- 1Department of Plant Science and Landscape Architecture, University of Connecticut, Storrs, CT, United States
- 2Section of Cell and Developmental Biology, University of California, San Diego, CA, United States
Genome editing technologies provide a powerful tool for genetic improvement of perennial ryegrass, an important forage and turfgrass species worldwide. The sole publication for gene editing in perennial ryegrass used gene-gun for plant transformation and a dual promoter based CRISPR/Cas9 system for editing. However, their editing efficiency was low (5.9% or only one gene-edited plant produced). To test the suitability of the maize Ubiquitin 1 (ZmUbi1) promoter in gene editing of perennial ryegrass, we produced ZmUbi1 promoter:RUBY transgenic plants. We observed that ZmUbi1 promoter was active in callus tissue prior to shoot regeneration, suggesting that the promoter is suitable for Cas9 and sgRNA expression in perennial ryegrass for high-efficiency production of bi-allelic mutant plants. We then used the ZmUbi1 promoter for controlling Cas9 and sgRNA expression in perennial ryegrass. A ribozyme cleavage target site between the Cas9 and sgRNA sequences allowed production of functional Cas9 mRNA and sgRNA after transcription. Using Agrobacterium for genetic transformation, we observed a 29% efficiency for editing the PHYTOENE DESATURASE gene in perennial ryegrass. DNA sequencing analyses revealed that most pds plants contained bi-allelic mutations. These results demonstrate that the expression of a single Cas9 and sgRNA transcript unit controlled by the ZmUbi1 promoter provides a highly efficient system for production of bi-allelic mutants of perennial ryegrass and should also be applicable in other related grass species.
Introduction
Perennial ryegrass (Lolium perenne L.) is one of the most popular and important bunch-type cool-season turfgrass species (Jiang and Huang, 2001; Emmons and Rossi, 2015; Grinberg et al., 2016). Owing to its rapid establishment, attractiveness, and leaf appearance, it is grown in various diverse areas such as residential lawns, national parks, athletic fields, and golf course fairways. Perennial ryegrass establishes faster than other turf species, therefore, it can be used to repair damaged lawns and athletic fields (Emmons and Rossi, 2015). In addition, perennial ryegrass is commonly used on athletic fields because of its wear and tear tolerance. However, perennial ryegrass is also susceptible to drought, extreme temperature, and diseases (Emmons and Rossi, 2015).
Modern breeding techniques such as transgenic and genome editing technologies promise to be more powerful, efficient, and precise compared to conventional breeding ones (Xiong et al., 2015; Hua et al., 2019; Ahmar et al., 2020). Transgenic technology has been used successfully to improve annual and perennial crops, but it faces social and political opposition. On the other hand, CRISPR/Cas9 assisted genome editing technology can be more acceptable for the genetic improvement of crop plants (Xiong et al., 2015; Hua et al., 2019). A simple and highly efficient genome editing system for perennial ryegrass could be helpful to genetically improve many of its traits. To date, only one study of CRISPR/Cas9 mediated genome editing of perennial ryegrass has been reported (Zhang et al., 2020). The study targeted the DISRUPTED MEIOTIC cDNA1 (DMC1) gene using a particle bombardment mediated-transformation method. However, only a single genome-edited perennial ryegrass plant (or 5.9% genome editing efficiency) was produced in their experiments.
Successful genome editing requires coordinated spatio-temporal expression of Cas9 protein and sgRNA, which has been achieved either by using a compatible set of two promoters or a single transcript unit (STU) system (Osakabe and Osakabe, 2015; Tang et al., 2016; Tang et al., 2019; Zhuo et al., 2021). Sometimes it can be difficult to achieve coordinated expression of Cas9 and sgRNAs in a dual promoter-based system, especially in non-model organisms where promoters have not been well characterized. A STU for CRISPR/Cas9 system relies on the expressions of Cas9 and sgRNAs under a single promoter, eliminating the need for multiple promoters working in concert. A STU CRISPR/Cas9 system has been reported in rice for high genome editing efficiencies (Tang et al., 2016). In their STU CRISPR/Cas9 system, Tang et al. (2016) co-expressed Cas9, sgRNA, and a self-cleaving hammerhead ribozyme (RZ) with a single maize Ubiquitin 1 (ZmUbi1) promoter. The single sgRNA and Cas9 transcripts are cleaved by the cis-acting ribozyme to generate functional Cas9 and sgRNAs.
In this study, we report the spatio-temporal activities of the ZmUbi1 promoter in perennial ryegrass using a RUBY reporter construct. We further report the use of Agrobacterium to deliver a single ZmUbi1 promoter based CRISPR/Cas9-sgRNA system into perennial ryegrass. Using our methodology, we have observed 29% editing efficiency in perennial ryegrass when the PHYTOENE DESATURASE (PDS) gene was used as a target.
