome distribution on LIMK1 sequence. (A) Total nucleosome distribution on genomic LIMK1 DNA; (B) strain-specific NFP values. The nucleosome-free regions (NFRs) are shown by numbers in round brackets (N), the polymorphic LIMK1 regions with the nucleosomes—by numbers in square brackets [N]. The area ~4,350–6,100 in all strains is covered by nucleosomes, except small region ~4950–5075 (NFP 0.5).
The regions with low NFP value might be of probable functional significance: mRNA A/C/E promoter region, mRNA A/C/E TSS, and mRNA D/F TSS (regions 1, 2, and 5, respectively). The nucleosome-free regions at 3′-end may facilitate the mRNAs transcription termination.
As to S-element, its ends are free from nucleosomes (5′: ~400 bp, 3′: ~300 bp). The insertion site of S-LIMK1 is within a nucleosome close to its end, followed by ~120 bp NFR(6) (Figure 3). The insertion enlarges this region by ~360 bp leaving some ~400 bp nucleosome-free DNA at 3′ end of the transposon. Thus, S-LIMK1 creates downstream LIMK1 a large nucleosome-free region which may participate in non-homologous interactions with other NFRs, for instance A/T rich 28 bp insertion. This is the most striking feature of agnts3 LIMK1 sequence.
Northern hybridization with RNAs isolated from the wild-type strain probe revealed three transcripts: a large (3.7-kb) transcript was detectable at all stages, while two other transcripts were smaller, became detectable in third-instar larvae, and increased in size in adult females. The agnts3 mutants (male third-instar larvae) showed another expression pattern (Savvateeva-Popova et al., 2004). The functional significance of this is still unclear.
DNA Palindromes and Non-Canonical Structures
A search for DNA palindromes and potential DNA hairpins was performed within intron 1 sequence and sequence including nucleosome free regions (NFR) NFR(5)—NFR(7), with S-LIMK1 insertion in agnts3 (Table 2). The total number of palindromes for intron I mainly of 6–7 bp size is higher in agnts3 and OrR than in annotated genomic and Ber sequence. In NFR(5)—NFR(7) region, S-LIMK1 insertion leads to excess in the total number of palindromes over the sum for S-LIMK1 and genomic sequence alone. Thus, DNA sequence downstream agnts3 LIMK1 possibly forms the non-canonical structures that may influence LIMK1 expression.
Since 28 bp insertion and S-LIMK1 are present together only in agnts3, this may explain the changes in agnts3 LIMK1 expression. The aforementioned local strand separation in superhelical DNA might be utilized due to interaction between these two insertions. Along these lines we found a partial similarity between 28 bp fragment and S-LIMK1 (Tc1/mariner) terminal repeats (17 nucleotides in S-LIMK1 5′ and 19 nucleotides in S-LIMK1 3′), which may cause pairing within LIMK1, changing its architecture (Figure 4).
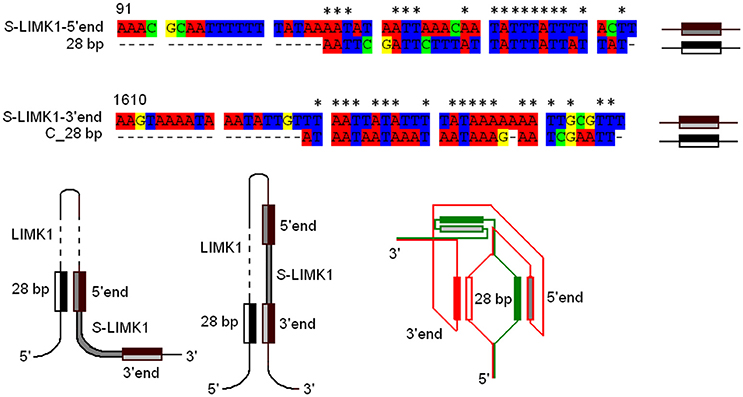
Figure 4. 28 bp—S-LIMK1 complex: possible DNA secondary structures. The sequence of 28 bp insertion was aligned with the sequences of S-LIMK1 5′- and 3′- inverted long terminal repeats (5′ end, 3′ end). C_28 bp—the sequence of “–”DNA strand complementary to 28 bp. Nucleotide similarities are indicated by asterisks (17 in S-LIMK1 5′ and 19 in 3′). Three possible secondary structures of 28 bp—S-LIMK1 complexes are shown: 28 bp—5′ end; 28 bp—3′ end; 28 bp—5′/3′ ends. For the third structure, two DNA single strands are shown by red and green colors.
miRNAs Strain-Specific Expression Profiles
The multiple phenotypic manifestations of agnts3 mutation permit to assume that it may affect some basic cellular regulatory mechanisms, in particular, the system of microRNA synthesis and processing. At present, 256 miRNA precursors and 466 mature miRNAs are known in D. melanogaster (miRBase 21 data) (Kozomara and Griffiths-Jones, 2014). The miRNAs expression profiles without and following HS given in development at the stage larvae III-prepupa to agnts, Ber and CS and assessed in 5-day old adults are presented in Figure 5. To reveal the miRNAs with significant changes in expression after HS exposure, similarly to previously described procedure (Funikov et al., 2016) only expression changes no less than log2FC ≥ 1.5 were considered. Furthermore, we discarded miRNAs with <50 counts as lowly expressed.
