- 1Division of Hematology-Oncology-Stem Cell Transplantation and Regenerative Medicine, Department of Pediatrics, Stanford Medical School, Palo Alto, CA, United States
- 2Center for Definitive and Curative Medicine, Stanford University School of Medicine, Palo Alto, CA, United States
Clustered regularly interspaced short palindromic repeats (CRISPR) genome editing platform heralds a new era of gene therapy. Innovative treatments for life-threatening monogenic diseases of the blood and immune system are transitioning from semi-random gene addition to precise modification of defective genes. As these therapies enter first-in-human clinical trials, their long-term safety and efficacy will inform the future generation of genome editing-based medicine. Here we discuss the significance of Inborn Errors of Immunity as disease prototypes for establishing and advancing precision medicine. We will review the feasibility of clustered regularly interspaced short palindromic repeats-based genome editing platforms to modify the DNA sequence of primary cells and describe two emerging genome editing approaches to treat RAG2 deficiency, a primary immunodeficiency, and FOXP3 deficiency, a primary immune regulatory disorder.
Introduction
Progress in developing new and curative therapies for rare monogenic diseases has dramatically improved in recent years. Specifically, in the past two decades, gene therapy-based precision medicine (Naldini, 2011; High and Roncarolo, 2019; Porteus, 2019) has shown remarkable promise and success in treating monogenic diseases in cases where the molecular aberrations leading to the disease pathology are defined. Among the ∼10,000 known rare monogenic diseases (Haendel et al., 2020), inborn errors of immunity (IEI), including Primary immunodeficiencies (PIDs) and Primary Immune Regulatory Disorders (PIRDs), are a growing class of rare diseases (Demirdag et al., 2021). PIDs and PIRDs affect 1:10,000 to 1,000,000 live births worldwide (Bousfiha et al., 2013; Tangye et al., 2020), with incidents as high as 1:500 in regions with consanguineous inheritance (Meshaal et al., 2019; Greenberg-Kushnir et al., 2020). The genetic cause of many PIDs (Notarangelo, 2010) and PIRDs is known, with testing and diagnosis being made available at birth. Early diagnosis is vital to enable management strategies, guide prognosis, and initiate life-saving treatments. As of 2022, there are 485 IEI (Tangye et al., 2022), each represented by unique gene mutations that partially or completely disrupt the normal development and function of the adaptive and/or innate immune system. Deep knowledge of IEI pathophysiology is necessary to establish safe and curative treatment approaches.
The decades-long effort to identify and modify pathogenic nucleotide variants in genomic DNA (Smithies et al., 1985; Rouet et al., 1994; Choulika et al., 1995; Jasin, 1996; Bibikova et al., 2003; Porteus and Baltimore, 2003; Porteus et al., 2003; Porteus and Carroll, 2005; Urnov et al., 2005; Grizot et al., 2009; Miller et al., 2011; Voit et al., 2014) has yielded a powerful technology—genome editing - based on inducing DNA double-stranded breaks (DSBs) or nicks to achieve defined genetic changes and restore normal gene function. The safety and efficacy of genome editing in human hematopoietic stem and progenitor cells (HSPCs) and T cells have been tested preclinically (Dever et al., 2016; Hubbard et al., 2016; Liang et al., 2017; Pavel-Dinu et al., 2019; Goodwin et al., 2020; Cromer et al., 2021; Gray et al., 2021; Sweeney et al., 2021; Vavassori et al., 2021; Wilkinson et al., 2021; Iancu et al., 2023) with some approved by Food and Drug Administration (FDA) for Phase 1 clinical trials in indications such as hemoglobinopathies (Porteus et al., 2022), congenital blindness (Maeder et al., 2019), and hearing loss (Botto et al., 2021). The therapeutic application of genome editing technology to correct gene dysfunction in IEIs has gained momentum, mainly due to the ability to preserve critical endogenous expression and regulatory features, which are indispensable for proper immune development and function.
This review focuses on the Clustered Regulatory Interspaced Short Palindromic Repeats (CRISPR) Cas9 nuclease-based genome editing toolbox and describes their applications in correcting genes regulating the immune system. We discuss the pre-clinical development of CRISPR/Cas9-based therapies for RAG2-SCID, a PID, and IPEX, a PIRD, which could benefit from genome editing to restore optimal immune function.
Genome editing technology emerges as a therapeutic tool
Innovations in DNA sequencing technology combined with an understanding of how to stimulate and modulate DNA repair to levels compatible with clinical applications (Lattanzi et al., 2021), set the foundation for genome editing. Genome editing is the process of engineering changes in the DNA sequence of a cell. The modification ranges from a single nucleotide to inserting a full-length complementary DNA (cDNA) (Porteus, 2019). Significant advancements in genome editing came from discovering CRISPR/Cas9, a bacterial nuclease used as a defense mechanism against invading parasites (Gasiunas et al., 2012; Jinek et al., 2012). By designing a chemically modified 20-nucleotide RNA guide (Hendel et al., 2015) (single guide RNA; sgRNA) complementary to a pre-selected DNA sequence in the human genome (Mali et al., 2013), CRISPR/Cas9-sgRNA can now be programmed to recognize and induce DSBs, at most genomic locations, an event that recruits DNA repair machinery and prompts DNA sequence modifications (Yeh et al., 2019). The versatility (Gaudelli et al., 2017; Kim et al., 2017; Komor et al., 2017; Bak et al., 2018; Koblan et al., 2018; Rees and Liu, 2018; Anzalone et al., 2019; Jeong et al., 2020; Sakata et al., 2020; Sato et al., 2020; Zeng et al., 2020; Anzalone et al., 2022), efficacy (Genovese et al., 2014; Hoban et al., 2015; Dever et al., 2016; Schiroli et al., 2017; Kuo et al., 2018; Pavel-Dinu et al., 2019; Roman-Rodriguez et al., 2019; Goodwin et al., 2020; Rai et al., 2020; Cromer et al., 2021; De Ravin et al., 2021; Sweeney et al., 2021; Iancu et al., 2023) and specificity (Vakulskas et al., 2018) of the CRISPR/Cas9-sgRNA-based genome editing platforms have revolutionized our ability to recognize and correct disease-causing variants directly in the genome of primary human cells.
Genome editing with a double strand break
Genome correction outcomes vary depending on the DNA template and repair pathway used to fix the Cas9-sgRNA-induced DSBs (Xue and Greene, 2021). In dividing cells, where homologous repair (HR) and the non-homologous end joining (NHEJ) DNA repair proteins are expressed, there are two primary editing outcomes (Figure 1A). When an exogenous DNA repair template carrying the desired DNA sequence modification is flanked by homology arms complementary to the DSB site, it acts as a template for HR. This corrective cassette is often delivered by an adeno-associated virus (AAV) with serotypes specific to the cell type of interest (e.g., AAV6 for HSPCs and T cells). The CRISPR/Cas9-AAV6 strategy was used to restore the endogenous expression of a mutated gene by inserting a therapeutic cassette that delivers a copy of a sequence (up to 4.7 kb) coding for a functional protein (Genovese et al., 2014; Hubbard et al., 2016; Schiroli et al., 2017; Pavel-Dinu et al., 2019; Goodwin et al., 2020; Cromer et al., 2021; Sweeney et al., 2021; Pavel-Dinu et al., 2022; Iancu et al., 2023).
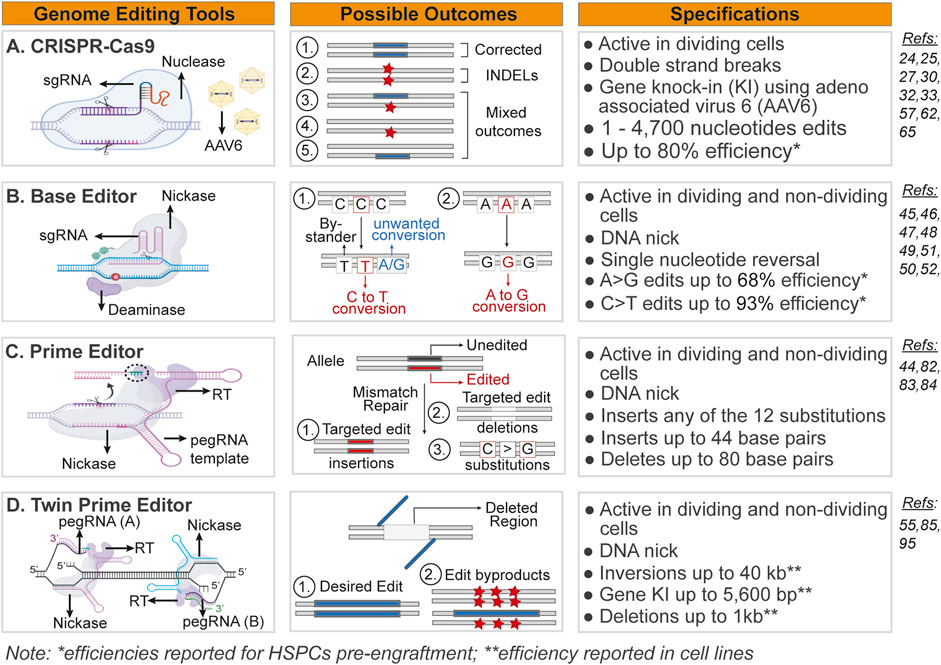
FIGURE 1. Overview of genome editing platforms discussed in this review. Schematics and description of the genome editing tools, genomic outcomes following editing and specifications for (A) CRISPR/Cas9-AAV6, (B) Base Editing (BE), (C) Prime Editing (PE), and (D) Twin Prime Editing platforms. sgRNA, single guide RNA; Nuclease, Cas9; Nickase, an engineered Cas9 nuclease with a single-stranded “nick” DNA cutting activity; AAV6, adeno-associated virus of serotype 6; INDELs, insertion and deletions; pegRNA, prime editing guide RNA; RT, reverse transcriptase; HSPCs, hematopoietic stem and progenitor cells.
