- 1Department of Biology and Ted R. Bradley Herbarium, George Mason University, Fairfax, VA, USA
- 2Department of Ecology and Evolutionary Biology, Brown University, Providence, RI, USA
- 3United States Botanical Garden, Washington, DC, USA
- 4Institute of Systematic Botany, The New York Botanical Garden, Bronx, NY, USA
- 5Department of Integrative Biology and Jepson and University Herbaria, University of California, Berkeley, CA, USA
Many angiosperm families are distributed pantropically, yet for any given continent little is known about which lineages are ancient residents or recent arrivals. Here we use a comprehensive sampling of the pantropical sister pair Anacardiaceae and Burseraceae to assess the relative importance of continental vicariance, long-distance dispersal and niche-conservatism in generating its distinctive pattern of diversity over time. Each family has approximately the same number of species and identical stem age, yet Anacardiaceae display a broader range of fruit morphologies and dispersal strategies and include species that can withstand freezing temperatures, whereas Burseraceae do not. We found that nuclear and chloroplast data yielded a highly supported phylogenetic reconstruction that supports current taxonomic concepts and time-calibrated biogeographic reconstructions that are broadly congruent with the fossil record. We conclude that the most recent common ancestor of these families was widespread and likely distributed in the Northern Hemisphere during the Cretaceous and that vicariance between Eastern and Western Hemispheres coincided with the initial divergence of the families. The tempo of diversification of the families is strikingly different. Anacardiaceae steadily accumulated lineages starting in the Late Cretaceous–Paleocene while the majority of Burseraceae diversification occurred in the Miocene. Multiple dispersal- and vicariance-based intercontinental colonization events are inferred for both families throughout the past 100 million years. However, Anacardiaceae have shifted climatic niches frequently during this time, while Burseraceae have experienced very few shifts between dry and wet climates and only in the tropics. Thus, we conclude that both Anacardiaceae and Burseraceae move easily but that Anacardiaceae have adapted more often, either due to more varied selective pressures or greater intrinsic lability.
Introduction
A richer understanding of factors underlying the origin of the diverse tropical flora depends on aggregating global biogeographic, taxonomic and phylogenetic information from multiple plant clades. Although much progress has been made reconstructing the tempo and genealogical patterns of angiosperm evolution in the past two decades, most of the major plant lineages lack the comprehensive global sampling and taxonomic studies necessary to address basic questions regarding the timing of radiations, geographic origins, and dispersal histories. Many angiosperm families are common to tropical forests around the world, yet very little is known about which lineages are ancient and have experienced multiple vicariance events, and which lineages have experienced more recent long-distance dispersal. Moreover, the timing and directionality of such dispersal events is often unknown, although recent studies have found that many tropical radiations appear to have coincided with climatic and geographic events, such as Oligocene–Miocene cooling and drying in both the Americas and Africa, the connection of North and South America, and the Andean uplift (Bouchenak-Khelladi et al., 2010; Hoorn et al., 2010; De-Nova et al., 2012; Hughes et al., 2013).
Recent studies have highlighted the importance of phylogenetic niche conservatism in understanding large-scale patterns of plant biogeography and evolution (Wiens and Donoghue, 2004; Donoghue, 2008). The hypothesis is that because adaptation to new climatic zones requires a complex array of morphological and physiological innovations, as long as dispersal is possible, in situ adaptation by the resident flora during periods of global cooling or drying may often be less common than immigration of other lineages that are pre-adapted to freezing or dry climates. For example, many Northern American temperate plant lineages have colonized the high-elevation South American Andes since overland connections were established between the two continents (Bell and Donoghue, 2005; Hughes and Eastwood, 2006). However, South American lineages have also colonized these freezing habitats, even from warm tropical lowlands (Donoghue, 2008), demonstrating that adaptation to new biomes, as well as immigration from other areas, can result in diversification within regions (Donoghue, 2008; Donoghue and Edwards, 2014).
The angiosperm lineages Anacardiaceae and Burseraceae (Sapindales) represent an excellent study system for investigating the biogeographic history of tropical diversification and the relative importance of movement and climatic adaptation in angiosperm evolution. Once recognized as the single taxon “Terebinthaceae” (Marchand, 1869) and subsequently shown to be sister taxa (Fernando et al., 1995; Gadek et al., 1996; Bremer et al., 1999; Savolainen et al., 2000a,b; Pell, 2004), these families collectively comprise ca. 1500 lianas, shrubs and trees distributed on every continent except Antarctica and are major elements of the structure and diversity of temperate, seasonally dry tropical forest and tropical wet forest floras (Gentry, 1988; Pennington et al., 2010) (Figure 1). Anacardiaceae species display a remarkable range of fruit morphologies and seed dispersal syndromes not present in Burseraceae (see Daly et al., 2011; Pell et al., 2011). This disparity is primarily responsible for the recognition of more genera in Anacardiaceae (82) as compared to Burseraceae (19), although each family has approximately the same number of species. However, the geographic range as well as the morphological and ecological diversity of Anacardiaceae considerably eclipses that of Burseraceae, which makes the Terebinthaceae lineage a valuable comparative model system for testing the relative contributions to diversification of climate adaptation and intercontinental movement.
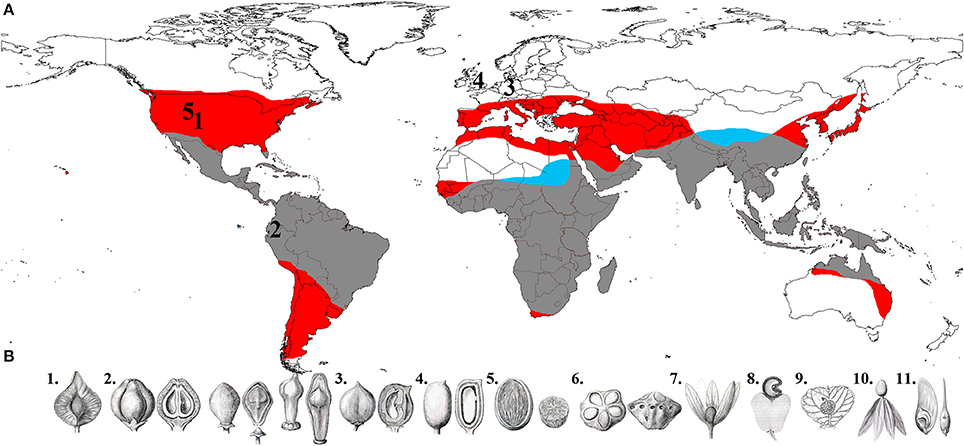
Figure 1. (A) Global distribution of Anacardiaceae and Burseraceae (red area is Anacardiaceae only, blue is Burseraceae only, and gray is where the two families' distributions overlap), and fossils used to calibrate the phylogenies in this study (1, Oligocene/Late Eocene Cotinus leaf; 2, Middle Miocene Loxopterygium fruit; 3, Middle Eocene Anacardium fruit; 4, Middle Eocene Bursericarpum aldwickense and Protocommiphora europea fruits; 5, Middle Eocene Bursera subgenus Elaphrium leaf). (B) Fruit diversity in Anacardiaceae and Burseraceae (1, Triomma pseudocapsule; 2, Protium, Bursera and Boswellia nuculaniums; 3, Garuga drupe; 4, Solenocarpus drupe; 5, Spondias, internally operculate drupe; 6, Dracontomelon, externally operculate drupe; 7, Loxostylis, drupe subtended by wing-like calyx; 8, Anacardium, drupe subtended by fleshy hypocarp; 9, Campylopetalum, drupe subtended by an accrescent bract; 10, Swintonia, drupe subtended by wing-like corolla; 11, Schinopsis, samara.) (illustrations 1–7 and 10–11 from Engler, 1883; 8 from Faguet, 1874/1875; 9 copyright Bobbi Angell).
The differences between the families raise the question of how sister lineages having identical ages and nearly equivalent numbers of species could have taken such different evolutionary trajectories whilst becoming so widespread. Anacardiaceae comprise a cosmopolitan group, are found at a greater range of latitudes and elevations, and a broader range of habitats than Burseraceae. All Burseraceae, by contrast, are intolerant of frost and are thus limited to lower elevation zones in the African, American, Asian and Pacific (sub-)tropics.
Testing which events may have been correlated with cladogenesis in Terebinthaceae is contingent on reconstructing a densely sampled, time-calibrated phylogeny for the group. Divergence time estimates are a critical component of testing historical biogeographic hypotheses for Terebinthaceae because routes of overland range expansion available to Anacardiaceae and Burseraceae may have been different as global climate fluctuated over geological time. For example, ancestral lineages of frost tolerant Anacardiaceae may have been able to disperse through regions located above the frost line during the Miocene (e.g., Rhus, Yi et al., 2004) whereas the entirely frost-intolerant Burseraceae may have been excluded from them. Historically, angiosperm biogeographers have hypothesized that cosmopolitan and pantropical groups experienced vicariance as a consequence of the break-up of Gondwana (Raven and Axelrod, 1974). Both Anacardiaceae and Burseraceae had been considered Gondwanan families in the past (Raven and Axelrod, 1974; Gentry, 1982). More recently, divergence time estimates for a number of angiosperm lineages having predominantly frost-free distributions, including Burseraceae, have indicated that the northern hemisphere land corridors have had a more influential role in establishing species' ranges than previously hypothesized (Chanderbali et al., 2001; Renner et al., 2001; Davis et al., 2004; Richardson et al., 2004; Weeks et al., 2005; Zerega et al., 2005). In the case of Burseraceae, Weeks et al. (2005) implicated a North American origin of the family followed by Paleocene migration of lineages eastward over the warm North Atlantic land bridge and along the Tethys Seaway to Southeast Asia, as well as early trans-oceanic dispersals to Africa and South America. However, this study did not optimize the ancestral distributions using a geographically diverse group of Anacardiaceae outgroup taxa nor did it incorporate known Anacardiaceae fossils as calibration points.
Contemporary studies have not clarified the relative contribution of vicariance and dispersal in generating the current distribution of both Anacardiaceae and Burseraceae and whether shifts in climatic niches may have promoted their diversification. Here, we address three sets of questions regarding the evolution of Terebinthaceae:
(i) What is the timing of diversification of Terebinthaceae? Have diversification rates varied through time?
(ii) What is the evolutionary history of geographic range in Terebinthaceae? Have lineages persisted in unique geographic regions or have they dispersed to new geographic regions (i.e., how common is dispersal)?
(iii) What is the evolutionary history of climatic niche (or climatic preference) in Terebinthaceae? Have lineages retained distinct climatic niches or have evolved climatic tolerances (i.e., how common is “niche expansion”)?
