- Department of Cellular and Integrative Physiology, The University of Texas Health Science Center at San Antonio, San Antonio, TX, United States
Neurological conditions like neurodevelopmental disorders and neurodegenerative diseases are quite complex and often exceedingly difficult for patients. Most of these conditions are due to a mutation in a critical gene. There is no cure for the majority of these neurological conditions and the availability of disease-modifying therapeutics is quite rare. The lion’s share of the treatments that are available only provide symptomatic relief, as such, we are in desperate need of an effective therapeutic strategy for these conditions. Considering the current drug development landscape, gene therapy is giving us hope as one such effective therapeutic strategy. Consistent efforts have been made to develop gene therapy strategies using viral and non-viral vectors of gene delivery. Here, we have discussed both of these delivery methods and their properties. We have summarized the relative advantages and drawbacks of viral and non-viral vectors from the perspectives of safety, efficiency, and productivity. Recent developments such as clustered regularly interspaced short palindromic repeats (CRISPR)/Cas9-mediated gene editing and its use in vivo have been described here as well. Given recent advancements, gene therapy shows great promise to emerge as a next-generation therapeutic for many of the neurodevelopmental and neurodegenerative conditions.
Introduction
The prevalence of neurological disorders has been rising across the globe and is often associated with an increasing socioeconomic burden. Many of these debilitating neurological conditions do not respond to conventional therapies. Therefore, it is imperative to explore novel treatment modalities like gene therapy. Recent groundbreaking gene therapy trials on patients with spinal muscular atrophy type 1 (SMA1) resulted in superior motor function and longer survival compared to the control group (Mendell et al., 2017). These investigations advocate for the further development of gene therapy strategies to treat neurological conditions by replacing the disease-causing mutant gene with a healthy copy or inactivating the malfunctioning disease-causing mutant gene. RNA interference (RNAi) technology, has been one of the popular approaches to inactivate target gene expression accomplished by a small non-coding RNA of varying length that binds a complementary sequence of the mRNA target (Davidson and Boudreau, 2007; Jagannath and Wood, 2007; Aguiar et al., 2017; Balwani et al., 2020). Besides these, studies have been conducted in recent years to edit the mutated copy of the gene itself in vitro and in vivo. Such an approach is known as gene editing (Li et al., 2020).
Clustered regularly interspaced short palindromic repeats (CRISPR)/Cas9 is one of the widely used gene editing tools. This method requires the delivery of Cas9 ribonucleoprotein (RNP) consisting of the Cas9 protein with guide RNA (gRNA) to the target cells. CRISPR/Cas9-mediated genome engineering is quite precise and straightforward due to the target specificity and simple design of gRNA. Studies employing CRISPR have already exhibited efficiency in preclinical models of neurological disorders (Gaj et al., 2017; Yang et al., 2017; György et al., 2018; Lee et al., 2018; Ekman et al., 2019; Ricci and Colasante, 2021). Moreover, a recent report suggests that CRISPR technology is in fact promising in clinical trials (Frangoul et al., 2021). While we expect CRISPR-based gene editing will soon change the therapeutic landscape of complex neurological disorders, the double-stranded break it creates in the host genome is the major drawback of Cas9-mediated editing. The non-homologous end joining (NHEJ) pathway is error-prone and often associated with undesired insertion or deletion (indel) mutations (Moore and Haber, 1996; Kosicki et al., 2018; Song et al., 2021). Further, non-dividing cells are incapable of undergoing homology-directed repair (HDR)-mediated editing which reduces the overall efficiency of these processes (Iyama and Wilson, 2013; Kantor et al., 2020). Base editing offers an alternative that could overcome these challenges given base editors do not rely on double-stranded DNA break (Komor et al., 2016; Eid et al., 2018; Anzalone et al., 2020). The two major base editors, cytosine base editors (CBEs) and adenine base editors (ABEs) can introduce all four transition mutations (A→G, G→A, C→T, T→C) (Gaudelli et al., 2017; Qi et al., 2020; Qin et al., 2020; Yu et al., 2020). In principle, ∼30% of all known human pathogenic single nucleotide polymorphisms (SNPs) can be targeted using base editors (Anzalone et al., 2020). Prime editors, the newest players in the league, are capable of introducing all four transitions, as well as all eight transversion mutations. Theoretically, prime editing can edit ∼89% of all known human pathogenic SNPs (Kantor et al., 2020). Hence, base editing and prime editing tools are perceived as extremely powerful strategies to target disease-causing point mutations. Such progress will be translated to patients and pave the way for gene therapy to be the next generation therapeutic by reverting the disease-causing mutation to the normal gene, a remarkable way to treat a disease.
Successful gene therapy strategies often rely on successful gene delivery methods. There are broadly two categories of gene delivery strategies; viral vector-mediated and nonviral vector-mediated gene delivery. However, apart from these two strategies, physical methods have also been tested as a gene delivery strategy. Nevertheless, due to a lack of target specificity, physical methods such as sonoporation are not widely accepted in the field. Here, we narrate the major gene delivery strategies and their relative advantages and disadvantages for brain targeting. Broadly, viral vectors are considered to be more efficient while non-viral vectors are on the whole less toxic and immunogenic. However, both delivery methods show exciting and hopeful recent advancements.
Viral Vector-Mediated Gene Delivery
Viruses have been an alluring vector for gene therapy due to their efficiency infecting and delivering genetic material to cells. However, selecting an appropriate viral vector for gene therapy requires stringent parameters. Generally, there are three universally accepted criteria for a good gene delivery viral vector (Scheller and Krebsbach, 2009); 1. safe and non-immunogenic, 2. able to protect the transgene, and 3. capable of prolonged and tissue-specific transgene expression (if applicable). Considering these factors in mind, adenoviruses, lentiviruses, and adeno-associated viruses (AAVs) have been used to develop effective gene delivery vehicles targeting a wide range of neurological indications (Lapchak et al., 1997; Azzouz et al., 2002; Albert et al., 2017; Mendell et al., 2017). As viral vectors manifest a high gene delivery efficiency in vivo, they have received the limelight in the current decade for their use in gene therapy. Their applications are summarized in Table 1 and briefly described below:
Adenovirus-based vectors: Adenoviral vectors can package 5–10 kb of naked double-stranded DNA (dsDNA) (He et al., 1998). They can infect quite a broad range of cells and express episomally (Leblois et al., 2000; Kreppel and Kochanek, 2004; Shu et al., 2016). Two of the major drawbacks of the adenoviral vectors have been their immunogenicity and low efficiency to cross blood-brain-barrier (BBB) (Kafri et al., 1998; Franklin et al., 1999; Tang et al., 2007; Lundstrom, 2018). Recently, efforts were made to utilize the transcellular transport pathway to facilitate the BBB penetration of adenovirus serotype-5 (Ad5) vectors. Re-direction of Ad5 vectors to melanotransferrin transcytosis system promoted Ad5-mediated gene delivery through BBB (Tang et al., 2007).
Lentivirus-based vectors: Lentiviral vectors are categorized under the retroviruses and are capable of infecting both dividing and non-dividing cells (Naldini et al., 1996; Blömer et al., 1997; Yang et al., 2008; Lundstrom, 2018). These vectors can package up to 10 kb of single-stranded RNA (ssRNA) manifesting long-term transgene expression (Sakuma et al., 2012; Lundstrom, 2018; Kalidasan et al., 2021). One key concern for the use of lentiviral vectors has been the possibility of their integration into the host genome leading to insertional mutagenesis. Integration-defective lentiviral vectors have been developed to circumvent the possibilities of insertional mutagenesis in the brain (Philippe et al., 2006).
AAV-based vectors: AAV-based vectors generally carry single-stranded DNA (ssDNA) and can package ∼4.8 kb of content (Carvalho et al., 2017; Lundstrom, 2018). Their ability to infect a wide range of dividing and non-dividing cells makes them suitable for many therapeutic trials. The existence of more than 12 serotypes and their various features have made AAV-based vectors the most attractive vehicle (Ingusci et al., 2019). A number of natural AAV serotypes exhibit both anterograde and retrograde trafficking while natural serotypes such as AAV1, AAV2, AAV6, and AAV9 require high vector doses for retrograde trafficking due to the relative inefficiency (Haery et al., 2019). AAV1, AAV5, AAV8, and AAV9 transduce neurons as well as astrocytes and oligodendrocytes while AAV2 transduce mostly neurons (Tenenbaum et al., 2004; Haery et al., 2019). Compared to other serotypes, AAV9 manifests superior BBB crossing ability when assessed in neonatal mouse CNS (Foust et al., 2009; Zhang et al., 2011; Haery et al., 2019). Various AAV serotypes can either exist as an episome or can integrate into the host genome. AAV vectors are often immunogenic, however, the use of different serotypes for subsequent administrations has shown promise in combating this (Lundstrom, 2018).