In the absence of an exogenous HR-dependent corrective template, the repeated cycles of CRISPR/Cas9-sgRNA cutting coupled with NHEJ repair lead to nucleotide insertions and deletions (INDELs) at the site of repair (Rose et al., 2017) that likely disrupts the expression of the gene, generating a knockout (KO). Depending on the gene’s function, a KO allele could be therapeutic if it disrupts a dominant negative function (e.g., the STING gene in SAVI disease) (Fremond et al., 2021). However, it could also pose a therapeutic risk if the presence of INDELs induces a secondary disease (e.g., HBB gene and B-thalassemia) (Cromer et al., 2021). The limit of HR-based genome editing with AAV6 is up to 4,700 nucleotides, making it a popular correction approach for genes carrying hundreds of pathogenic variants spread throughout the coding sequence (Hirsch, 2015). However, this approach has several limitations. First, the process requires dividing cells, limiting the cell types amenable to such a genome editing approach (Xue and Greene, 2021). Second, delivery of the donor template to target cells depends on the availability of a viral vector with a serotype permissible to the modified cells. Third, a viral vector could trigger an immune response that could partially reduce the health of the cells being edited (Cromer et al., 2018; Schiroli et al., 2019). New technologies using viral vector-free delivery of the donor DNA template are being developed and hold promises (Shy et al., 2022).
Despite no major safety concerns have emerged in preclinical in vitro and in vivo studies, DNA editing with CRISPR/Cas9-AAV6 requires a careful evaluation of possible off-target sites, especially when those sites are present within genes affecting hematopoiesis. Currently, this evaluation is performed by a combination of methods, including in silico prediction and deep sequencing of in vitro edited healthy donors’ and patients’ derived HSPCs. Recent advancements in CRISPR/Cas9 technology have generated a new version of the Cas9 nuclease with significantly improved fidelity (Vakulskas et al., 2018), which has reduced and, in many cases, almost eliminated off-target site nuclease activity (Gomez-Ospina et al., 2019; Pavel-Dinu et al., 2019).
In addition to the unintended cutting activity, genotoxicity can be caused by chromosomal aberration typically induced by two or more guides or by using a single guide with additional homologous cut sites in the genome. However, in preclinical studies where the correction of a single defective gene relies on a single guide to introduce the desired genomic modification, the likelihood of introducing chromosomal aberrations is significantly reduced (Lattanzi et al., 2021; Cromer et al., 2022). To advance the preclinical studies for in-human-testing rigorous analyzes of the potential structural aberrations should therefore be performed using up-to-date technologies (Turchiano et al., 2021; Park et al., 2022).
The ex vivo editing of human HSPCs has been shown to trigger cellular response to CRISPR/Cas9-induced double-strand breaks and elicit an immune response to AAV6 containing a homologous template (Cromer et al., 2018; Allen et al., 2022; Ferrari et al., 2022). These responses could affect the stemness of the interventional products, especially when using patients’ HSPCs that have been exposed to prolonged inflammation in vivo. The preclinical studies using primary and secondary transplants in humanized mice show that edited HSPCs can sustain long-term engraftment and multilineage differentiation potential (Pavel-Dinu et al., 2019; Goodwin et al., 2020; Scharenberg et al., 2020), however, the frequency of edited HSPCs that persist long-term in humanized mice is diminished (Pavel-Dinu et al., 2019; Scharenberg et al., 2020). Depending on the disease indication (e.g., conditions where corrected HSPCs have a survival advantage over non-corrected ones) this decrease can still translate into a clinical outcome of disease correction. In other indications, the level of gene correction and frequency of edited HSPCs that need to persist long-term in the graft might need to be higher.
AAV6 delivery of the therapeutic cassette remains a promising and efficient approach. However, further improvements are necessary to reduce or eliminate potential toxicity from AAV6 exposure, an area of ongoing investigation (Scala et al., 2018; Ferrari et al., 2020; Sharma et al., 2021). In addition to AAV6, other viral delivery systems such as integrase-defective lentiviral vectors may provide certain benefits and be used for disease applications (Ferrari et al., 2022).
To address these limitations, increase the homogeneity of the genome editing outcomes and ensure the highest ratio of HR to INDELs, the genome editing toolbox has expanded to include new innovative tools—Base Editing (BE) (Komor et al., 2016; Jeong et al., 2020; Zeng et al., 2020), Prime Editing (PE) (Urnov, 2020; Nelson et al., 2022), and Twin Prime Editing (twinPE) (Anzalone et al., 2022; Choi et al., 2022) - that are active in non-dividing cells and are not reliant on double-strand breaks.
Genome editing with a nick
Cas9 is a versatile nuclease: it can be engineered to introduce double-strand or single-strand nicks. It can also ferry protein subunits with gene regulatory functions (Qi et al., 2013; Gilbert et al., 2014; Anzalone et al., 2019; Pickar-Oliver and Gersbach, 2019). Three new genome editing platforms were recently engineered as protein chimeras by fusing the Cas9 nuclease to a deaminase, dubbed as Base Editing (BE), or linked to reverse transcriptase (RT), generating the Prime Editing (PE) and Twin Primer Editing (twin PE) strategies.
These newly developed genome editing platforms were designed to precisely edit DNA sequences independent of cell cycle status and without inducing DSBs in the mammalian genome. BE platform (Komor et al., 2016) can correct pathogenic variants and inactivate genes or cis-regulatory elements in HSPCs. Conversion of a C:G to a T:A base pair using cytosine BE (CBE) (Sakata et al., 2020) or the alternative reaction, T:A to C:G using adenine BE (ABE) (Gaudelli et al., 2017), is achieved using a catalytically dead or nickase Cas9-sgRNA complex fused to a deaminase (Figure 1B). Following DNA unwinding and complementary base pairing between the sgRNA and the targeted DNA sequence, the deaminase converts the targeted base located at a distance from protospacer (PAM): cytosine to uracil or adenosine to inosine, resulting in a C to T or an A to G change, respectively. Four generations of BE have been created to increase efficiency and improve safety (Komor et al., 2016; Komor et al., 2017). Further advancements have been implemented to broaden the targeting range using nickase Cas9 and deaminase variants (Gehrke et al., 2018), to recognize different protospacer adjacent motifs (PAM) (Hu et al., 2018; Nishimasu et al., 2018) or non-NGG PAMs (Chatterjee et al., 2018), and edit previously inaccessible genomic regions (e.g., methylated regions) (Wang et al., 2018), respectively.
Unlike BE platform, which allows for A>G, C>T, and C>G substitutions, PE was developed to ensure that all 12 possible base conversions—transversions and transitions—can be created. It is based on chimeric Cas9 nickase fused to reverse transcriptase (RT). It uses prime editing guide RNA (pegRNA) to serve as a sgRNA and a template for genomic modification without requiring a PAM sequence to mark the target site (Anzalone et al., 2019). The pegRNA moiety hybridizes with the nicked DNA strand, which acts as a primer for the RT reaction. The newly synthesized 3’ end of the DNA containing the desired edit invades the nearby DNA strand and primes the non-edited strand, thus introducing the desired DNA change in both strands (Figure 1C). The final products of PE-based genome editing range from single nucleotide changes to insertions (up to 44 base pairs) and deletions (up to 80 base pairs) at 5–50 base pairs away from the nick site in non-dividing cells (Anzalone et al., 2019). Although PE cannot modify thousands of nucleotides like a DBS-induced HR-based genome editing platform, it can correct an exon of a gene. We refer to the following reviews for a detailed discussion of the BE and PE mechanisms (Antoniou et al., 2021; Porteus et al., 2022).
TwinPE platform enables targeted integration of gene-size DNA fragments (Anzalone et al., 2022). Like, PE, twinPE uses a catalytically impaired Cas9 nickase. TwinPE has evolved to use two nickases, two RT enzymes, and two pegRNAs to specify the targeted genomic sequence and insert the desired edit (Figure 1D). Variations of this platform can generate sequence inversions and large knock-in (KI) (Anzalone et al., 2022; Choi et al., 2022; Zhuang et al., 2022). However, the latter is less effective than the CRISPR-Cas9-AAV6 platform.
In the long term, these innovations to the genome editing toolbox will expand the therapeutic options available to patients. Although, pre-clinical studies and further developments are still required to characterize the safety and efficacy of these platforms in primary cells, these new technologies hold great promises for advancing medicine.
Applying DSB-based genome editing technology for correcting IEIs
Genome editing for RAG2-deficiency, a primary immunodeficiency
The Recombinase Activating Genes 1 and 2 (RAG1 and RAG2) are the natural genome-editing nucleases (Alt et al., 1992), whose activity is restricted to the lymphoid lineage and the G0/G1 cell cycle phase to prevent the risk of genotoxicity from promiscuous nuclease activity (Miyazaki and Miyazaki, 2021). Adaptive immunity depends on the function of these two nucleases to promote naturally occurring genome editing events necessary for normal development and the function of B and T cells. Through a process involving DNA cleavage and NHEJ-dependent DNA repair, the Variable (V), Diversity (D), and Joining (J) coding elements at the T cell receptor (TCR) and B cell receptor (BCR) loci rearrange to produce functional cell surface receptors. Productive and diverse rearrangements of the V-(D)-J give rise to functional antigen-specific receptors that ensure proper signals are received by T and B cells, respectively. These signals are required for normal development, elimination of lymphocytes reactive to self-antigens, and lymphocyte homeostasis. A diverse receptor repertoire also ensures that lymphocytes recognize a broad range of antigens and mount a normal immune response (Notarangelo et al., 2016). Over 200 unique pathogenic mutations (Corneo et al., 2001) are known to disrupt RAG1/2 proteins function resulting in RAG deficiency (Notarangelo et al., 2016; Tirosh et al., 2019), a PID with an incidence rate of 1:250,000 (Kwan et al., 2013; Dvorak et al., 2019). RAG1/2-deficient patients present with a broad spectrum of clinical manifestations that range from severe combined immunodeficiency (SCID) caused by biallelic null mutations (<5% recombinase activity) to atypical SCID (AS), delayed-onset combined immunodeficiency with granulomas and/or autoimmunity (CID-G/AI) and Omenn Syndrome (OS), and the latter being OS is caused by hypomorphic RAG variants that support residual (5%–30%) recombinase activity (Villa et al., 2001; Delmonte et al., 2018; Villa and Notarangelo, 2019; Delmonte et al., 2020).