Materials and Methods
Taxon Sampling
Ingroup species from Anacardiaceae and Burseraceae were chosen on the basis of assembling the most complete biogeographic coverage possible from all major lineages. Species were selected with reference to preexisting phylogenies (Pell, 2004; Weeks et al., 2005; Fine et al., 2014) as well as to recent taxonomic literature that recognizes the new segregate genus Poupartiopsis (Mitchell et al., 2006) and the newly circumscribed genera Searsia and Protorhus (Moffett, 2007; Pell et al., 2008; Table 1). From Anacardiaceae we obtained 67 of 82 genera (169 species) and sampling was spread across the higher ranks of recently published classifications: (1) the five tribes sensu Mitchell and Mori (1987) as updated by Pell (2004), including Anacardieae (7/8 genera sampled/genera total), Dobineae (2/2), Rhoeae (39/47), Semecarpeae (3/5), Spondiadeae (16/20); and (2) Pell's and Mitchell's (Mitchell et al., 2006) modifications to Takhtajan's (1987) subfamilial system, including Spondioideae (16/20) and Anacardioideae (50/60). From Burseraceae, we obtained 16 of 19 genera (136 spp.) from the five taxonomic alliances sensu Daly et al. (2011): the Beiselia alliance (1/1 genus), the Protium alliance (3/3 genera), the Boswellia alliance (2/2 genera), the Bursera alliance (3/3 genera) and the Canarium alliance (7/10 genera). Burseraceae includes several disjunct genera (Canarium, Commiphora, Dacryodes, Protium) whose species are distributed in African, American, Asian, and Pacific regions, and care was taken to sample representatives from each. Outgroup taxa from Sapindales were chosen with reference to Gadek et al. (1996) and comprise species of Meliaceae (1 sp.; Muellner et al., 2006), Rutaceae (5 spp.; Groppo et al., 2008), and Sapindaceae incl. Aceraceae (15 spp.; Harrington et al., 2005).
Marker Selection and Sequence Alignment
Sequence data for assessing the individual phylogenies of Anacardiaceae and Burseraceae have been generated by current authors using multiple phylogenetic markers (Weeks, 2003; Pell, 2004; Fine et al., 2005, 2014; Weeks et al., 2005; Pell et al., 2008). The published datasets overlapped for three DNA sequence regions: the nuclear ribosomal external transcribed spacer (ETS), the chloroplast trnL intron and trnL-F intergenic spacer (trnL-F region), and the chloroplast rps16 intron. All of these regions have proven alignable across the targeted taxa and useful for investigating phylogeny at the familial and generic levels. These three datasets were expanded with additional taxa for the current study using amplification and sequencing protocols as outlined in publications referenced above. Multiple sequence alignment for each locus was carried out in MAFFT v7.0 (Katoh and Standley, 2013) with the E-INS-i algorithm. To improve alignment quality, we ran GBlocks V0.91b (Castresana, 2000) with parameters −b3 = 4, −b4 = 10, −b5 = h to clean the alignments as this has been shown to improve subsequent phylogenetic analyses (Talavera and Castresana, 2007). Before phylogenetic inference, we evaluated whether the final concatenated matrix should be partitioned by marker or by any combination of markers, and which nucleotide substitution model should be employed for the final partition scheme. For this analysis, we used the Bayesian Information Criterion as implemented in PartitionFinder (Lanfear et al., 2012) using the greedy algorithm, and we unlinked branch length estimates for each of the substitution models in each partition. Results of this analysis showed that the matrix should be treated as a single partition evolving under the GTR+I+Gamma model of nucleotide substitution.
Sources of Fossil Calibration Points
Both families have rich micro- and macro-fossil records with which to calibrate their phylogeny and test hypotheses of historical biogeographical evolution in Terebinthaceae. The three Anacardiaceae fossils chosen for calibration are classified within extant lineages: (1) an early Oligocene/Late Eocene Cotinus leaf fossil from the Florissant flora, Colorado, United States (34 Ma; MacGinitie, 1953); (2) a Middle Miocene Loxopterygium fruit fossil from the Ecuadorian Andes (10 Ma; Burnham and Carranco, 2004); and (3) a Middle Eocene Anacardium fruit fossil from Germany (47 Ma; Manchester et al., 2007). Ages of all Anacardiaceae fossils included herein are associated with sediments that have been dated radiometrically. Three Burseraceae fossils were selected. Two fossils are from the London Clay and are Early Eocene fruit casts of Bursericarpum aldwickense Chandler, which is assignable to extant Protieae on the basis of the number of pyrenes per fruit (Chandler, 1961; Harley and Daly, 1995), and Protocommiphora europea Reid and Chandler, which is similar to extant Commiphora (Reid and Chandler, 1933; Collinson, 1983). The age of these fossils is estimated as 48.6 Ma, the age of the lowest stratum of the Middle Eocene (Lutetian) and the upper-most bound of the Early Eocene (Ypresian). The remaining Burseraceae fossil is a leaf impression ascribed to Bursera subgenus Elaphrium from the Green River Flora of Colorado and Utah (MacGinitie, 1969; Plate 30, Figure 2), whose base has a radiometrically-determined age of 49.7–50.7 Ma (Clyde et al., 1995). Dates for all geological periods and epochs follow those of the International Commission on Stratigraphy.
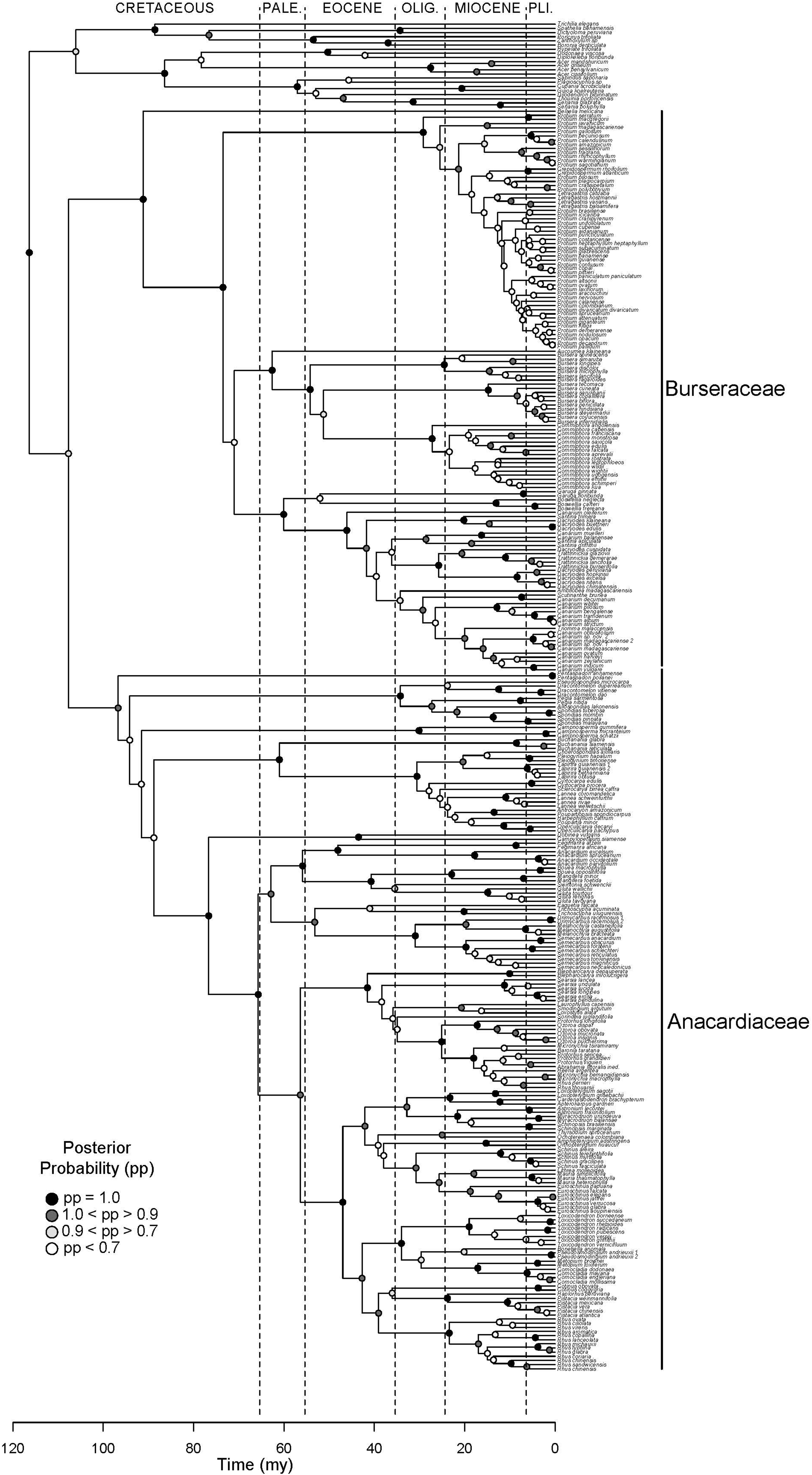
Figure 2. Molecular phylogeny of Terebinthaceae. Maximum clade credibility tree (MCCT) summarizing results of Bayesian dating analysis. Families Anacardiaceae and Burseraceae indicated to the right of the clades. Posterior probabilities for branches are shown on descendant nodes.
Phylogenetic Dating and Diversification Analyses
The chronogram and divergence times were co-estimated using Markov Chain Monte Carlo (MC2) sampling in BEAST v1.8 (Drummond et al., 2012). A birth-death speciation process (Gernhard, 2008) was specified as a tree prior with a death rate parameter sampled from a U(0,1) prior distribution, and a growth rate parameter sampled from a U(0,inf) prior distribution. Rate heterogeneity among lineages was modeled using an uncorrelated lognormal relaxed molecular clock (Drummond et al., 2006) with a mean sampled from an Exp(10) prior distribution. We used a secondary calibration to set the prior on the age of the root using a N(85,8) prior distribution; this parameterization accounts for the uncertainty surrounding the age of the Sapindales (Muellner et al., 2006; Magallón and Castillo, 2009). We used the six Terebinthaceae fossils (see above) to set priors on six nodes: the most recent common ancestor (MRCA) of Cotinus, the MRCA of Loxopterygium, the MRCA of Anacardium, the MRCA of the Protieae, the MRCA of Commiphora, and the MRCA of Bursera subgenus Elaphrium. Because all of these fossils are fragmentary, it is not possible to be certain that any of those fossils possess features that would place them in the crown groups. Therefore, we took a conservative approach and used them as minimum calibrations of the stem groups (Forest, 2009). All these nodes were parameterized with Exponential distributions in which the offset matched the minimum bound set by the fossil age, and the mean was set to be 10% older than this value. Because random starting trees did not satisfy the temporal and topological constraints associated with some fossil calibrations, we used ExaML v1.0.12 (Stamatakis and Aberer, 2013) to estimate a maximum likelihood tree, transformed it into a chronogram using penalized likelihood (Sanderson, 2002; Paradis, 2013), and used it as starting topology in BEAST. The MC2 was run for 6 × 107 generations sampling every 4 × 103 with the first 20% of the samples discarded as burn-in. Convergence to stationarity of the MC2 sampling was determined with time-series plots of the likelihood scores and cumulative split frequencies, and assessing that estimated effective sample sizes for the chronograms and model parameters were at least 100. Post burn-in chronograms were summarized with a majority clade credibility tree (MCCT) using median branch lengths.