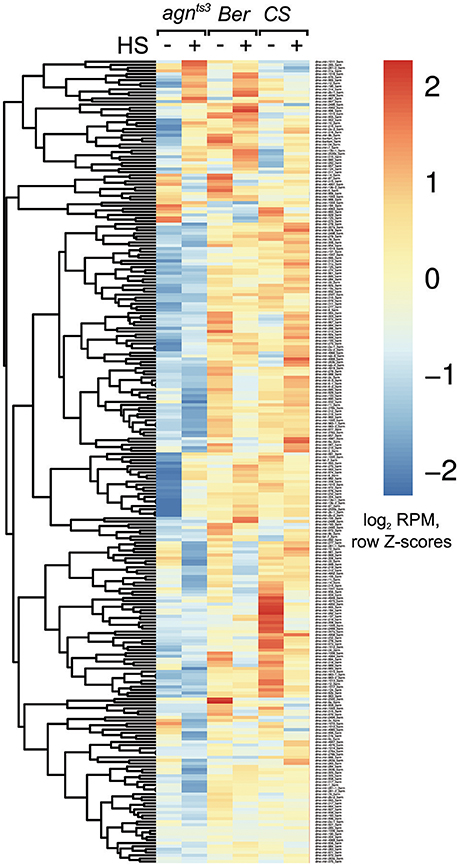
Figure 5. The expression of miRNAs in agnts3 compared to Berlin (Ber) and Canton- S (CS) without (–) and after (+) heat shock (HS). The heat map represents the RPM-normalized and log2-transformed counts of miRNAs reads with z-scale normalization of the rows. Thirty percent of low-expressed miRNAs were removed from further analysis.
Correlation analysis and principal component analysis (PCA), were performed. It revealed a high degree of miRNA expression similarity between biological replicates (Figure 6). One can see, that agnts3 demonstrates significant difference from the wild-type strains both without and after HS treatment (position on PC1 axis). At the same time the direction of miRNA expression changes after HS in agnts3 is similar to Berlin (increasing along PC2 axis, decreasing along PC1 axis), and somewhat less similar to Canton-S (increasing along PC2 axis).
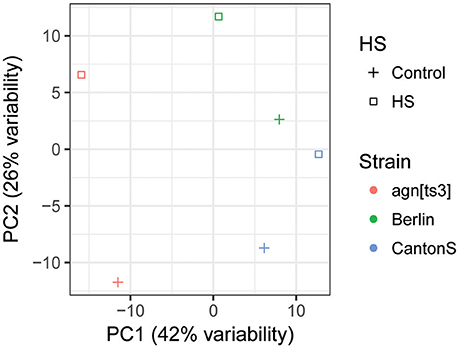
Figure 6. Principal component analysis (PCA) of miRNA expression for mutant agnts3 and two wild-type strains—Berlin and Canton-S.
In agnts3, 158 mature miRNAs demonstrate drastically reduced expression level relative to the other strains and as demonstrated by PCA, agnts3 has a very unique miRNA expression signature. Many of them are involved in regulation of the nervous system development, behavior, and cell survival, possibly explaining the agnts3—specific physiological and behavioral traits. Dramatic decrease of numerous miRNAs observed in agnts3 may be due to a mutation in a component of RNA interference machinery induced by EMS treatment used in the experiments where agnts3 was isolated. To check this possibility we monitored the expression of major genes involved in RNA interference in the mutant and other strains used in the study (Figure S2).
The observed diminished content of Dicer in agnts3 in comparison to Ber might be responsible for the manifestation of such a distinctive feature of agnts3, as the total decrease of miRNAs level compared to the wild-type strains. However, apparently it is not the case because the expression of Dicer 1 in agnts3and OrR does not differ (data not shown).
Bioinformatics Analysis of LIMK1-miRNAs Binding and miRNAs Formation
Seven D. melanogaster miRNAs according to TargetScanFly data may interact with LIMK1 3'-UTR. Six of them (92a_3arm, 92b_3arm, 310_3arm—313_3arm) belong to 7mer-1A family having the common binding site (6,685—6,691 bp).
Insertion of transposons may cause elongation of 3'-UTRs, thereby creating new miRNAs binding sites. Analysis of D. melanogaster miRNAs binding to agnts3 S-LIMK1 performed according to (Segal lab data; −10 kcal/mol threshold level of binding energy) demonstrates that it contains eight additional binding sites for miRNAs: 124_3arm, 312_3arm, 92_3arm, 34_5arm, 966_5arm, 87_3arm, 92b_3arm, and 1002_5arm (Figure S3).
Discussion
During the past 35 years the convergence of DNA structural biology, genetic, and genomic studies, bioinformatics, and medicine has led to a notion that in both prokaryotes and eukaryotes segments of DNA are conformationally polymorphic. They can exist in alternative non-B DNA forms, such as, cruciforms, slipped structures, triplexes, left-handed Z-DNA, and tetraplexes. These non-B DNA conformations at specific loci of chromosomes serve as a trigger of ~20 human neurological diseases and about 50 genomic disorders. Among psychiatric diseases are schizophrenia, drug and alcohol abuse, attention-deficit hyperactivity disorder, and anorexia-bulimia. These findings have brought a new paradigm in the etiology of human genetic diseases (Wells, 2007).
It states, that although chromosomal DNA exists predominantly in right-handed B form with Watson–Crick base pairing for most of the time, different environmental events, such as temperature fluctuations and drastic changes in concentration of certain cations, radiation, etc., can cause transition of individual DNA segments from the linear B-DNA form to at least 10 non-B DNA conformations. The transition based on flexibility and plasticity of DNA may be either temporal, or long lasting. They may either cause mutations, or provide “behavioral adaptation to new experiences in a rapidly changing environment” (Medrano-Fernández and Barco, 2016). This requires short and long-range interactions between DNA sequences that are located thousands of bases apart or even in different chromosomes for coordinated regulation and implies chromatin loopings that activate regulatory sequences within discrete genomic foci (Zhang et al., 2013). Recurrent genomic copy number variants (CNVs) is a by-product of such interactions. Among the de novo CNVs of known pathogenic significance observed in a number of genomic disorders (Kirov et al., 2012) was a duplication at the WBS region at 7q11.23. Noteworthy, LIMK1 is among the 25 genes deleted in WBS (Tassabehji et al., 1999; Medrano-Fernández and Barco, 2016), however, the gene is duplicated in some patients with autism or schizophrenia.
