- 1Estonian Marine Institute, University of Tartu, Tallinn, Estonia
- 2Coastal People Southern Skies, Centre of Research Excellence, Dunedin, New Zealand
- 3Department of Marine Science, University of Otago, Dunedin, New Zealand
The carbon acquisition strategies of aquatic photosynthetic organisms play a key role in the growth and survival of a species. There is much research indicating that the predicted changes (e.g., the balance between carbonate species: CO2, , and ) in the seawater carbonate chemistry, due to ocean acidification, could affect benthic primary producers and their communities. However, considerably less is known about brackish water (e.g., the Baltic Sea), and even less about the possible effects of acidification on freshwater biota. This study aimed to compare the carbon uptake strategies of two dominant charophyte species: Chara aspera and Chara tomentosa growing in freshwater lakes of Estonia and in the brackish NE Baltic Sea. This could indicate how they might respond to the predicted increasing CO2 concentration linked to climate change scenrios. Carbon use strategies in charophytes were determined by analysing natural carbon isotope signatures (δ13C), pH drift experiments and photosynthesis vs. dissolved inorganic carbon curves. The study showed that freshwater and brackish water C. aspera and C. tomentosa likely use different carbon uptake mechanisms. Our results indicated that freshwater charophytes preferentially use CO2 and brackish water charophytes , likely due to their local acclimatization to different growth environments. Also, C. tomentosa and C. aspera from the studied lakes are likely carbon saturated (photosynthetic processes are operating at their maximum efficiency due to the availability of dissolved inorganic carbon in their environment) and probably will not gain photosynthetic advantages from acidification. However, the predicted increase in CO2 concentration may positively affect the growth of the charophytes in the brackish Baltic Sea.
1 Introduction
Ocean acidification (OA) is a well-known phenomenon, a direct consequence of increasing carbon dioxide (CO2) concentration in the atmosphere. The understanding of the effects of OA on ocean biota at organism, community, and ecosystem levels has increased in the last decade (Gattuso and Hansson, 2011; Doney et al., 2020; Terhaar et al., 2020). However, considerably less is known about brackish water (e.g., the Baltic Sea), and even less about the possible effects of acidification on freshwater biota. The future OA scenarios for the Baltic Sea could be more severe than predicted for oceans because of the low buffering capacity of brackish water (Omstedt et al., 2012; Melzner et al., 2013), the high freshwater input with high levels of dissolved organic carbon (DOC) and low alkalinity (Melzner et al., 2013). Also, the extent of acidification could markedly vary between different areas of the Baltic Sea due to geological, biogeochemical, and riverine input differences. It is estimated that the Baltic Sea surface water pH levels could decline by 0.25–0.5 units by the end of the century (Omstedt et al., 2010, 2012; Kuznetsov and Neumann, 2013).
Lakes could act as a source or sink of CO2 depending on the chemical, physical, and biological processes (McKinley et al., 2011; Shao et al., 2015). Many small lakes are known to be net CO2 emitters (Hanson et al., 2004; Alin and Johnson, 2007; Cole et al., 2007). However, it does not mean that acidification due to increasing CO2 could not affect these lakes (Phillips et al., 2015). Rising CO2 levels can lower the pH of lake water, potentially disrupting aquatic ecosystems, affecting the solubility of nutrients and metals, and harming organisms sensitive to changes in acidity. In general, it is difficult to assess the degree to which inorganic carbon dynamics affect pCO2 (partial pressure of carbon dioxide) in the lakes because there is usually a lack of data about the carbon cycles and lake-atmosphere CO2 fluxes (Eadie and Robertson, 1976; Atilla et al., 2011; Bennington et al., 2012; Vesala et al., 2012). However, it is generally known that diel cycles of productivity, driven by photosynthesis during the day and respiration at night, can lead to significant fluctuations in pH and CO2 in different aquatic systems, e.g., in lake and marine environments. These cycles cause pH to rise during the day as CO2 is consumed and decrease at night as CO2 is produced (Andersen et al., 2017). Also, the impact of catchments e.g., the delivery of dissolved inorganic carbon (DIC) and dissolved organic carbon (DOC) play a big role in especially small lakes alkalinity and carbon budgets (McConnaughey et al., 1994; Einsele et al., 2001; Stets et al., 2009).
Estonian lakes, due to their unique geological and hydrological characteristics, exhibit distinct patterns of carbon dynamics (Ott and Kõiv, 1999; Simm et al., 2006; Nõges et al., 2010). Could be expected that the presence of limestone and other carbonate-rich substrates can contribute to higher alkalinity levels, enhancing the lakes' buffering capacity and pH stability. The relatively shallow nature of many Estonian lakes (Simm et al., 2006; Nõges et al., 2010) can lead to more pronounced diel variations in pH and CO2 levels, as sunlight impacts the entire water column directly. However, each Estonian lake has unique properties, making it difficult to generalize or compare carbon cycling processes even within the region, complicating the comparison with lakes in other regions, this emphasizes the need for site-specific studies to understand carbon dynamics accurately.