We carried out diversification analyses in two ways. First, we used BayesRate (Silvestro et al., 2011) to evaluate whether a single birth-death diversification process for the whole Terebinthaceae, or two birth-death diversification processes, one for Anacardiaceae and one for Burseraceae, better explain the accumulation of lineages through time. For this analysis, we used flat priors, clade-specific taxon sampling proportions (PAnacardiaceae = 0.21, PBurseraceae = 0.19), we unlinked rates between clades, and ran the MC2 for 1 × 105 generations, sampling every 1 × 102, and discarding the first 10% as burn-in. For model selection, we used Bayes Factors using the marginal likelihoods calculated using thermodynamic integration. Second, we used BAMM (Rabosky, 2014) to automatically detect shifts in diversification process through time without defining tree partitions a priori. For this analysis, we used 1.0 for the Poisson rate prior, the lambda initial prior, and the extinction rate prior. We included a global taxon sampling proportion P = 0.20. We ran 1 × 107 generations of MC2, sampling every 1 × 103, and discarding the first 10% as burn-in, with two independent runs to assess convergence.
Geographic Range and Climatic Niche Evolution Analyses
To study geographic range evolution through time, we employed maximum-likelihood inference of geographic range evolution using the dispersal, extinction, and cladogenesis (DEC) model (Ree et al., 2005; Ree and Smith, 2008) implemented in Lagrange version 0.1β, and estimated split and ancestral states concurrently. We described the geographic distributions of each Anacardiaceae and Burseraceae taxon following the biogeographic divisions of Good (1974) and Olson and Carlquist (2001) with some modifications. We assigned each species to one or more of the following seven areas: NA: North America (including Central America and the Caribbean); SA: South America; EA: Eurasia (including North Africa/Mediterranean/Arabian Peninsula); SSA: sub-Saharan Africa; MAD: Madagascar, SeA: Southeast Asia (including India); and OC: Oceania (including Papua New Guinea/Tropical Australia/New Caledonia, and Tropical Pacific Islands). We ran a single DEC unconstrained model assuming rates of dispersal/expansion and extinction were uniform across the areas in the model and across the phylogeny. We estimated D, the dispersal/expansion rate across the phylogeny (Ree and Smith, 2008) for each family, by running two more DEC analyses, one for each family.
To study climatic niche evolution through time, we carried out a second DEC analysis. Although DEC was initially designed for modeling geographic range evolution, it provides a sound framework for modeling the evolution of other type of characters (Ree and Smith, 2008), in particular climatic niche. We found two significant benefits of DEC over alternative approaches for modeling the evolution of climatic niche. First, broad climatic niches encompassing two or more unique climatic niches are valid states for single species; many species in nature display broad climatic tolerances. Second, we reasoned that, analogous to geographic range, for an ancestor with a broad climatic niche, climatic adaptation and lineage divergence can result in daughter species inheriting mutually exclusive climatic niches, or one daughter species inheriting one climatic niche, while the other (the remainder of the ancestral lineage) inheriting the ancestral climatic niche (for details and further discussion, see Ree et al., 2005; Ree and Smith, 2008). For this analysis we assigned each species to one or more of the following climatic categories: Temperate (frost-tolerant), Tropical Seasonal Dry Forest/Savannah/Scrubland, and Tropical Moist/Wet Forest. Taxa were assigned to these regions on the basis of the authors' knowledge of the taxa and published sources (Daly et al., 2011; Pell et al., 2011). We ran a single DEC unconstrained model assuming rates of dispersal/expansion and extinction were uniform across the climatic niches in the model and across the phylogeny. We estimated D, the dispersal/expansion rate across the phylogeny (Ree and Smith, 2008), for each family by running two more DEC analyses, one for each family.
Results
Genbank accession information for all taxa is listed in Table 1. Alignments, analyses and trees generated by the study are posted to Treebase, study number 16073 (www.treebase.org).
Phylogenetic Relationships
Results support the monophyly of Anacardiaceae and Burseraceae individually and their relationship as sister clades (Figure 2). Within Anacardiaceae, phylogenetic results pose challenges to all published subfamilial classifications of the family (Figure 3). The most widely used classification of the family includes five tribes, Anacardieae, Dobineae, Rhoeae, Semecarpeae, Spondiadeae, (Engler, 1881, 1883, 1892; modified by Mitchell and Mori, 1987; and again by Pell, 2004), only two of which are monophyletic as circumscribed, Dobineae (Campylopetalum and Dobinea) and Semecarpeae (represented here by Drimycarpus, Melanochyla and Semecarpus, but also including Holigarna and Nothopegia). Pell and Mitchell (Mitchell et al., 2006) proposed the most recent classification, which includes two subfamilies, Anacardioideae and Spondioideae, both of which are shown here to be polyphyletic. Our results do provide some clarity for the position of three genera that have been difficult to place within the evolutionary context of the family: Buchanania, Campnosperma, and Pentaspadon. Although it is most often treated as a member of tribe/subfamily Anacardieae/Anacardioideae, Buchanania is here resolved sister to a clade of taxa traditionally recognized within tribe/subfamily Spondiadeae/Spondioideae. Campnosperma and Pentaspadon remain challenging but are resolved as sister lineages to much larger clades: Pentaspadon is sister to the rest of Anacardiaceae and Campnosperma is sister to a clade that includes all of subfamily Anacardioideae included in our sampling (excluding Pentaspadon from the Mitchell et al. (2006) circumscription; Buchanania + clade of Dobinea to Rhus chinensis Mill.) and most of Spondioideae (clade containing Choerospondias to Operculicarya).
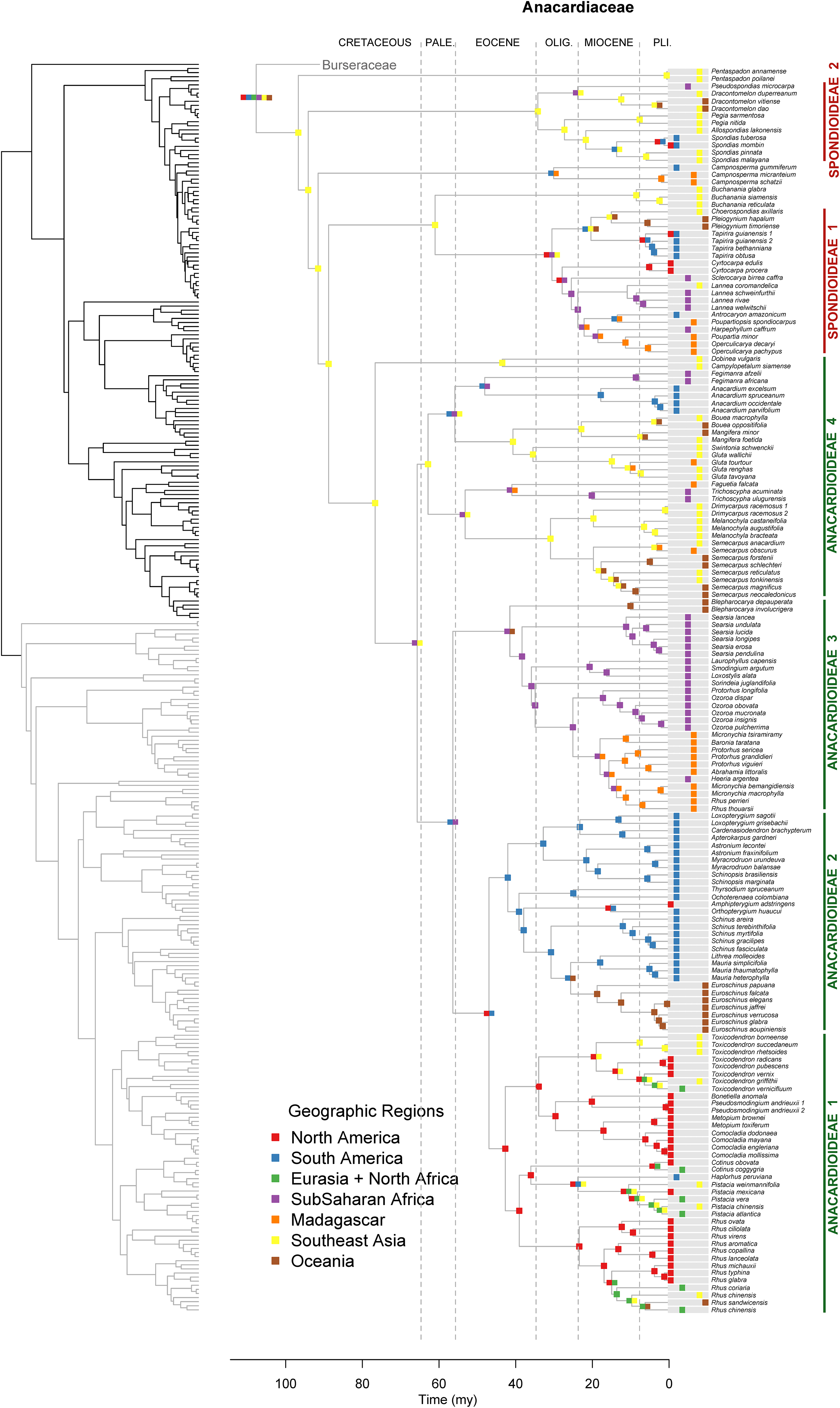
Figure 3. Maximum likelihood reconstruction of geographic range evolution for Anacardiaceae on the maximum clade credibility tree. Ancestral geographic ranges are shown at nodes. For each species, the extant geographic range is represented by colored boxes.
Within Burseraceae, phylogenetic relationships show the monotypic Mexican species Beiselia mexicana sister to a clade containing three well-supported lineages that correspond to the Protium alliance or Protieae (sensu Thulin et al., 2008), the Bursera alliance or Bursereae (sensu Thulin et al., 2008) and the Boswellia + Canarium alliance hereafter referred to as Garugeae (sensu Thulin et al., 2008) (Figure 4). Within Protieae, a clade of Southeast Asian and Madagascan species is sister to the species-rich American clade containing the remaining Protium species and the genera Tetragastris and Crepidospermum (see also Fine et al., 2014). Within Bursereae, the monotypic West African Aucoumea klaineana is sister to a well-supported clade containing American Bursera and predominantly African Commiphora. Our study finds marginal support for a paraphyletic Bursera with the lineage corresponding to Bursera subg. Bursera sister to Commiphora and Bursera subg. Elaphrium. More robustly sampled phylogenies of Anacardiaceae and Burseraceae (Pell et al. in prep.; Weeks et al. in prep.) will expand on the brief taxonomic results presented in this publication.