Currently, the only definitive therapy for patients with RAG1/2 deficiency is allogeneic hematopoietic stem cell transplantation (allo-HSCT), with survival rates as high as 90% for SCID patients when using HLA-matched donors (Castagnoli et al., 2019). For RAG1/2-OS, due to the severity exacerbated by the autoimmune manifestations, significant limitations are associated with allo-HSCT (Mazzolari et al., 2005). RAG1/2 patients with AS or OS forms have a worse survival outcome than the typical SCID (Villa et al., 1999; Mazzolari et al., 2005).
Pre-clinical studies using Rag1 (Pike-Overzet et al., 2011; Pike-Overzet et al., 2014; van Til et al., 2014) or Rag2 (van Til et al., 2012) deficient mice models have shown variable therapeutic efficacy of the lentivirus (LV)-based gene addition approach in the HSPCs. For Rag1, the lymphoid lineage was only partially rescued by LV addition of a RAG1 cDNA, with some mice showing signs of inflammation resembling OS (Pike-Overzet et al., 2011; van Til et al., 2014). For Rag2, the therapeutic efficacy of LV-based delivery of a codon-optimized (coRAG2) cDNA was more substantial but incomplete, with B cell immunity only partially restored (van Til et al., 2012). These findings suggest that autologous transplantation of LV-modified HSPCs overexpressing RAG1/2 cDNAs might not recapitulate the endogenous levels of regulations and expression necessary to support healthy immune system development and function (van Til et al., 2012; van Til et al., 2014). In addition, ectopic expression of RAG1/2 driven by exogenous promoters could lead to uncontrolled nuclease activity and translocation events. Results from an ongoing clinical trial for RAG1 deficiency (NCT04797260) will offer more insight into the safety and efficacy of LV-based semi-random insertion of a nuclease into human stem cells’ genome.
Autologous transplantation of RAG1/2-deficient gene-corrected HSPCs could offer several benefits over the semi-random LV-gene addition strategy, including: preservation of endogenous regulation and expression, restriction of gene functions to the lymphoid lineage and the G0/G1 cell cycle phase, and avoiding the genotoxic risk from ectopic RAG1/2 activity.
We have recently developed and tested a genome editing approach to correct RAG2 deficiency. In the first study (Gardner et al., 2021), we used induced pluripotent stem cells (iPSCs) and the CRISPR/Cas9-AAV6 platform to correct a RAG2-SCID mutation (c.831T>A) by inserting a functional codon-optimized (co) RAG2 cDNA. Using an artificial thymic organoid (ATO) system that supports only in vitro T-cell differentiation, we showed that, unlike the RAG2null iPSCs, RAG2-corrected iPSCs demonstrated correction of the V(D)J recombination, thus overcoming the T-lymphocyte developmental blockage and differentiating into CD3+TCRαβ+ CD4/CD8 single positive lymphocytes, at levels comparable to healthy donor iPSCs. TCR sequencing of the derived T cells confirmed a polyclonal pattern of TCR alpha and beta chain rearrangement.
In a more recent publication (Iancu et al., 2023), another in vitro RAG2-SCID disease model was established. In this model, the RAG2 gene was KO in human CD34+ cells using CRISPR/Cas9-AAV6 platform. The RAG2 KO and control CD34+ cells were differentiated into T cells in vitro. The RAG2-deficient CD34+ cells failed to differentiate into CD3+ T cells, thus recapitulating the defect in RAG2-SCID patients. A very similar genome editing approach to ours for the correction of the RAG2 gene was used. In this approach, a full-length codon-optimized RAG2 cDNA was inserted at exon 1 of the endogenous RAG2 gene, but unlike ours, it included a marker gene (NGFR) downstream of the therapeutic cassette, under the control of a constitutive promoter, allowing the selection of the edited cells. The resulting edited NGFR-purified CD34+ cells from healthy donors differentiate into CD3+ T cells with oligoclonal TCR repertoire diversity comparable to the controls. Importantly, similar results were obtained using RAG2-SCID patients’ CD34+ cells. Collectively, both in vitro studies support the feasibility of the CRISPR/Cas9-AAV6 editing platform to correct the RAG2-SCID.
We extended our studies in vivo (Pavel-Dinu et al., 2022), using healthy donors and patient-derived stem cells, to assess if the V(D)J activity can be reinstated in support of normal lymphoid lineage development (B cells in addition to T cells). Using a CRISPR/Cas9-AAV6 platform, we report 85% of alleles being genome-edited, of which 50% carried INDELs, and 30% were HR-modified. Since allo-HSCT studies show that a minimum of 20% donor chimerism is necessary to achieve clinical correction in RAG-SCID patients (Mazzolari et al., 2005), our reported levels of genomic modification surpassed the requirement for attaining clinically relevant levels of correction.
We further showed that the RAG2-corrected HSPCs derived from healthy donors and one RAG2-SCID patient could sustain long-term engraftment and multi-lineage differentiation following transplantation into immunodeficient NSG-SGM3 mice. Compared with uncorrected HSPCs, the patient-derived RAG2-corrected and engrafted HSPCs restored B cell development generating immunoglobulins M (IgM) expressing all 7-immunoglobulin heavy chain Variable gene families. We further detected T cells (CD3+, CD4+, CD8+) in the bone marrow and spleen of mice engrafted with RAG2-corrected HSPCs. The T cell receptors of patient-derived RAG2-corrected HSPCs showed comparable oligoclonal rearrangement patterns at the TRA/TRD locus compared to healthy donors and a similar pattern in TRAV and TRAJ gene usage.
Lastly, in vivo studies showed that the genome correction strategy shifted the predominant NK population from immature to mature natural killer (NK) cells phenotype. The immature NK cell phenotype was recently reported in patients with SCID caused by RAG1/2 (Karo et al., 2014) and NHEJ deficiency (Dobbs et al., 2017). This is important to note because, in the absence of conditioning, this immature NK population with known heightened cytotoxic activity can attack the graft following allo-HSCT (Schuetz et al., 2014).
This is the first in vivo study that showed genome editing-based correction of V(D)J recombinase results in phenotypic correction of the lymphoid lineage defect in a typical RAG2-SCID patient-derived HSPCs, using the CRISPR/Cas9-AAV6 platform. This work provides the rationale for further development and testing of this strategy in the treatment and characterization of the autoimmune manifestations in RAG2-deficiency. The existing in vivo murine models of Rag2 deficiencies (Mombaerts et al., 1992; Shinkai et al., 1992; Khiong et al., 2007; Marrella et al., 2007; Giblin et al., 2009; Ott de Bruin et al., 2016; Ott de Bruin et al., 2018) offer an optimal platform to assess the extent of the disease correction and safety, as a necessary step for advancing this approach to in human testing.
Genome editing for FOXP3-deficiency, a primary immune regulatory disorder
Immune dysregulation polyendocrinopathy enteropathy X linked (IPEX) syndrome is a prototype monogenic autoimmune disease or PIRD caused by loss of regulatory T cell (Treg) function. IPEX syndrome is due to mutations in the FOXP3 gene, a critical transcriptional factor that regulates and maintains the suppressive function of Treg cells (Powell et al., 1982; Bennett et al., 2001; Wildin et al., 2001). Over 200 pathogenic variants span both protein-coding and non-coding regions, though usually not abrogating FOXP3 protein expression (Barzaghi et al., 2018; Gambineri et al., 2018). Loss of Treg function due to FOXP3 mutations leads to the loss of peripheral self-tolerance, expansion of autoreactive T cells, and the development of multiorgan autoimmunity (Bennett et al., 2001; Wildin et al., 2001; Fontenot et al., 2003; Borna et al., 2022a). IPEX typically manifests within the first year of life with type 1 diabetes, enteropathy, and eczema (Barzaghi et al., 2018; Gambineri et al., 2018). However, atypical forms of IPEX, including lupus-like manifestations or neuromuscular impairment, are becoming more frequently reported (Consonni et al., 2021; Rim et al., 2021). Current treatment options, including pharmacological immunosuppression and allogenic-HSCT, are only partially beneficial with limited outcomes (Barzaghi et al., 2018; Gambineri et al., 2018). Therefore, gene therapy is a great promise to address this unmet medical need.
For an overview of the various gene therapy approaches to treat IPEX, please see our recent review (Borna et al., 2022b) and the open clinical trial (NCT05241444). Here, we focus on the CRISPR/Cas9 gene editing approach in HSPC. Like the RAG2 gene, FOXP3 gene expression and functions are tightly and differentially regulated in specific subtypes of CD4+ T cells. The most well-described regulatory components of the FOXP3 gene are three conserved non-coding DNA sequence (CNS) elements. CNS1 plays a role in the generation of Treg cells from effector T cells (Teff) in the periphery (Zheng et al., 2010). CNS2 contains a Treg-specific demethylation region (TSDR). The TSDR is demethylated only in Treg, to support high and stable FOXP3 expression (Floess et al., 2007; Polansky et al., 2008; Kressler et al., 2020). The TSDR is fully methylated in Teff, where FOXP3 expression is upregulated only transiently in response to TCR stimulation (Baron et al., 2007). Lastly, CNS3 is a pioneering element with a critical role in the induction of FOXP3 expression during Treg development (Zheng et al., 2010). The optimal FOXP3 editing strategy in HSPCs faces several challenges (Figure 2). It must preserve these regulatory mechanisms to achieve 1) physiologically high expression of the FOXP3 in Treg, 2) transient FOXP3 expression in Teff upon TCR stimulation, and 3) prevent ectopic FOXP3 expression in other leukocyte subsets.