This study focuses on charophytes, which are a globally widespread group of submerged algae with well-developed complex thalli and morphology. They grow in freshwater lakes, ponds, streams, brackish environments, and even in hypersaline water bodies to a limited extent (Krause, 1997). Charophyte communities are important habitats for various invertebrate species, and they provide feeding and nursery areas for many fish species (Blindow and van de Weyer, 2016). In the Baltic Sea, charophytes inhabit sheltered soft-bottom coastal areas where their distribution pattern is primarily controlled by exposure, sediment type, and salinity regime (Schubert and Yousef, 2001; Torn et al., 2004). While some euryhaline charophyte species are found all over the Baltic Sea, the majority of the species are growing below salinity 7 PSU (Practical Salinity Unit; Schubert and Blindow, 2003).
The genus Chara grows predominantly in calcium-rich waters and only with a few exceptions in oligotrophic soft-waters conditions (Torn et al., 2015; Blindow and van de Weyer, 2016). The Chara species are capable of forming very dense canopies in oligotrophic, calcareous lakes and ponds as well as in sheltered bays in the Baltic Sea (Schubert and Blindow, 2003). Charophytes have been reported to be able to utilize bicarbonate as a carbon source (McConnaughey et al., 1994; Herbst and Schubert, 2018) and have a higher affinity to than vascular macrophytes (Van den Berg et al., 1998). Internodal cells of Characean are able to generate spatially separated bands of high and low pH in the medium adjacent to their cell surface (Spear et al., 1969; Lucas and Smith, 1973). When exposed to light, the internodes show alkaline regions with pH values between 8.5 and 9.5 and acid regions with a pH of about 5.5. The bands disappear when photosynthesis is inhibited and the pH at the cell surface becomes uniform with values of about pH 6.0 (Lucas and Smith, 1973). During the pH banding, Characean cell walls calcify in the alkaline regions where the pH increases and consequently shift the chemical equilibrium toward the formation of carbonate ions (Stumm and Morgan, 1970; McConnaughey and Falk, 1991), which precipitate with calcium in the form of calcite. This process itself significantly depletes bicarbonate ions, which has been observed within a dense Chara cover (Van den Berg et al., 1998). The extent of calcification, however, depends on pH and the concentrations of bicarbonate and calcium (McConnaughey and Whelan, 1997). Also, on individual characteristics of the charophyte species (Andersen et al., 2017).
The study focuses on two charophyte species: Chara aspera Willd. and Chara tomentosa L. growing in freshwater lakes and in the brackish Baltic Sea. C. aspera has slender, small to medium size (5–20 cm) thalli. The species has a very broad tolerance to environmental conditions and therefore, it is the most widespread charophyte species in the coastal seas and freshwater lakes of Estonia. C. tomentosa is fairly large (25–60 cm) in size compared to C. aspera and is also a widely distributed species in Estonia, mostly occurring sparsely or forming patches within communities dominated by other charophytes (Torn et al., 2015).
The aim was to compare the carbon uptake strategies of the two species, which could indicate how they might respond to the predicted increasing CO2 concentration associated with climate change. The mechanisms of DIC uptake and carbon requirements for macroalgal growth are species-specific (Hepburn et al., 2011; Albert et al., 2020; Pajusalu et al., 2020). It is thought that the species that lack a carbon concentrating mechanism (CCM)—relying solely on CO2, CCMs with low affinities for DIC, and CCMs that can be downregulated, could benefit from increasing CO2 concentration (Giordano et al., 2005). Species with efficient CCMs that are not capable of taking up additional CO2 or species with CCMs which could not be downregulated are thought to be unaffected by OA or the benefit could be considerably lower (Beardall and Raven, 2004; Cornwall et al., 2017). In this study the carbon use strategies in charophytes were determined by analysing natural carbon isotope signatures (δ13C), pH drift experiments and photosynthesis vs. dissolved inorganic carbon (P vs. DIC) curves. We hypothesized that there is a significant difference between the freshwater and brackish water C. aspera and C. tomentosa carbon uptake strategies. Also, based on our earlier work (Pajusalu et al., 2015) the brackish Baltic Sea charophytes are carbon limited during the active vegetation period under the current DIC availability and therefore benefit from increasing CO2 concentration.