The development of the instruments controlling transition between conformations might serve as a new therapeutic strategy for these human diseases. This requires the usage of simple models and the plausible one is Drosophila model for WBS, i.e., the mutant and spontaneous variants of the agnostic locus harboring the X-chromosome-linked LIMK1 gene (Savvateeva-Popova et al., 2004; Nikitina et al., 2014a). The region is involved in Kosikov duplication characterized by homology between the X-chromosome regions 11A and 12D and between 11B and 12E (Kosikov, 1936), the genomic material from 11A10-A11 and 11B3-11B9 mirrors each other (Savvateeva-Popova et al., 2002, 2004). It is a hot spot of chromosomal breaks, ectopic contacts, underreplication (Zhimulev et al., 1982; Belyaeva et al., 1998), and recombination (Hawley, 1980) facilitated by chemical mutagen ethyl methanesulfonate (EMS) (Xamena et al., 1985). According to current views (Medrano-Fernández and Barco, 2016), this region apparently belongs to topological domains or topologically associating domains (TADs).
Temperature-sensitive (ts) mutation agnts3 showing ts-lethality at all stages of development at 29°C, but not in adulthood, was isolated in a screen for the X-chromosome EMS induced ts- mutations affecting cAMP metabolism (Savvateeva and Kamyshev, 1981; Medvedeva and Savvateeva, 1991). Adult agnts3 flies demonstrate three diagnostic features of NDs (Hirsch, 2006): memory defects, locomotor impairments, and amyloid-like brain inclusions at normal temperature. HS treatment resulted in disappearance of these mutant manifestations (Medvedeva et al., 2008).
Besides, agnts3 mutation leads to: (1) an increased level of LIMK1 and p-cofilin in the adult brain or 3rd instar larval salivary glands at 22°–25°C and a return to the wild type level at elevated temperature; (2) high rates of recombination modulated by temperature in the region of agnts3 localization; (3) three-fold increase in frequency of ectopic contacts in the same region (Medvedeva et al., 2008). Thus, agnts3 is a mutation not only changing the single-gene activity, but also the chromatin structure, and, as shown in this study, is expressed on the background of decreased miRNAs expression. Along these lines, recent findings indicate that 3D architecture of chromatin is involved in the transcriptional regulation of miRNAs (Chen et al., 2014). Also, a number of studies on different animal and plant species demonstrated that stress results in the modulation of miRNA levels. As shown in Drosophila (Funikov et al., 2016) strain-specific microRNA levels form a uniform microRNA pattern after HS. Besides, the different groups of such HS-sensitive miRNAs regulate functionally similar genes during the heat shock response (HSR).
In this study, we demonstrate that contrary to these recent findings, agnts3 has a very unique miRNA expression signature and manifests different types of HSR: miRNAs expression might increase, decrease, or remain unchanged. This follows from the analysis of heat maps of micro RNAs expression and PCA (principal component analysis) in agnts3 and the wild-type strains Belin, Canton-S, and Oregon-R (Figures 5, 6). Also, contrary to observed (Funikov et al., 2016), different strains may demonstrate an inverse HSR of same microRNAs. As for agnts3, the overall level of microRNAs expression both under normal conditions and after HS is significantly lower than in other strains studied herein. However, the expression of certain microRNAs blocks increases after HS up to the wild type level (Table S3). Since HS restores learning acquisition and memory retention in agnts3, the HS-induced increase in expression of specific microRNAs deserves a special attention: mir-1000 (negative regulation of glutamate secretion, neurotransmission), let-7, mir-8 (neuroblast development, regulation of NMJ development), members of the cluster mir-304 and mir-12 (regulation of smoothened signaling pathway). Similarly to our recent observation (Funikov et al., 2016), the expression levels of several clustered miRNAs respond to HS individually and independently of each other: in the cluster let-7, mir-100, mir-125 HS up-regulates let-7 expression, but down-regulates mir-100. Also, HS leads to down-expression of mir-277 cluster (mir-34, mir-317) and mir-306 cluster (mir-9c, mir-9b, mir-79). Noteworthy, these microRNAs belong to biomarkers of neurodegenerative diseases (NDs) (Maciotta et al., 2013) and all vertebrate miRNA families have representatives in Drosophila (Ibáñez-Ventoso et al., 2008). Since a single miRNA can regulate thousands of target genes, their deregulation is a major cause of NDDs, also termed as RNA disorders (Johnson et al., 2012). Moreover, among the 35–40 miRNAs highly abundant in the human CNS only six are the key players in chronic inflammatory NDDs. These are stress-regulated miRNA-7, miRNA-9, miRNA-34a, miRNA-125b, miRNA-146a, and miRNA-155 (Maciotta et al., 2013). The biomarker of PD is let-7/miR-184* (Maciotta et al., 2013). Findings in Drosophila (Venderova et al., 2009), demonstrate that let-7 target gene is LRRK2 (leucine-rich repeat kinase2). It is involved in regulation of actin cytoskeleton and interacts with the protein products of partner genes of LIMK1, such as Parkin and Pink. In Drosophila, let-7 is sensitive to steroid hormones and determines the onset of neuron formation in the brain mushroom bodies (Chawla and Sokol, 2012; Kucherenko and Shcherbata, 2013). A biomarker for AD is miR-34. This steroid hormones-sensitive miRNA is considered to be a key regulator of age-related physiological changes, because its target genes include tau, creb, and hsp70. Therefore, miR34 is involved in negative regulation of aging and death of neurons (Ghosh et al., 2008; Maciotta et al., 2013; Feng et al., 2014). The remarkable role of miR-34 in development and disease (Rokavec et al., 2014) is explained by the existence of the p53/miR-34 axis. The tumor suppressor p53 responding to a myriad different types of stress (among them hypoxia) and contributing to the pathology of NDDs binds directly to response elements within the miR-34a and miR-34b/c promoters that contain inverted repeats creating local cruciform structures (Coufal et al., 2013).
As show in this study, additional binding site for mir-34 is created by the insertion of S-element from Tc1/mariner family in agnts3 (Figure S3). The biomarker of HD is miR-36 (Maciotta et al., 2013). This individual manner of clustered miRNAs response to HS, specifically the miRNAs belonging to cluster 277–34, was also observed (Funikov et al., 2016). It could be, that similarly to recently revealed in C. elegans (Nehammer et al., 2015), this is a specific set of Drosophila miRNAs which play a crucial role during developmental survival and in behavioral functions after HS.