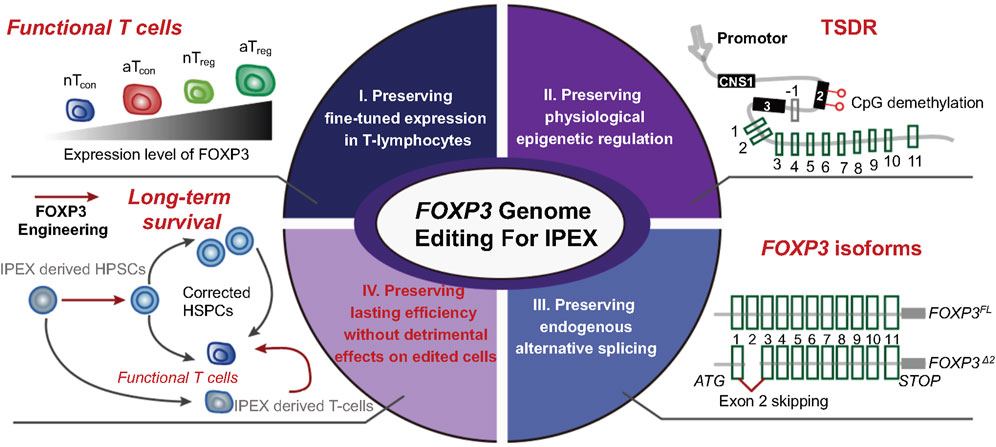
FIGURE 2. Challenges of FOXP3 genome editing in HSPCs for therapeutic purposes. Schematic representation of various features of the FOXP3 gene, which need to be preserved to achieve therapeutic safety and efficacy. nT, natural occurring regulatory or conventional T cells, aT, activated regulatory or conventional T cells, TSDR, Treg specific demethylated region, CNS, conserved non-coding sequence elements. Inspired from Figure 2, Porteus et al. (2022).
The consequence of constitutive and ectopic FOXP3 expression outside the Treg cell compartment is well documented, using humanized mice. The immunodeficient mice were reconstituted with HSPCs transduced with a LV construct encoding a FOXP3 cDNA under the control of a constitutive promoter. In these mice, the human-derived T cells were hyporesponsive to TCR stimulation suggesting that ectopic FOXP3 expression may result in immunodeficiency (Santoni de Sio et al., 2017). Recently, we published a genome editing strategy to restore the expression of the FOXP3 gene (Goodwin et al., 2020). Using CRISPR/Cas9-AAV6 platform, a cDNA coding for full-length FOXP3 was inserted upstream of exon 1 of the endogenous FOXP3 gene. The construct also contained an exogenous 3-phosphoglycerate kinase (PGK) promoter to drive the expression of a truncated form of Nerve Growth Factor Receptor (
The FOXP3 edited HSPCs engrafted into immunodeficient mice demonstrated preserved multilineage differentiation potential. However, the number of Treg in the spleen of these mice was significantly reduced, indicating that further optimization of the gene editing strategy is needed before going into the clinic. The reduced Treg cell numbers in vivo, in the humanized mice, and the reduced suppressive capacity of the edited Treg in vitro may be due to the lack of the additional FOXP3 splice isoforms physiologically expressed in Treg. The alternative splicing of the endogenous FOXP3 transcript gives rise to several splice isoforms. Of those, the full-length and the isoform lacking exon 2 (
Conclusion
Clinical application of genome editing is already a reality with different indications in medicine. Several approaches are in the clinic in oncology, mainly in ex vivo generated CAR-T. HR-mediated genome editing is a promising treatment for monogenic diseases of the blood and immune system. Ongoing trials for sickle cell and thalassemia begin to generate essential data for improving the current editing protocols. GraphiteBio gave a recent press release on the slow engraftment of the gene-corrected product in the first patient treated as part of the CEDAR clinical trial NCT04819841. The field looks forward to more details behind this report so that a better understanding and potential solutions might be developed.
A combination of newborn screening and genome editing in autologous HSPCs could provide a one-time definitive therapy for several IEIs. Moreover, autoimmunity and inflammation could also benefit from the knock-in genome editing strategy to modulate regulatory genes (e.g., FOXP3) in specific cell subsets (e.g., conventional T cells) (Honaker et al., 2020). In addition, new approaches are being developed to eliminate and change the TCR specificity of a T cell product to modulate immune responses (CAR-Treg genome editing).
The editing platforms will continue to advance, allowing simultaneous editing of multiple genes, as in the case of patients diagnosed with multiple Variants of Unknown Significance (VUS).
Optimizing DSB-independent and viral-free genome editing platforms could be a significant step forward for IEIs. Overall, the CRISPR/Cas9-based editing technology provides hope for a new generation of precision medicine treatments over a wide range of indications, provided it is ethically performed to overcome incurable diseases. The big challenge for the future will be to make these therapies accessible to all patients in need (Nature Medicine, 2021).
Author contributions
MP-D wrote the sections on genome editing platforms, RAG2 deficiency, and made Figure 1. SB wrote the section on FOXP3 gene editing. RB lead the work and conceptualized Figure 2. All authors reviewed the manuscript and provided comments.
Funding
The in vivo RAG2-SCID study was supported by the Primary Immunodeficiency Treatment Consortium (PIDTC) training grant U54AI082973 awarded to MP-D, by the Amon Carter Foundation and the Lacob Endowment, awarded to Dr. Matthew Porteus and by the Division of Intramural Research (NIH/NIAID) awarded to Dr. Luigi Notarangelo. The IPEX editing study was supported by: Dr. Bacchetta, MD, has an Endowed Anne T. and Robert M. Bass Faculty Scholar in Pediatric Cancer and Blood Diseases, Stanford University School of Medicine; Simon Borna, PhD, has a Stanford Maternal and Child Health Research Institute (MCHRI) Postdoctoral Grant. In addition, this work was supported by the Center for Genetic Immune Diseases (CGID), the Center for Definitive and Curative Medicine (CDCM) at Stanford University, and by the California Institute for Regenerative Medicine (CIRM) through several grants to RB.
Acknowledgments
We gratefully acknowledge Dr. Rhonda Perriman for thoughtful feedback and revision of the manuscript. We acknowledge BioRender graphic software for high-quality schematics. We acknowledge Dr. Bing Wang for generating Figure 2. We acknowledge Stanford’s Binns Program for Cord Blood Research for providing cell products for our research. We are thankful to patients and their families for contributing to advance rare disease research and treatment.
Conflict of interest
The authors declare that the research was conducted in the absence of any commercial or financial relationships that could be construed as a potential conflict of interest.
Publisher’s note
All claims expressed in this article are solely those of the authors and do not necessarily represent those of their affiliated organizations, or those of the publisher, the editors and the reviewers. Any product that may be evaluated in this article, or claim that may be made by its manufacturer, is not guaranteed or endorsed by the publisher.
References
Allen, D., Weiss, L. E., Saguy, A., Rosenberg, M., Iancu, O., Matalon, O., et al. (2022). High-throughput imaging of CRISPR- and recombinant adeno-associated virus-induced DNA damage response in human hematopoietic stem and progenitor cells. CRISPR J. 5, 80–94. doi:10.1089/crispr.2021.0128
Alt, F. W., Rathbun, G., Oltz, E., Taccioli, G., and Shinkai, Y. (1992). Function and control of recombination-activating gene activity. Ann. N. Y. Acad. Sci. 651, 277–294. doi:10.1111/j.1749-6632.1992.tb24626.x
Antoniou, P., Miccio, A., and Brusson, M. (2021). Base and prime editing technologies for blood disorders. Front. Genome Ed. 3, 618406. doi:10.3389/fgeed.2021.618406
Anzalone, A. V., Gao, X. D., Podracky, C. J., Nelson, A. T., Koblan, L. W., Raguram, A., et al. (2022). Programmable deletion, replacement, integration and inversion of large DNA sequences with twin prime editing. Nat. Biotechnol. 40, 731–740. doi:10.1038/s41587-021-01133-w
Anzalone, A. V., Randolph, P. B., Davis, J. R., Sousa, A. A., Koblan, L. W., Levy, J. M., et al. (2019). Search-and-replace genome editing without double-strand breaks or donor DNA. Nature 576, 149–157. doi:10.1038/s41586-019-1711-4
Bak, R. O., Dever, D. P., and Porteus, M. H. (2018). CRISPR/Cas9 genome editing in human hematopoietic stem cells. Nat. Protoc. 13, 358–376. doi:10.1038/nprot.2017.143
Baron, U., Floess, S., Wieczorek, G., Baumann, K., Grutzkau, A., Dong, J., et al. (2007). DNA demethylation in the human FOXP3 locus discriminates regulatory T cells from activated FOXP3(+) conventional T cells. Eur. J. Immunol. 37, 2378–2389. doi:10.1002/eji.200737594
Barzaghi, F., Amaya Hernandez, L. C., Neven, B., Ricci, S., Kucuk, Z. Y., Bleesing, J. J., et al. (2018). Long-term follow-up of IPEX syndrome patients after different therapeutic strategies: An international multicenter retrospective study. J. Allergy Clin. Immunol. 141, 1036–1049.e5. doi:10.1016/j.jaci.2017.10.041