2 Materials and methods
Charophytes from the brackish Baltic Sea were collected in July 2018 by Scuba diving from the Väike Strait Northern Gulf of Riga (58.5155°N, 23.2035°E), at a depth of 2 m. At this depth, C. aspera is the dominant species and C. tomentosa grows in patches. The strait is part of the Väinameri Sea and is characterized by lots of islets and shoals. The strait is 2–4 km wide and generally < 3 m deep. Salinity in Väike Strait ranges from 3.7 to 6.3 PSU, during the sampling period, the salinity was 6 PSU. In Estonia, charophytes from freshwater (salinity below 0.2 PSU) were collected with a hook from two lakes 16 km apart. C. aspera was collected in July 2018 from Seljajärv (58.9199°N, 26.2753°E), which is originally an old sand quarry, however, not been used for several decades and now characterized by limestone bedrock and dense charophyte mat, where the species C. aspera forms a monodominant community in shallower depths (0.3–0.5 m). C. tomentosa was collected in July 2018 from Äntu Sinijärv (59.0621°N, 26.2404°E) at a depth of 0.4 m where the species grows sparsely in a dense community formed by Chara papillosa Kütz (Figure 1). Äntu Sinijärv is a calcareous lake with a high visibility down to ~15 m, pH of 7.4–8.0 and an content of 262–274 mgL−1 (Laumets et al., 2014). The lake is fed by direct precipitation and by groundwater from a limestone aquifer that discharges into the lake through at least seven submerged spring vents (Saarse and Liiva, 1995). Throughout the paper, C. tomentosa collected from Äntu Sinijärv and C. aspera collected from Seljajärv are referred to as freshwater charophytes.
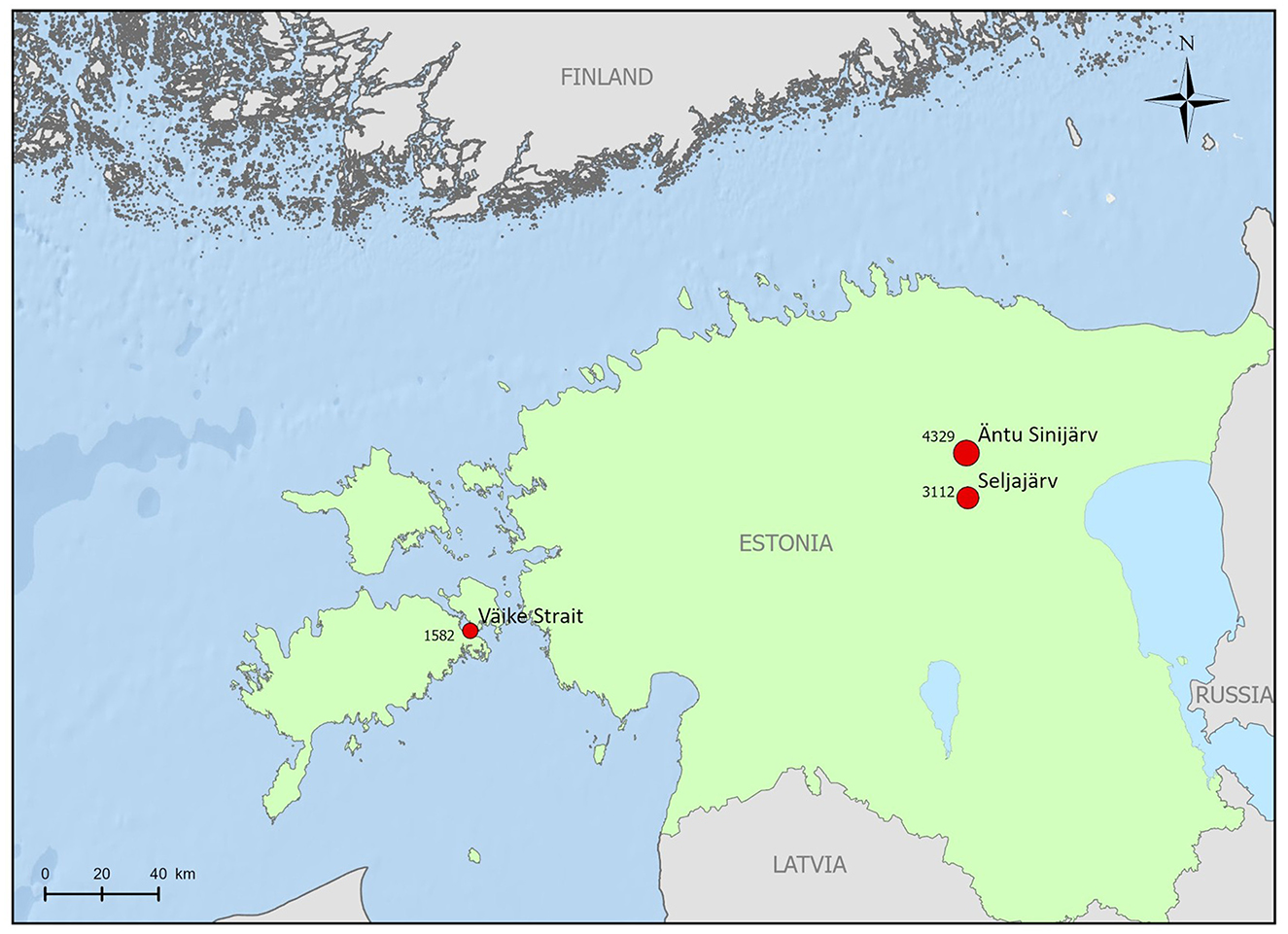
Figure 1. Geographic locations of the studied sites in Estonia, including Väike Strait, Seljajärv, and Äntu Sinijärv, with corresponding DIC (μmol kg−1) values calculated based on pH and total alkalinity measurements.