Materials and methods
PDS gene sequence analysis and vector information
Homologous nucleotide sequences of PDS gene of wheat, rice, bermudagrass, and rigid ryegrass were retrieved by using the BLAST function from the NCBI database (www.ebi.ac.uk/Tools/sss/ncbiblast/nucleotide.html). The retrieved sequences were then used for BLAST analysis in perennial ryegrass transcriptome to identify a PDS gene (Byrne et al., 2015). PDS CRISPR/Cas9 (www.addgene.org/89269/) and visual marker RUBY (www.addgene.org/160909/) constructs were purchased from addgene (www.addgene.org). The RUBY reporter contains three genes CYP76AD1, L-DOPA 4,5-dioxygenase (DODA), and glucosyltransferase (Figure 1A). These three genes were linked by sequences that encode self-cleaving 2A peptides, which produce three functional proteins when the 2A peptides cleave themselves after translation (He et al., 2020). The PDS CRISPR/Cas9 construct used in this study was previously used for genome editing in rice (Tang et al., 2016). Nucleotide sequences of perennial ryegrass and rice PDS gene were perfectly matched at target and PAM location. In the CRISPR/Cas9 vector, a ZmUbi1 promoter was used to control the expression of Cas9 and gRNA (ZmUbi1:Cas9:gRNA). The sgRNA was flanked by RZ cleavage sites (Figure 1B). Both constructs contained a hygromycin B resistant gene as a selectable marker.
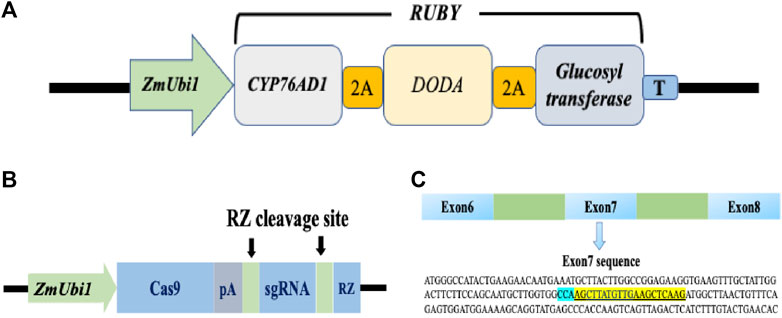
FIGURE 1. RUBY and CRISPR/Cas9 vector maps and target sequence for the perennial ryegrass PDS gene. (A) Schematic map of the RUBY construct. RUBY construct was designed by (He ct al., 2020). The three betalai biosynthetic genes were fused into a single open reading frame, The single transcript was expressed using a single ZmUbil promoter. 2A peptide coding sequences were inserted between these genes. A fler translation, the 2A peptides undergo self-cleavage. Thus releasing the individual proteins for betalain biosynthesss. (B) The ZmUbil promoter was used to contro] the expression of Cas9, PRNA, and RZ in a CRISPR/Cas9 construct. CRISPR'Cas9 construct was designed by Tang et al. (2016). A synthetic polyA (pA) sequence was used to terminate Cas9 mRNA translation. The RZ sequence (in blue) and its recognition sequence (in green) are used for producing functional Cas9 and sgRNA after transcription. (C) The gRNA sequence for the perennial ryegrass PDS gene.
Agrobacterium-mediated transformation of perennial ryegrass
Perennial ryegrass (L. perenne L.) cultivar “Fiesta-4” seeds were used for embryogenic callus induction. The seeds were de-husked by soaking them in 50% H2SO4 for 30 min. The de-husked seeds were surface sterilized with 3% (w/v) sodium hypochloride for 15 min, then washed several times with sterile distilled water. Sterilized seeds were bisected longitudinally with a sterilized blade into two parts, then cultured on a callus induction medium (3.98 g/L N6 basal salts, 1 mg/L thiamine-HCL, 1 g/L Casein hydrolysate, 800 mg/L proline, 20 mg/L L-Gln, 10 mg/L lipoic acid, 5 mg/L 2,4-D, 30 g/L maltose, 0.1 mg/L 6-benzyladenine (BA), and 3 g/L phytagel, pH 5.9). After the first week, growing shoots were cut at 4–6 days intervals and the callus induction medium was changed every 3 weeks. The CRISPR/Cas9 and RUBY vectors DNA were transferred into the Agrobacterium tumefaciens strain EHA105 (Wise et al., 2006) and transferred into embryogenic calli of perennial ryegrass using an Agrobacterium-mediated transformation method (Patel et al., 2013). The infected calli were subsequently cultured on a co-culture medium for 3 days at 25°C in a dark growth chamber. The infected calli were transferred to a resting medium (Callus induction medium and 200 mg/L timentin, pH 5.9) at 25°C in the dark for 5 days. Infected calli were subsequently grown on a selection medium (Callus induction medium, 200 mg/L timentin, and 50 mg/L hygromycin, pH 5.9) at 25°C in a dark growth chamber for 45 days, transferring the calli to a fresh selection medium every 15 days. Hygromycin resistant calli were transferred to a shoot regeneration medium (MS salts, 0.5 mg/L 6-benzyladenine (BA), 30 g/L maltose, 3 g/L phytagel, 150 mg/L timentin, and 50 mg/L hygromycin, pH 5.9) for the regeneration at 22°C under fluorescent light (16h light/8h dark).