Advantages of Using Viral Gene Delivery
In recent days, the AAV vectors have gained a lot of attention for gene therapy given their broad range of cell tropism and suitability for subsequent engineering (Srivastava, 2016; Li and Samulski, 2020). Recombinant AAV vectors have unique features such as their capability to transport through extracellular space due to the small particle size. Furthermore, replication-incompetent AAV vectors are often considered as one of the safer options for in vivo use (Hudry and Vandenberghe, 2019). They can elicit a stable and sustained transgene expression as an episome. Recently, the field has seen enormous progress in developing AAV-mediated targeted gene therapy approaches in the CNS (see Table 1).
Disadvantages of Using Viral Gene Delivery
Despite having numerous advantages, AAV-mediated gene delivery requires further fine-tuning. Some of the critical challenges include identifying a safe and less-invasive route of administration, overcoming large-scale manufacturing obstacles, and successful translation from preclinical models to humans (complicated by immunological differences and presence of BBB). Besides these, another key challenge has been the delivery of larger cargos. Recent advancements in gene editing technology require the delivery of massive machinery like CRISPR/Cas9 to target cells. However, considering the limited carrying capacity of AAV, the cargo would have to be split into two or more vectors (Trapani, 2019; Akil, 2020). Such an approach reduces transduction efficiency as the chances of simultaneous reach of multiple vectors to the target cells become stochastic. Therefore, it is important to have a vehicle that can carry larger complexes to target cells.
Non-Viral Vector-Mediated Gene Delivery
Non-viral vectors have been used as a vehicle for many different gene therapy trials even though the system has not gotten the same fame seen with the viral vector models. Nevertheless, non-viral vectors have shown promise in many of the aspects of an ideal gene therapy vector. Broadly, there are three major strategies of non-viral vector-mediated gene therapy such as polymer-based vectors, lipid-based vectors, and nanoparticle-based vectors (Mintzer and Simanek, 2009; Jayant et al., 2016; Salameh et al., 2020). See Table 1 for their applications.
Polymer-based vectors: Polyethyleneimine (PEI) and poly-L-Lysine (PLL) are cationic polymers which are popularly tested in vivo CNS for gene therapy (Boussif et al., 1995; Mousazadeh et al., 2007; Mintzer and Simanek, 2009; Sheikh et al., 2017; Zheng et al., 2021). Each polymer-based vector demonstrates distinct features; PEI is versatile given it can be designed to be different lengths, be branched or linear, undergo functional group substitution or addition (Morille et al., 2008; Jayant et al., 2016); PLL is unique due to its biodegradable nature, which is advantage for in vivo use (Morille et al., 2008; Jayant et al., 2016). Studies demonstrated that cytotoxicity of PEI and PLL is directly related to molecular weight and pKa, with higher molecular weight and more cationic materials being more toxic (Mintzer and Simanek, 2009; Monnery et al., 2017). Further investigation showed PLL conjugated with apoprotein E (apoE)-derived peptide demonstrated to cross BBB (Mousazadeh et al., 2007). Dendrimers, highly branched spherical polymers, have also been widely investigated as a gene delivery vehicle. Polyamidoamine (PAMAM) is the most common form of a dendrimer due to its ample transfection efficiency (Wang et al., 2011; Zhu et al., 2019; Mignani et al., 2021). Cytotoxicity of dendrimers as a result of their surface charge and chemical structure was shown to be alleviated by polyethylene glycol (PEG) modification (PEGylation) (Jayant et al., 2016). Additionally, a single intranasal administration of PAMAM dendrimers was shown to modulate brain-derived neurotrophic factor gene expression in the brain (Win-Shwe et al., 2014).
Lipid-based vectors: Liposomes consist of spherical concentric bilipid layers capable of carrying nucleic acid to target cells (Gao et al., 2013). Liposomes have been widely used as non-viral vectors for CNS targeting (Zhang et al., 2003; Zhang et al., 2004; Dos Santos Rodrigues et al., 2019). However, simple liposomes face multiple obstacles including lysosome-mediated degradation and reduced nuclear uptake. Cationic liposomes are comparatively more efficient in transfecting larger nucleic acids and are easy to handle, though, they tend to form an aggregate in biological fluids (Jayant et al., 2016; Ewert et al., 2021). Niosomes are more stable than liposomes (Bartelds et al., 2018; Ge et al., 2019). The major limitation of niosomes has been the possible aggregation, fusion, and leaking, which could be circumvented by the usage of proniosomes (Hu and Rhodes, 2000). Further, PEGylation of niosomes led to improved gene delivery, reduced interaction with plasma proteins, and prohibited aggregation in the serum (Huang et al., 2008). To foster the BBB crossing, trojan horse liposomes (THL) has been developed. THL relies on its monoclonal antibody component to bind with the cognate receptors (e.g., transferrin receptors or insulin receptors) present on BBB or the cell surface (Boado and Pardridge, 2011). Another approach is designing dual-functionalized liposomes having penetratin and transferrin attached to the surface (Dos Santos Rodrigues et al., 2018; Dos Santos Rodrigues et al., 2019).
Nanoparticle-based vectors: Nanoparticle-based vectors are at the forefront of gene delivery modalities as a result of their safety profile as well as their cost-effective production method. Nanoparticles are defined as solid colloidal particles with sizes ranging from 1 to 1000 nm consisting of macromolecular materials in which the active compound (drug/biologically active material e.g., DNA/RNA/protein) is encapsulated, absorbed, or entrapped (Kreuter, 2014). Generally, nanoparticles are biocompatible and readily biodegradable, making them suitable gene delivery vehicles for CNS targeting (Calzoni et al., 2019). Major components of nanoparticles are poly-butylcyanoacrylide (PBCA), poly-lactic acid (PLA) and related copolymers (Kreuter, 2014). In many cases, modulating the surface properties of these nanoparticles by PEGylation, or polysorbate-80 coating improves gene delivery efficiency. PEGylation, in particular, prolongs systemic circulation time and reduces the immunogenicity of the nanoparticles (Suk et al., 2016). Whereas, polysorbate-80 coating was shown to enhance BBB crossing (Ren et al., 2009). Similar to liposomes, functionalizing nanoparticles with specific ligands shown to be capable to cross BBB (Lombardo et al., 2020). For example, ApoE-modified nanoparticles have been shown to circumvent BBB (Wagner et al., 2012). Other investigations demonstrated that insulin-targeted gold nanoparticles can effectively cross BBB via receptor-mediated endocytosis (Shilo et al., 2014). Gold nanoparticle was also shown to deliver the large cargo such as CRISPR/Cas9 RNP leading to a behavioral rescue in a preclinical mouse model of fragile X syndrome (FXS) without showing any significant cytotoxicity (Horejs, 2018; Lee et al., 2018; Trenkmann, 2018).
Advantages of Using Non-Viral Gene Delivery
Non-viral vectors have multiple advantages over viral vectors. Lower toxicity and immunogenicity make non-viral vectors a safer tool for gene delivery. Other advantages of the non-viral vectors would be the ease of production, thereby, cost-effectiveness, and their ability to be engineered (Ramamoorth and Narvekar, 2015). On top of that, non-viral vectors can transfer bigger sizes of nucleic acid residues and/or proteins (Morrell et al., 2008; Chang et al., 2017; Lee et al., 2018; Park et al., 2019; Zhang et al., 2021; Jubair et al., 2021; O'Keeffe Ahern et al., 2022). Considering the current progress in gene editing technology, transferring larger biomolecule complexes is desired.