Bennett, C. L., Christie, J., Ramsdell, F., Brunkow, M. E., Ferguson, P. J., WhiteseLL, L., et al. (2001). The immune dysregulation, polyendocrinopathy, enteropathy, X-linked syndrome (IPEX) is caused by mutations of FOXP3. Nat. Genet. 27, 20–21. doi:10.1038/83713
Bibikova, M., Beumer, K., Trautman, J. K., and Carroll, D. (2003). Enhancing gene targeting with designed zinc finger nucleases. Science 300, 764. doi:10.1126/science.1079512
Borna, S., Lee, E., and Lakshmanan, U. (2022). Loss of FOXP3 function causes expansion of two pools of autoreactive T cells in patients with IPEX syndrome. bioRxiv. doi:10.1101/2022.07.10.499494
Borna, S., Lee, E., Sato, Y., and Bacchetta, R. (2022). Towards gene therapy for IPEX syndrome. Eur. J. Immunol. 52, 705–716. doi:10.1002/eji.202149210
Botto, C., Dalkara, D., and El-Amraoui, A. (2021). Progress in gene editing tools and their potential for correcting mutations underlying hearing and vision loss. Front. Genome Ed. 3, 737632. doi:10.3389/fgeed.2021.737632
Bousfiha, A. A., Jeddane, L., Ailal, F., Benhsaien, I., Mahlaoui, N., Casanova, J. L., et al. (2013). Primary immunodeficiency diseases worldwide: More common than generally thought. J. Clin. Immunol. 33, 1–7. doi:10.1007/s10875-012-9751-7
Castagnoli, R., Delmonte, O. M., Calzoni, E., and Notarangelo, L. D. (2019). Hematopoietic stem cell transplantation in primary immunodeficiency diseases: Current status and future perspectives. Front. Pediatr. 7, 295. doi:10.3389/fped.2019.00295
Chatterjee, P., Jakimo, N., and Jacobson, J. M. (2018). Minimal PAM specificity of a highly similar SpCas9 ortholog. Sci. Adv. 4, eaau0766. doi:10.1126/sciadv.aau0766
Choi, J., Chen, W., Suiter, C. C., Lee, C., Chardon, F. M., Yang, W., et al. (2022). Precise genomic deletions using paired prime editing. Nat. Biotechnol. 40, 218–226. doi:10.1038/s41587-021-01025-z
Choulika, A., Perrin, A., Dujon, B., and Nicolas, J. F. (1995). Induction of homologous recombination in mammalian chromosomes by using the I-SceI system of Saccharomyces cerevisiae. Mol. Cell Biol. 15, 1968–1973. doi:10.1128/mcb.15.4.1968
Consonni, F., Ciullini Mannurita, S., and Gambineri, E. (2021). Atypical presentations of IPEX: Expect the unexpected. Front. Pediatr. 9, 643094. doi:10.3389/fped.2021.643094
Corneo, B., Moshous, D., Gungor, T., Wulffraat, N., PhiliPPet, P., Le Deist, F. L., et al. (2001). Identical mutations in RAG1 or RAG2 genes leading to defective V(D)J recombinase activity can cause either T-B-severe combined immune deficiency or Omenn syndrome. Blood 97, 2772–2776. doi:10.1182/blood.v97.9.2772
Cromer, M. K., Barsan, V. V., Jaeger, E., Wang, M., Hampton, J. P., Chen, F., et al. (2022). Ultra-deep sequencing validates safety of CRISPR/Cas9 genome editing in human hematopoietic stem and progenitor cells. Nat. Commun. 13, 4724. doi:10.1038/s41467-022-32233-z
Cromer, M. K., Camarena, J., Martin, R. M., Lesch, B. J., Vakulskas, C. A., Bode, N. M., et al. (2021). Gene replacement of alpha-globin with beta-globin restores hemoglobin balance in beta-thalassemia-derived hematopoietic stem and progenitor cells. Nat. Med. 27, 677–687. doi:10.1038/s41591-021-01284-y
Cromer, M. K., Vaidyanathan, S., Ryan, D. E., Curry, B., Lucas, A. B., Camarena, J., et al. (2018). Global transcriptional response to CRISPR/Cas9-AAV6-Based genome editing in CD34(+) hematopoietic stem and progenitor cells. Mol. Ther. 26, 2431–2442. doi:10.1016/j.ymthe.2018.06.002
De Ravin, S. S., Brault, J., Meis, R. J., Liu, S., Li, L., Pavel-Dinu, M., et al. (2021). Enhanced homology-directed repair for highly efficient gene editing in hematopoietic stem/progenitor cells. Blood 137, 2598–2608. doi:10.1182/blood.2020008503
Delmonte, O. M., Schuetz, C., and Notarangelo, L. D. (2018). RAG deficiency: Two genes, many diseases. J. Clin. Immunol. 38, 646–655. doi:10.1007/s10875-018-0537-4
Delmonte, O. M., Villa, A., and Notarangelo, L. D. (2020). Immune dysregulation in patients with RAG deficiency and other forms of combined immune deficiency. Blood 135, 610–619. doi:10.1182/blood.2019000923
Demirdag, Y., Fuleihan, R., Orange, J. S., and Yu, J. E. (2021). New primary immunodeficiencies 2021 context and future. Curr. Opin. Pediatr. 33, 657–675. doi:10.1097/MOP.0000000000001075
Dever, D. P., Bak, R. O., Reinisch, A., Camarena, J., Washington, G., Nicolas, C. E., et al. (2016). CRISPR/Cas9 beta-globin gene targeting in human haematopoietic stem cells. Nature 539, 384–389. doi:10.1038/nature20134
Dobbs, K., Tabellini, G., Calzoni, E., Patrizi, O., Martinez, P., Giliani, S. C., et al. (2017). Natural killer cells from patients with recombinase-activating gene and non-homologous end joining gene defects comprise a higher frequency of CD56(bright) NKG2A(+++) cells, and yet display increased degranulation and higher perforin content. Front. Immunol. 8, 798. doi:10.3389/fimmu.2017.00798
Dvorak, C. C., Haddad, E., Buckley, R. H., Cowan, M. J., Logan, B., Griffith, L. M., et al. (2019). The genetic landscape of severe combined immunodeficiency in the United States and Canada in the current era (2010-2018). J. Allergy Clin. Immunol. 143, 405–407. doi:10.1016/j.jaci.2018.08.027
Ferrari, S., Jacob, A., Beretta, S., Unali, G., Albano, L., Vavassori, V., et al. (2020). Efficient gene editing of human long-term hematopoietic stem cells validated by clonal tracking. Nat. Biotechnol. 38, 1298–1308. doi:10.1038/s41587-020-0551-y
Ferrari, S., Jacob, A., Cesana, D., Laugel, M., Beretta, S., Varesi, A., et al. (2022). Choice of template delivery mitigates the genotoxic risk and adverse impact of editing in human hematopoietic stem cells. Cell Stem Cell 29, 1428–1444.e9. doi:10.1016/j.stem.2022.09.001
Floess, S., Freyer, J., Siewert, C., Baron, U., Olek, S., Polansky, J., et al. (2007). Epigenetic control of the foxp3 locus in regulatory T cells. PLoS Biol. 5, e38. doi:10.1371/journal.pbio.0050038
Fontenot, J. D., Gavin, M. A., and Rudensky, A. Y. (2003). Foxp3 programs the development and function of CD4+CD25+ regulatory T cells. Nat. Immunol. 4, 330–336. doi:10.1038/ni904
Fremond, M. L., Hadchouel, A., Berteloot, L., Melki, I., Bresson, V., Barnabei, L., et al. (2021). Overview of STING-associated vasculopathy with onset in infancy (SAVI) among 21 patients. J. Allergy Clin. Immunol. Pract. 9, 803–818.e11. doi:10.1016/j.jaip.2020.11.007
Gambineri, E., Ciullini Mannurita, S., Hagin, D., Vignoli, M., Anover-Sombke, S., DeBoer, S., et al. (2018). Clinical, immunological, and molecular heterogeneity of 173 patients with the phenotype of immune dysregulation, polyendocrinopathy, enteropathy, X-linked (IPEX) syndrome. Front. Immunol. 9, 2411. doi:10.3389/fimmu.2018.02411
Gardner, C. L., Pavel-Dinu, M., Dobbs, K., Bosticardo, M., Reardon, P. K., Lack, J., et al. (2021). Gene editing rescues in vitro T cell development of RAG2-deficient induced pluripotent stem cells in an artificial thymic organoid system. J. Clin. Immunol. 41, 852–862. doi:10.1007/s10875-021-00989-6
Gasiunas, G., Barrangou, R., Horvath, P., and Siksnys, V. (2012). Cas9-crRNA ribonucleoprotein complex mediates specific DNA cleavage for adaptive immunity in bacteria. Proc. Natl. Acad. Sci. U. S. A. 109, E2579–E2586. doi:10.1073/pnas.1208507109
Gaudelli, N. M., Komor, A. C., Rees, H. A., Packer, M. S., Badran, A. H., Bryson, D. I., et al. (2017). Programmable base editing of A*T to G*C in genomic DNA without DNA cleavage. Nature 551, 464–471. doi:10.1038/nature24644
Gehrke, J. M., Cervantes, O., Clement, M. K., Wu, Y., Zeng, J., Bauer, D. E., et al. (2018). An APOBEC3A-Cas9 base editor with minimized bystander and off-target activities. Nat. Biotechnol. 36, 977–982. doi:10.1038/nbt.4199
Genovese, P., Schiroli, G., Escobar, G., Tomaso, T. D., Firrito, C., Calabria, A., et al. (2014). Targeted genome editing in human repopulating haematopoietic stem cells. Nature 510, 235–240. doi:10.1038/nature13420
Giblin, W., Chatterji, M., Westfield, G., Masud, T., Theisen, B., Cheng, H. L., et al. (2009). Leaky severe combined immunodeficiency and aberrant DNA rearrangements due to a hypomorphic RAG1 mutation. Blood 113, 2965–2975. doi:10.1182/blood-2008-07-165167