Confirmation of genome editing via HindIII digestion and DNA sequencing
Transgenic plants were identified by the polymerase chain reaction (PCR) assay using a direct-PCR approach (Phire Plant Direct PCR Kit, Thermo Fisher Scientific Co., United States) according to the manufacturer’s protocol. A pair of Cas9 based primers (F- GAGGATGCTCGCTTCTGCTG and R- CGAGGTTTGCATCAGCGAGG) was designed to amplify a 229 bp long region to identify transgenic plants. Another pair of primers from the perennial ryegrass GA20ox1 gene (F-TCTGACGAGAACACCCTTGA and R-AGCACCTCCA TGATCTCCAG) was designed to amplify a 94 bp long region for internal control. Another pair of primers (F-GGGCCATACTGAAGAACAATG and R-TTCATTTAT GGACCTAGCC ACG) flanking the target site was used to amplify the 302 bp sgRNA-target region for HindIII digestion and Sanger DNA sequencing. A 6 bp long HindIII restriction site was located adjacent to the protospacer-adjacent motif (PAM) sequence, therefore, any mutation that occurred in the restriction site will be detected by HindIII digestion. The amplified PCR products were digested by HindIII restriction enzyme (New England Biolabs), as per the manufacturer’s instructions. For the Sanger DNA sequencing, the PCR products were run in 2% agarose gel, and specific bands were eluted and purified by using Nucleospin Gel and PCR Clean-up kit (Machery-Nagel #740609). For the Illumina sequence analysis, a 199 bp sgRNA-target region was amplified using a pair of primers, and these primers (F-ACACTCTTTCCCTACACGACGCTC TTCCGATCTTGGGCCATACTGAAGAACAATG and R-G TGACTGGAGTTCAGACGTGTGCTCTTCCGATCTGAGA CG GCTATGTGTTCAGTAC) had overhanging sequences (underline) with them for sequencing. The PCR products from all pds mutants were sequenced using Illumina MiSeq plateform. The raw Illumina reads were mapped to the 199 bp reference perennial ryegrass PDS gene sequence using bwa mem (-c 300000 -v 2) of BWA v0.7.17 (Li and Durbin, 2009; Chen et al., 2018).
Results
ZmUbi1 promoter shows activity in transformed calli and regenerating shoots
RUBY is a novel reporter gene system for higher plant that produces a red color pigment, betalain, if expressed (He et al., 2020). We investigated the spatio-temporal activities of the ZmUbi1 promoter in perennial ryegrass using the easily visible RUBY reporter. The RUBY construct was delivered in perennial ryegrass calli via an Agrobacterium-mediated transformation method. The use of RUBY made it easier to distinguish the transformed cells from untransformed ones owing to the red coloration. We observed that red color in explants 3 days post the Agrobacterium infection, suggesting that the ZmUbi1 promoter is active at a very early stage of transformation (data not shown). We produced twenty-three independents transgenic RUBY calli and 22 transgenic independent RUBY plant lines. As shown in Figures 2A–C–C, the ZmUbi1 promoter is active in calli and throughout the plant life cycle in roots, shoots and leaves. Because the ZmUbi1 promoter is active in calli before shoot regeneration, it should be a good candidate for controlling the expression of Cas9 and sgRNAs in perennial ryegrass to allow genome editing at the single-cell (callus) stage.