For background information, the daily natural fluctuations of pH levels, photosynthetically active radiation (PAR), and oxygen (mg l−1) were measured in shallow water (ca 2 m). Measurements were carried out in Väike Strait during the active vegetation period in July (58.5126°N, 23.1981°E; Figure 2). The coverage of the species were C. aspera 100%, C. tomentosa 1%, and C. connivens 1% in the study area. Measurements were performed using an optical CO2 sensor (AMT Analysenmesstechnik GmbH, Germany) attached to a CTD sensor system (Sun & Sea Technology GmbH, Germany), which was placed among vegetation at a depth of 2 m during a full 24-h cycle with a frequency of 20 min in July 2018. The irradiance at a depth of 2 m was measured as PAR using an ODYSSEY PAR Logger.
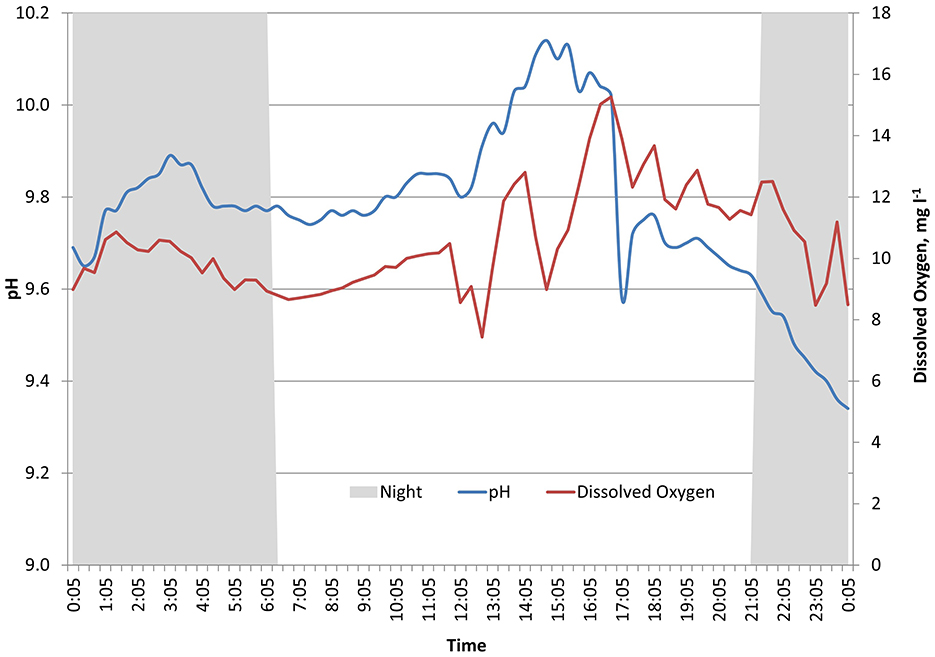
Figure 2. Natural fluctuations of brackish water pH and dissolved oxygen (mg l−1) measured among charophytes habitat at the depth of 2 m, during an active vegetation period in July, in the Väike Strait (continuous recordings within 24 h). The gray area represents night (PAR < 50 μmol m−2 s−1).
2.1 Carbonate system calculations
For the calculation of carbonate chemistry, we used total alkalinity (TA), pHNBS (National Bureau of Standards scale), temperature, and salinity values. These parameters were input into the CO2SYS software (Lewis and Wallace, 1998) to compute the carbonate chemistry (Table 1). For alkalinity values, the water samples (250 ml each) were collected from Väike Strait, Seljajärv, and Äntu Sinijärv at a depth of 0.5 m using laboratory glass bottles, which were filled carefully to avoid any air bubbles. The samples were preserved at 4°C until the analysis of TA, following the HELCOM draft guidelines for sampling and determination of TA (HELCOM, 2016). Triplicate subsamples were analyzed for TA using potentiometric titration with a Metrohm 848 Titrino Plus. The pHNBS value used for the carbonate system calculations is the year-round average pH of the site, and the temperature is the summer average of that year when the alkalinity samples were collected.