Gilbert, L. A., Horlbeck, M. A., Adamson, B., Villalta, J. E., Chen, Y., Whitehead, E. H., et al. (2014). Genome-scale CRISPR-mediated control of gene repression and activation. Cell 159, 647–661. doi:10.1016/j.cell.2014.09.029
Gomez-Ospina, N., Scharenberg, S. G., Mostrel, N., Bak, R. O., Mantri, S., Quadros, R. M., et al. (2019). Human genome-edited hematopoietic stem cells phenotypically correct Mucopolysaccharidosis type I. Nat. Commun. 10, 4045. doi:10.1038/s41467-019-11962-8
Goodwin, M., Lee, E., Lakshmanan, U., Shipp, S., Froess, L., Barzaghi, F., et al. (2020). CRISPR-based gene editing enables FOXP3 gene repair in IPEX patient cells. Sci. Adv. 6, eaaz0571. doi:10.1126/sciadv.aaz0571
Gray, D. H., Villegas, I., Long, J., Santos, J., Keir, A., Abele, A., et al. (2021). Optimizing integration and expression of transgenic bruton's tyrosine kinase for CRISPR-cas9-mediated gene editing of X-linked agammaglobulinemia. CRISPR J. 4, 191–206. doi:10.1089/crispr.2020.0080
Greenberg-Kushnir, N., Lee, Y. N., Simon, A. J., Lev, A., Marcus, N., Abuzaitoun, O., et al. (2020). A large cohort of RAG1/2-deficient SCID patients-clinical, immunological, and prognostic analysis. J. Clin. Immunol. 40, 211–222. doi:10.1007/s10875-019-00717-1
Grizot, S., Smith, J., Daboussi, F., Prieto, J., Redondo, P., Merino, N., et al. (2009). Efficient targeting of a SCID gene by an engineered single-chain homing endonuclease. Nucleic Acids Res. 37, 5405–5419. doi:10.1093/nar/gkp548
Haendel, M., Vasilevsky, N., Unni, D., Bologa, C., Harris, N., Rehm, H., et al. (2020). How many rare diseases are there? Nat. Rev. Drug Discov. 19, 77–78. doi:10.1038/d41573-019-00180-y
Hendel, A., Bak, R. O., Clark, J. T., Kennedy, A. B., Ryan, D. E., Roy, S., et al. (2015). Chemically modified guide RNAs enhance CRISPR-Cas genome editing in human primary cells. Nat. Biotechnol. 33, 985–989. doi:10.1038/nbt.3290
High, K. A., and Roncarolo, M. G. (2019). Gene therapy. N. Engl. J. Med. 381, 455–464. doi:10.1056/NEJMra1706910
Hirsch, M. L. (2015). Adeno-associated virus inverted terminal repeats stimulate gene editing. Gene Ther. 22, 190–195. doi:10.1038/gt.2014.109
Hoban, M. D., Cost, G. J., Mendel, M. C., Romero, Z., Kaufman, M. L., Joglekar, A. V., et al. (2015). Correction of the sickle cell disease mutation in human hematopoietic stem/progenitor cells. Blood 125, 2597–2604. doi:10.1182/blood-2014-12-615948
Honaker, Y., Hubbard, N., Xiang, Y., Fisher, L., Hagin, D., Sommer, K., et al. (2020). Gene editing to induce FOXP3 expression in human CD4(+) T cells leads to a stable regulatory phenotype and function. Sci. Transl. Med. 12, eaay6422. doi:10.1126/scitranslmed.aay6422
Hu, J. H., Miller, S. M., Geurts, M. H., Tang, W., Chen, L., Sun, N., et al. (2018). Evolved Cas9 variants with broad PAM compatibility and high DNA specificity. Nature 556, 57–63. doi:10.1038/nature26155
Hubbard, N., Hagin, D., Sommer, K., Song, Y., Khan, I., Clough, C., et al. (2016). Targeted gene editing restores regulated CD40L function in X-linked hyper-IgM syndrome. Blood 127, 2513–2522. doi:10.1182/blood-2015-11-683235
Iancu, O., Allen, D., Knop, O., Zehavi, Y., Breier, D., Arbiv, A., et al. (2023). Multiplex HDR for disease and correction modeling of SCID by CRISPR genome editing in human HSPCs. Mol. Ther. Nucleic Acids 31, 105–121. doi:10.1016/j.omtn.2022.12.006
Jasin, M. (1996). Genetic manipulation of genomes with rare-cutting endonucleases. Trends Genet. 12, 224–228. doi:10.1016/0168-9525(96)10019-6
Jeong, Y. K., Song, B., and Bae, S. (2020). Current status and challenges of DNA base editing tools. Mol. Ther. 28, 1938–1952. doi:10.1016/j.ymthe.2020.07.021
Jinek, M., Chylinski, K., Fonfara, I., Hauer, M., Doudna, J. A., and Charpentier, E. (2012). A programmable dual-RNA-guided DNA endonuclease in adaptive bacterial immunity. Science 337, 816–821. doi:10.1126/science.1225829
Karo, J. M., Schatz, D. G., and Sun, J. C. (2014). The RAG recombinase dictates functional heterogeneity and cellular fitness in natural killer cells. Cell 159, 94–107. doi:10.1016/j.cell.2014.08.026
Khiong, K., Murakami, M., Kitabayashi, C., Ueda, N., Sawa, S. i., Sakamoto, A., et al. (2007). Homeostatically proliferating CD4 T cells are involved in the pathogenesis of an Omenn syndrome murine model. J. Clin. Invest. 117, 1270–1281. doi:10.1172/JCI30513
Kim, Y. B., Komor, A. C., Levy, J. M., Packer, M. S., Zhao, K. T., and Liu, D. R. (2017). Increasing the genome-targeting scope and precision of base editing with engineered Cas9-cytidine deaminase fusions. Nat. Biotechnol. 35, 371–376. doi:10.1038/nbt.3803
Koblan, L. W., Doman, J. L., Wilson, C., Levy, J. M., Tay, T., Newby, G. A., et al. (2018). Improving cytidine and adenine base editors by expression optimization and ancestral reconstruction. Nat. Biotechnol. 36, 843–846. doi:10.1038/nbt.4172
Komor, A. C., Kim, Y. B., Packer, M. S., Zuris, J. A., and Liu, D. R. (2016). Programmable editing of a target base in genomic DNA without double-stranded DNA cleavage. Nature 533, 420–424. doi:10.1038/nature17946
Komor, A. C., Zhao, K. T., Packer, M. S., Gaudelli, N. M., Waterbury, A. L., Koblan, L. W., et al. (2017). Improved base excision repair inhibition and bacteriophage Mu Gam protein yields C:G-to-T:A base editors with higher efficiency and product purity. Sci. Adv. 3, eaao4774. doi:10.1126/sciadv.aao4774
Kressler, C., Gasparoni, G., Nordstrom, K., Hamo, D., Salhab, A., Dimitropoulos, C., et al. (2020). Targeted de-methylation of the FOXP3-TSDR is sufficient to induce physiological FOXP3 expression but not a functional Treg phenotype. Front. Immunol. 11, 609891. doi:10.3389/fimmu.2020.609891
Kuo, C. Y., Long, J. D., Campo-Fernandez, B., de Oliveira, S., Cooper, A. R., Romero, Z., et al. (2018). Site-specific gene editing of human hematopoietic stem cells for X-linked hyper-IgM syndrome. Cell Rep. 23, 2606–2616. doi:10.1016/j.celrep.2018.04.103
Kwan, A., Church, J. A., Cowan, M. J., Agarwal, R., Kapoor, N., Kohn, D. B., et al. (2013). Newborn screening for severe combined immunodeficiency and T-cell lymphopenia in California: Results of the first 2 years. J. Allergy Clin. Immunol. 132, 140–150. doi:10.1016/j.jaci.2013.04.024
Lattanzi, A., Camarena, J., Lahiri, P., Segal, H., Srifa, W., Vakulskas, C. A., et al. (2021). Development of beta-globin gene correction in human hematopoietic stem cells as a potential durable treatment for sickle cell disease. Sci. Transl. Med. 13, eabf2444. doi:10.1126/scitranslmed.abf2444
Liang, P., Ding, C., Sun, H., Xie, X., Xu, Y., Zhang, X., et al. (2017). Correction of beta-thalassemia mutant by base editor in human embryos. Protein Cell 8, 811–822. doi:10.1007/s13238-017-0475-6
Maeder, M. L., Stefanidakis, M., Wilson, C. J., Baral, R., Barrera, L. A., Bounoutas, G. S., et al. (2019). Development of a gene-editing approach to restore vision loss in Leber congenital amaurosis type 10. Nat. Med. 25, 229–233. doi:10.1038/s41591-018-0327-9
Mailer, R. K. W. (2018). Alternative splicing of FOXP3-virtue and vice. Front. Immunol. 9, 530. doi:10.3389/fimmu.2018.00530
Mali, P., Yang, L., Esvelt, K. M., Aach, J., Guell, M., DiCarlo, J. E., et al. (2013). RNA-guided human genome engineering via Cas9. Science 339, 823–826. doi:10.1126/science.1232033
Marrella, V., Poliani, P. L., Casati, A., Rucci, F., Frascoli, L., Gougeon, M. L., et al. (2007). A hypomorphic R229Q Rag2 mouse mutant recapitulates human Omenn syndrome. J. Clin. Invest. 117, 1260–1269. doi:10.1172/JCI30928
Mazzolari, E., Moshous, D., Forino, C., De Martiis, D., Offer, C., LanfrAnchi, A., et al. (2005). Hematopoietic stem cell transplantation in Omenn syndrome: A single-center experience. Bone Marrow Transpl. 36, 107–114. doi:10.1038/sj.bmt.1705017
Meshaal, S. S., El Hawary, R. E., Abd Elaziz, D. S., EldAsh, A., Alkady, R., Lotfy, S., et al. (2019). Phenotypical heterogeneity in RAG-deficient patients from a highly consanguineous population. Clin. Exp. Immunol. 195, 202–212. doi:10.1111/cei.13222
Miller, J. C., Tan, S., Qiao, G., Barlow, K. A., Wang, J., Xia, D. F., et al. (2011). A TALE nuclease architecture for efficient genome editing. Nat. Biotechnol. 29, 143–148. doi:10.1038/nbt.1755