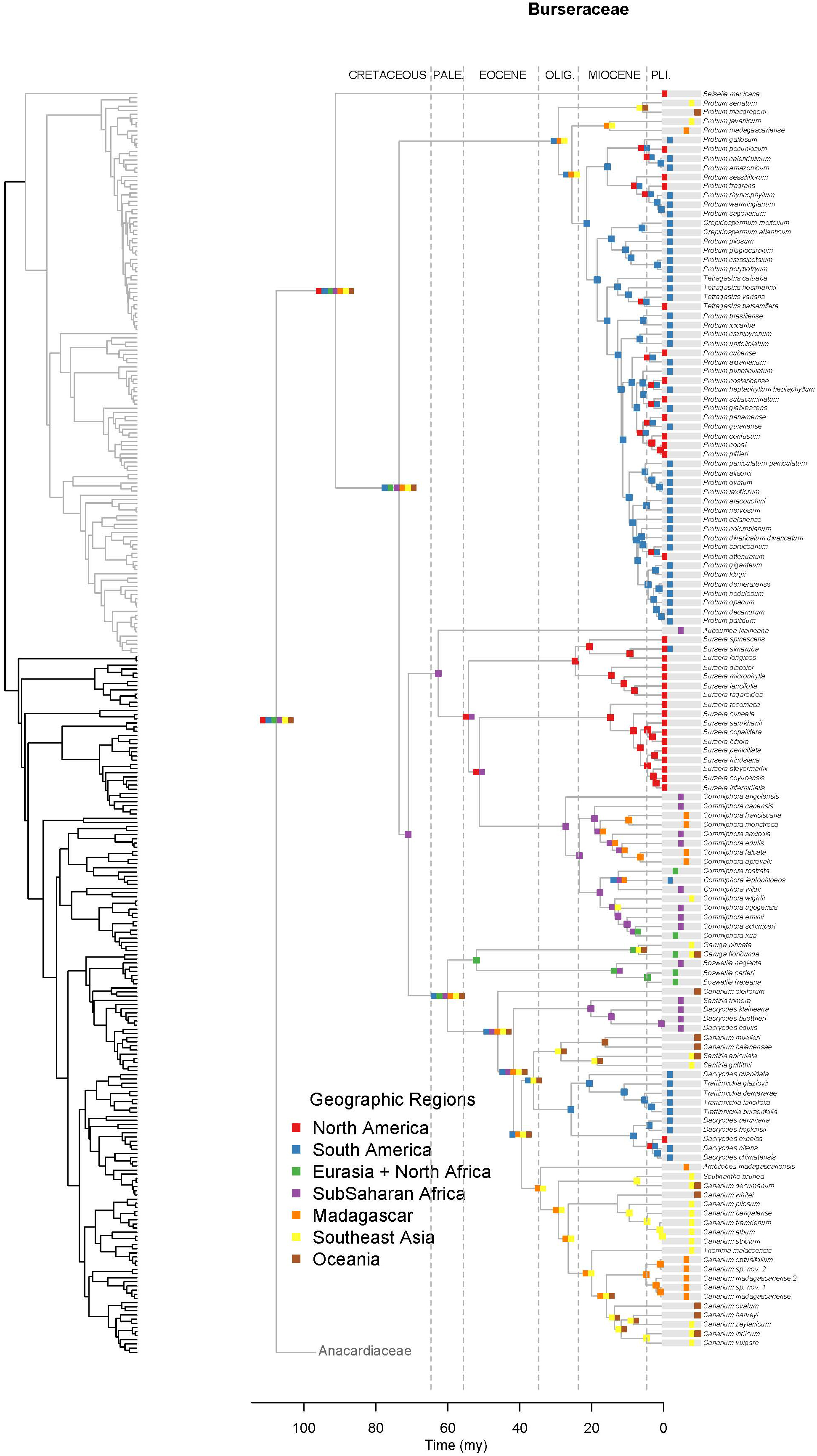
Figure 4. Maximum likelihood reconstruction of geographic range evolution for the Burseraceae on the maximum clade credibility tree. Ancestral geographic ranges are shown at nodes. For each species, the extant geographic range is represented by colored boxes.
Timing of Diversification and Diversification Patterns
Early diversification events in Terebinthaceae including stem and crown divergences of both families occurred in the Early to Late Cretaceous (Figure 2, Supplementary Material Figures S1A,B). The divergence of Terebinthaceae from the other Sapindales lineages and its split into Anacardiaceae and Burseraceae spanned the Albian–Aptian of the Early Cretaceous (116 Ma, 105–127 Ma; mean age, 95% HPD and 108 Ma, 95–121 Ma, respectively). The crown radiations of Anacardiaceae (97 Ma, 83–128 Ma) and Burseraceae (91 Ma, 78–106 Ma) were centered on the Cenomanian of the Late Cretaceous. Lineage through time (LTT) plots show that even though the radiation of Anacardiaceae is slightly older than that of Burseraceae, accumulation of lineages through time has been roughly equivalent in both families (Figure 5). Nevertheless, there is evidence that two, rather than one diversification process has governed the evolution of these families. The highest marginal likelihood was assigned to the model with different Birth-Death diversification processes for each family (LM = −1188.75). A Bayes Factor analysis shows very strong evidence in favor of this model against a model with a single Birth-Death diversification process for the whole tree (BF = 12). However, the mean net diversification rate for both families is approximately the same (2.4 vs. 2.5). BAMM analysis found strong support for a model with one rate shift, with a posterior probability p = 0.74. Bayes Factors show strong evidence in favor of this model vs. a null model with no shifts (BF = 574.73). These results suggest a substantial increase in speciation rate in the ancestral lineage leading to the Protieae within Burseraceae (Supplementary Material Figure S2). The posterior probability of a rate shift occurring in the three deepest branches of the Protieae is p = 0.91. Bayes Factors indicate overwhelming evidence in favor of a rate shift in the branch leading to the Neotropical Protieae (BF = 2004.07).
Geographic Range and Climatic Niche Evolution
Lagrange analyses show there is uncertainty in the geographic ranges and climatic niches for several ancestors (Supplementary Material Figure S3, Tables S1, S2). We define uncertainty as when multiple ancestral states are within two log-likelihood scores. With this in mind, we restrict our description of results and discussion only to the most likely reconstructions (i.e., the one with the highest relative probability).
Lagrange analysis indicates that the most recent common ancestor of Terebinthaceae had a widespread geographic range and it occurred in wet and dry tropical climatic niches (Figures 6, 7). Speciation within this broad geographic range led to a lineage restricted to tropical wet climates in Southeast Asia (for the most recent common ancestor of Anacardiaceae), and to a lineage inheriting the widespread ancestral geographic range and ancestral climatic niche (for the most recent common ancestor of Burseraceae). Within Anacardiaceae, subsequent speciation events during the Cretaceous occurred within Southeast Asia with a geographic range expansion into the tropical wet forests of sub–Saharan Africa around the Cretaceous–Paleocene boundary. During the Paleocene, geographic range expansion into sub-Saharan Africa led to allopatric divergence, with a daughter species inheriting the Southeast Asian geographic range and its sister species inheriting the sub-Saharan African range and further expanding into South America. The Southeast Asian ancestor later diverged into descendant species that expanded their geographic ranges to sub-Saharan Africa and colonizing South America. Although these geographic range expansions did not involve changes in the ancestral Tropical Wet climatic niche, the most recent common ancestor of Buchanania and Operculicarya expanded into tropical dry climates in Southeast Asia. During the Eocene, Anacardiaceae dispersed into North America, Oceania, and Madagascar, with some ancestors from tropical wet climatic niches expanding into tropical dry as well as temperate climatic niches. From the Miocene forward, there were multiple ancestral geographic range and climatic niche expansions, including the colonization of Eurasia and diversification in temperate climates. Overall, the predominant pattern in the geographic range and climatic niche evolution of Anacardiaceae is that ancestors with widespread geographic ranges and broad climatic tolerances were common and persisted through multiple speciation events, suggesting that dispersal and evolution of climatic tolerances are common in this family. The overall rate of dispersal/expansion for geographic range evolution was D = 0.15, and it was D = 0.14 for climatic niche evolution. Transitions between climatic niches were extremely common and included 74 instances of expansions or specializations to new niches (Figure 6).
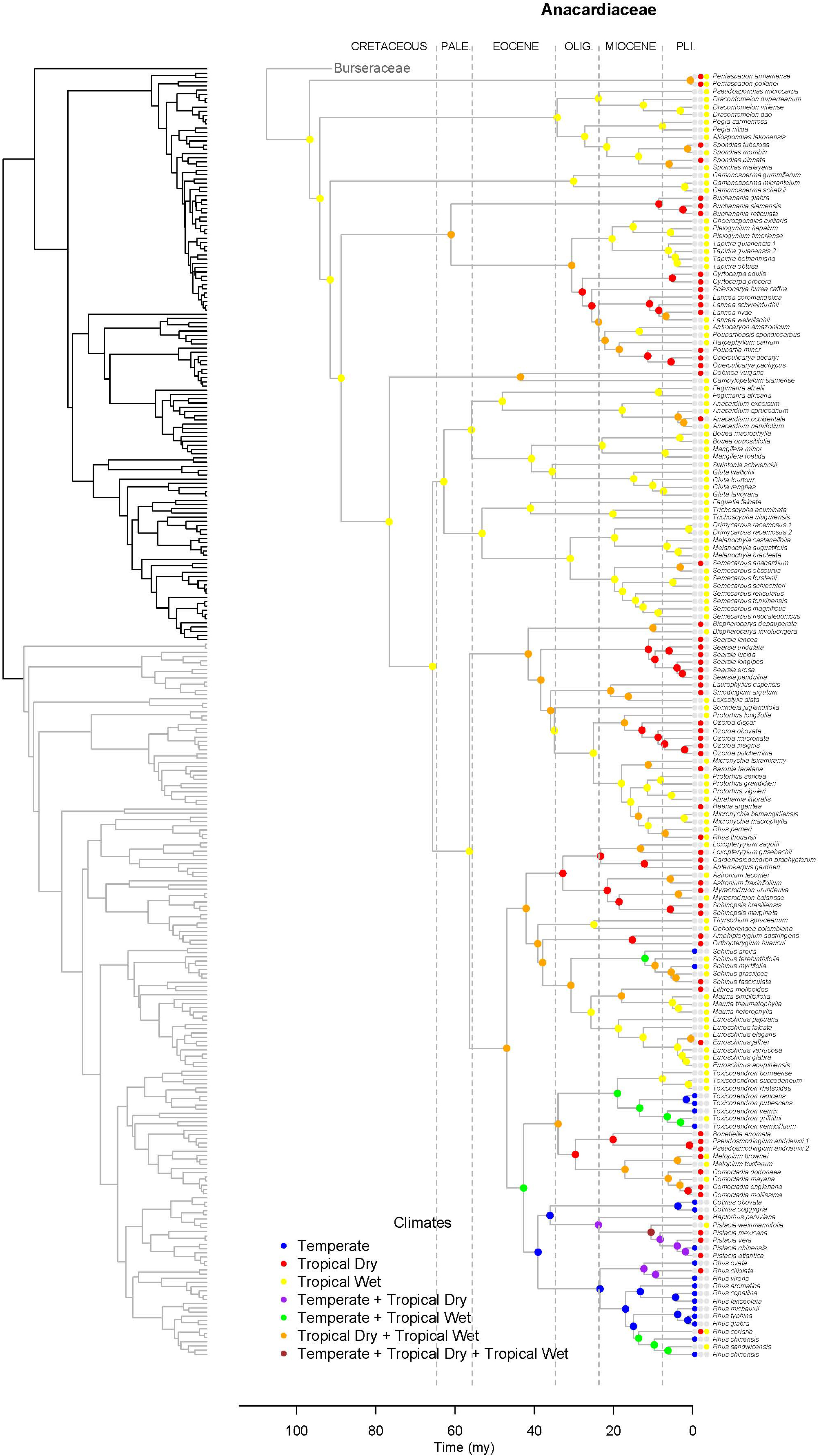
Figure 6. Maximum likelihood reconstruction of climatic niche evolution for Anacardiaceae on the maximum clade credibility tree. Ancestral climatic niches are shown at nodes. For each species, the current climatic niche is represented by colored circles.