In this study we have found strain-specific INDEL polymorphisms in LIMK1 sequence in the wild-type strains (CS, OrR, Ber) and in agnts3 (Table 1, Table S1). The unique feature of agnts3 compared to the wild-type strains is the presence of S-element from Tc1/mariner family located 456 bp downstream LIMK1 transcription termination site (TTS). As shown in Drosophila, insertions of TEs downstream genes within 500 bp of TTS may efficiently suppress gene activity (Cridland et al., 2015). Notably, in humans members of Tc1/mariner superfamily posses numerous INDELs and serve as markers of a 1.7-kb recombination hot spot in genetic disorders such as Charcot–Marie–Tooth, Prader-Willi, Angelman, and Williams syndromes (Feschotte, 2008). Besides, the copies of this element located in multiple sites of D.melanogaster genome participate in the stimulation of homologous recombination between repeated sequences through the introduction of DBSs in DNA near to sites of strand exchange (Reiter et al., 1999). Probably, agnts3 represents an example of a chimeric gene born by capture of the TE (Cordaux et al., 2006) during EMS-induced mutagenesis, since EMS serves as an amplifier of pre-existing natural variability (Ohnishi, 1977). Moreover, S-element in agnts3 X:11AB region affects the chromatin structure changing the pattern of ectopic contacts and, thereby LIMK1 expression level. Interestingly, as shown in this study, four miRNAs reside in the X-chromosome regions which form ectopic contacts with X:11AB region (Table S3). Two of them, dme-mir-304_5arm and dme-mir -12_5arm, both located in 13C7 are involved in positive regulation of smoothened signaling pathway, dme-mir-969_3arm (16F7) is involved in germ cell development and dme-mir-2535b_3arm (19F3) has yet unknown functions. Interestingly, smoothened, and type 6 serotonin receptor involved in stress response are found in cilia, actin-based subcellular structures present in a majority of cells including neurons. They are envisioned as the cellular “antennae” attuned for detecting a range of environmental signals including photons, odorants, morphogens, hormones, and mechanical forces (Qiu et al., 2016).
Another kind of explanation of agnts3 ts-phenotypes and a support to a notion (Wells, 2007) that different environmental factors including HS, can cause transition of individual DNA segments from the linear B-DNA form to non-B DNA conformations comes from our previous findings (Lushnikov et al., 2014). First, unusual conformational DNA dynamics of 119 bp DNA fragment isolated from agnts3 Intron 1 harboring 28 bp A/T-rich insertion has been found by physical methods of Brillouin light scattering upon heating the DNA sample till temperatures of DNA denaturation. Second, computer modeling of possible conformations which might be attained by the A/T rich 28 bp DNA insertion has shown that cruciforms, nodes and bubbles might evolve upon increasing temperature in 22°–37°C range. These structures occur in vivo during local strand separation required for replication, recombination regulation of gene expression, and nucleosome remodeling (Brázda et al., 2011; Kim and Kim, 2016).
In agnts3 this insertion occurs together with the insertion of S-element and shows a partial similarity to its terminal repeats. This may cause pairing within LIMK1, changing its architecture (Figure 4) and thereby affecting agnts3 LIMK1 expression, at least in response to different temperature regimes (temperature sensitivity). As shown here (Figure 3), the inverted terminal repeats of S-LIMK1 (Tc1/mariner) are nucleosome-free, as well as A/T rich 28 bp insertion in intron 1. A genome-wide analysis of conformational properties of naked DNA in yeast (Deniz et al., 2011) demonstrates that nucleosome positions adjacent to TSS (transcription start site) and TTS (transcription termination site) mostly depend on physical features of the naked DNA that govern equilibrium of its conformations. Therefore, it is not surprising that Palindrome analyzer (Brázda et al., 2016) reveals a huge prevalence in cruciform structures formed in agnts3 LIMK1 sequence homing both S-LIMK1 (Tc1/mariner) and A/T rich 28 bp insertion in intron 1.
Moreover, as we have shown (Figure S1), that insertion of S-LIMK1 (Tc1/mariner) creates additional 13 TF binding sites, such as Foxa2, Foxd3, Foxq1, and NFY, and promotes an appearance of additional br_Z1 binding sites (ecdysone-sensitivity) in agnts3. Notably, spatial or temporal patterns of gene expression are set by the DNA cis-regulatory elements termed enhancers. They are enriched in TF binding sites that regulate gene activation and act from a distance to the TSS of their target genes. Besides, noncoding RNAs can be transcribed from enhancers (Plank and Dean, 2014). TF binding itself can realize in nucleosome-depleted stretches of DNA through interaction with other TFs in the same or other chromosome, thus providing a topological basis for transcription regulation (Li et al., 2012).
Therefore, spatial localization of S-element and 28 bp A/T-rich insertion sharing partial homology with terminal repeats of the transposon is capable of providing such a topological basis in agnts3 which may be considered as “a conformational mutant.”
Taken together, our results help to highlight the DNA conformational dynamics as a point of application of therapeutic strategies for neurological diseases and genomic disorders caused by INDELs, Transposable Elements of the Tc1/mariner Superfamily and microRNAs.
Author Contributions
Conceived and designed the experiments: AM, EN, JD, OZ, ME, and ES. Performed the experiments: AZ, GZ, AK, AM, EN, ET, JD, DK, OZ, SF, and SR. Analyzed the data: AZ, GZ, AK, AM, EN, ET, JD, DK, OZ, SF, SR, ME, and ES. Contributed reagents/materials/analysis tools: GZ, VB, ME, and ES. Wrote the paper: AZ, AM, JD, GZ, SF, ME, and ES.
Funding
All studies of miRNAs were supported by the Russian Science Foundation grant №14-50-00060 ME. This work was supported by the grant of the Russian Foundation for Basic Research (№15-04-07738 ES-P).
Conflict of Interest Statement
The authors declare that the research was conducted in the absence of any commercial or financial relationships that could be construed as a potential conflict of interest.
Supplementary Material
The Supplementary Material for this article can be found online at: http://journal.frontiersin.org/article/10.3389/fgene.2017.00123/full#supplementary-material
Figure S1. Transcription factor binding sites in LIMK1 and S-LIMK1 sequences (Mapper data). Red square: TF binding site. Two red rectangles: TF dimer binding site. Green square: the variable TF binding site and nucleotide polymorphism.