Disadvantages of Using Non-Viral Gene Delivery
Regardless of many advantages over the viral vectors, non-viral vectors do face some challenges. Firstly, the comprehensive mechanisms of their actions are not well elucidated (Wu et al., 2018). Hence, it has been difficult to strike the optimum balance between efficiency and toxicity. Secondly, non-viral vectors generally exhibit lower efficiency for CNS targeting compared to viral vectors (Mintzer and Simanek, 2009; Jayant et al., 2016). However, recent advancements in chemical modifications of the lipid carriers and the other non-viral vectors are promising in enhancing CNS targeting. Another hurdle has been ‘protein corona’ formation (Corbo et al., 2016). Systemically administered lipid nanoparticles encounter and interact with countless biomolecules which change their surface properties and form protein coronas (Caracciolo, 2015; Giulimondi et al., 2019). This phenomenon can impact cellular uptake, biodistribution, immune reaction, and toxicity of the vector (Corbo et al., 2016). Therefore, it is imperative to develop a safe yet efficient delivery modality.
Discussion
Gene therapy for complex neurological conditions including neurodevelopmental and neurodegenerative disorders is still in its adolescence. The field has encountered a lot of challenges in the last couple of decades. One of the major challenges has been safety and tolerability. Subsequent efforts and advancements have led to many successful clinical trials addressing the safety concern. Nevertheless, we have seen a surge in the number of clinical trials targeting complex disorders employing gene therapy approaches in the last couple of decades (Kaplitt et al., 2007; Mittermeyer et al., 2012; Palfi et al., 2014; Mendell et al., 2017; Palfi et al., 2018; Christine et al., 2019). It is noteworthy to mention that the proportion of these gene therapy trials is still not on par with conventional small-molecule therapeutic development. Therefore, it is critical to reiterate that more efforts are needed for the successful translation of gene therapy strategies from bench to bedside. Gene therapy could be a game-changer in treating rare monogenic disorders in the years to come. Debilitating indications like amyotrophic lateral sclerosis (ALS) and Huntington’s disease (HD) are quite aggressive and lack an effective disease-modifying therapy. In most cases, the only treatment available is symptomatic alleviation. In such a scenario, targeting the underlying etiology could be both beneficial and advantageous. The development of gene therapy strategies has shown us a light at the end of the tunnel.
Successful gene delivery strategies are often the rate-limiting step for efficient gene therapy (see Figure 1). Viral vectors have been designed to evade the immune system and deliver their nucleic acids to target organs (Chan et al., 2021). Such a function constitutes one of the mechanisms of increased efficiency of viral vector-mediated gene delivery. The American Society of Gene & Cell Therapy (ASGCT) proclaimed in the Q1-2021 quarterly data report that 89% of the total gene therapies in development employ viral vectors including AAV (42%), lentivirus (29.9%), and adenovirus (12.6%). A similar trend is expected for the gene therapy landscape of neurological disorders. Considering current gene therapy preclinical development, neurological disorders are the second most widely targeted non-cancer non-rare indications. Furthermore, Zolgensma, the only FDA-approved gene therapy against SMA utilizes the AAV9 vector, indicating that viral vectors have been the primary choice (Al-Zaidy et al., 2019; Urquhart, 2019).
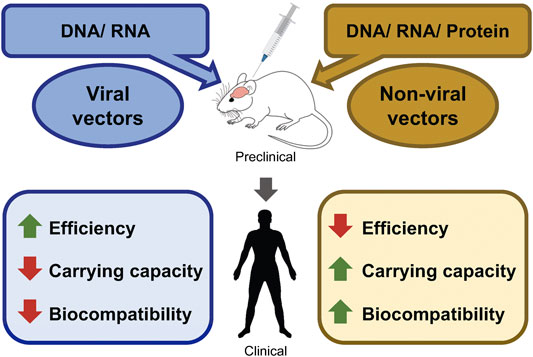
FIGURE 1. Perspectives of advantages/disadvantages of viral-, and non-viral gene delivery methods. Schematic diagram shows the possible packaging materials of viral and non-viral vectors. Packaged vectors can be tested in the preclinical models of various neurological disorders. Followed by successful preclinical trials, human clinical trials can be conducted. The advantages and the disadvantages of each vector are marked in green and red, respectively.
Gene delivery strategies are rapidly evolving to deliver a wide assortment of bioactive materials such as nucleic acids, proteins, or gene editing tools into the brain. However, bioactive materials (e.g., nucleic acids) are susceptible to degradation by serum nucleases and need protective modifications for successful delivery to the cells (Chiou et al., 1994; Juliano, 2016; Roberts et al., 2020). Therefore, current progress towards designing non-viral vectors which can protect bioactive materials against degradation inside the body becomes promising. Gene delivery through PEGylated liposomes can effectively protect nucleic acids while retaining their bioavailability (Suk et al., 2016). In addition, toxicity has been one of the major concerns masking the flare of gene therapy strategies. A good number of efforts have been made to design biocompatible and biodegradable cargo for safe gene delivery (Han et al., 2000). Non-viral vectors have shown an upper hand when it comes to safe gene delivery to the target tissues. Studies are ongoing to make non-viral vectors more efficient above and beyond being safe. Current advancements in gene editing technology also contribute to the popularization of non-viral vectors.
Lastly, most of the efficient viral vectors (AAV-mediated vectors) have limitations in their carrying capacity, whereas, non-viral vectors can carry larger DNA/RNA as well as proteins quite effortlessly (see Figure 1). This makes them suitable to deliver gene editing machinery such as CRISPR/Cas9. The development of virus-like particles (VLP) which are composed of virus assembly proteins, but lack the viral genetic material is one of the recent advancements in the field to overcome the limitations of both types of vectors. VLPs have become an attractive vehicle as they possess a similar efficiency to viral vectors without having the associated risk of genomic integration of viral gene construct. VLP-mediated gene editing was successful in ex vivo human cells as well as in vivo mouse brains (Banskota et al., 2022). With these results, VLP could turn out to be an efficient gene therapy vector in the future.
In conclusion, gene therapy is undoubtedly one of the crucial developments of this century. No wonder the Nobel prize 2020 (Chemistry) was conferred to Drs. Jennifer Doudna and Emmanuelle Charpentier for their discoveries in CRISPR/Cas9-mediated gene editing. The cutting-edge technological advancements in gene therapy are giving hope to millions of people suffering from excruciating neurological conditions. Despite great progress in the field, we are still dealing with challenges in bringing gene therapy medicines to market. Nevertheless, the consistent efforts and developments by experts across the globe are encouraging. With the increase in cross-functional collaborations in these sectors, we can expect to see various approved gene therapy treatments for patients in the near future. Gene therapy will emerge as next-generation therapeutics for many neurodevelopmental and neurodegenerative diseases in the decades to come.
Author Contributions
AP conceived the topic ideas and wrote the manuscript. MC contributed to drafting and revising the manuscript. HYL supervised the whole project and wrote the manuscript.
Funding
This work was supported by the National Institute of Mental Health (R01MH125979), the National Institute on Aging (R21AG072423), the UT Rising STARs award, and the Simons Foundation Autism Research Initiative (SFARI) pilot award (#574967).
Conflict of Interest
The authors declare that the research was conducted in the absence of any commercial or financial relationships that could be construed as a potential conflict of interest.
Publisher’s Note
All claims expressed in this article are solely those of the authors and do not necessarily represent those of their affiliated organizations, or those of the publisher, the editors and the reviewers. Any product that may be evaluated in this article, or claim that may be made by its manufacturer, is not guaranteed or endorsed by the publisher.
Acknowledgments
We acknowledge Dr. Niren Murthy and his research team for fruitful discussions and insightful comments on gene editing strategies in neurological disorders.