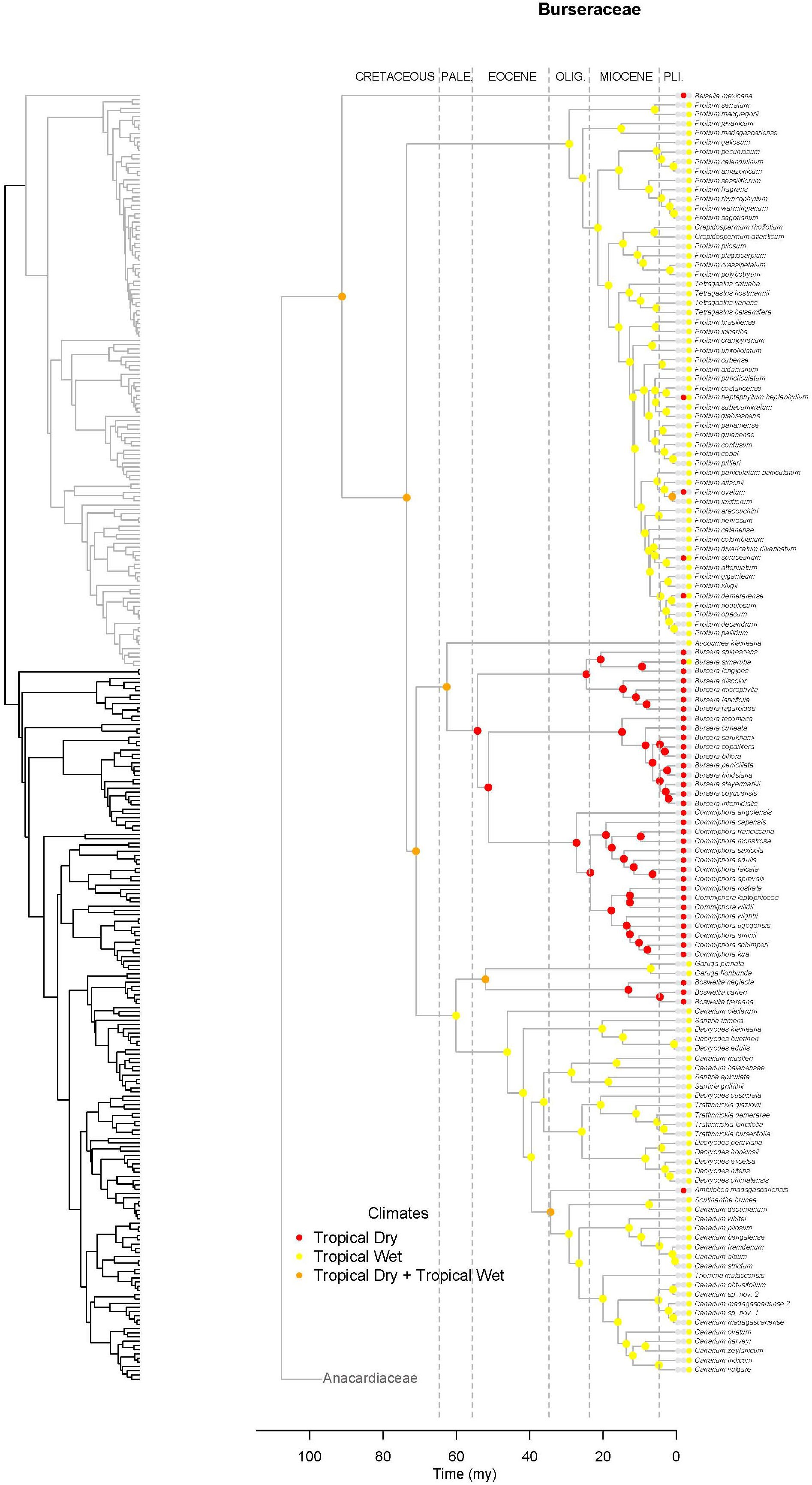
Figure 7. Maximum likelihood reconstruction of climatic niche evolution for Burseraceae on the maximum clade credibility tree. Ancestral climatic niches are shown at nodes. For each species, the current climatic niche is represented by colored circle.
Unlike Anacardiaceae, Burseraceae did not experience as many geographic range expansions and clearly fewer climatic niche expansions (Figure 7). In general, several ancestors with widespread geographic ranges spanned multiple speciation events. However, multiple ancestors diversified within distinct geographic regions. The overall rate of dispersal/expansion for geographic range evolution was D = 0.22. In contrast, few ancestors occurred across wet and dry tropical climates, and these quickly specialized to either tropical wet or tropical dry climates. The overall rate of dispersal/expansion for climatic niche evolution was D = 0.05. In total, there were only 11 cladogenic events that coincided with the transition from broad tropical climates to tropical dry or tropical wet climates. The only exception to this pattern is the Garugeae clade in which widespread geographic ranges among ancestors were maintained across multiple cladogenic events. Nevertheless, this clade remained highly specialized to tropical wet climates.
Discussion
Origins of Terebinthaceae and Patterns of Diversification in Anacardiaceae and Burseraceae
The Cretaceous age of the stem and crown lineages of Terebinthaceae (95–127 Ma) coincides with several episodes of continental vicariance that may have contributed to their early widespread distribution and spurred their diversification through allopatric speciation. Biogeographic reconstructions place the common ancestor of extant Anacardiaceae in Southeast Asia and extant Burseraceae in virtually all pantropical locations soon after their divergence. This reconstruction, combined with the Northern Hemisphere distribution of pre-Eocene Terebinthaceae fossils and the earliest diverging extant taxa, points most strongly to an origin of Terebinthaceae in Laurasia rather than Gondwana. In Burseraceae, the oldest fossils attributable to the clade derive from the Paleocene and Eocene of North America and Britain (Daly et al., 2011). Definitively Anacardiaceae fossils appear in the Eocene floras of North America and Europe (e.g., Taylor, 1990; Manchester, 1994; Ramírez and Cevallos-Ferriz, 2002; Meyer, 2003). An intriguing possibility is that the northward incursion of the Atlantic Ocean between North America and Eurasia/proto Southeast Asia during the Upper Cretaceous (Seton et al., 2012) contributed to these early diversification events by interrupting gene flow among the increasingly isolated regions of Laurasia.
After the divergence of the families, the Upper Cretaceous brought crown radiations and the establishment of at least four lineages in Burseraceae and six in Anacardiaceae (Figures 2–4 and Supplementary Material). Timing of the older diversification events within Burseraceae is broadly comparable to that found by previous studies of the family. Similar studies in Anacardiaceae are lacking, with the biogeography of only some of the more recently diversified clades having been evaluated. Our study places the crown radiation of Burseraceae firmly in the Upper Cretaceous, notably older than the Paleocene age estimated by Weeks et al. (2005) and Fine et al. (2014). This discrepancy may be caused by the inclusion of the species sister to all other members of the family, Beiselia mexicana, a monotypic genus distributed in western Mexico. It has highly divergent DNA sequences and may inflate older ages as a consequence of its effect on the DNA alignment across Sapindalean taxa.
During the Cretaceous, Anacardiaceae accumulated more lineages than Burseraceae, either through increased speciation or decreased extinction (Figures 2–5). Diversifications of Burseraceae also span the K-T boundary. The stem and crown ages of Burseraceae's Protieae, Bursereae and Garugeae clades closely match the Paleocene ages estimated by studies that sample fewer Sapindalean outgroups (Weeks et al., 2005; De-Nova et al., 2012; Fine et al., 2014). Phylogenetic relationships resolved within these large clades reveal the ancient but relatively rapid establishment of pantropical distributions.
Our analyses of diversification through time indicate that the evolutionary history of Terebinthaceae has been shaped by a mixture of heterogeneous processes. We found strong evidence for an explosive burst in speciation associated with the origin of the Neotropical Protieae. Fine et al. (2014) also found support for a rate shift for this clade using a different modeling approach. Taken together, this suggests that the acceleration in rates during this time interval likely reflects the occurrence of a key innovation or the colonization of a new geographic region and open ecological opportunities. It is noteworthy that similar geographic range expansions did not lead to rate shifts elsewhere in the history of Terebinthaceae [e.g., the clade Bursera (Burseraceae) or the clade Anacardioideae 2 (Anacardiaceae)]. Although sampling bias may influence the inference of diversification dynamics, the fast and recent radiation of the Neotropical Protieae (Fine et al., 2014) deeply altered the steady the accumulation of lineages in the history of Terebinthaceae from the Cretaceous to the present. Increased sampling in other species-rich clades such as Bursera could inform whether more rate shifts have shaped the evolutionary history of Terebinthaceae.
Geographic Range Evolution: Have Lineages Persisted in Unique Geographic Regions or have they Dispersed to New Geographic Regions (i.e., how Common is Dispersal)?
Long-distance dispersal features prominently in the biogeographic history of Terebinthaceae. Although dispersal rates estimated with Lagrange may not be precise (Ree and Smith, 2008), our results suggest that dispersal rates for both Anacardiaceae and Burseraceae are relatively high and similar (DAnacardiaceae = 0.15, DBurseraceae = 0.22). While frequency of long-distance dispersal in plants is not necessarily correlated to dispersal syndrome (Higgins et al., 2003), seeds of the majority of Burseraceae and Anacardiaceae taxa are dispersed by animals (esp. birds, bats, terrestrial mammals; Daly et al., 2011; Pell et al., 2011). Some members of both families are wind-dispersed and a few Anacardiaceae species are water dispersed. A closer examination of Terebinthaceae evolution reveals cases in which repeated short-distance dispersals or extreme long-distance dispersal must be invoked. In Burseraceae, within Garugeae, separation of Southeast Asian and African taxa occurs (Boswellia, Garuga; 52 Ma, 33–68 Ma) along with a separation of a New Caledonian endemic taxon, Canarium oleiferum, from the remaining pantropical Garugeae clade (46 Ma, 36–59 Ma). In Anacardiaceae, sister lineages from South America and sub-Saharan Africa diverge (Anacardium, Fegimanra; 48 Ma, 47–51 Ma), African and Oceanian taxa split (Blepharocarya, rest of Anacardioideae ‘2 Ma, 30–56 Ma), Madagascan and African taxa diverge (Faguetia, Trichoscypha; 41 Ma, 25–58 Ma), and South and North American lineages diverge (Anacardioideae 1, 2; 47 Ma, 39–57 Ma). The similar timing of these biogeographic expansions suggests relatively rapid dispersal among continents followed by radiation within continental regions but does not indicate shared routes. For instance, Fine et al. (2014) posits that the predominantly South American Protieae derived from an ancestor that dispersed across the Atlantic Ocean from North America or Africa, whereas Weeks and Simpson (2007) suggest the ancestor of Paleotropical Commiphora migrated from the Americas to the Old World across the North Atlantic boreotropical land-bridge.