Figure S2. miRNA processing genes expression for agnts3 and Ber. Quantitative real-time PCR data. Relative expression levels of miRNA biogenesis pathway genes in studied Drosophila strains; mean values ± error bars for three replicates are represented. agnts3 genes with statistically significant differences (P ≤ 0.05) in expression level compared to Ber are shown by asterisk.
Figure S3. miRNA binding sites within polymorphic areas of LIMK1. miRNA binding sites are shown by triangles, only for extensive polymorphic areas. Ex—exon, i—intron.
Table S1. Strain-specific LIMK1 polymorphisms. Ins—insertion, del—deletion. The base number and nucleotides are shown for genomic sequence. For strain-specific sequnces, only the variants different from genomic sequence are shown. Red color: the unique agnts3 strain-specific polymorphism.
Table S2. The detected strain-specific polymorphisms in LIMK1 sequence and their possible effects.
References
Arnold, K., Bordoli, L., Kopp, J., and Schwede, T. (2006). The SWISS-MODEL workspace: a web-based environment for protein structure homology modelling. Bioinformatics 22, 195–201. doi: 10.1093/bioinformatics/bti770
Bacolla, A., Jaworski, A., Larson, J., Jakupciak, J., Chuzhanova, N., Abeysinghe, S., et al. (2004). Breakpoints of gross deletions coincide with non-B DNA conformations. Proc. Natl. Acad. Sci. U.S.A. 101, 14162–14167. doi: 10.1073/pnas.0405974101
Belyaeva, E., Zhimulev, I., Volkova, E., Alekseyenko, A., Moshkin, Y., and Koryakov, D. (1998). Su(UR)ES: a gene suppressing DNA underreplication in intercalary and pericentric heterochromatin of Drosophila melanogaster polytene chromosomes. Proc. Natl. Acad. Sci. U.S.A. 95, 7532–7537. doi: 10.1073/pnas.95.13.7532
Bhattacharya, A., Ziebarth, J., and Cui, Y. (2012). Systematic analysis of microRNA targeting impacted by small insertions and deletions in human genome. PLoS ONE 7:e46176. doi: 10.1371/journal.pone.0046176
Brázda, V., Kolomazník, J., Lýsek, J., Hároníková, L., Coufal, J., and Št'astný, J. (2016). Palindrome analyser - A new web-based server for predicting and evaluating inverted repeats in nucleotide sequences. Biochem. Biophys. Res. Commun. 478, 1739–1745. doi: 10.1016/j.bbrc.2016.09.015
Brázda, V., Laister, R., Jagelská, E., and Arrowsmith, C. (2011). Cruciform structures are a common DNA feature important for regulating biological processes. BMC Mol. Biol. 12:33. doi: 10.1186/1471-2199-12-33
Cao, D.-D., Li, L., and Chan, W.-Y. (2016). MicroRNAs: key regulators in the central nervous system and their implication in neurological diseases. Int. J. Mol. Sci. 17:E842. doi: 10.3390/ijms17060842
Carvalho, C., and Lupski, J. (2016). Mechanisms underlying structural variant formation in genomic disorders. Nat. Rev. Genet. 17, 224–238. doi: 10.1038/nrg.2015.25
Chawla, G., and Sokol, N. (2012). Hormonal activation of let-7-C microRNAs via EcR is required for adult Drosophila melanogaster morphology and function. Development. 139, 1788–1797. doi: 10.1242/dev.077743
Chen, D., Fu, L.-Y., Zhang, Z., Li, G., Zhang, H., Jiang, L., et al. (2014). Dissecting the chromatin interactome of microRNA genes. Nucleic Acids Res. 42, 3028–3043. doi: 10.1093/nar/gkt1294
Cordaux, R., Udit, S., Batzer, M., and Feschotte, C. (2006). Birth of a chimeric primate gene by capture of the transposase gene from a mobile element. Proc. Natl. Acad. Sci. U.S.A. 103, 8101–8106. doi: 10.1073/pnas.0601161103
Coufal, J., Jagelská, E., Liao, J., and Brázda, V. (2013). Preferential binding of p53 tumor suppressor to p21 promoter sites that contain inverted repeats capable of forming cruciform structure. Biochem. Biophys. Res. Commun. 441, 83–88. doi: 10.1016/j.bbrc.2013.10.015
Cridland, J., Thornton, K., and Long, A. (2015). Gene expression variation in Drosophila melanogaster due to rare transposable element insertion alleles of large effect. Genetics 199, 85–93. doi: 10.1534/genetics.114.170837
Cusco, I., Corominas, R., Bayés, M., Flores, R., Rivera-Brugués, N., Campuzano, V., et al. (2008). Copy number variation at the 7q11.23 segmental duplications is a susceptibility factor for the Williams-Beuren syndrome deletion. Genome Res. 18, 683–694. doi: 10.1101/gr.073197.107
Deniz, O., Flores, O., Battistini, F., Pérez, A., Soler-López, M., and Orozco, M. (2011). Physical properties of naked DNA influence nucleosome positioning and correlate with transcription start and termination sites in yeast. BMC Genomics 12:489. doi: 10.1186/1471-2164-12-489
Feng, Y., Huang, W., Meng, W., Jegga, A. G., Wang, Y., Cai, W., et al. (2014). Heat shock improves Sca-1+ stem cell survival and directs ischemic cardiomyocytes toward a prosurvival phenotype via exosomal transfer: a critical role for HSF1/miR-34a/HSP70 pathway. Stem Cells 32, 462–472. doi: 10.1002/stem.1571
Feschotte, C. (2008). Transposable elements and the evolution of regulatory networks. Nat. Rev. Genet. 9, 397–405. doi: 10.1038/nrg2337
Field, Y., Kaplan, N., Fondufe-Mittendorf, Y., Moore, I. K., Sharon, E., Lubling, Y., et al. (2008). Distinct modes of regulation by chromatin encoded through nucleosome positioning signals. PLoS Comput. Biol. 4:e1000216. doi: 10.1371/journal.pcbi.1000216
Funikov, S., Ryazansky, S., Kanapin, A., Logacheva, M., Penin, A., Snezhkina, A., et al. (2016). Interplay between RNA interference and heat shock response systems in Drosophila melanogaster. Open Biol. 6:E160224. doi: 10.1098/rsob.160224
Ghosh, T., Soni, K., Scaria, V., Halimani, M., Bhattacharjee, C., and Pillai, B. (2008). MicroRNA-mediated up-regulation of an alternatively polyadenylated variant of the mouse cytoplasmic {beta}-actin gene. Nucleic Acids Res. 36, 6318–6332. doi: 10.1093/nar/gkn624
Gil, E., Bosch, A., Lampe, D., Lizcano, J., Perales, J., Danos, O., et al. (2013). Functional characterization of the human mariner transposon Hsmar2. PLoS ONE 8:e73227. doi: 10.1371/journal.pone.0073227
Gouy, M., Guindon, S., and Gascuel, O. (2010). SeaView version 4: a multiplatform graphical user interface for sequence alignment and phylogenetic tree building. Mol. Biol. Evol. 27, 221–224. doi: 10.1093/molbev/msp259
Guex, N., and Peitsch, M. (1997). SWISS-MODEL and the Swiss-PdbViewer: an environment for comparative protein modeling. Electrophoresis 18, 2714–2723. doi: 10.1002/elps.1150181505
Hastings, P., Lupski, J., Rosenberg, S., and Ira, G. (2009). Mechanisms of change in gene copy number. Nat. Rev. Genet. 10, 551–564. doi: 10.1038/nrg2593
Hawley, R. (1980). Chromosomal sites necessary for normal levels of meiotic recombination in Drosophila melanogaster. I. Evidence for and mapping of the sites. Genetics 94, 625–646.