References
Aguiar, S., van der Gaag, B., and Cortese, F. A. B. (2017). RNAi Mechanisms in Huntington's Disease Therapy: siRNA versus shRNA. Transl. Neurodegener. 6, 30. doi:10.1186/s40035-017-0101-9
Akil, O. (2020). Dual and Triple AAV Delivery of Large Therapeutic Gene Sequences into the Inner Ear. Hear. Res. 394, 107912. doi:10.1016/j.heares.2020.107912
Al-Zaidy, S., Pickard, A. S., Kotha, K., Alfano, L. N., Lowes, L., Paul, G., et al. (2019). Health Outcomes in Spinal Muscular Atrophy Type 1 Following AVXS-101 Gene Replacement Therapy. Pediatr. Pulmonol. 54 (2), 179–185. doi:10.1002/ppul.24203
Albert, K., Voutilainen, M. H., Domanskyi, A., and Airavaara, M. (2017). AAV Vector-Mediated Gene Delivery to Substantia Nigra Dopamine Neurons: Implications for Gene Therapy and Disease Models. Genes (Basel) 8 (2). doi:10.3390/genes8020063
Anzalone, A. V., Koblan, L. W., and Liu, D. R. (2020). Genome Editing with CRISPR-Cas Nucleases, Base Editors, Transposases and Prime Editors. Nat. Biotechnol. 38 (7), 824–844. doi:10.1038/s41587-020-0561-9
Arora, S., Kanekiyo, T., and Singh, J. (2022). Functionalized Nanoparticles For Brain Targeted BDNF Gene Therapy To Rescue Alzheimer's Disease Pathology in Transgenic Mouse Model. Int. J. Biol. Macromol. 208, 901–911. doi:10.1016/j.ijbiomac.2022.03.203
Azzouz, M., Martin-Rendon, E., Barber, R. D., Mitrophanous, K. A., Carter, E. E., Rohll, J. B., et al. (2002). Multicistronic Lentiviral Vector-Mediated Striatal Gene Transfer of Aromatic L-Amino Acid Decarboxylase, Tyrosine Hydroxylase, and GTP Cyclohydrolase I Induces Sustained Transgene Expression, Dopamine Production, and Functional Improvement in a Rat Model of Parkinson's Disease. J. Neurosci. 22 (23), 10302–10312. doi:10.1523/jneurosci.22-23-10302.2002
Balwani, M., Sardh, E., Ventura, P., Peiró, P. A., Rees, D. C., Stölzel, U., et al. (2020). Phase 3 Trial of RNAi Therapeutic Givosiran for Acute Intermittent Porphyria. N. Engl. J. Med. 382 (24), 2289–2301. doi:10.1056/nejmoa1913147
Banskota, S., Raguram, A., Suh, S., Du, S. W., Davis, J. R., Choi, E. H., et al. (2022). Engineered Virus-like Particles for Efficient In Vivo Delivery of Therapeutic Proteins. Cell 185 (2), 250–265.e16. doi:10.1016/j.cell.2021.12.021
Bartelds, R., Nematollahi, M. H., Pols, T., Stuart, M. C. A., Pardakhty, A., Asadikaram, G., et al. (2018). Niosomes, an Alternative for Liposomal Delivery. PLoS One 13 (4), e0194179. doi:10.1371/journal.pone.0194179
Bartus, R. T., Baumann, T. L., Siffert, J., Herzog, C. D., Alterman, R., Boulis, N., et al. (2013). Safety/Feasibility of Targeting The Substantia Nigra With AAV2-Neurturin in Parkinson Patients. Neurology 80 (18), 1698–1701. doi:10.1212/WNL.0b013e3182904faa
Bemelmans, A. P., Horellou, P., Pradier, L., Brunet, I., Colin, P., Mallet, J., et al. (1999). Brain-Derived Neurotrophic Factor-Mediated Protection of Striatal Neurons in an Excitotoxic Rat Model of Huntington’s Disease, as Demonstrated by Adenoviral Gene Transfer. Hum. Gene Ther. 10 (18), 2987–2997. doi:10.1089/10430349950016393
Blömer, U., Naldini, L., Kafri, T., Trono, D., Verma, I. M., and Gage, F. H. (1997). Highly Efficient and Sustained Gene Transfer in Adult Neurons with a Lentivirus Vector. J. Virol. 71 (9), 6641–6649. doi:10.1128/jvi.71.9.6641-6649.1997
Boado, R. J., and Pardridge, W. M. (2011). The Trojan Horse Liposome Technology for Nonviral Gene Transfer across the Blood-Brain Barrier. J. Drug Deliv. 2011, 296151. doi:10.1155/2011/296151
Boussif, O., Lezoualc'h, F., Zanta, M. A., Mergny, M. D., Scherman, D., Demeneix, B., et al. (1995). A Versatile Vector for Gene and Oligonucleotide Transfer into Cells in Culture and In Vivo: Polyethylenimine. Proc. Natl. Acad. Sci. U.S.A. 92 (16), 7297–7301. doi:10.1073/pnas.92.16.7297
Calzoni, E., Cesaretti, A., Polchi, A., Di Michele, A., Tancini, B., and Emiliani, C. (2019). Biocompatible Polymer Nanoparticles for Drug Delivery Applications in Cancer and Neurodegenerative Disorder Therapies. J. Funct. Biomater. 10 (1). doi:10.3390/jfb10010004
Caracciolo, G. (2015). Liposome-protein Corona in a Physiological Environment: Challenges and Opportunities for Targeted Delivery of Nanomedicines. Nanomedicine Nanotechnol. Biol. Med. 11 (3), 543–557. doi:10.1016/j.nano.2014.11.003
Carvalho, L. S., Turunen, H. T., Wassmer, S. J., Luna-Velez, M. V., Xiao, R., Bennett, J., et al. (2017). Evaluating Efficiencies of Dual AAV Approaches for Retinal Targeting. Front. Neurosci. 11, 503. doi:10.3389/fnins.2017.00503
Chan, Y. K., Wang, S. K., Chu, C. J., Copland, D. A., Letizia, A. J., Costa Verdera, H., et al. (2021). Engineering Adeno-Associated Viral Vectors to Evade Innate Immune and Inflammatory Responses. Sci. Transl. Med. 13 (580). doi:10.1126/scitranslmed.abd3438
Chang, H., Lv, J., Gao, X., Wang, X., Wang, H., Chen, H., et al. (2017). Rational Design of a Polymer with Robust Efficacy for Intracellular Protein and Peptide Delivery. Nano Lett. 17 (3), 1678–1684. doi:10.1021/acs.nanolett.6b04955
Chiou, H. C., Tangco, M. V., Kormis, K., Levine, S. M., Robertson, D., Kormis, K., et al. (1994). Enhanced Resistance to Nuclease Degradation of Nucleic Acids Complexed to Asialoglycoprotein-Polylysine Carriers. Nucl. Acids Res. 22 (24), 5439–5446. doi:10.1093/nar/22.24.5439
Choi-Lundberg, D. L., Lin, Q., Schallert, T., Crippens, D., Davidson, B. L., Chang, Y. N., et al. (1998). Behavioral and Cellular Protection of Rat Dopaminergic Neurons by an Adenoviral Vector Encoding Glial Cell Line-Derived Neurotrophic Factor. Exp. Neurol. 154 (2), 261–275. doi:10.1006/exnr.1998.6887
Christine, C. W., Bankiewicz, K. S., Van Laar, A. D., Richardson, R. M., Ravina, B., Kells, A. P., et al. (2019). Magnetic Resonance Imaging-Guided Phase 1 Trial of Putaminal AADC Gene Therapy for Parkinson's Disease. Ann. Neurol. 85 (5), 704–714. doi:10.1002/ana.25450
Corbo, C., Molinaro, R., Parodi, A., Toledano Furman, N. E., Salvatore, F., and Tasciotti, E. (2016). The Impact of Nanoparticle Protein Corona on Cytotoxicity, Immunotoxicity and Target Drug Delivery. Nanomedicine 11 (1), 81–100. doi:10.2217/nnm.15.188
Davidson, B. L., and Boudreau, R. L. (2007). RNA Interference: a Tool for Querying Nervous System Function and an Emerging Therapy. Neuron 53 (6), 781–788. doi:10.1016/j.neuron.2007.02.020
Dos Santos Rodrigues, B., Kanekiyo, T., and Singh, J. (2019). ApoE-2 Brain-Targeted Gene Therapy through Transferrin and Penetratin Tagged Liposomal Nanoparticles. Pharm. Res. 36 (11), 161. doi:10.1007/s11095-019-2691-7
Dos Santos Rodrigues, B., Oue, H., Banerjee, A., Kanekiyo, T., and Singh, J. (2018). Dual Functionalized Liposome-Mediated Gene Delivery across Triple Co-culture Blood Brain Barrier Model and Specific In Vivo Neuronal Transfection. J. Control. Release 286, 264–278. doi:10.1016/j.jconrel.2018.07.043
Eid, A., Alshareef, S., and Mahfouz, M. M. (2018). CRISPR Base Editors: Genome Editing without Double-Stranded Breaks. Biochem. J. 475 (11), 1955–1964. doi:10.1042/bcj20170793
Ekman, F. K., Ojala, D. S., Adil, M. M., Lopez, P. A., Schaffer, D. V., and Gaj, T. (2019). CRISPR-Cas9-Mediated Genome Editing Increases Lifespan and Improves Motor Deficits in a Huntington's Disease Mouse Model. Mol. Ther. - Nucleic Acids 17, 829–839. doi:10.1016/j.omtn.2019.07.009