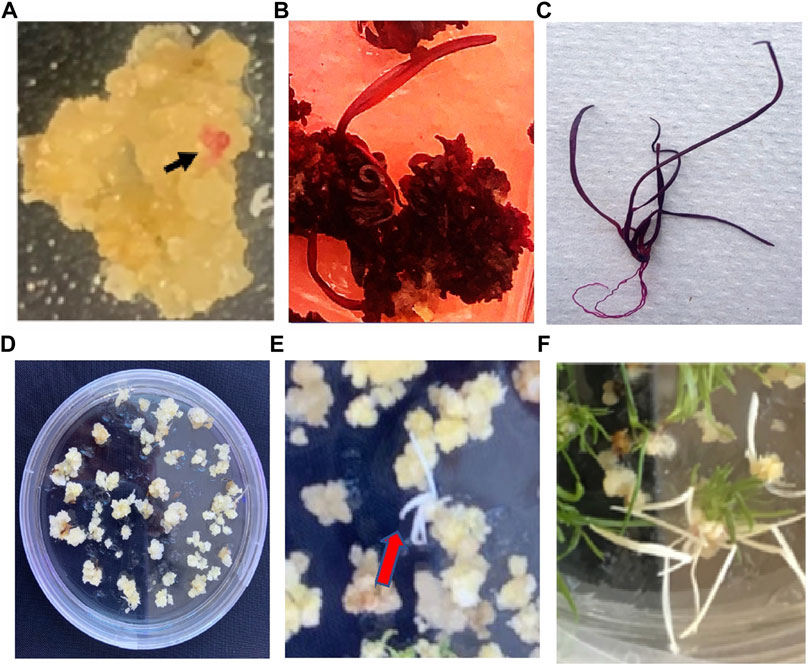
FIGURE 2. The activity of the ZmUbil promoter driving RUBY and production of pds mutants of perennial ryegrass. The ZmUbsl promoter:RUBY gene was expressed in call (A) (indicated by black arrows), shoots (B), and root and shoot (C). Putative CRISPR'Cas9 transformed perennial ryegrass calius under 50 mg/L hygromycin selection (D). Regenerating PDS genc-edited albino plants at the carty stage as indicated by orange arrows (E). PDS gene-cdited perennial ryegrass plant show mg albino phenotype (F).
Elevated hygromycin concentrations reduce natural albinism in perennial ryegrass generated from tissue culture
Spontaneous albinism is a common problem encountered during tissue culture and regeneration of perennial ryegrass without genetic transformation (Liu et al., 2006). The presence of these naturally occurring albino plants could complicate the identification of gene-edited pds mutant plants. To reduce that problem, we tested the effects of concentrations of hygromycin in the transgenic cell/plant selection medium on albinism frequency. A construct with no Cas9 or sgRNA with hygromycin resistance was used as a control when examining the effects of hygromycin on spontaneous albinism. As shown in Table 1, 16% of regenerated plants in regeneration medium without hygromycin were found to be albino. These albino plants were able to survive on culture media for months. When hygromycin was used in the media, we observed reductions in natural albinism (Table 1). Addition of 10, 20, and 30 mg/L hygromycin in the media resulted in production of 13, 10, and 4% albino plants, respectively. A complete suppression of natural albino plants was observed with 50 mg/L hygromycin in the media. Wild type perennial ryegrass albino shoots were not capable of tolerating 50 mg/L hygromycin, which led to the elimination of spontaneous albinism and also allowed more effective selection of transgenic plants and pds mutant plants.
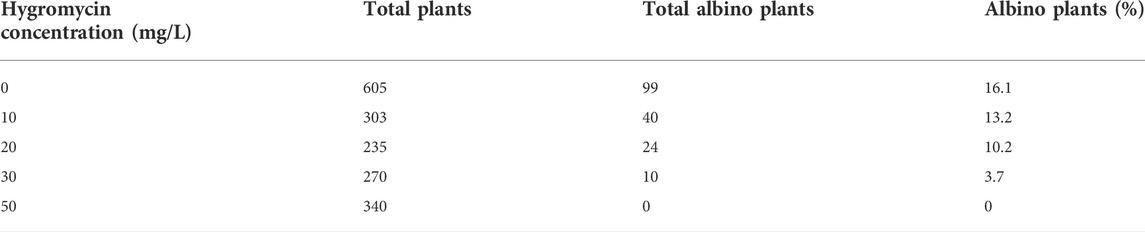
TABLE 1. Effects of hygromycin concentrations on natural albino plant percentage in perennial ryegrass tissue culture, transformed with a control vector containing no Cas9. Hygromycin resistant calli were transferred to a shoot regeneration medium supplemented with 0, 10, 20, 30, and 50 mg/L hygromycin.
Production of pds albino perennial ryegrass plants by targeted mutagenesis
The two sgRNAs in the CRISPR/Cas9 construct used in this study was originally designed for the rice PDS gene (Tang et al., 2016). One of these two sgRNAs is perfectly matched with the perennial ryegrass PDS gene sequence but another rice sgRNA is not (Figure 1S). Therefore, the characterization of mutations in the edited pds mutants of perennial ryegrass was on the sequence well matched with the rice sgRNA sequence. The CRISPR/Cas9 and control (no Cas9/sgRNA) constructs containing the hygromycin phosphotransferase gene were delivered in calli via an Agrobacterium-mediated transformation method. The embryogenic calli were subsequently transferred into a selection medium containing hygromycin (50 mg/L) consecutively for 45 days (Figure 2D). Hygromycin-resistant calli were transferred to a shoot regeneration medium containing hygromycin (50 mg/L). Shoots were visible 2 weeks after transferring calli to the shoot regeneration medium (Figures 2E,F). We did three transformation experiments and observed 25, 27, and 33% editing efficiency for the PDS gene, respectively (Table 2). Also, as expected, all albino plants from the three transformation experiments had mutations in the PDS gene, indicating that 50 mg/L hygromycin in shoot regeneration medium effectively eliminated natural albino plants.