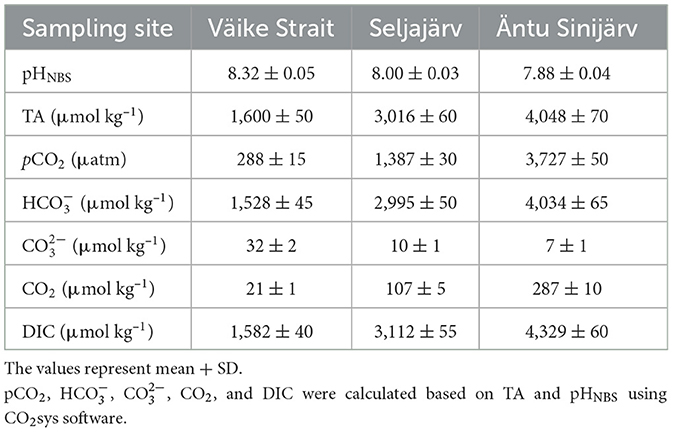
Table 1. Carbonate chemistry of Väike Strait (brackish Baltic Sea), Seljajärv (a freshwater lake), and Äntu Sinijärv (a freshwater lake).
2.2 Laboratory experiments
For the laboratory experiments, all specimens collected from Väike Strait, Äntu Sinijärv, and Seljajärv were placed in coolers containing water collected at the site and transported to the laboratory immediately. P vs. DIC curves and pH drift experiments were carried out using the laboratory facilities of the Kõiguste field station at the Estonian Marine Institute, the University of Tartu in Saaremaa Island. Using forceps the collected specimens were carefully cleaned of all macroscopic epiphytes before the start of the experiments.
2.2.1 pH drift experiments
The aim of the pH drift experiment was to determine the relative affinity of charophyte species. When algae are capable of raising the pH above 9 in seawater (≈35 PSU and 2,300 μmol/L carbonate alkalinity) then they are theoretically able to use bicarbonate (), because this is the point when CO2 is virtually absent in the medium or appreciably low and should limit the photosynthesis for obligate CO2 users (Maberly, 1990). Due to differences in salinity and DIC availability between the seawater and the brackish and freshwater used in this study, revised pH compensation points were calculated from data from the sites (Table 1) using the R package seacarb (Gattuso et al., 2022). In this study, the pH compensation point was taken to be 9.2 for brackish water (Väike Strait) and 9.8 for freshwater lakes (Seljajärv and Äntu Sinijärv). To detect whether charophytes C. aspera and C. tomentosa collected from brackish water and freshwater lakes are capable of using bicarbonate as an external carbon source for photosynthesis the pH was measured before and after the incubation of the algae (Maberly, 1990; Raven et al., 2005; Hepburn et al., 2011; Cornwall et al., 2017).
Before the start of the experiments, three individuals (n = 3) of both charophyte species from brackish Baltic Sea and freshwater lakes were cut ~0.5–0.6 g wet weight apical parts and acclimatized in water collected from the site for 24 h. In the experiment, the acclimatized specimens were incubated in 60 ml sealed, transparent containers filled with brackish water (pH 8.15; salinity 6 PSU) and freshwater (pH 8.10; salinity 0.2 PSU). Waters used for experiments were sterilized with an AquaCristal 18W ultraviolet sterilizer and passed through a Glass Microfiber filter (GF/F, Ø 47 mm). All replicates were placed randomly onto a shaker table under a continuous irradiance of 80 μmol m−2 s−1 of PAR at 15°C. The PAR was measured with an LI-COR 250 light meter.
In the containers, the pHNBS readings were taken after 24, 32, and 48 h. After the final pH measurements, the algal material was removed and the containers with brackish and freshwater were left open for 48 h. Then the pH was measured again to determine whether the brackish and freshwater had re-equilibrated with the atmosphere. The pH was measured using a METTLER TOLEDO InLab® Expert Pro-ISM-IP67 pH-electrode (accuracy: ±0.001 and resolution: ±0.002) connected to a METTLER TOLEDO model Seven2GoTM pro S8 pH/Ion Meter and calibrated against the NBS buffers.
2.2.2 Photosynthesis vs. dissolved inorganic carbon
The aim of photosynthesis vs. dissolved inorganic carbon experiment was to provide characteristics of carbon acquisition for C. aspera and C. tomentosa from brackish water and freshwater lakes under different DIC concentrations by using the oxygen evolution rates. Before the start of the experiments, three individuals of both charophyte species from brackish Baltic Sea and freshwater lakes were cut ~0.5–0.7 g wet weight apical parts and acclimatized in brackish and freshwater for 24 h. Then the waters used for the experiment were filtered and UV-treated, as described in detail above.
To maintain the pH constant throughout the experiment Tris buffer solution was used. The effect of the Tris buffer on the photosynthetic ability was tested on both species and both habitats –brackish water (n = 3) and freshwater (n = 3). There was no significant difference in photosynthesis rate between the trials with and without Tris (final concentration of 15 mM, data not shown).