Ewert, K. K., Scodeller, P., Simón-Gracia, L., Steffes, V. M., Wonder, E. A., Teesalu, T., et al. (2021). Cationic Liposomes as Vectors for Nucleic Acid and Hydrophobic Drug Therapeutics. Pharmaceutics 13 (9). doi:10.3390/pharmaceutics13091365
Foust, K. D., Nurre, E., Montgomery, C. L., Hernandez, A., Chan, C. M., and Kaspar, B. K. (2009). Intravascular AAV9 Preferentially Targets Neonatal Neurons and Adult Astrocytes. Nat. Biotechnol. 27 (1), 59–65. doi:10.1038/nbt.1515
Frangoul, H., Altshuler, D., Cappellini, M. D., Chen, Y.-S., Domm, J., Eustace, B. K., et al. (2021). CRISPR-Cas9 Gene Editing for Sickle Cell Disease and β-Thalassemia. N. Engl. J. Med. 384 (3), 252–260. doi:10.1056/nejmoa2031054
Franklin, R. J. M., Quick, M. M., and Haase, G. (1999). Adenoviral Vectors for In Vivo Gene Delivery to Oligodendrocytes: Transgene Expression and Cytopathic Consequences. Gene Ther. 6 (8), 1360–1367. doi:10.1038/sj.gt.3300971
Gadalla, K. K. E., Vudhironarit, T., Hector, R. D., Sinnett, S., Bahey, N. G., Bailey, M. E. S., et al. (2017). Development of a Novel AAV Gene Therapy Cassette with Improved Safety Features and Efficacy in a Mouse Model of Rett Syndrome. Mol. Ther. Methods. Clin. Dev. 5, 180–190. doi:10.1016/j.omtm.2017.04.007
Gaj, T., Ojala, D. S., Ekman, F. K., Byrne, L. C., Limsirichai, P., and Schaffer, D. V. (2017). In Vivo genome Editing Improves Motor Function and Extends Survival in a Mouse Model of ALS. Sci. Adv. 3 (12), eaar3952. doi:10.1126/sciadv.aar3952
Gao, W., Hu, C. M., Fang, R. H., and Zhang, L. (2013). Liposome-like Nanostructures for Drug Delivery. J. Mater Chem. B 1 (48). doi:10.1039/C3TB21238F
Gaudelli, N. M., Komor, A. C., Rees, H. A., Packer, M. S., Badran, A. H., Bryson, D. I., et al. (2017). Programmable Base Editing of at to GC in Genomic DNA without DNA Cleavage. Nature 551 (7681), 464–471. doi:10.1038/nature24644
Ge, X., Wei, M., He, S., and Yuan, W. E. (2019). Advances of Non-ionic Surfactant Vesicles (Niosomes) and Their Application in Drug Delivery. Pharmaceutics 11 (2). doi:10.3390/pharmaceutics11020055
Giulimondi, F., Digiacomo, L., Pozzi, D., Palchetti, S., Vulpis, E., Capriotti, A. L., et al. (2019). Interplay of Protein Corona and Immune Cells Controls Blood Residency of Liposomes. Nat. Commun. 10 (1), 3686. doi:10.1038/s41467-019-11642-7
György, B., Lööv, C., Zaborowski, M. P., Takeda, S., Kleinstiver, B. P., Commins, C., et al. (2018). CRISPR/Cas9 Mediated Disruption of the Swedish APP Allele as a Therapeutic Approach for Early-Onset Alzheimer's Disease. Mol. Ther. Nucleic Acids 11, 429–440. doi:10.1016/j.omtn.2018.03.007
Haery, L., Deverman, B. E., Matho, K. S., Cetin, A., Woodard, K., Cepko, C., et al. (2019). Adeno-Associated Virus Technologies and Methods for Targeted Neuronal Manipulation. Front. Neuroanat. 13, 93. doi:10.3389/fnana.2019.00093
Han, S.-o., Mahato, R. I., Sung, Y. K., and Kim, S. W. (2000). Development of Biomaterials for Gene Therapy. Mol. Ther. 2 (4), 302–317. doi:10.1006/mthe.2000.0142
He, T.-C., Zhou, S., da Costa, L. T., Yu, J., Kinzler, K. W., and Vogelstein, B. (1998). A Simplified System for Generating Recombinant Adenoviruses. Proc. Natl. Acad. Sci. U.S.A. 95 (5), 2509–2514. doi:10.1073/pnas.95.5.2509
Horejs, C.-M. (2018). A Golden Ticket to the Brain. Nat. Rev. Mater 3 (8), 225. doi:10.1038/s41578-018-0035-6
Hu, C., and Rhodes, D. G. (2000). Proniosomes: a Novel Drug Carrier Preparation. Int. J. Pharm. 206 (1-2), 110–122. doi:10.1016/s0378-5173(00)00513-5
Hu, K., Chen, X., Chen, W., Zhang, L., Li, J., Ye, J., et al. (2018). Neuroprotective Effect of Gold Nanoparticles Composites in Parkinson's Disease Model. Nanomedicine 14 (4), 1123–1136. doi:10.1016/j.nano.2018.01.020
Huang, R., Han, L., Li, J., Ren, F., Ke, W., Jiang, C., et al. (2009). Neuroprotection in A 6-Hydroxydopamine-Lesioned Parkinson Model Using Lactoferrin-Modified Nanoparticles. J. Gene. Med. 11 (9), 754–763. doi:10.1002/jgm.1361
Huang, R., Ke, W., Liu, Y., Wu, D., Feng, L., Jiang, C., et al. (2010). Gene Therapy Using Lactoferrin-Modified Nanoparticles in A Rotenone-Induced Chronic Parkinson Model. J. Neurol. Sci. 290 (1-2), 123–130. doi:10.1016/j.jns.2009.09.032
Huang, Y., Chen, J., Chen, X., Gao, J., and Liang, W. (2008). PEGylated Synthetic Surfactant Vesicles (Niosomes): Novel Carriers for Oligonucleotides. J. Mater Sci. Mater Med. 19 (2), 607–614. doi:10.1007/s10856-007-3193-4
Hudry, E., and Vandenberghe, L. H. (2019). Therapeutic AAV Gene Transfer to the Nervous System: A Clinical Reality. Neuron 101 (5), 839–862. doi:10.1016/j.neuron.2019.02.017
Ingusci, S., Verlengia, G., Soukupova, M., Zucchini, S., and Simonato, M. (2019). Gene Therapy Tools for Brain Diseases. Front. Pharmacol. 10, 724. doi:10.3389/fphar.2019.00724
Iyama, T., and Wilson, D. M. (2013). DNA Repair Mechanisms in Dividing and Non-dividing Cells. DNA Repair 12 (8), 620–636. doi:10.1016/j.dnarep.2013.04.015
Jagannath, A., and Wood, M. (2007). RNA Interference Based Gene Therapy for Neurological Disease. Briefings Funct. Genomics Proteomics 6 (1), 40–49. doi:10.1093/bfgp/elm005
Jayant, R. D., Sosa, D., Kaushik, A., Atluri, V., Vashist, A., Tomitaka, A., et al. (2016). Current Status of Non-viral Gene Therapy for CNS Disorders. Expert Opin. Drug Deliv. 13 (10), 1433–1445. doi:10.1080/17425247.2016.1188802
Jubair, L., Lam, A. K., Fallaha, S., and McMillan, N. A. J. (2021). CRISPR/Cas9-loaded Stealth Liposomes Effectively Cleared Established HPV16-Driven Tumours in Syngeneic Mice. PLoS One 16 (1), e0223288. doi:10.1371/journal.pone.0223288
Juliano, R. L. (2016). The Delivery of Therapeutic Oligonucleotides. Nucleic Acids Res. 44 (14), 6518–6548. doi:10.1093/nar/gkw236
Kafri, T., Morgan, D., Krahl, T., Sarvetnick, N., Sherman, L., and Verma, I. (1998). Cellular Immune Response to Adenoviral Vector Infected Cells Does Not Require De Novo Viral Gene Expression: Implications for Gene Therapy. Proc. Natl. Acad. Sci. U.S.A. 95 (19), 11377–11382. doi:10.1073/pnas.95.19.11377
Kalidasan, V., Ng, W. H., Ishola, O. A., Ravichantar, N., Tan, J. J., and Das, K. T. (2021). A Guide in Lentiviral Vector Production for Hard-To-Transfect Cells, Using Cardiac-Derived C-Kit Expressing Cells as a Model System. Sci. Rep. 11 (1), 19265. doi:10.1038/s41598-021-98657-7
Kantor, A., McClements, M. E., and MacLaren, R. E. (2020). CRISPR-Cas9 DNA Base-Editing and Prime-Editing. Int. J. Mol. Sci. 21 (17). doi:10.3390/ijms21176240
Kaplitt, M. G., Feigin, A., Tang, C., Fitzsimons, H. L., Mattis, P., Lawlor, P. A., et al. (2007). Safety and Tolerability of Gene Therapy with an Adeno-Associated Virus (AAV) Borne GAD Gene for Parkinson's Disease: an Open Label, Phase I Trial. Lancet 369 (9579), 2097–2105. doi:10.1016/s0140-6736(07)60982-9
Katsouri, L., Lim, Y. M., Blondrath, K., Eleftheriadou, I., Lombardero, L., Birch, A. M., et al. (2016). Ppargamma-Coactivator-1alpha Gene Transfer Reduces Neuronal Loss and Amyloid-Beta Generation by Reducing Beta-Secretase in an Alzheimer's Disease Model. Proc. Natl. Acad. Sci. U S A. 113 (43), 12292–12297.