TABLE 2. Production of pds perennial ryegrass mutants by targeted mutagenesis. Genome editing was confirmed by HindIII digestion and Sanger, and high-throughput Illumina sequencing.
Confirmation of genome editing by HindIII digestion and sanger DNA sequencing
Mutations in the PDS gene in the albino plants were confirmed using HindIII digestion and Sanger DNA sequencing. The Cas9 cleavage site coincides with the HindIII restriction enzyme recognition sequence; thus, the genome editing at the HindIII site led to disruption of the HindIII digestion. We used HindIII digestion to confirm mutations in the PDS gene in the albino plants. A pair of primers flanking the target site was used to amplify a 302 bp sgRNA-target region. A HindIII digestion of the wild type PCR product produced two bands, 214 bp and 88 bp, respectively (Figure 3A, Figure 2S). Out of 11 plants, 10 albino plants showed undigested PCR products, indicating mutations in the HindIII enzyme recognition site. Seven plants had shown completely undigested products suggesting that the PDS gene in these plants had been mutated completely. The presence of the HindIII digested products suggests no mutations in the PDS gene or mutations outside the HindIII recognition site. A 302 bp PCR amplified fragment containing the sgRNA targeting region from albino plants was sequenced using a Sanger DNA sequencing method to initially identify mutations in the albino plants. The results show various deletions in the targeted region and are consistent with the HindIII digestion results (Figures 3B,C, Figure 3S). However, we recognize that our Sanger sequencing could not identify multi-allelic mutations because we did not sub-clone PCR products into an E. coli vector and sequence these clones.
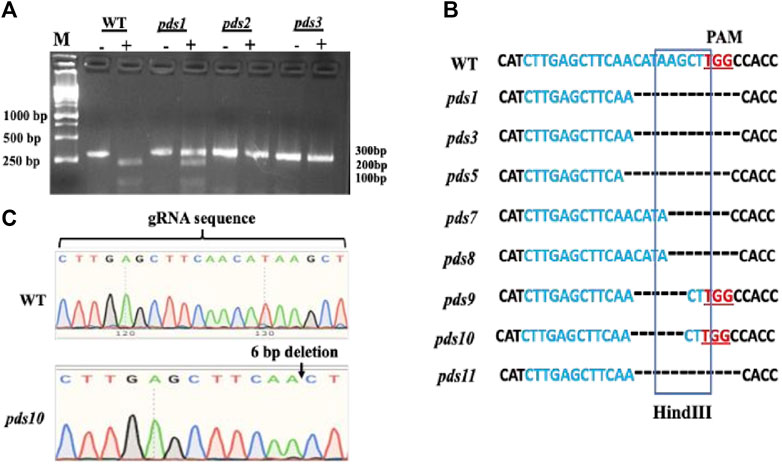
FIGURE 3. Confirmation of editing of the PDS gene by Hindlll digestion and Sanger DNA sequencing method, (A) PDS gene editing was confirmed by Hindfll digestion of PCR products. Hindlll treated PCR DNA products were run im an agarose gel. The “—” symbol indicates PCR product without restriction digestion and the “+” symbol indicates products after the restricoon digestion. PDS gene-edited pkints have mutations in the restriction site as aresuk their PCR products were not digested. (B) Mutations were confirmed by Sanger DNA sequencing of the gRNA-target region. The “—” symbol indicates muckotides deletion in the pds matants: by CRISPR/Cas9. (C) Sanger DNA sequencing sgRNA region of the chromosome DNA from the WT and pds10 masant depicting a 6 bp deletion in the target region.
Illumina sequencing of targeted mutations in perennial ryegrass pds mutants
To reveal multi-allelic mutations in the pds mutants, we used Illumina sequencing for a more detailed characterization of the mutations in representative pds mutant plant. A 199 bp long DNA spanning the targeted region was amplified from five representative albino plants and used for Illumina sequencing analysis. The sequencing results of the target region were shown in Table 3. The selected pds mutant plants had a variety of mutated sequences at and near the PAM sequence of the target site. Mutations were identified in all five plants with 1–12 bp deletions in the target region. Four plants had bi-allelic mutations, suggesting that genome editing mainly took place at the single-cell stage of shoot regeneration. Furthermore, a majority of mutants contained 1 to 2 bp deletions which result in a frame shift of the coding sequence. In addition, the sequencing results also showed that pds4 mutant contained bi-allelic mutations outside of the HindIII restriction site which could not be detected by digestion. However, we did not observe any insertion events in these mutant plants. The Illumina sequencing analysis show that most pds mutant plants we produced contained bi-allelic mutations, which reduces chances for production of chimeric plants.