Hehir-Kwa, J. Y., Rodríguez-Santiago, B., Vissers, L. E., de Leeuw, N., Pfundt, R., Buitelaar, J. K., et al. (2011). De novo copy number variants associated with intellectual disability have a paternal origin and age bias. Am. J. Med. Genet. A 158A, 1493–1497. doi: 10.1136/jmedgenet-2011-100147
Heinemeyer, T., Wingender, E., Reuter, I., Hermjakob, H., Kel, A. E., Kel, O. V., et al. (1998). Databases on transcriptional regulation: TRANSFAC, TRRD and COMPEL. Nucleic Acids Res. 26, 362–367. doi: 10.1093/nar/26.1.362
Hirsch, E. (2006). How to judge animal models of Parkinson's disease in terms of neuroprotection. J. Neural Transm. Suppl. 70, 255–260. doi: 10.1007/978-3-211-45295-0_39
Hoogenraad, C., Akhmanova, A., Galjart, N., and De Zeeuw, C. (2004). LIMK1 and CLIP-115: linking cytoskeletal defects to Williams syndrome. Bioessays 26, 141–150. doi: 10.1002/bies.10402
Humphrey, W., Dalke, A., and Schulten, K. (1996). VMD: visual molecular dynamics. J. Mol. Graph. 14, 33–38, 27–28. doi: 10.1016/0263-7855(96)00018-5
Ibáñez-Ventoso, C., Vora, M., and Driscoll, M. (2008). Sequence relationships among C. elegans, D. melanogaster and human microRNAs highlight the extensive conservation of microRNAs in biology. PLoS ONE 3:e2818. doi: 10.1371/journal.pone.0002818
Inagaki, H., Ohye, T., Kogo, H., Kato, T., Bolor, H., Taniguchi, M., et al. (2009). Chromosomal instability mediated by non-B DNA: cruciform conformation and not DNA sequence is responsible for recurrent translocation in humans. Genome Res. 19, 191–198. doi: 10.1101/gr.079244.108
Johnson, R., Noble, W., Tartaglia, G. G., and Buckley, N. (2012). Neurodegeneration as an RNA disorder. Prog. Neurobiol. 99, 293–315. doi: 10.1016/j.pneurobio.2012.09.006
Kaminskaya, A., Nikitina, E., Medvedeva, A., Gerasimenko, M., Chernikova, D., and Savateeva-Popova, E. (2015). Influence of limk1 gene polymorphism on learning acquisition and memory formation with pCREB distribution and aggregate formation in neuromuscular junctions in Drosophila melanogaster. Genetika 51, 685–693. doi: 10.1134/S1022795415060071
Kaminskaya, A., Nikitina, E., Payalina, T., Molotkov, D., Zakharov, G., Popov, A., et al. (2012). Effect of the LIM kinase 1 isoform ratio on Drosophila melanogaster courtship behavior: a complex approach. Russ. J. Genet. Appl. Res. 2, 367–377. doi: 10.1134/S2079059712050024
Kertesz, M., Iovino, N., Unnerstall, U., Gaul, U., and Segal, E. (2007). The role of site accessibility in microRNA target recognition. Nat. Genet. 39, 1278–1284. doi: 10.1038/ng2135
Kim, Y.-J., and Kim, D.-N. (2016). Structural basis for elastic mechanical properties of the DNA double helix. PLoS ONE 11:e0153228. doi: 10.1371/journal.pone.0153228
Kirov, G., Pocklington, A. J., Holmans, P., Ivanov, D., Ikeda, M., Ruderfer, D., et al. (2012). De novo CNV analysis implicates specific abnormalities of postsynaptic signalling complexes in the pathogenesis of schizophrenia. Mol. Psychiatry 17, 142–153. doi: 10.1038/mp.2011.154
Kosikov, K. (1936). A new duplication in the Drosophila melanogaster X chromosome and its evolutionary significance. Dokl. Akad. Nauk SSSR 3, 297–300.