Closer examination of the biogeographical reconstructions of these two families reveal repeated instances of lineage divergences associated with two different regions and/or continents. These splits occurred throughout the past 100 million years, including some very recent events. While some of these divergences may have been due to vicariance, we believe the great majority of them have been due to long-distance dispersal events. Recent reviews of the biogeographic history tropical woody plant lineages have emphasized the importance of long-distance dispersal (Lavin et al., 2004; Pennington and Dick, 2004; Renner, 2004). Our results support this view, and we conclude that both Anacardiaceae and Burseraceae have moved easily across oceans.
Climatic Niche Evolution: Have Lineages Retained Distinct Climatic Niches or have they Evolved Climatic Tolerances (i.e., how Common is “Niche Expansion”)?
Burseraceae are characterized by a low degree of climatic niche evolution. There are no frost-tolerant species, i.e., all Burseraceae are restricted to tropical and subtropical latitudes. Although Burseraceae are common and even dominant elements in seasonally-dry tropical forests and xeric scrublands as well as moist/wet rain forests across the tropics, switches between wet and dry climates are relatively rare in the family (Figure 7). For example, Commiphora and Bursera, which dominate some seasonally-dry regions of sub-Saharan Africa and Mesoamerica respectively, share a common ancestor that almost certainly was a dry forest specialist. Both dry-forest and wet-forest lineages are ancient in Burseraceae, and both climatic niches have included Burseraceae taxa since before the Paleocene.
Unlike Burseraceae, transitions among climatic niches are extremely common in Anacardiaceae. Our estimate of the overall rate of dispersal/expansion for Anacardiaceae is at least twice the rate in Burseraceae (DAnacardiaceae = 0.14, DBurseraceae = 0.05). In the Lagrange analysis (Figure 6), the most recent common ancestor of all Anacardiaceae and all deep nodes within the family are hypothesized to be wet forest taxa until the first expansion into dry climates during the Paleocene and then many more during the Eocene. Colonization of the temperate zone occurred early in Anacardiaceae evolution, likely during the Eocene. There have been at least 74 climate transitions (expansions and specializations) across all climatic niches examined here. Interestingly, although transitions between wet and dry climates are very common in Anacardiaceae, expansion into the temperate climate appears to have occurred in only one clade (although may have been lost and re-evolved in the same clade several times). This clade includes a broad selection of genera primarily in the Americas with a scattering of taxa from Europe, Asia, and the Pacific. Clades within this lineage in which frost tolerance has evolved are Cotinus, Pistacia, Rhus s.s., Schinus, and Toxicodendron. Within this clade, there have been multiple transitions among temperate and wet and dry tropical climates.
It is clear that climatic niche evolution is not integral to explaining the high species diversity of Burseraceae, as close relatives almost always occur in the same climatic niche. However, for Anacardiaceae, it is tempting to make a connection between climatic niche evolution and diversification. For several clades, sister species (or sister clades) share the same geographic region but inhabit different climatic niches, suggesting that specialization to dry or wet (or temperate vs. tropical) could arise after a widespread taxon lived in more than one climate. Tradeoffs involved in drought or frost tolerance are likely involved in such specialization. For example, Pittermann et al. (2012) showed that adaptation to dry biomes by Cupressaceae trees involves cavitation resistant xylem which results in reduced photosynthetic rates causing low growth rates which presumably prevents dry-adapted lineages from competing successfully in wet biomes.
Wind dispersal occurs in both families but to a greater degree of frequency and complexity in Anacardiaceae. Burseraceae have five wind-dispersed genera (Aucoumea, Ambilobea, Beiselia, Boswellia and Triomma; ca. 24 species) in all of which wings are obtained via conplanation of the pyrene, whereas Anacardiaceae have 23 genera, including ca. 75 species, in which a wide variety of mechanisms have evolved to facilitate wind dispersal. These include, for example, wings developed from petals, sepals, pericarp, subtending bracts, and whole inflorescences. Wind dispersal is more common in tropical dry forests than in tropical wet forests (Gentry, 1982, 1991), and thus perhaps affords an evolutionary advantage in this habitat. Fruit structure in Anacardiaceae may be more plastic than in Burseraceae, suggested by the fruit diversity in the family and the multiple times wind dispersal has evolved through the modification of different structures. Anacardiaceae, after being dispersed to a dry habitat, may have evolved more advantageous wind dispersed fruits quickly in situ, or they may have evolved wind-dispersed fruits in wet habitats then dispersed to dry habitats. Physiological responses to environment may also play a role in the ability of Anacardiaceae to change habitats. In some wet to dry switching lineages, like Astronium, the moist forest species occur in areas that often have a briefly drier period during which the species may lose their leaves. Ancestors of these lineages may have also had periodic deciduousness, possibly pre-adapting them to more seasonal forests with longer, more extreme dry periods. Other Anacardiaceae lineages include examples of morphological plasticity with respect to leaf morphology. For example in the genera Loxopterygium and Astronium leaves of most wet-habitat taxa are mostly entire margined, while leaves of most dry-habitat taxa have toothed margins.
In contrast to Anacardiaceae, it appears that the great majority of diversification occurs within climatic niches for Burseraceae. There are many mechanisms that could yield this pattern. First, if a lineage has frequent dispersal to the same climatic niche in different geographic regions, allopatric speciation could occur, and then re-dispersal back to the original region could inflate sympatric species totals. Second, habitat specialization to other habitats within climatic niches or niche partitioning along other niche axes within habitats can increase the numbers of species of a lineage within a climatic niche. For example, edaphic specialization to different soil types has been implicated in the diversification of the Protieae (Fine et al., 2005, 2014). Finally, escape from natural enemies through effective chemical defenses may promote species radiations within biomes (Ehrlich and Raven, 1964). Becerra (2007) showed that the terpene defenses of Bursera were more divergent than expected by co-occurring species within regions, and she suggested that coevolutionary interactions between Burseraceae-feeding beetles promoted chemical divergence and speciation in this group. Other Burseraceae lineages such as the Protieae have also been shown to express a wide diversity of terpenes and other non-volatile antiherbivore defenses Fine et al., 2006, 2014; Zapata and Fine, 2013.
Conclusion
We found that a densely sampled, comprehensive geographic sample of Anacardiaceae and Burseraceae taxa has yielded a highly supported phylogenetic reconstruction that supports current taxonomic concepts of both families. Moreover, our fossil-calibrated chronogram and biogeographic analyses give results that are broadly congruent with the fossil record. We conclude that the most common ancestor to these families was widespread and likely originated in Northern Hemisphere during the Cretaceous. Continental vicariance between hemispheres may have spurred initial divergence into Burseraceae and Anacardiaceae and indeed the two families followed different evolutionary trajectories since their split, with Anacardiaceae steadily accumulating lineages since the late Cretaceous–Paleocene while the majority of Burseraceae's diversification has occurred much more recently, with Miocene radiations of the Protieae and Bursereae. Both families have relied on effective wind and animal dispersal to achieve pantropical distributions with multiple intercontinental colonization events inferred for both families throughout the past 100 million years. Anacardiaceae have shifted climatic niches frequently during this time, including colonization of the temperate biomes, while Burseraceae have experienced very few shifts between tropical dry and tropical wet climates, with no temperate zone adaptation. Thus, in the context of the question of whether is it easier for these plant lineages to move or to evolve, we conclude that both Anacardiaceae and Burseraceae move easily, but Anacardiaceae have a much greater capacity to adapt to new climate regimes than Burseraceae and this is one of the most striking features of their evolutionary history.
Conflict of Interest Statement
The authors declare that the research was conducted in the absence of any commercial or financial relationships that could be construed as a potential conflict of interest.
Acknowledgments
Research presented was funded by: NSF DEB awards 0919179 to Weeks, 0919567 to Fine, 0918600 to Daly and Mitchell, and 0919485 to Pell; the Thomas and Kate Jeffress Memorial Foundation (Weeks), Conservation International (Pell), and the Beneficia Foundation (Pell). Any opinions, findings, and conclusions or recommendations expressed in this material are those of the authors and do not necessarily reflect the views of the National Science Foundation or our other sponsors. Analyses were conducted with computational resources and services at the Center for Computation and Visualization at Brown University, supported in part by NSF EPSCoR EPS-1004057 and the State of Rhode Island. We would like to thank the following herbaria for allowing us to destructively sample specimens for this project: BKL, GMUF, H, JEPS, L, MO, NY, UC, US.
Supplementary Material
The Supplementary Material for this article can be found online at: http://www.frontiersin.org/journal/10.3389/fgene.2014.00409/abstract
References
Becerra, J. X. (2007). The impact of plant–herbivore coevolution on plant community structure. Proc. Natl. Acad. Sci. U.S.A. 104, 7843–7488. doi: 10.1073/pnas.0608253104
Bell, C. D., and Donoghue, M. J. (2005). Dating the diversification of Dipsacales: comparing models, genes, and evolutionary implications. Am. J. Bot. 92, 284–296. doi: 10.3732/ajb.92.2.284
Pubmed Abstract | Pubmed Full Text | CrossRef Full Text | Google Scholar
Bouchenak-Khelladi, Y., Maurin, O., Hurter, J., and Van Der Bank, M. (2010). The evolutionary history and biogeography of Mimosoideae (Leguminosae): an emphasis on African acacias. Mol. Phylogenet. Evol. 57, 495–508. doi: 10.1016/j.ympev.2010.07.019
Pubmed Abstract | Pubmed Full Text | CrossRef Full Text | Google Scholar
Bremer, K., Bremer, B., and Thulin, M. (1999). Introduction to Phylogeny and Systematics of Flowering Plants, 5th Edn. Uppsala: Uppsala University.
Burnham, R. J., and Carranco, N. L. (2004). Miocene winged fruits of Loxopterygium (Anacardiaceae) from the ecuadorian andes. Am. J. Bot. 91, 1767–1773. doi: 10.3732/ajb.91.11.1767
Pubmed Abstract | Pubmed Full Text | CrossRef Full Text | Google Scholar
Castresana, J. (2000). Selection of conserved blocks from multiple alignments for their use in phylogenetic analysis. Mol. Biol. Evol. 17, 540–552. doi: 10.1093/oxfordjournals.molbev.a026334
Pubmed Abstract | Pubmed Full Text | CrossRef Full Text | Google Scholar
Chanderbali, A. S. H., Van Der Werff, H., and Renner, S. S. (2001). The relationships and historical biogeography of Lauraceae: evidence from the chloroplast and nuclear genomes. Ann. Mo. Bot. Gard. 88, 104–134. doi: 10.2307/2666133
Chandler, M. E. J. (1961). The Lower Tertiary Floras of Southern England. London: British Museum of Natural History.
Clyde, W., Stamatakos, J., Zonneveld, J.-P., Gunnell, G. F., and Bartels, W. S. (1995). Timing of the Wasatchian–Briderian (early–middle eocene) faunal transition in the Green River Basin. J. Vert. Paleont. 15(Suppl. 3), 24A.
Daly, D. C., Harley, M. M., Martínez-Habibe, M.-C., and Weeks, A. (2011). “Burseraceae,” in The Families and Genera of Vascular Plants, Vol. 10, ed. K. Kubitzki (New York, NY: Springer-Verlag), 76–104.