Discussion
CRISPR/Cas9 systems have become powerful tools for targeted mutagenesis to study gene functions and improve crop plants. However, an efficient CRISPR/Cas9 genome editing system in perennial ryegrass is needed. In the current study, we used a single promoter based CRISPR/Cas9 system for targeted mutagenesis in perennial ryegrass using an Agrobacterium-mediated transformation method. Using ZmUbi1 promoter:RUBY transgenic plants, we demonstrated that ZmUbi1 promoter was active in callus tissues, young shoots, and entire plants, suggesting that the promoter is a good candidate for driving Cas9 and sgRNA expression in perennial ryegrass. Combining Agrobacterium-mediated transformation with a single promoter based CRISPR/Cas9 system led to a 29% gene editing efficiency in perennial ryegrass, which is a drastic improvement from the previously published protocol (Zhang et al., 2020). Further, most of the mutants produced using our system contain bi-allelic mutations.
The promoter used to drive the expression of Cas9 and sgRNAs is a key component influencing the efficiency of gene editing and also the likelihood of bi-allelic, or multi-allelic mutants in diploid plants (Yan et al., 2015; Zhang et al., 2017). If tissue culture/plant regeneration procedures are used for development of gene edited plants, promoters that are active in callus cells before differentiated into shoots or embryos should be good choices for high efficiencies of gene-editing and bi-allelic mutant plant production (Ma et al., 2015; Wang et al., 2015; Osakabe et al., 2016; Zhuo et al., 2021). Because few promoters have been characterized in perennial ryegrass and other turf grasses, our evaluation of the spatial and temporal expression patterns of the ZmUbi1 promoter in perennial ryegrass generates useful information for the utility of the promoter in gene editing and other applications. That fact that most of our pds mutant plants we produced have bi-allelic mutations indicates that gene editing took place during the single-cell stage of shoot regeneration, which further confirms the ZmUbi1 promoter is a good choice for controlling Cas9 and sgRNA expression. Also, the high activity the ZmUbi1 promoter in callus cells should be a basis for the relatively high gene editing efficiency we have observed.
The use of the STU (single transcription unit) system for both Cas9 and sgRNA helps to achieve a coordinated expression of Cas9 and sgRNAs in the same cell at the same time, which should also contribute to high editing efficiency reported here. Coordinated expression of Cas9 and sgRNAs can sometimes be a challenge if two different gene promoters are used to control the expression of Cas9 and sgRNA, respectively (Tang et al., 2019). The sole perennial ryegrass gene editing study published was done by using a dual promoter based CRISPR system (Zhang et al., 2020). In addition to the fact that they produced only one gene edited plants, the editing in that edited plant was about 75% (Zhang et al., 2020). While we conclude that STU contributes to higher editing efficiency as we reported here, it is also possible sgRNA sequences used may contribute to the differences in the editing efficiencies between a STU we used and a two transcript system reported by Zhang et al. (2020). On the other hand, with the STU CRISPR/Cas9 system to co-express Cas9 and sgRNA, along with the use of a hammerhead ribozyme system (Tang et al., 2016; Tang et al., 2019), functional Cas9 mRNA and sgRNAs can be produced from a single transcript. Such an expression system leads to expression of Cas9 and sgRNAs in the same cell at the same time, which should contribute to the high editing efficiencies in perennial ryegrass as we have observed. We therefore suggest that a STU CRISPR/Cas9 system should be a better choice for coordinated expression of both Cas9 and sgRNAs in order to achieve high editing efficiencies for perennial ryegrass and other closely related species.
Agrobacterium and particle bombardment transformation methods have been used for genetic transformation of perennial ryegrass, but Agrobacterium is a much simpler and easier method with high efficiency (Hansen and Wright, 1999; Travella et al., 2005; Zhang and Zheng, 2008; Patel et al., 2013; Zhang et al., 2013; Zhang et al., 2020). Zhang et al. (2020) used a particle bombardment-mediated transformation method in their CRISPR/Cas9-mediated gene editing study, but their transformation efficiency was 2.66%. In our case, we used Agrobacterium-mediated transformation to deliver the Cas9 and sgRNA into perennial ryegrass and our transformation efficiency was 20%, consistent with the transformation efficiencies previously reported (Patel et al., 2013; Zhang et al., 2013). Thus, we suggest that Agrobacterium should be used for delivery of Cas9 and sgRNAs into perennial ryegrass for high editing efficiency.
In summary, we have demonstrated a significant improvement in genome editing efficiency in perennial ryegrass compared to the only published method (Zhang et al., 2020). The key component of our method is to use Agrobacterium to deliver the CRISPR/Cas9 system that produces a STU for Cas9 and sgRNA driven by the ZmUbi1 gene promoter. The method described here should also be applicable for other related grass species.