Kozomara, A., and Griffiths-Jones, S. (2014). miRBase: annotating high confidence microRNAs using deep sequencing data. Nucleic Acids Res. 42, D68–D73. doi: 10.1093/nar/gkt1181
Kucherenko, M., and Shcherbata, H. (2013). Steroids as external temporal codes act via microRNAs and cooperate with cytokines in differential neurogenesis. Fly 7, 173–183. doi: 10.4161/fly.25241
Kumar, D. (2008). Disorders of the genome architecture: a review. Genomic Med. 2, 69–76. doi: 10.1007/s11568-009-9028-2
Li, G., Ruan, X., Auerbach, R., Sandhu, K. S., Zheng, M., Wang, P., et al. (2012). Extensive promoter-centered chromatin interactions provide a topological basis for transcription regulation. Cell 148, 84–98. doi: 10.1016/j.cell.2011.12.014
Lushnikov, S., Dmitriev, A., Fedoseev, A., Zakharov, G., Zhuravlev, A., Medvedeva, A., et al. (2014). Low-frequency dynamics of DNA in Brillouin light scattering spectra. JETP Lett. 98, 735–741. doi: 10.1134/S0021364013240168
Maciotta, S., Meregalli, M., and Torrente, Y. (2013). The involvement of microRNAs in neurodegenerative diseases. Front. Cell Neurosci. 7:0265. doi: 10.3389/fncel.2013.00265
Marinescu, V., Kohane, I., and Riva, A. (2005). MAPPER: a search engine for the computational identification of putative transcription factor binding sites in multiple genomes. BMC Bioinformatics 6:79. doi: 10.1186/1471-2105-6-79
Mattick, J. (2011). The central role of RNA in human development and cognition. FEBS Lett. 585, 1600–1616. doi: 10.1016/j.febslet.2011.05.001
Medrano-Fernández, A., and Barco, A. (2016). Nuclear organization and 3D chromatin architecture in cognition and neuropsychiatric disorders. Mol. Brain 9:E83. doi: 10.1186/s13041-016-0263-x
Medvedeva, A., Molotkov, D., Nikitina, E., Popov, A., Karagodin, D., Baricheva, E., et al. (2008). Systemic regulation of genetic and cytogenetic processes by a signal cascade of actin remodeling: locus agnostic in Drosophila. Russ. J. Genet. 44, 669–681. doi: 10.1134/S1022795408060069
Medvedeva, A., and Savvateeva, E. (1991). The effects of the agnostic gene ts-mutations that control calmodulin functions and learning ability on ectopic pairing of Drosophila polytene chromosomes. Dokl. Akad. Nauk SSSR 318, 733–736.
Meng, Y., Zhang, Y., Tregoubov, V., Janus, C., Cruz, L., Jackson, M., et al. (2002). Abnormal spine morphology and enhanced LTP in LIMK-1 knockout mice. Neuron 35, 121–133. doi: 10.1016/S0896-6273(02)00758-4
Mills, R., Luttig, C., Larkins, C., Beauchamp, A., Tsui, C., Pittard, S., et al. (2006). An initial map of insertion and deletion (INDEL) variation in the human genome. Genome Res. 16, 1182–1190. doi: 10.1101/gr.4565806
Morris, K., and Mattick, J. (2014). The rise of regulatory RNA. Nat. Rev. Genet. 15, 423–437. doi: 10.1038/nrg3722
Mullaney, J., Mills, R., Pittard, S., and Devine, S. (2010). Small insertions and deletions (INDELs) in human genomes. Hum. Mol. Genet. 19, R131–R136. doi: 10.1093/hmg/ddq400
Nehammer, C., Podolska, A., Mackowiak, S. D., Kagias, K., and Pocock, R. (2015). Specific microRNAs regulate heat stress responses in Caenorhabditis elegans. Sci. Rep. 5:8866. doi: 10.1038/srep08866
Nikitina, E., Medvedeva, A., Zakharov, G., and Savvateeva-Popova, E. (2014a). The Drosophila agnostic Locus: involvement in the formation of cognitive defects in williams syndrome. Acta Nat. 6, 53–61.
Nikitina, E., Medvedeva, A., Zakharov, G., and Savvateeva-Popova, E. (2014b). Williams syndrome as a model for elucidation of the pathway genes - the brain - cognitive functions: genetics and epigenetics. Acta Nat. 6, 9–22.
Ohnishi, O. (1977). Spontaneous and ethyl methanesulfonate-induced mutations controlling viability in Drosophila melanogaster. II. Homozygous effect of polygenic mutations. Genetics 87, 529–545.
Ostertag, E., and Kazazian, H. (2001). Biology of mammalian L1 retrotransposons. Annu. Rev. Genet. 35, 501–538. doi: 10.1146/annurev.genet.35.102401.091032
Pérez Jurado, L. A. (2003). Williams–Beuren syndrome: a model of recurrent genomic mutation. Horm. Res. 59, 106–113.
Plank, J., and Dean, A. (2014). Enhancer function: mechanistic and genome-wide insights come together. Mol. Cell. 55, 5–14. doi: 10.1016/j.molcel.2014.06.015
Qiu, L., LeBel, R., Storm, D., and Chen, X. (2016). Type 3 adenylyl cyclase: a key enzyme mediating the cAMP signaling in neuronal cilia. Int. J. Physiol. Pathophysiol. Pharmacol. 8, 95–108.