Miyazaki, K., and Miyazaki, M. (2021). The interplay between chromatin architecture and lineage-specific transcription factors and the regulation of Rag gene expression. Front. Immunol. 12, 659761. doi:10.3389/fimmu.2021.659761
Mombaerts, P., Iacomini, J., Johnson, R. S., Herrup, K., Tonegawa, S., and Papaioannou, V. E. (1992). RAG-1-deficient mice have no mature B and T lymphocytes. Cell 68, 869–877. doi:10.1016/0092-8674(92)90030-g
Naldini, L. (2011). Ex vivo gene transfer and correction for cell-based therapies. Nat. Rev. Genet. 12, 301–315. doi:10.1038/nrg2985
Nature Medicine (2021). Gene therapies should be for all. Nat. Med. 27, 1311. doi:10.1038/s41591-021-01481-9
Nelson, J. W., Randolph, P. B., Shen, S. P., Everette, K. A., Chen, P. J., Anzalone, A. V., et al. (2022). Engineered pegRNAs improve prime editing efficiency. Nat. Biotechnol. 40, 402–410. doi:10.1038/s41587-021-01039-7
Nishimasu, H., Shi, X., Ishiguro, S., Gao, L., Hirano, S., Okazaki, S., et al. (2018). Engineered CRISPR-Cas9 nuclease with expanded targeting space. Science 361, 1259–1262. doi:10.1126/science.aas9129
Notarangelo, L. D., Kim, M. S., Walter, J. E., and Lee, Y. N. (2016). Human RAG mutations: Biochemistry and clinical implications. Nat. Rev. Immunol. 16, 234–246. doi:10.1038/nri.2016.28
Notarangelo, L. D. (2010). Primary immunodeficiencies. J. Allergy Clin. Immunol. 125, S182–S194. doi:10.1016/j.jaci.2009.07.053
Ott de Bruin, L., Yang, W., Capuder, K., Lee, Y. N., Antolini, M., Meyers, R., et al. (2016). Rapid generation of novel models of RAG1 deficiency by CRISPR/Cas9-induced mutagenesis in murine zygotes. Oncotarget 7, 12962–12974. doi:10.18632/oncotarget.7341
Ott de Bruin, L. M., Bosticardo, M., Barbieri, A., Lin, S. G., Rowe, J. H., Poliani, P. L., et al. (2018). Hypomorphic Rag1 mutations alter the preimmune repertoire at early stages of lymphoid development. Blood 132, 281–292. doi:10.1182/blood-2017-12-820985
Park, S. H., Cao, M., Pan, Y., Davis, T. H., Saxena, L., Deshmukh, H., et al. (2022). Comprehensive analysis and accurate quantification of unintended large gene modifications induced by CRISPR-Cas9 gene editing. Sci. Adv. 8, eabo7676. doi:10.1126/sciadv.abo7676
Pavel-Dinu, M., Gardner, C. L., and Nakauchi, Y. (2022). Genetically corrected <em>RAG2</em>-SCID human hematopoietic stem cells restore V(D)J-Recombinase and rescue lymphoid deficiency. bioRxiv. doi:10.1101/2022.07.12.499831
Pavel-Dinu, M., Wiebking, V., Dejene, B. T., Srifa, W., Mantri, S., Nicolas, C. E., et al. (2019). Gene correction for SCID-X1 in long-term hematopoietic stem cells. Nat. Commun. 10, 1634. doi:10.1038/s41467-019-09614-y
Pickar-Oliver, A., and Gersbach, C. A. (2019). The next generation of CRISPR-Cas technologies and applications. Nat. Rev. Mol. Cell Biol. 20, 490–507. doi:10.1038/s41580-019-0131-5
Pike-Overzet, K., Baum, C., Bredius, R. G., Cavazzana, M., Driessen, G. J., Fibbe, W. E., et al. (2014). Successful RAG1-SCID gene therapy depends on the level of RAG1 expression. J. Allergy Clin. Immunol. 134, 242–243. doi:10.1016/j.jaci.2014.04.033
Pike-Overzet, K., Rodijk, M., Ng, Y. Y., Baert, M. R. M., Lagresle-Peyrou, C., SchAmbAch, A., et al. (2011). Correction of murine Rag1 deficiency by self-inactivating lentiviral vector-mediated gene transfer. Leukemia 25, 1471–1483. doi:10.1038/leu.2011.106
Polansky, J. K., Kretschmer, K., Freyer, J., Floess, S., Garbe, A., Baron, U., et al. (2008). DNA methylation controls Foxp3 gene expression. Eur. J. Immunol. 38, 1654–1663. doi:10.1002/eji.200838105
Porteus, M. H. (2019). A new class of medicines through DNA editing. N. Engl. J. Med. 380, 947–959. doi:10.1056/NEJMra1800729
Porteus, M. H., and Baltimore, D. (2003). Chimeric nucleases stimulate gene targeting in human cells. Science 300, 763. doi:10.1126/science.1078395
Porteus, M. H., and Carroll, D. (2005). Gene targeting using zinc finger nucleases. Nat. Biotechnol. 23, 967–973. doi:10.1038/nbt1125
Porteus, M. H., Cathomen, T., Weitzman, M. D., and Baltimore, D. (2003). Efficient gene targeting mediated by adeno-associated virus and DNA double-strand breaks. Mol. Cell Biol. 23, 3558–3565. doi:10.1128/mcb.23.10.3558-3565.2003
Porteus, M. H., Pavel-Dinu, M., and Pai, S. Y. (2022). A curative DNA code for hematopoietic defects: Novel cell therapies for monogenic diseases of the blood and immune system. Hematol. Oncol. Clin. North Am. 36, 647–665. doi:10.1016/j.hoc.2022.05.002
Powell, B. R., Buist, N. R., and Stenzel, P. (1982). An X-linked syndrome of diarrhea, polyendocrinopathy, and fatal infection in infancy. J. Pediatr. 100, 731–737. doi:10.1016/s0022-3476(82)80573-8
Qi, L. S., Larson, M. H., Gilbert, L. A., Doudna, J. A., Weissman, J. S., Arkin, A. P., et al. (2013). Repurposing CRISPR as an RNA-guided platform for sequence-specific control of gene expression. Cell 152, 1173–1183. doi:10.1016/j.cell.2013.02.022
Rai, R., Romito, M., Rivers, E., Turchiano, G., Blattner, G., Vetharoy, W., et al. (2020). Targeted gene correction of human hematopoietic stem cells for the treatment of Wiskott - aldrich Syndrome. Nat. Commun. 11, 4034. doi:10.1038/s41467-020-17626-2
Rees, H. A., and Liu, D. R. (2018). Base editing: Precision chemistry on the genome and transcriptome of living cells. Nat. Rev. Genet. 19, 770–788. doi:10.1038/s41576-018-0059-1
Rim, J., Byler, M., Soldatos, A., Notarangelo, L., Leibovitch, E., Jacobson, S., et al. (2021). Opinion and special articles: Cerebellar ataxia and liver failure complicating IPEX syndrome. Neurology 96, e956–e959. doi:10.1212/WNL.0000000000011195
Roman-Rodriguez, F. J., Ugalde, L., Alvarez, L., Diez, B., Ramirez, M. J., Risueno, C., et al. (2019). NHEJ-mediated repair of CRISPR-cas9-induced DNA breaks efficiently corrects mutations in HSPCs from patients with fanconi anemia. Cell Stem Cell 25, 607–621. doi:10.1016/j.stem.2019.08.016
Rose, J. C., Stephany, J. J., Valente, W. J., Trevillian, B. M., Dang, H. V., Bielas, J. H., et al. (2017). Rapidly inducible Cas9 and DSB-ddPCR to probe editing kinetics. Nat. Methods 14, 891–896. doi:10.1038/nmeth.4368
Rouet, P., Smih, F., and Jasin, M. (1994). Expression of a site-specific endonuclease stimulates homologous recombination in mammalian cells. Proc. Natl. Acad. Sci. U. S. A. 91, 6064–6068. doi:10.1073/pnas.91.13.6064
Sakata, R. C., Ishiguro, S., Mori, H., Tanaka, M., Tatsuno, K., Ueda, H., et al. (2020). Base editors for simultaneous introduction of C-to-T and A-to-G mutations. Nat. Biotechnol. 38, 865–869. doi:10.1038/s41587-020-0509-0
Santoni de Sio, F. R., Passerini, L., Valente, M. M., Russo, F., NaLdini, L., Roncarolo, M. G., et al. (2017). Ectopic FOXP3 expression preserves primitive features of human hematopoietic stem cells while impairing functional T cell differentiation. Sci. Rep. 7, 15820. doi:10.1038/s41598-017-15689-8
Sato, Y., Liu, J., Lee, E., Perriman, R., Roncarolo, M. G., and Bacchetta, R. (2021). Co-expression of FOXP3FL and FOXP3Δ2 isoforms is required for optimal treg-like cell phenotypes and suppressive function. Front. Immunol. 12, 752394. doi:10.3389/fimmu.2021.752394
Sato, Y., Passerini, L., Piening, B. D., Uyeda, M. J., Goodwin, M., Gregori, S., et al. (2020). Human-engineered Treg-like cells suppress FOXP3-deficient T cells but preserve adaptive immune responses in vivo. Clin. Transl. Immunol. 9, e1214. doi:10.1002/cti2.1214
Scala, S., Basso-Ricci, L., Dionisio, F., Pellin, D., Giannelli, S., Salerio, F. A., et al. (2018). Dynamics of genetically engineered hematopoietic stem and progenitor cells after autologous transplantation in humans. Nat. Med. 24, 1683–1690. doi:10.1038/s41591-018-0195-3
Scharenberg, S. G., Poletto, E., Lucot, K. L., Colella, P., Sheikali, A., Montine, T. J., et al. (2020). Engineering monocyte/macrophage-specific glucocerebrosidase expression in human hematopoietic stem cells using genome editing. Nat. Commun. 11, 3327. doi:10.1038/s41467-020-17148-x
Schiroli, G., Conti, A., Ferrari, S., Della Volpe, L., Jacob, A., Albano, L., et al. (2019). Precise gene editing preserves hematopoietic stem cell function following transient p53-mediated DNA damage response. Cell Stem Cell 24, 551–565. doi:10.1016/j.stem.2019.02.019