Data availability statement
The datasets presented in this study can be found in online repositories. The names of the repository/repositories and accession number(s) can be found below: https://www.ncbi.nlm.nih.gov/, SAMN26236011; https://www.ncbi.nlm.nih.gov/, OM849229-OM849237.
Author contributions
RK performed the experiments, analyzed the DNA sequencing data, organized and interpreted the data. DT helped the transformation of Agrobacterium with plasmid DNA. RK, RB TK, DT, HY and YZ were involved in manuscript writing and editing. YL supervised the experiments, data organization and interpretation, and edited manuscript.
Funding
This project is financially supported by BARENBRUG to YL.
Conflict of interest
The authors declare that the research was conducted in the absence of any commercial or financial relationships that could be construed as a potential conflict of interest.
Publisher’s note
All claims expressed in this article are solely those of the authors and do not necessarily represent those of their affiliated organizations, or those of the publisher, the editors and the reviewers. Any product that may be evaluated in this article, or claim that may be made by its manufacturer, is not guaranteed or endorsed by the publisher.
Supplementary material
The Supplementary Material for this article can be found online at: https://www.frontiersin.org/articles/10.3389/fgeed.2022.960414/full#supplementary-material
References
Ahmar, S., Gill, R. A., Jung, K.-H., Faheem, A., Qasim, M. U., Mubeen, M., et al. (2020). Conventional and molecular techniques from simple breeding to speed breeding in crop plants: Recent advances and future outlook. Int. J. Mol. Sci. 21, 2590. doi:10.3390/ijms21072590
Byrne, S. L., Nagy, I., Pfeifer, M., Armstead, I., Swain, S., Studer, B., et al. (2015). A synteny-based draft genome sequence of the forage grass Lolium perenne. Plant J. 84, 816–826. doi:10.1111/tpj.13037
Chen, L., Li, W., Katin-Grazzini, L., Ding, J., Gu, X., Li, Y., et al. (2018). A method for the production and expedient screening of CRISPR/Cas9-mediated non-transgenic mutant plants. Hortic. Res. 5, 13. doi:10.1038/s41438-018-0023-4
Emmons, R., and Rossi, F. (2015). Turfgrass science and management. Boston, Massachusetts, United States: Cengage Learning.
Grinberg, N. F., Lovatt, A., Hegarty, M., Lovatt, A., Skøt, K. P., Kelly, R., et al. (2016). Implementation of genomic prediction in lolium perenne (L.) breeding populations. Front. Plant Sci. 7, 133. doi:10.3389/fpls.2016.00133
Hansen, G., and Wright, M. S. (1999). Recent advances in the transformation of plants. Trends Plant Sci. 4, 226–231. doi:10.1016/s1360-1385(99)01412-0
He, Y., Zhang, T., Sun, H., Zhan, H., and Zhao, Y. (2020). A reporter for noninvasively monitoring gene expression and plant transformation. Hortic. Res. 7, 152. doi:10.1038/s41438-020-00390-1
Hua, K., Zhang, J., Botella, J. R., Ma, C., Kong, F., Liu, B., et al. (2019). Perspectives on the application of genome-editing technologies in crop breeding. Mol. Plant 12, 1047–1059. doi:10.1016/j.molp.2019.06.009
Jiang, Y., and Huang, B. (2001). Physiological responses to heat stress alone or in combination with drought: A comparison between tall fescue and perennial ryegrass. HortScience 36, 682–686. doi:10.21273/hortsci.36.4.682
Li, H., and Durbin, R. (2009). Fast and accurate short read alignment with Burrows–Wheeler transform. bioinformatics 25, 1754–1760. doi:10.1093/bioinformatics/btp324
Liu, P., Zhang, Z.-X., Yuan, J.-G., Xi, J.-B., Du, X.-L., and Yang, Z.-Y. (2006). Callus induction and plant regeneration in eleven perennial ryegrass cultivars. Biotechnol. Biotechnol. Equip. 20, 30–37. doi:10.1080/13102818.2006.10817377
Ma, X., Zhang, Q., Zhu, Q., Liu, W., Chen, Y., Qiu, R., et al. (2015). A robust CRISPR/Cas9 system for convenient, high-efficiency multiplex genome editing in monocot and dicot plants. Mol. Plant 8, 1274–1284. doi:10.1016/j.molp.2015.04.007
Osakabe, Y., and Osakabe, K. (2015). Genome editing with engineered nucleases in plants. Plant Cell Physiol. 56, 389–400. doi:10.1093/pcp/pcu170