Komor, A. C., Kim, Y. B., Packer, M. S., Zuris, J. A., and Liu, D. R. (2016). Programmable Editing of a Target Base in Genomic DNA without Double-Stranded DNA Cleavage. Nature 533 (7603), 420–424. doi:10.1038/nature17946
Kosicki, M., Tomberg, K., and Bradley, A. (2018). Repair of Double-Strand Breaks Induced by CRISPR-Cas9 Leads to Large Deletions and Complex Rearrangements. Nat. Biotechnol. 36 (8), 765–771. doi:10.1038/nbt.4192
Kreppel, F., and Kochanek, S. (2004). Long-term Transgene Expression in Proliferating Cells Mediated by Episomally Maintained High-Capacity Adenovirus Vectors. J. Virol. 78 (1), 9–22. doi:10.1128/jvi.78.1.9-22.2004
Kreuter, J. (2014). Drug Delivery to the Central Nervous System by Polymeric Nanoparticles: what Do We Know? Adv. Drug Deliv. Rev. 71, 2–14. doi:10.1016/j.addr.2013.08.008
Lapchak, P. A., Araujo, D. M., Hilt, D. C., Sheng, J., and Jiao, S. (1997). Adenoviral Vector-Mediated GDNF Gene Therapy in a Rodent Lesion Model of Late Stage Parkinson's Disease. Brain Res. 777 (1-2), 153–160. doi:10.1016/s0006-8993(97)01100-1
Leblois, H., Roche, C., Di Falco, N., Orsini, C., Yeh, P., and Perricaudet, M. (2000). Stable Transduction of Actively Dividing Cells via a Novel Adenoviral/episomal Vector. Mol. Ther. 1 (4), 314–322. doi:10.1006/mthe.2000.0042
Lee, B., Lee, K., Panda, S., Gonzales-Rojas, R., Chong, A., Bugay, V., et al. (2018). Nanoparticle Delivery of CRISPR into the Brain Rescues a Mouse Model of Fragile X Syndrome from Exaggerated Repetitive Behaviours. Nat. Biomed. Eng. 2 (7), 497–507. doi:10.1038/s41551-018-0252-8
Levy, J. M., Yeh, W. H., Pendse, N., Davis, J. R., Hennessey, E., Butcher, R., et al. (2020). Cytosine and Adenine Base Editing of the Brain, Liver, Retina, Heart and Skeletal Muscle of Mice Via Adeno-Associated Viruses. Nat. Biomed. Eng. 4 (1), 97–110. doi:10.1038/s41551-019-0501-5
Li, C., and Samulski, R. J. (2020). Engineering Adeno-Associated Virus Vectors for Gene Therapy. Nat. Rev. Genet. 21 (4), 255–272. doi:10.1038/s41576-019-0205-4
Li, H., Yang, Y., Hong, W., Huang, M., Wu, M., and Zhao, X. (2020). Applications of Genome Editing Technology in the Targeted Therapy of Human Diseases: Mechanisms, Advances and Prospects. Sig Transduct. Target Ther. 5 (1), 1. doi:10.1038/s41392-019-0089-y
Lim, C. K. W., Gapinske, M., Brooks, A. K., Woods, W. S., Powell, J. E., Zeballos, C. M. A., et al. (2020). Treatment of a Mouse Model of ALS by In Vivo Base Editing. Mol. Ther. 28 (4), 1177–1189. doi:10.1016/j.ymthe.2020.01.005
Liu, L., Li, M., Xu, M., Wang, Z., Zeng, Z., Li, Y., et al. (2020). Actively Targeted Gold Nanoparticle Composites Improve Behavior and Cognitive Impairment in Parkinson's Disease Mice. Mater. Sci. Eng. C. Mater. Biol. Appl. 114, 111028doi:10.1016/j.msec.2020.111028
Lombardo, S. M., Schneider, M., Türeli, A. E., and Günday Türeli, N. (2020). Key for Crossing the BBB with Nanoparticles: the Rational Design. Beilstein J. Nanotechnol. 11, 866–883. doi:10.3762/bjnano.11.72
Mandel, R. J. (2010). CERE-110, an Adeno-Associated Virus-Based Gene Delivery Vector Expressing Human Nerve Growth Factor For The Treatment of Alzheimer's Disease. Curr. Opin. Mol. Ther. 12 (2), 240–247.