For the experiments, DIC-free brackish and freshwater were created following the methods of Beardall and Roberts (1999). Briefly, the pH of Tris-buffered seawater was lowered to 3 using a 1 M HCl solution, sparged with nitrogen gas for 2 h and then raised to the experimental pH of 8.1 using 1 M NaOH. Photosynthetic experiments were carried out at 10°C in 245 mL airtight chambers under photosynthetically saturating irradiance (500 μmol photons m−2 s−1 of PAR). The water temperature was maintained at 10°C by immersing the chamber in a water bath, with a magnetic stir bar (separated from the algal tissue with a mesh screen) to provide water motion inside the chamber. Approximately every 7–10 min, 0.3 M solution was injected into the chamber to increase the DIC concentrations sequentially to 0.2, 0.6, 1.2, 2, 3, 4.5, 7, and 10 mM. The oxygen evolution was measured using an Ocean Optics′ NeoFox-TP oxygen probe connected to Ocean Optics′ NeoFox Viewer software. Probes were calibrated using a two-point calibration, achieved by bubbling DIC-free brackish and freshwater with nitrogen and ambient air. Three replicate curves of all studied species were conducted. After the experiments, samples were dried at 60°C until a constant weight was reached. Dry weights were used to normalize the photosynthetic rate (μmol O2 hour−1 gDW−1) of the individuals at each DIC concentration.
2.2.3 Natural carbon isotope (δ13C) analysis
The goal of the carbon isotope (δ13C) analysis was to detect the presence or lack of a carbon CCM operation for C. aspera and C. tomentosa from brackish water and freshwater lakes. When the value of δ13C is between −30 and −10‰ it indicates the presence of CCM and the uptake of both carbon forms (CO2 and ) for macroalgal photosynthesis (Raven et al., 2002). The δ13C values above −10‰ indicate the presence of CCM and primarily the use of . The δ13C values below −30‰ indicate that there is no CCM present and macroalgae rely purely on diffusive CO2 supply (Raven et al., 2002).
Three individual (n = 3) specimens of both species from brackish Baltic Sea and freshwater lakes were cut ~0.5–0.6 g wet weight sections and were dried at 60°C until a constant weight was reached, ground into a fine powder using a mortar and pestle and stored in microcentrifuge tubes. Three replicates of both studied species from both habitats were analyzed using an elemental analyzer (FlashEA 1112 HT, Thermo Scientific) interfaced through a ConFlo IV dilution device (Thermo Scientific) with an isotope ratio mass spectrometer (Delta V Plus, Thermo Scientific). About 1 mg of the sample was weighed into tin capsules for determination of δ13C/δ12C. The C isotope composition is reported per mill respective to Vienna Pee Dee Belemnite (V-PDB) and calibrated using international IAEA standards IAEA-CH-3 and IAEA-CH-6. Long-term reproducibility precision and accuracy were ±0.1‰. δ13C isotopic analysis of DIC was conducted from both studied lakes. Waters containing DIC were analyzed by Continuous Flow Isotope Ratio Mass Spectrometry (CF-IRMS) using a Thermo Finnigan GasBench coupled to a DeltaVPlus.
2.3 Statistics
Statistical analysis was conducted using the R statistical software platform (R Core Team, 2017). For the pH drift experiment, the final (48 h) pH values for C. aspera and C. tomentosa from brackish water and freshwater were compared using a two-way ANOVA post-hoc-test and two-tailed t-test. For the P vs. DIC experiment, a Michaelis-Menten curve (Johnson and Goody, 2011) was fitted to plots of photosynthetic rate vs. DIC concentration. The Michaelis-Menten equation is P = Pmax/DIC + K0.5, where Pmax is the point at which the maximum photosynthetic rate of the organism is reached and K0.5 is the concentration of DIC at which the photosynthetic rate of the organism is half of Pmax (Johnson and Goody, 2011). A maximum likelihood, non-linear mixed-effects modeling was approached using the lme4 package in R (Bates et al., 2015). This approach allowed a single model to be used, whilst accounting for species-level (fixed factor, C. aspera and C. tomentosa from brackish water and freshwater) and individual-level (random factor, replicate incubation id) variability in Pmax and K0.5. Within-species comparisons of Pmax and K0.5 were made using simultaneous t-tests, using the R package multcomp (Hothorn et al., 2008).
3 Results
The pH compensation points of C. aspera and C. tomentosa were significantly higher in brackish than in freshwater (two-way ANOVA: f = 17.39, p = 0.003). In brackish water, the pH compensation points of C. aspera and C. tomentosa were 10.51 ± 0.06 and 10.51 ± 0.04 (mean ± SE; n = 3), respectively. In fresh water, the pH compensation points of C. aspera and C. tomentosa were 8.99 ± 0.14 (n = 3) and 9.45 ± 0.10 (n = 3), respectively. Also, there was no significant difference between C. aspera and C. tomentosa collected from the freshwater lakes (two-tailed t-test: t = 1.1043, p = 0.3316) and from the brackish Baltic Sea (two-tailed t-test: t = 4.7058, p = 0.112).