Osakabe, Y., Takahito, W., Shigeo, S. S., Risa, U., Ryosuke, I., Kazuo, S., et al. (2016). Optimization of CRISPR/Cas9 genome editing to modify abiotic stress responses in plants. Sci. Rep. 6, 26685–26710. doi:10.1038/srep26685
Patel, M., Dewey, R. E., and Qu, R. (2013). Enhancing Agrobacterium tumefaciens-mediated transformation efficiency of perennial ryegrass and rice using heat and high maltose treatments during bacterial infection. Plant Cell Tissue Organ Cult. 114, 19–29. doi:10.1007/s11240-013-0301-7
Tang, X., Ren, Q., Yang, L., Bao, Y., Zhong, Z., He, Y., et al. (2019). Single transcript unit CRISPR 2.0 systems for robust Cas9 and Cas12a mediated plant genome editing. Plant Biotechnol. J. 17, 1431–1445. doi:10.1111/pbi.13068
Tang, X., Zheng, X., Qi, Y., Zhang, D., Cheng, Y., Tang, A., et al. (2016). A single transcript CRISPR-cas9 system for efficient genome editing in plants. Mol. Plant 9, 1088–1091. doi:10.1016/j.molp.2016.05.001
Travella, S., Ross, S. M., Harden, J., Everett, C., Snape, J. W., and Harwood, W. A. (2005). A comparison of transgenic barley lines produced by particle bombardment and Agrobacterium-mediated techniques. Plant Cell Rep. 23, 780–789. doi:10.1007/s00299-004-0892-x
Wang, Z. P., Xing, H. L., Dong, L., Zhang, H. Y., Han, C. Y., Wang, X. C., et al. (2015). Egg cell-specific promoter-controlled CRISPR/Cas9 efficiently generates homozygous mutants for multiple target genes in Arabidopsis in a single generation. Genome Biol. 16, 144–212. doi:10.1186/s13059-015-0715-0
Wise, A. A., Liu, Z., and Binns, A. N. (2006). Three methods for the introduction of foreign DNA into Agrobacterium. Methods Mol. Biol. 343, 43–53. doi:10.1385/1-59745-130-4:43
Xiong, J.-S., Ding, J., and Li, Y. (2015). Genome-editing technologies and their potential application in horticultural crop breeding. Hortic. Res. 2, 15019. doi:10.1038/hortres.2015.19
Yan, L., Wei, S., Wu, Y., Hu, R., Li, H., Yang, W., et al. (2015). High-efficiency genome editing in Arabidopsis using YAO promoter-driven CRISPR/Cas9 system. Mol. Plant 8, 1820–1823. doi:10.1016/j.molp.2015.10.004
Zhang, F., LeBlanc, C., Irish, V. F., and Jacob, Y. (2017). Rapid and efficient CRISPR/Cas9 gene editing in Citrus using the YAO promoter. Plant Cell Rep. 36, 1883–1887. doi:10.1007/s00299-017-2202-4
Zhang, W. J., Dewey, R. E., Boss, W., Phillippy, B. Q., and Qu, R. (2013). Enhanced Agrobacterium-mediated transformation efficiencies in monocot cells is associated with attenuated defense responses. Plant Mol. Biol. 81, 273–286. doi:10.1007/s11103-012-9997-8
Zhang, Y., Ran, Y., Nagy, I., Lenk, I., Qiu, J.-L., Asp, T., et al. (2020). Targeted mutagenesis in ryegrass (Lolium spp.) using the CRISPR/Cas9 system. Plant Biotechnol. J. 18, 1854–1856. doi:10.1111/pbi.13359
Zhang, Z. X., and Zheng, Y. Z. (2008). Overexpression of nicotianamine synthase (NAS) gene results in enhanced drought tolerance in perennial ryegrass. Biotechnol. Biotechnol. Equip. 22, 938–941. doi:10.1080/13102818.2008.10817583
Keywords: perennial ryegrass, single promoter, CRISPR/Cas9, PDS, ZmUbi1, ruby, genome editing, single transcript unit
Citation: Kumar R, Kamuda T, Budhathoki R, Tang D, Yer H, Zhao Y and Li Y (2022) Agrobacterium- and a single Cas9-sgRNA transcript system-mediated high efficiency gene editing in perennial ryegrass. Front. Genome Ed. 4:960414. doi: 10.3389/fgeed.2022.960414
Received: 02 June 2022; Accepted: 15 August 2022;
Published: 06 September 2022.
Edited by:
Nian Wang, University of Florida, United StatesReviewed by:
Guiyan Huang, Gannan Normal University, ChinaYinong Yang, Pennsylvania State University (PSU), United States
Copyright © 2022 Kumar, Kamuda, Budhathoki, Tang, Yer, Zhao and Li. This is an open-access article distributed under the terms of the Creative Commons Attribution License (CC BY). The use, distribution or reproduction in other forums is permitted, provided the original author(s) and the copyright owner(s) are credited and that the original publication in this journal is cited, in accordance with accepted academic practice. No use, distribution or reproduction is permitted which does not comply with these terms.
*Correspondence: Yi Li, eWkubGlAdWNvbm4uZWR1