Davis, C. C., Fritsch, P. W., Bell, C. D., and Mathews, S. (2004). High latitude tertiary migrations of an exclusively tropical clade: evidence from Malpighiaceae. Int. J. Plant Sci. 165, S107–S121. doi: 10.1086/383337
De-Nova, J., Medina, R., Montero, J. C., Weeks, A., Rosell, J. A., Olson, M. E., et al. (2012). Insights into the historical construction of species-rich Mesoamerican seasonally dry tropical forests: the diversification of Bursera (Burseraceae, Sapindales). New Phytol. 193, 276–287. doi: 10.1111/j.1469-8137.2011.03909.x
Pubmed Abstract | Pubmed Full Text | CrossRef Full Text | Google Scholar
Donoghue, M. J. (2008). A phylogenetic perspective on the distribution of plant diversity. Proc. Natl. Acad. Sci. U.S.A. 105, 11549–11555. doi: 10.1073/pnas.0801962105
Pubmed Abstract | Pubmed Full Text | CrossRef Full Text | Google Scholar
Donoghue, M. J., and Edwards, E. J. (2014). Biome shifting in plant evolution. Annu. Rev. Ecol. Evol. Syst. 45, 547–572. doi: 10.1146/annurev-ecolsys-120213-091905
Drummond, A. J., Ho, S. Y. W., Phillips, M. J., and Rambaut, A. (2006). Relaxed phylogenetics and dating with confidence. PLoS Biol. 4:e88. doi: 10.1371/journal.pbio.0040088
Pubmed Abstract | Pubmed Full Text | CrossRef Full Text | Google Scholar
Drummond, A. J., Suchard, M. A., Xie, D., and Rambaut, A. (2012). Bayesian phylogenetics with BEAUti and BEAST 1.7. Mol. Biol. Evol. 29, 1969–1973. doi: 10.1093/molbev/mss075
Pubmed Abstract | Pubmed Full Text | CrossRef Full Text | Google Scholar
Ehrlich, P. R., and Raven, P. (1964). Butterflies and plants: a study in coevolution. Evolution 18, 586–606. doi: 10.2307/2406212
Engler, A. (1881). Über die morphologischen verhältnisse und die geographische verbreitung der gattung Rhus, wie der mit ihr verwandten, lebenden und ausgestorbenen Anacardiaceae. Bot. Jahrb. Syst. Pflangengeschichte Pflanzengeographie 1, 365–426.
Engler, A. (1883). “Anacardiaceae,” in Monographie Phanerogamarum, Vol. 4, eds A. P. de Candolle and A. C. de Candolle (Paris: G. Masson), 171–546.
Engler, A. (1892). “Anacardiaceae,” in die Natürlichen Pflanzenfamilien Vol. 3, eds. A. Engler and K. Prantl (Leipzig: W. Engelmann), 138–178.
Faguet, A. (1874/1875). “Terebinthaceae,” in Histoire Des Plantes, Vol. 5, ed H. E. Baillon (Paris: Librairie Hachette), 257–341.
Fernando, E. S., Gadek, P. A., and Quinn, C. J. (1995). Simaroubaceae, an artificial construct: evidence from rbcL sequence variation. Am. J. Bot. 82, 92–103. doi: 10.2307/2445791
Fine, P. V. A., Daly, D. C., Villa, F. G., Mesones, I., and Cameron, K. (2005). The contribution of edaphic heterogeneity to the evolution and diversity of Burseraceae trees in the Western Amazon. Evolution 59, 1464–1478. doi: 10.1554/04-745
Pubmed Abstract | Pubmed Full Text | CrossRef Full Text | Google Scholar
Fine, P. V. A., Miller, Z. J., Mesones, I., Irazuzta, S., Appel, H. M., Henry, M., et al. (2006). The growth-defense trade-off and habitat specialization by plants in Amazonian forests. Ecology 87, S150–S162. doi: 10.1890/0012-9658(2006)87[150:TGTAHS]2.0.CO;2
Pubmed Abstract | Pubmed Full Text | CrossRef Full Text | Google Scholar
Fine, P. V. A., Zapata, F., and Daly, D. C. (2014). Investigating processes of neotropical rain forest tree diversification by examining the evolution and historical biogeography of the Protieae (Burseraceae). Evolution 68, 1988–2004. doi: 10.1111/evo.12414
Pubmed Abstract | Pubmed Full Text | CrossRef Full Text | Google Scholar
Forest, F. (2009). Calibrating the tree of life: fossils, molecules and evolutionary timescales. Ann. Bot. 104, 789–794. doi: 10.1093/aob/mcp192
Pubmed Abstract | Pubmed Full Text | CrossRef Full Text | Google Scholar
Gadek, P. A., Fernando, E. S., Quinn, C. J., Hoot, S. B., Terrazas, T., Sheahan, M. C., et al. (1996). Sapindales: molecular delimitation and infraordinal groups. Am. J. Bot. 83, 802–811. doi: 10.2307/2445857
Gentry, A. H. (1982). Neotropical floristic diversity: phytogeographical connections between Central and South America, Pleistocene climatic fluctuations, or an accident of the Andean orogeny? Ann. Mol. Bot. Gard. 69, 557–593. doi: 10.2307/2399084
Gentry, A. H. (1988). Changes in plant community diversity and floristic composition on environmental and geographical gradients. Ann. Mo. Bot. Gard. 75, 1–34. doi: 10.2307/2399464
Gentry, A. H. (1991). “Breeding and dispersal systems of lianas,” in The Biology of Vines, eds F. E. Putz and H. A. Mooney (Cambridge: Cambridge University Press), 393–424.
Gernhard, T. (2008). The conditioned reconstructed process. J. Theor. Biol. 253, 769–778. doi: 10.1016/j.jtbi.2008.04.005
Pubmed Abstract | Pubmed Full Text | CrossRef Full Text | Google Scholar
Groppo, M., Pirani, J. R., Salatino, M. L. F., Blanco, S. R., and Kallunki, J. A. (2008). Phylogeny of Rutaceae based on two noncoding regions from cpDNA. Am. J. Bot. 95, 985–1005. doi: 10.3732/ajb.2007313
Pubmed Abstract | Pubmed Full Text | CrossRef Full Text | Google Scholar
Harley, M. M., and Daly, D. C. (1995). Burseraceae Kunth, Protieae March. em. Engl. World Pollen Spore Flora 20, 1–44.
Harrington, M. G., Edwards, K. J., Johnson, S. A., Chase, M. W., and Gadek, P. A. (2005). Phylogenetic inference in Sapindaceae sensu lato using plastid matK and rbcL DNA sequences. Syst. Bot. 30, 366–382. doi: 10.1600/0363644054223549
Higgins, S. I., Nathan, R., and Cain, M. L. (2003). Are long-distance events in plants usually caused by nonstandard means of dispersal? Ecology 84, 1945–1956. doi: 10.1890/01-0616
Hoorn, C., Wesselingh, F. P., ter Steege, H., Bermudez, M. A., Mora, A., Sevink, J., et al. (2010). Amazonia through time: andean uplift, climate change, landscape evolution, and biodiversity. Science 330, 927–931. doi: 10.1126/science.1194585
Pubmed Abstract | Pubmed Full Text | CrossRef Full Text | Google Scholar
Hughes, C., and Eastwood, R. (2006). Island radiation on a continental scale: exceptional rates of plant diversification after uplift of the Andes. Proc. Natl. Acad. Sci. U.S.A. 103, 10334–10339. doi: 10.1073/pnas.0601928103
Pubmed Abstract | Pubmed Full Text | CrossRef Full Text | Google Scholar
Hughes, C. E., Pennington, R. T., and Antonelli, A. (2013). Neotropical plant evolution: assembling the big picture. Bot. J. Linn. Soc. 171, 1–18. doi: 10.1111/boj.12006
Katoh, K., and Standley, D. M. (2013). MAFFT Multiple sequence alignment software version 7: improvements in performance and usability. Mol. Biol. Evol. 30, 772–780. doi: 10.1093/molbev/mst010
Pubmed Abstract | Pubmed Full Text | CrossRef Full Text | Google Scholar
Lanfear, R., Calcott, B., Ho, S. Y. W., and Guindon, S. (2012). PartitionFinder: combined selection of partitioning schemes and substitution models for phylogenetic analyses. Mol. Biol. Evol. 29, 1695–1701. doi: 10.1093/molbev/mss020
Pubmed Abstract | Pubmed Full Text | CrossRef Full Text | Google Scholar
Lavin, M., Schrire, B. D., Lewis, G. P., Pennington, R. T., Delgado-Salinas, A., Thulin, M., et al. (2004). Metacommunity process rather than continental tectonic history better explains geographically structured phylogenies in legumes. Phil. Trans. R. Soc. Lond. B 359, 1509–1522. doi: 10.1098/rstb.2004.1536
Pubmed Abstract | Pubmed Full Text | CrossRef Full Text | Google Scholar
MacGinitie, H. D. (1953). Fossil plants of the Florissant beds, Colorado. Carnegie Inst. Wash. 599, 1–188.
MacGinitie, H. D. (1969). The Eocene Green River flora of northwestern Colorado and northeastern Utah. Univ. Calif. Publ. Geol Sci. 83, 1–140.
Magallón, S., and Castillo, A. (2009). Angiosperm diversification through time. Am. J. Bot. 96, 349–365. doi: 10.3732/ajb.0800060
Pubmed Abstract | Pubmed Full Text | CrossRef Full Text | Google Scholar
Manchester, S. R. (1994). Fruits and seeds of the middle Eocene nut beds flora, Clarno Formation, Oregon. Palaeontographica Americana 58, 1–205.
Manchester, S. R., Wilde, V., and Collinson, M. E. (2007). Fossil cashew nuts from the Eocene of Europe: biogeographic links between Africa and South America. Intl. J. Plant. Sci. 68, 1199–1206. doi: 10.1086/520728
Mitchell, J. D., Daly, D. C., Pell, S. K., and Randrianasolo, A. (2006). Poupartiopsis gen. nov. and its context in Anacardiaceae classification. Syst. Bot. 31, 337–348. doi: 10.1600/036364406777585757
Mitchell, J. D., and Mori, S. A. (1987). The cashew and its relatives (Anacardium: Anacardiaceae). Mem. N.Y. Bot. Gard. 42, 1–76.
Moffett, R. O. (2007). Name changes in the old world Rhus and recognition of Searsia (Anacardiaceae). Bothalia 37, 165–175.
Muellner, A. N., Savolainen, V., Samuel, R., and Chase, M. W. (2006). The mahogany family “out-of-Africa”: divergence time estimation, global biogeographic patterns inferred from plastid rbcL DNA sequences, extant, and fossil distribution of diversity. Mol. Phylogenet. Evol. 40, 236–250. doi: 10.1016/j.ympev.2006.03.001
Pubmed Abstract | Pubmed Full Text | CrossRef Full Text | Google Scholar
Olson, M. E., and Carlquist, S. (2001). Stem and root anatomical correlations with life form diversity, ecology and systematics in Moringa (Moringaceae). Bot. J. Linn. Soc. 135, 315–348. doi: 10.1111/j.1095-8339.2001.tb00786.x
Paradis, E. (2013). Molecular dating of phylogenies by likelihood methods: a comparison of models and a new information criterion. Mol. Phylogenet. Evol. 67, 436–444. doi: 10.1016/j.ympev.2013.02.008
Pubmed Abstract | Pubmed Full Text | CrossRef Full Text | Google Scholar
Pell, S. K. (2004). Molecular Systematics of the Cashew Family (Anacardiaceae). Baton Rouge, LA: Doctoral Dissertation, Louisiana State University.