Mendell, J. R., Al-Zaidy, S., Shell, R., Arnold, W. D., Rodino-Klapac, L. R., Prior, T. W., et al. (2017). Single-Dose Gene-Replacement Therapy for Spinal Muscular Atrophy. N. Engl. J. Med. 377 (18), 1713–1722. doi:10.1056/nejmoa1706198
Mignani, S., Shi, X., Karpus, A., and Majoral, J.-P. (2021). Non-invasive Intranasal Administration Route Directly to the Brain Using Dendrimer Nanoplatforms: An Opportunity to Develop New CNS Drugs. Eur. J. Med. Chem. 209, 112905. doi:10.1016/j.ejmech.2020.112905
Mintzer, M. A., and Simanek, E. E. (2009). Nonviral Vectors for Gene Delivery. Chem. Rev. 109 (2), 259–302. doi:10.1021/cr800409e
Mittermeyer, G., Christine, C. W., Rosenbluth, K. H., Baker, S. L., Starr, P., Larson, P., et al. (2012). Long-term Evaluation of a Phase 1 Study of AADC Gene Therapy for Parkinson's Disease. Hum. Gene Ther. 23 (4), 377–381. doi:10.1089/hum.2011.220
Monnery, B. D., Wright, M., Cavill, R., Hoogenboom, R., Shaunak, S., Steinke, J. H. G., et al. (2017). Cytotoxicity of Polycations: Relationship of Molecular Weight and the Hydrolytic Theory of the Mechanism of Toxicity. Int. J. Pharm. 521 (1-2), 249–258. doi:10.1016/j.ijpharm.2017.02.048
Moore, J. K., and Haber, J. E. (1996). Cell Cycle and Genetic Requirements of Two Pathways of Nonhomologous End-Joining Repair of Double-Strand Breaks in Saccharomyces cerevisiae. Mol. Cell Biol. 16 (5), 2164–2173. doi:10.1128/mcb.16.5.2164
Morille, M., Passirani, C., Vonarbourg, A., Clavreul, A., and Benoit, J. P. (2008). Progress in Developing Cationic Vectors for Non-viral Systemic Gene Therapy against Cancer. Biomaterials 29 (24-25), 3477–3496. doi:10.1016/j.biomaterials.2008.04.036
Morrell, N. T., Leucht, P., Zhao, L., Kim, J.-B., ten Berge, D., Ponnusamy, K., et al. (2008). Liposomal Packaging Generates Wnt Protein with In Vivo Biological Activity. PLoS One 3 (8), e2930. doi:10.1371/journal.pone.0002930
Mousazadeh, M., Palizban, A., Salehi, R., and Salehi, M. (2007). Gene Delivery to Brain Cells with Apoprotein E Derived Peptide Conjugated to Polylysine (apoEdp-PLL). J. Drug Target. 15 (3), 226–230. doi:10.1080/10611860601148908
Mueller, C., Berry, J. D., McKenna-Yasek, D. M., Gernoux, G., Owegi, M. A., Pothier, L. M., et al. (2020). SOD1 Suppression With Adeno-Associated Virus and Microrna in Familial ALS. N. Engl. J. Med. 383 (2), 151–158. doi:10.1056/NEJMoa2005056
Naldini, L., Blömer, U., Gage, F. H., Trono, D., and Verma, I. M. (1996). Efficient Transfer, Integration, and Sustained Long-Term Expression of the Transgene in Adult Rat Brains Injected with a Lentiviral Vector. Proc. Natl. Acad. Sci. U.S.A. 93 (21), 11382–11388. doi:10.1073/pnas.93.21.11382
Niu, S., Zhang, L. K., Zhang, L., Zhuang, S., Zhan, X., Chen, W. Y., et al. (2017). Inhibition by Multifunctional Magnetic Nanoparticles Loaded with Alpha-Synuclein RNAi Plasmid in a Parkinson's Disease Model. Theranostics 7 (2), 344–356. doi:10.7150/thno.16562
O'Keeffe Ahern, J., Lara-Sáez, I., Zhou, D., Murillas, R., Bonafont, J., Mencía, Á., et al. (2022). Non-viral Delivery of CRISPR-Cas9 Complexes for Targeted Gene Editing via a Polymer Delivery System. Gene Ther. 29 (3-4), 157–170. doi:10.1038/s41434-021-00282-6
Palfi, S., Gurruchaga, J. M., Lepetit, H., Howard, K., Ralph, G. S., Mason, S., et al. (2018). Long-Term Follow-Up of a Phase I/II Study of ProSavin, a Lentiviral Vector Gene Therapy for Parkinson's Disease. Hum. Gene Ther. Clin. Dev. 29 (3), 148–155. doi:10.1089/humc.2018.081
Palfi, S., Gurruchaga, J. M., Ralph, G. S., Lepetit, H., Lavisse, S., Buttery, P. C., et al. (2014). Long-term Safety and Tolerability of ProSavin, a Lentiviral Vector-Based Gene Therapy for Parkinson's Disease: a Dose Escalation, Open-Label, Phase 1/2 Trial. Lancet 383 (9923), 1138–1146. doi:10.1016/s0140-6736(13)61939-x
Pardridge, W. M. (2005). Tyrosine Hydroxylase Replacement in Experimental Parkinson's Disease With Transvascular Gene Therapy. NeuroRx. 2 (1), 129–138. doi:10.1602/neurorx.2.1.129
Park, H., Oh, J., Shim, G., Cho, B., Chang, Y., Kim, S., et al. (2019). In Vivo neuronal Gene Editing via CRISPR-Cas9 Amphiphilic Nanocomplexes Alleviates Deficits in Mouse Models of Alzheimer's Disease. Nat. Neurosci. 22 (4), 524–528. doi:10.1038/s41593-019-0352-0
Park, T. E., Singh, B., Li, H., Lee, J. Y., Kang, S. K., Choi, Y. J., et al. (2015). Enhanced BBB Permeability of Osmotically Active Poly(Mannitol-Co-PEI) Modified With Rabies Virus Glycoprotein Via Selective Stimulation of Caveolar Endocytosis For Rnai Therapeutics in Alzheimer's Disease. Biomaterials. 38, 61–71. doi:10.1016/j.biomaterials.2014.10.068
Philippe, S., Sarkis, C., Barkats, M., Mammeri, H., Ladroue, C., Petit, C., et al. (2006). Lentiviral Vectors with a Defective Integrase Allow Efficient and Sustained Transgene Expression In Vitro and In Vivo. Proc. Natl. Acad. Sci. U.S.A. 103 (47), 17684–17689. doi:10.1073/pnas.0606197103
Qi, T., Wu, F., Xie, Y., Gao, S., Li, M., Pu, J., et al. (2020). Base Editing Mediated Generation of Point Mutations into Human Pluripotent Stem Cells for Modeling Disease. Front. Cell Dev. Biol. 8, 590581. doi:10.3389/fcell.2020.590581
Qin, L., Li, J., Wang, Q., Xu, Z., Sun, L., Alariqi, M., et al. (2020). High‐efficient and Precise Base Editing of CG to TA in the Allotetraploid Cotton (Gossypium Hirsutum ) Genome Using a Modified CRISPR/Cas9 System. Plant Biotechnol. J. 18 (1), 45–56. doi:10.1111/pbi.13168
Ramamoorth, M., and Narvekar, A. (2015). Non Viral Vectors in Gene Therapy- an Overview. J. Clin. Diagn. Res. 9 (1), GE01–6. doi:10.7860/JCDR/2015/10443.5394
Ren, T., Xu, N., Cao, C., Yuan, W., Yu, X., Chen, J., et al. (2009). Preparation and Therapeutic Efficacy of Polysorbate-80-Coated Amphotericin B/PLA-b-PEG Nanoparticles. J. Biomaterials Sci. Polym. Ed. 20 (10), 1369–1380. doi:10.1163/092050609x12457418779185
Ricci, R., and Colasante, G. (2021). CRISPR/dCas9 as a Therapeutic Approach for Neurodevelopmental Disorders: Innovations and Limitations Compared to Traditional Strategies. Dev. Neurosci. 43 (3-4), 253–261. doi:10.1159/000515845
Roberts, T. C., Langer, R., and Wood, M. J. A. (2020). Advances in Oligonucleotide Drug Delivery. Nat. Rev. Drug Discov. 19 (10), 673–694. doi:10.1038/s41573-020-0075-7
Sakuma, T., Barry, M. A., and Ikeda, Y. (2012). Lentiviral Vectors: Basic to Translational. Biochem. J. 443 (3), 603–618. doi:10.1042/bj20120146
Salameh, J. W., Zhou, L., Ward, S. M., Santa Chalarca, C. F., Emrick, T., and Figueiredo, M. L. (2020). Polymer-mediated Gene Therapy: Recent Advances and Merging of Delivery Techniques. Wiley Interdiscip. Rev. Nanomed. Nanobiotechnol. 12 (2), e1598. doi:10.1002/wnan.1598
Scheller, E. L., and Krebsbach, P. H. (2009). Gene Therapy: Design and Prospects for Craniofacial Regeneration. J. Dent. Res. 88 (7), 585–596. doi:10.1177/0022034509337480
Sheikh, M. A., Malik, Y. S., Xing, Z., Guo, Z., Tian, H., Zhu, X., et al. (2017). Polylysine-modified Polyethylenimine (PEI-PLL) Mediated VEGF Gene Delivery Protects Dopaminergic Neurons in Cell Culture and in Rat Models of Parkinson's Disease (PD). Acta Biomater. 54, 58–68. doi:10.1016/j.actbio.2016.12.048