The value of δ13C for brackish water C. aspera was −13.11‰ ± 0.26 (mean ± SE, n = 3), for brackish water C. tomentosa −13.88‰ ± 0.22 (n = 3), indicating the presence of CCM operation (uptake of both CO2 and ). The carbon isotope value for freshwater C. aspera was −30.23‰ ± 0.27 (n = 3) and for freshwater C. tomentosa −32.33‰ ± 0.90, indicating the reliance of mainly on CO2 (Figure 3). The differences in δ13C in DIC values between Seljajärv (−9.24‰) and Äntu Sinijärv (−12.93‰) suggest variations in carbon sources or processes affecting DIC in these lakes.
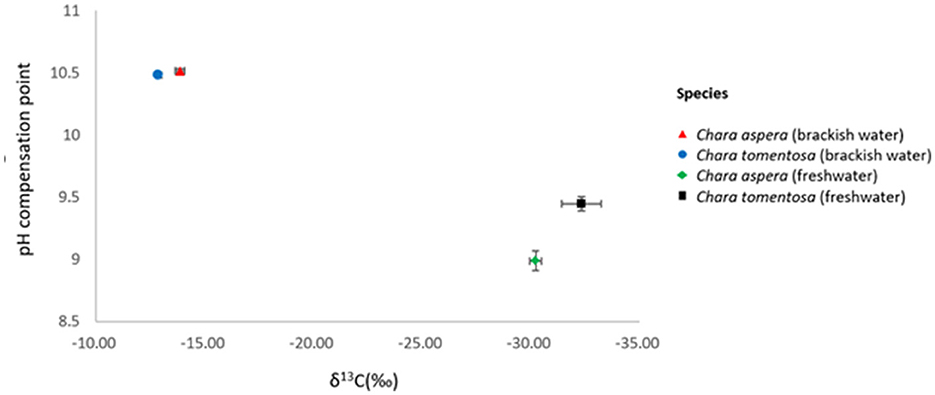
Figure 3. pH compensation points vs. carbon stable isotope (δ13C) values of the charophyte species C. aspera and C. tomentosa from freshwater and brackish Baltic Sea ±SE (n = 3).
Pmax of C. tomentosa from brackish water was 114.68 ± 12.39 (RATE ± SE, μmol O2 hour−1 gDW−1), was significantly higher than C. tomentosa from freshwater 52.14 ± 7.57 (RATE ± SE, μmol O2 hour−1 gDW−1; post-hoc test; p = 0.002) and C. aspera from freshwater 58.68 ± 6.65 (RATE ± SE, μmol O2 hour−1 gDW−1; post-hoc test; p = 0.005). There were, however, no significant differences between the K0.5 values for these curves (p = 0.915 and 0.999 respectively), as well as no other significant differences between Pmax and K0.5 values for any other comparisons (Figure 4; Table 2).
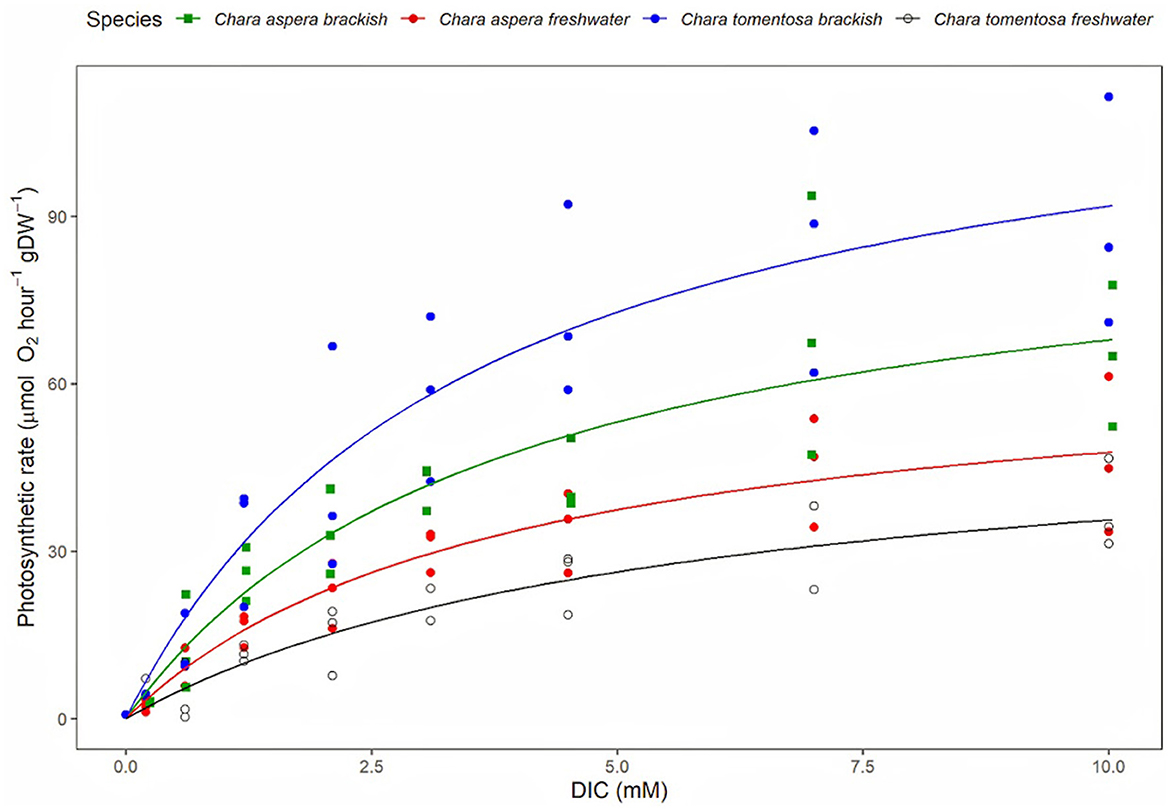
Figure 4. The photosynthetic rates of C. aspera and C. tomentosa, from freshwater (n = 3) and brackish Baltic Sea (n = 3), at different concentrations of dissolved inorganic carbon (mM).
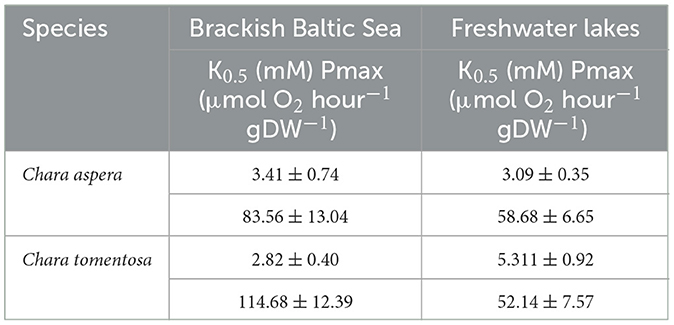
Table 2. The half-saturation point (K0.5) and maximum photosynthetic rate (Pmax) of brackish and freshwater C. aspera and C. tomentosa ±SE (n = 3).
4 Discussion
Over a decade of research into ocean acidification illuminates various responses by oceanic biota (Gattuso and Hansson, 2011; Mercado and Gordillo, 2011; Terhaar et al., 2020). However, in the Baltic Sea, there is a lack of data to predict the response of macrophyte communities to acidification and in freshwater lakes (all over the world), this information is basically absent. In this study, we expected the same charophyte species growing in different habitats will likely use different carbon uptake mechanisms.