Quinlan, A., and Hall, I. (2010). BEDTools: a flexible suite of utilities for comparing genomic features. Bioinformatics 26, 841–842. doi: 10.1093/bioinformatics/btq033
Reiter, L., Liehr, T., Rautenstrauss, B., Robertson, H., and Lupski, J. (1999). Localization of mariner DNA transposons in the human genome by PRINS. Genome Res. 9, 839–843. doi: 10.1101/gr.9.9.839
Robinson, M., McCarthy, D., and Smyth, G. (2010). edgeR: a Bioconductor package for differential expression analysis of digital gene expression data. Bioinformatics 26, 139–140. doi: 10.1093/bioinformatics/btp616
Rokavec, M., Li, H., Jiang, L., and Hermeking, H. (2014). The p53/miR-34 axis in development and disease. J. Mol. Cell Biol. 6, 214–230. doi: 10.1093/jmcb/mju003
Ruby, G., Stark, A., Johnston, W., Kellis, M., Bartel, D., and Lai, E. (2007). Evolution, biogenesis, expression, and target predictions of a substantially expanded set of Drosophila microRNAs. Genome Res. 17, 1850–1864. doi: 10.1101/gr.6597907
Sandelin, A., Wasserman, W., and Lenhard, B. (2004). ConSite: web-based prediction of regulatory elements using cross-species comparison. Nucleic Acids Res. 32, W249–W252. doi: 10.1093/nar/gkh372
Savvateeva, E., and Kamyshev, N. (1981). Behavioral effects of temperature sensitive mutations affecting metabolism of cAMP in D. melanogaster. Pharm. Biochem. Behav. 14, 603–611. doi: 10.1016/0091-3057(81)90119-2
Savvateeva-Popova, E., Peresleni, A., Scharagina, L., Medvedeva, A., Korochkina, S., Grigorieva, I., et al. (2004). Architecture of the X chromosome, expression of LIM Kinase 1, and recombination in the agnostic mutants of Drosophila: a model for human williams syndrome. Russ. J. Genet. 40, 605–624. doi: 10.1023/B:RUGE.0000033308.97306.9a
Savvateeva-Popova, E., Peresleny, A., Scharagina, L., Tokmacheva, E., Medvedeva, A., Kamyshev, N., et al. (2002). Complex study of Drosophila mutants in the agnostic locus: a model for coupling chromosomal architecture and cognitive functions. J. Evol. Biochem. Physiol. 38, 706–733. doi: 10.1023/A:1023003625014
Savvateeva-Popova, E., Popov, A., Grossman, A., Nikitina, E., Medvedeva, A., Molotkov, D., et al. (2008). Non-coding RNA as a trigger of neuropathologic disorder phenotypes in transgenic Drosophila. J. Neural Transm. 115, 1629–1642. doi: 10.1007/s00702-008-0078-8
Schwede, T., Kopp, J., Guex, N., and Peitsch, M. (2003). SWISS-MODEL: an automated protein homology-modeling server. Nucleic Acids Res. 31, 3381–3385. doi: 10.1093/nar/gkg520
Tassabehji, M., Metcalfe, K., Karmiloff-Smith, A., Carette, M. J., Grant, J., Dennis, N., et al. (1999) Williams syndrome: use of chromosomal microdeletions as a tool to dissect cognitive physical phenotypes. Am. J. Hum. Genet. 64, 118–125. doi: 10.1086/302214
Valero, M. C., de Luis, O., Cruces, J., and Pérez Jurado, L. A. (2000). Fine-scale comparative mapping of the human 7q11.23 region and the orthologous region on mouse chromosome 5G: the low-copy repeats that flank the Williams-Beuren syndrome deletion arose at breakpoint sites of an evolutionary inversion(s). Genomics 69, 1–13. doi: 10.1006/geno.2000.6312
Venderova, K., Kabbach, G., Abdel-Messih, E., Zhang, Y., Parks, R., Imai, Y., et al. (2009). Leucine-Rich Repeat Kinase 2 interacts with Parkin, DJ-1 and PINK-1 in a Drosophila melanogaster model of Parkinson's disease. Hum. Mol. Genet. 18, 4390–4404. doi: 10.1093/hmg/ddp394
Wells, R. (2007). Non-B DNA conformations, mutagenesis and disease. Trends Biochem. Sci. 32, 271–278. doi: 10.1016/j.tibs.2007.04.003
Wells, R. (2009). Discovery of the role of Non-B DNA structures in mutagenesis and human genomic disorders. J. Biol. Chem. 284, 8997–9009. doi: 10.1074/jbc.X800010200
Xamena, N., Creus, A., and Macros, R. (1985). Effect of intercalating mutagens on crossing over in Drosophila melanogaster females. Experientia 41, 1078–1081. doi: 10.1007/BF01952152
Xi, L., Fondufe-Mittendorf, Y., Xia, L., Flatow, J., Widom, J., and Wang, J.-P. (2010). Predicting nucleosome positioning using a duration Hidden Markov Model. BMC Bioinformatics 11:346. doi: 10.1186/1471-2105-11-346ss
Zhang, Y., Wong, C.-H., Birnbaum, R., Li, G., Favaro, R., Ngan, C. Y., et al. (2013). Chromatin connectivity maps reveal dynamic promoter–enhancer long-range associations. Nature 504, 306–310. doi: 10.1038/nature12716
Keywords: Drosophila, LIM-kinase 1, microRNA, nucleosome formation probability, transposable elements, non-canonical DNA structures
Citation: Savvateeva-Popova EV, Zhuravlev AV, Brázda V, Zakharov GA, Kaminskaya AN, Medvedeva AV, Nikitina EA, Tokmatcheva EV, Dolgaya JF, Kulikova DA, Zatsepina OG, Funikov SY, Ryazansky SS and Evgen‘ev MB (2017) Drosophila Model for the Analysis of Genesis of LIM-kinase 1-Dependent Williams-Beuren Syndrome Cognitive Phenotypes: INDELs, Transposable Elements of the Tc1/Mariner Superfamily and MicroRNAs. Front. Genet. 8:123. doi: 10.3389/fgene.2017.00123
Received: 06 July 2017; Accepted: 04 September 2017;
Published: 20 September 2017.
Edited by:
Mehdi Pirooznia, National Heart Lung and Blood Institute (NIH), United StatesReviewed by:
Federica Calore, The Ohio State University Columbus, United StatesOwen Murray Rennert, National Institute of Child Health and Human Development, National Institutes of Health, United States
Copyright © 2017 Savvateeva-Popova, Zhuravlev, Brázda, Zakharov, Kaminskaya, Medvedeva, Nikitina, Tokmatcheva, Dolgaya, Kulikova, Zatsepina, Funikov, Ryazansky and Evgen‘ev. This is an open-access article distributed under the terms of the Creative Commons Attribution License (CC BY). The use, distribution or reproduction in other forums is permitted, provided the original author(s) or licensor are credited and that the original publication in this journal is cited, in accordance with accepted academic practice. No use, distribution or reproduction is permitted which does not comply with these terms.
*Correspondence: Elena V. Savvateeva-Popova, ZXNhdnZhdGVldmFAbWFpbC5ydQ==