Shilo, M., Motiei, M., Hana, P., and Popovtzer, R. (2014). Transport of Nanoparticles through the Blood-Brain Barrier for Imaging and Therapeutic Applications. Nanoscale 6 (4), 2146–2152. doi:10.1039/c3nr04878k
Shu, Y., Tao, Y., Li, W., Shen, J., Wang, Z., and Chen, Z. Y. (2016). Adenovirus Vectors Target Several Cell Subtypes of Mammalian Inner Ear In Vivo. Neural Plast. 2016, 9409846. doi:10.1155/2016/9409846
Song, B., Yang, S., Hwang, G-H., Yu, J., and Bae, S. (2021). Analysis of NHEJ-Based DNA Repair after CRISPR-Mediated DNA Cleavage. Int. J. Mol. Sci. 22 (12). doi:10.3390/ijms22126397
Spronck, E. A., Vallès, A., Lampen, M. H., Montenegro-Miranda, P. S., Keskin, S., Heijink, L., et al. (2021). Intrastriatal Administration of AAV5-miHTT in Non-Human Primates and Rats Is Well Tolerated and Results in miHTT Transgene Expression in Key Areas of Huntington Disease Pathology. Brain Sci. 11 (2), 129. doi:10.3390/brainsci11020129
Srivastava, A. (2016). In Vivo tissue-tropism of Adeno-Associated Viral Vectors. Curr. Opin. Virology 21, 75–80. doi:10.1016/j.coviro.2016.08.003
Su, X., Kells, A. P., Huang, E. J., Lee, H. S., Hadaczek, P., Beyer, J., et al. (2009). Safety Evaluation of AAV2-GDNF Gene Transfer Into The Dopaminergic Nigrostriatal Pathway in Aged and Parkinsonian Rhesus Monkeys. Hum. Gene Ther. 20 (12), 1627–1640. doi:10.1089/hum.2009.103
Suk, J. S., Xu, Q., Kim, N., Hanes, J., and Ensign, L. M. (2016). PEGylation as a Strategy for Improving Nanoparticle-Based Drug and Gene Delivery. Adv. Drug Deliv. Rev. 99 (Pt A), 28–51. doi:10.1016/j.addr.2015.09.012
Tang, Y., Han, T., Everts, M., Zhu, Z. B., Gillespie, G. Y., Curiel, D. T., et al. (2007). Directing Adenovirus across the Blood-Brain Barrier via Melanotransferrin (P97) Transcytosis Pathway in an In Vitro Model. Gene Ther. 14 (6), 523–532. doi:10.1038/sj.gt.3302888
Tenenbaum, L., Chtarto, A., Lehtonen, E., Velu, T., Brotchi, J., and Levivier, M. (2004). Recombinant AAV-Mediated Gene Delivery to the Central Nervous System. J. Gene Med. 6 (Suppl. 1), S212–S222. doi:10.1002/jgm.506
Trapani, I. (2019). Adeno-Associated Viral Vectors as a Tool for Large Gene Delivery to the Retina. Genes (Basel) 10 (4). doi:10.3390/genes10040287
Trenkmann, M. (2018). Gold Rush to Gene-Editing in the Brain. Nat. Rev. Genet. 19 (9), 532–533. doi:10.1038/s41576-018-0038-6
Urquhart, L. (2019). FDA New Drug Approvals in Q2 2019. Nat. Rev. Drug Discov. 18 (8), 575. doi:10.1038/d41573-019-00121-9
Wagner, S., Zensi, A., Wien, S. L., Tschickardt, S. E., Maier, W., Vogel, T., et al. (2012). Uptake Mechanism of ApoE-Modified Nanoparticles on Brain Capillary Endothelial Cells as a Blood-Brain Barrier Model. PLoS One 7 (3), e32568. doi:10.1371/journal.pone.0032568
Wang, H., Shi, H.-B., and Yin, S.-K. (2011). Polyamidoamine Dendrimers as Gene Delivery Carriers in the Inner Ear: How to Improve Transfection Efficiency. Exp. Ther. Med. 2 (5), 777–781. doi:10.3892/etm.2011.296
Win-Shwe, T.-T., Sone, H., Kurokawa, Y., Zeng, Y., Zeng, Q., Nitta, H., et al. (2014). Effects of PAMAM Dendrimers in the Mouse Brain after a Single Intranasal Instillation. Toxicol. Lett. 228 (3), 207–215. doi:10.1016/j.toxlet.2014.04.020
Wu, P., Chen, H., Jin, R., Weng, T., Ho, J. K., You, C., et al. (2018). Non-viral Gene Delivery Systems for Tissue Repair and Regeneration. J. Transl. Med. 16 (1), 29. doi:10.1186/s12967-018-1402-1
Yang, L., Yang, H., Rideout, K., Cho, T., Joo, K. i., Ziegler, L., et al. (2008). Engineered Lentivector Targeting of Dendritic Cells for In Vivo Immunization. Nat. Biotechnol. 26 (3), 326–334. doi:10.1038/nbt1390
Yang, S., Chang, R., Yang, H., Zhao, T., Hong, Y., Kong, H. E., et al. (2017). CRISPR/Cas9-mediated Gene Editing Ameliorates Neurotoxicity in Mouse Model of Huntington's Disease. J. Clin. Invest. 127 (7), 2719–2724. doi:10.1172/jci92087
Yu, Y., Leete, T. C., Born, D. A., Young, L., Barrera, L. A., Lee, S.-J., et al. (2020). Cytosine Base Editors with Minimized Unguided DNA and RNA Off-Target Events and High On-Target Activity. Nat. Commun. 11 (1), 2052. doi:10.1038/s41467-020-15887-5
Yue, P., Miao, W., Gao, L., Zhao, X., and Teng, J. (2018). Ultrasound-Triggered Effects of the Microbubbles Coupled to GDNF Plasmid-Loaded PEGylated Liposomes in a Rat Model of Parkinson's Disease. Front. Neurosci. 12, 222. doi:10.3389/fnins.2018.00222
Zhang, H., Yang, B., Mu, X., Ahmed, S. S., Su, Q., He, R., et al. (2011). Several rAAV Vectors Efficiently Cross the Blood-Brain Barrier and Transduce Neurons and Astrocytes in the Neonatal Mouse Central Nervous System. Mol. Ther. 19 (8), 1440–1448. doi:10.1038/mt.2011.98
Zhang, S., Shen, J., Li, D., and Cheng, Y. (2021). Strategies in the Delivery of Cas9 Ribonucleoprotein for CRISPR/Cas9 Genome Editing. Theranostics 11 (2), 614–648. doi:10.7150/thno.47007
Zhang, Y., Calon, F., Zhu, C., Boado, R. J., and Pardridge, W. M. (2003). Intravenous Nonviral Gene Therapy Causes Normalization of Striatal Tyrosine Hydroxylase and Reversal of Motor Impairment in Experimental Parkinsonism. Hum. Gene Ther. 14 (1), 1–12. doi:10.1089/10430340360464660
Zhang, Y., Schlachetzki, F., Zhang, Y.-F., Boado, R. J., and Pardridge, W. M. (2004). Normalization of Striatal Tyrosine Hydroxylase and Reversal of Motor Impairment in Experimental Parkinsonism with Intravenous Nonviral Gene Therapy and a Brain-specific Promoter. Hum. Gene Ther. 15 (4), 339–350. doi:10.1089/104303404322959498
Zheng, M., Pan, M., Zhang, W., Lin, H., Wu, S., Lu, C., et al. (2021). Poly(α-l-lysine)-based Nanomaterials for Versatile Biomedical Applications: Current Advances and Perspectives. Bioact. Mater. 6 (7), 1878–1909. doi:10.1016/j.bioactmat.2020.12.001
Keywords: CRISPR/Cas9, gene therapy, in vivo gene editing, neurodevelopmental disorders, neurodegenerative diseases, therapeutics, viral vector, non-viral vector
Citation: Paul A, Collins MG and Lee HY (2022) Gene Therapy: The Next-Generation Therapeutics and Their Delivery Approaches for Neurological Disorders. Front. Genome Ed. 4:899209. doi: 10.3389/fgeed.2022.899209
Received: 18 March 2022; Accepted: 05 May 2022;
Published: 22 June 2022.
Edited by:
Kyle David Fink, University of California, Davis, United StatesReviewed by:
Marta Olejniczak, Institute of Bioorganic Chemistry (PAS), PolandCopyright © 2022 Paul, Collins and Lee. This is an open-access article distributed under the terms of the Creative Commons Attribution License (CC BY). The use, distribution or reproduction in other forums is permitted, provided the original author(s) and the copyright owner(s) are credited and that the original publication in this journal is cited, in accordance with accepted academic practice. No use, distribution or reproduction is permitted which does not comply with these terms.
*Correspondence: Hye Young Lee, bGVlaDZAdXRoc2NzYS5lZHU=