Schiroli, G., Ferrari, S., Conway, A., Jacob, A., Capo, V., Albano, L., et al. (2017). Preclinical modeling highlights the therapeutic potential of hematopoietic stem cell gene editing for correction of SCID-X1. Sci. Transl. Med. 9, eaan0820. doi:10.1126/scitranslmed.aan0820
Schuetz, C., Neven, B., Dvorak, C. C., Leroy, S., Ege, M. J., Pannicke, U., et al. (2014). SCID patients with ARTEMIS vs RAG deficiencies following HCT: Increased risk of late toxicity in ARTEMIS-deficient SCID. Blood 123, 281–289. doi:10.1182/blood-2013-01-476432
Sharma, R., Dever, D. P., Lee, C. M., Azizi, A., Pan, Y., Camarena, J., et al. (2021). The TRACE-Seq method tracks recombination alleles and identifies clonal reconstitution dynamics of gene targeted human hematopoietic stem cells. Nat. Commun. 12, 472. doi:10.1038/s41467-020-20792-y
Shinkai, Y., Rathbun, G., Lam, K. P., Oltz, E. M., Stewart, V., Mendelsohn, M., et al. (1992). RAG-2-deficient mice lack mature lymphocytes owing to inability to initiate V(D)J rearrangement. Cell 68, 855–867. doi:10.1016/0092-8674(92)90029-c
Shy, B. R., Vykunta, V. S., Ha, A., Talbot, A., Roth, T. L., Nguyen, D. N., et al. (2022). High-yield genome engineering in primary cells using a hybrid ssDNA repair template and small-molecule cocktails. Nat. Biotechnol. doi:10.1038/s41587-022-01418-8
Smithies, O., Gregg, R. G., Boggs, S. S., Koralewski, M. A., and Kucherlapati, R. S. (1985). Insertion of DNA sequences into the human chromosomal beta-globin locus by homologous recombination. Nature 317, 230–234. doi:10.1038/317230a0
Sweeney, C. L., Pavel-Dinu, M., Choi, U., Brault, J., Liu, T., Koontz, S., et al. (2021). Correction of X-CGD patient HSPCs by targeted CYBB cDNA insertion using CRISPR/Cas9 with 53BP1 inhibition for enhanced homology-directed repair. Gene Ther. 28, 373–390. doi:10.1038/s41434-021-00251-z
Tangye, S. G., Al-Herz, W., Bousfiha, A., Chatila, T., Cunningham-Rundles, C., Etzioni, A., et al. (2020). Human inborn errors of immunity: 2019 update on the classification from the international union of immunological societies expert committee. J. Clin. Immunol. 40, 24–64. doi:10.1007/s10875-019-00737-x
Tangye, S. G., Al-Herz, W., Bousfiha, A., Cunningham-Rundles, C., Franco, J. L., Holland, S. M., et al. (2022). Human inborn errors of immunity: 2022 update on the classification from the international union of immunological societies expert committee. J. Clin. Immunol. 42, 1473–1507. doi:10.1007/s10875-022-01289-3
Tirosh, I., Yamazaki, Y., Frugoni, F., Ververs, F. A., Allenspach, E. J., Zhang, Y., et al. (2019). Recombination activity of human recombination-activating gene 2 (RAG2) mutations and correlation with clinical phenotype. J. Allergy Clin. Immunol. 143, 726–735. doi:10.1016/j.jaci.2018.04.027
Turchiano, G., Andrieux, G., Klermund, J., Blattner, G., Pennucci, V., El Gaz, M., et al. (2021). Quantitative evaluation of chromosomal rearrangements in gene-edited human stem cells by CAST-Seq. Cell Stem Cell 28, 1136–1147.e5. doi:10.1016/j.stem.2021.02.002
Urnov, F. D., Miller, J. C., Lee, Y. L., Beausejour, C. M., Rock, J. M., Augustus, S., et al. (2005). Highly efficient endogenous human gene correction using designed zinc-finger nucleases. Nature 435, 646–651. doi:10.1038/nature03556
Urnov, F. D. (2020). Prime time for genome editing? N. Engl. J. Med. 382, 481–484. doi:10.1056/NEJMcibr1914271
Vakulskas, C. A., Dever, D. P., Rettig, G. R., Turk, R., Jacobi, A. M., Collingwood, M. A., et al. (2018). A high-fidelity Cas9 mutant delivered as a ribonucleoprotein complex enables efficient gene editing in human hematopoietic stem and progenitor cells. Nat. Med. 24, 1216–1224. doi:10.1038/s41591-018-0137-0
van Til, N. P., de Boer, H., Mashamba, N., Wabik, A., Huston, M., Visser, T. P., et al. (2012). Correction of murine Rag2 severe combined immunodeficiency by lentiviral gene therapy using a codon-optimized RAG2 therapeutic transgene. Mol. Ther. 20, 1968–1980. doi:10.1038/mt.2012.110
van Til, N. P., Sarwari, R., Visser, T. P., Hauer, J., Lagresle-Peyrou, C., van der Velden, G., et al. (2014). Recombination-activating gene 1 (Rag1)-deficient mice with severe combined immunodeficiency treated with lentiviral gene therapy demonstrate autoimmune Omenn-like syndrome. J. Allergy Clin. Immunol. 133, 1116–1123. doi:10.1016/j.jaci.2013.10.009
Vavassori, V., Mercuri, E., Marcovecchio, G. E., Castiello, M. C., Schiroli, G., Albano, L., et al. (2021). Modeling, optimization, and comparable efficacy of T cell and hematopoietic stem cell gene editing for treating hyper-IgM syndrome. EMBO Mol. Med. 13, e13545. doi:10.15252/emmm.202013545
Villa, A., and Notarangelo, L. D. (2019). RAG gene defects at the verge of immunodeficiency and immune dysregulation. Immunol. Rev. 287, 73–90. doi:10.1111/imr.12713
Villa, A., Santagata, S., Bozzi, F., Imberti, L., and Notarangelo, L. D. (1999). Omenn syndrome: A disorder of Rag1 and Rag2 genes. J. Clin. Immunol. 19, 87–97. doi:10.1023/a:1020550432126
Villa, A., Sobacchi, C., Notarangelo, L. D., Bozzi, F., Abinun, M., Abrahamsen, T. G., et al. (2001). V(D)J recombination defects in lymphocytes due to RAG mutations: Severe immunodeficiency with a spectrum of clinical presentations. Blood 97, 81–88. doi:10.1182/blood.v97.1.81
Voit, R. A., Hendel, A., Pruett-Miller, S. M., and Porteus, M. H. (2014). Nuclease-mediated gene editing by homologous recombination of the human globin locus. Nucleic Acids Res. 42, 1365–1378. doi:10.1093/nar/gkt947
Wang, X., Li, J., Wang, Y., Yang, B., Wei, J., Wu, J., et al. (2018). Efficient base editing in methylated regions with a human APOBEC3A-Cas9 fusion. Nat. Biotechnol. 36, 946–949. doi:10.1038/nbt.4198
Wildin, R. S., Ramsdell, F., Peake, J., Faravelli, F., Casanova, J. L., Buist, N., et al. (2001). X-linked neonatal diabetes mellitus, enteropathy and endocrinopathy syndrome is the human equivalent of mouse scurfy. Nat. Genet. 27, 18–20. doi:10.1038/83707
Wilkinson, A. C., Dever, D. P., Baik, R., Camarena, J., Hsu, I., Charlesworth, C. T., et al. (2021). Cas9-AAV6 gene correction of beta-globin in autologous HSCs improves sickle cell disease erythropoiesis in mice. Nat. Commun. 12, 686. doi:10.1038/s41467-021-20909-x
Xue, C., and Greene, E. C. (2021). DNA repair pathway choices in CRISPR-cas9-mediated genome editing. Trends Genet. 37, 639–656. doi:10.1016/j.tig.2021.02.008
Yeh, C. D., Richardson, C. D., and Corn, J. E. (2019). Advances in genome editing through control of DNA repair pathways. Nat. Cell Biol. 21, 1468–1478. doi:10.1038/s41556-019-0425-z
Zeng, J., Wu, Y., Ren, C., Bonanno, J., Shen, A. H., Shea, D., et al. (2020). Therapeutic base editing of human hematopoietic stem cells. Nat. Med. 26, 535–541. doi:10.1038/s41591-020-0790-y
Zheng, Y., Josefowicz, S., Chaudhry, A., Peng, X. P., Forbush, K., and Rudensky, A. Y. (2010). Role of conserved non-coding DNA elements in the Foxp3 gene in regulatory T-cell fate. Nature 463, 808–812. doi:10.1038/nature08750
Keywords: CRISPR/Cas9, adeno-associated virus 6, base editing, prime and twin prime editing, hematopoietic stem and progenitor cells, RAG2 deficiency, FOXP3, IPEX syndrome
Citation: Pavel-Dinu M, Borna S and Bacchetta R (2023) Rare immune diseases paving the road for genome editing-based precision medicine. Front. Genome Ed. 5:1114996. doi: 10.3389/fgeed.2023.1114996
Received: 03 December 2022; Accepted: 26 January 2023;
Published: 08 February 2023.
Edited by:
Donald B. Kohn, University of California, Los Angeles, United StatesReviewed by:
Ayal Hendel, Bar-Ilan University, IsraelCopyright © 2023 Pavel-Dinu, Borna and Bacchetta. This is an open-access article distributed under the terms of the Creative Commons Attribution License (CC BY). The use, distribution or reproduction in other forums is permitted, provided the original author(s) and the copyright owner(s) are credited and that the original publication in this journal is cited, in accordance with accepted academic practice. No use, distribution or reproduction is permitted which does not comply with these terms.
*Correspondence: Rosa Bacchetta, cm9zYWJAc3RhbmZvcmQuZWR1