Pell, S. K., Mitchell, J. D., Lobova, T., and Miller, A. J. (2011). “Anacardiaceae,” in The Families and Genera of Vascular Plants, Vol. 10, ed K. Kubitzki (New York, NY: Springer-Verlag), 7–50.
Pell, S. K., Mitchell, J. D., Randrianasolo, A., Lowry, P., and Urbatsch, L. E. (2008). Phylogenetic split of Malagasy and African taxa of Protorhus and Rhus (Anacardiaceae) based on cpDNA trnL-trnF and nrDNA ETS and ITS sequence data. Syst. Bot. 33, 375–383. doi: 10.1600/036364408784571545
Pennington, R. T., and Dick, C. W. (2004). The role of immigrants in the assembly of the South American rainforest tree flora. Phil. Trans. R. Soc. Lond. B 359, 1611–1622. doi: 10.1098/rstb.2004.1532
Pubmed Abstract | Pubmed Full Text | CrossRef Full Text | Google Scholar
Pennington, R. T., Lavin, M., Särkinen, T., Lewis, G. P., Klitgaard, B. B., and Hughes, C. E. (2010). Contrasting plant diversification histories within the Andean biodiversity hotspot. Proc. Natl. Acad. Sci. U.S.A. 107, 13783–13787. doi: 10.1073/pnas.1001317107
Pubmed Abstract | Pubmed Full Text | CrossRef Full Text | Google Scholar
Pittermann, J., Stuart, S. A., Dawson, T. E., and Moreau, A. (2012). Cenozoic climate change shaped the evolutionary ecophysiology of the Cupressaceae conifers. Proc. Natl. Acad. Sci. U.S.A. 109, 9647–9652. doi: 10.1073/pnas.1114378109
Pubmed Abstract | Pubmed Full Text | CrossRef Full Text | Google Scholar
Rabosky, D. (2014). Automatic detection of key innovations, rate shifts, and the diversity-dependence on phylogenetic trees. PLOS ONE 9:e89543. doi: 10.1371/journal.pone.0089543
Pubmed Abstract | Pubmed Full Text | CrossRef Full Text | Google Scholar
Ramírez, J. L., and Cevallos-Ferriz, S. R. S. (2002). A diverse assemblage of Anacardiaceae from Oligocene sediments, Tepexi de Rodriquez, Puebla, Mexico. Am. J. Bot. 89, 535–545. doi: 10.3732/ajb.89.3.535
Pubmed Abstract | Pubmed Full Text | CrossRef Full Text | Google Scholar
Raven, P. H., and Axelrod, D. I. (1974). Angiosperm biogeography and past continental movements. Ann. Mo. Bot. Gard. 61, 539–673. doi: 10.2307/2395021
Ree, R. H., Moore, B. R., Webb, C. O., and Donoghue, M. J. (2005). A likelihood framework for inferring the evolution of geographic range on phylogenetic trees. Evolution 59, 2299–2311. doi: 10.1111/j.0014-3820.2005.tb00940.x
Pubmed Abstract | Pubmed Full Text | CrossRef Full Text | Google Scholar
Ree, R. H., and Smith, S. A. (2008). Maximum likelihood inference of geographic range evolution by dispersal, local extinction, and cladogenesis. Syst. Biol. 57, 4–14. doi: 10.1080/10635150701883881
Pubmed Abstract | Pubmed Full Text | CrossRef Full Text | Google Scholar
Reid, E. M., and Chandler, M. E. J. (1933). The London Clay flora. London: British Museum of Natural History.
Renner, S. S. (2004). Plant dispersal across the tropical Atlantic by wind and sea currents. Int. J. Plant. Sci. 165, S23–S33. doi: 10.1086/383334
Renner, S. S., Clausing, G., and Meyer, K. (2001). Historical biogeography of Melastomataceae: the roles of Tertiary migration and long-distance dispersal. Am. J. Bot. 88, 1290–1300. doi: 10.2307/3558340
Pubmed Abstract | Pubmed Full Text | CrossRef Full Text | Google Scholar
Richardson, J. M., Pennington, R. T., Pennington, T. D., and Hollingsworth, P. M. (2004). Rapid diversification of a species-rich genus of Neotropical rain forest trees. Science 293, 2242–2245. doi: 10.1126/science.1061421
Pubmed Abstract | Pubmed Full Text | CrossRef Full Text | Google Scholar
Sanderson, M. J. (2002). Estimating absolute rates of molecular evolution and divergence times: a penalized likelihood approach. Mol. Biol. Evol. 19, 101–109. doi: 10.1093/oxfordjournals.molbev.a003974
Pubmed Abstract | Pubmed Full Text | CrossRef Full Text | Google Scholar
Savolainen, V., Chase, M. W., Hoot, S. B., Morton, C. M., Soltis, D. E., Bayer, C., et al. (2000a). Phylogenetics of flowering plants based on combined analysis of plastid atpB and rbcL sequences. Syst. Biol. 49, 306–362. doi: 10.1093/sysbio/49.2.306
Pubmed Abstract | Pubmed Full Text | CrossRef Full Text | Google Scholar
Savolainen, V., Fay, M. F., Albach, D. C., Backlund, A., Van der Bank, M., Cameron, K. M., et al. (2000b). Phylogeny of the eudicots: a nearly complete familial analysis based on rbcL gene sequences. Kew Bull. 55, 257–309. doi: 10.2307/4115644
Seton, M., Müller, R. D., Zahirovic, S., Gaina, C., Torsvik, T., Shephard, G., et al. (2012). Global continental and ocean basin reconstructions since 200 Ma. Earth Sci. Rev. 113, 212–270. doi: 10.1016/j.earscirev.2012.03.002
Silvestro, D., Schnitzler, J., and Zizka, G. (2011). A Bayesian framework to estimate diversification rates and their variation through time and space. BMC Evol. Biol. 11:311. doi: 10.1186/1471-2148-11-311
Pubmed Abstract | Pubmed Full Text | CrossRef Full Text | Google Scholar
Stamatakis, A., and Aberer, A. J. (2013). “Novel parallelization schemes for large-scale likelihood-based phylogenetic inference,” in Proceedings of IEEE 27th International Symposium on Parallel and Distributed Processing (IPDPS) (Boston, MA), 1195–1204.
Talavera, G., and Castresana, J. (2007). Improvement of phylogenies after removing divergent and ambiguously aligned blocks from protein sequence alignments. Syst. Biol. 56, 564–577. doi: 10.1080/10635150701472164
Pubmed Abstract | Pubmed Full Text | CrossRef Full Text | Google Scholar
Taylor, D. W. (1990). Paleobiogeographic relationships of angiosperms from the cretaceous and early tertiary of the North American area. Bot. Rev. 56, 279–417. doi: 10.1007/BF02995927
Thulin, M., Beier, B. A., Razafimandimbison, S. G., and Banks, H. I. (2008). Ambilobea, a new genus from Madagascar, the position of Aucoumea, and comments on the tribal classification of the frankincense and myrrh family. Nord. J. Bot. 26, 218–229. doi: 10.1111/j.1756-1051.2008.00245.x
Weeks, A. (2003). The Molecular Systematics and Biogeography of the Burseraceae. Ph.D. dissertation, The University of Texas at Austin.
Weeks, A., Daly, D. C., and Simpson, B. B. (2005). Phylogenetic relationships and historical biogeography of the Burseraceae based on nuclear and chloroplast sequence data. Mol. Phylogenet. Evol. 35, 85–101. doi: 10.1016/j.ympev.2004.12.021
Weeks, A., and Simpson, B. B. (2007). Molecular phylogenetic analysis of Commiphora (Burseraceae) yields insight on the evolution and historical biogeography of an “impossible” genus. Mol. Phylogenet. Evol. 42, 62–79. doi: 10.1016/j.ympev.2006.06.015
Pubmed Abstract | Pubmed Full Text | CrossRef Full Text | Google Scholar
Wiens, J. J., and Donoghue, M. J. (2004). Historical biogeography, ecology, and species richness. Trends Ecol. Evol. 19, 639–644. doi: 10.1016/j.tree.2004.09.011
Pubmed Abstract | Pubmed Full Text | CrossRef Full Text | Google Scholar
Yi, T., Miller, A., and Wen, J. (2004). Phylogenetic and biogeographic diversification of Rhus (Anacardiaceae) in Northern Hemisphere. Mol. Phylogenet. Evol. 33, 861–879. doi: 10.1016/j.ympev.2004.07.006
Pubmed Abstract | Pubmed Full Text | CrossRef Full Text | Google Scholar
Zapata, F., and Fine, P. V. A. (2013). Diversification of the monoterpene synthase gene family (TPSb) in Protium, a highly diverse genus of tropical trees. Mol. Phylogenet. Evol. 68, 432–442. doi: 10.1016/j.ympev.2013.04.024
Pubmed Abstract | Pubmed Full Text | CrossRef Full Text | Google Scholar
Zerega, N. J. C., Clement, W. L., Datwyler, S. L., and Weiblen, G. D. (2005). Biogeography and divergence times in the mulberry family (Moraceae). Mol. Phylogenet. Evol. 37, 402–416. doi: 10.1016/j.ympev.2005.07.004
Pubmed Abstract | Pubmed Full Text | CrossRef Full Text | Google Scholar
Keywords: biogeography, biome shifts, continental vicariance, diversification, long-distance dispersal, phylogenetic niche conservatism
Citation: Weeks A, Zapata F, Pell SK, Daly DC, Mitchell JD and Fine PVA (2014) To move or to evolve: contrasting patterns of intercontinental connectivity and climatic niche evolution in “Terebinthaceae” (Anacardiaceae and Burseraceae). Front. Genet. 5:409. doi: 10.3389/fgene.2014.00409
Received: 01 July 2014; Accepted: 04 November 2014;
Published online: 28 November 2014.
Edited by:
Toby Pennington, Royal Botanic Garden Edinburgh, UKReviewed by:
Matthew T. Lavin, Montana State University, USAWolf L. Eiserhardt, Royal Botanic Gardens, Kew, UK
Copyright © 2014 Weeks, Zapata, Pell, Daly, Mitchell and Fine. This is an open-access article distributed under the terms of the Creative Commons Attribution License (CC BY). The use, distribution or reproduction in other forums is permitted, provided the original author(s) or licensor are credited and that the original publication in this journal is cited, in accordance with accepted academic practice. No use, distribution or reproduction is permitted which does not comply with these terms.
*Correspondence: Andrea Weeks, Department of Biology, George Mason University, 4400 University Drive, 3E1, Fairfax, VA 22030, USA e-mail:YXdlZWtzM0BnbXUuZWR1