Our results from the pH drift experiment showed that C. aspera and C. tomentosa growing in brackish water likely possess a CCM. Both species raised the pH well above the pH compensation point. The freshwater C. aspera and C. tomentosa pH drift values stayed under the calculated thresholds, indicating the reliance on solely CO2. Furthermore, the pH compensation points for brackish C. aspera and C. tomentosa were significantly higher compared to freshwater C. aspera and C. tomentosa. The brackish water pH drift results presented here are also compatible with our measurements from the Väike Strait, which show charophytes ability to raise pH above 10 in their natural community in summer conditions. This indicates an effective CCM operation since at pH 9.2 the concentration of CO2 is too low to support photosynthetic activity for obligate CO2 users. Ray et al. (2003) documented the occurrence of charasomes in the cell membrane of C. tomentosa growing in the northern Baltic Sea, the simultaneous high periplasmic carbonic anhydrase and proton pump activities suggest proton-pump driven H+ extrusion and membrane transport of CO2 derived from as the major form of DIC acquisition in this species. In general, macrophytes having the ability to use are better adapted to environments with high pH and low CO2 e.g., the brackish Baltic Sea (Maberly and Spence, 1983; Ray et al., 2003).
Our pH drift experiment results of brackish water charophytes are also consistent with the carbon isotope (δ13C) values which were between −30 and −10‰ indicating the presence of CCM and the uptake of both carbon forms (CO2 and ) for photosynthesis. However, we acknowledge that the baseline δ13C values for DIC in different areas of the Baltic Sea may vary due to the nature of the brackish environment. Given the δ13C values of −13.11‰ and −13.88‰ for C. aspera and C. tomentosa in our study, these values fit within the expected range for a mixed marine and freshwater system, indicating the presence of CCMs and utilization of both CO2 and for photosynthesis (Rolff and Almesjö, 2009; Emeis et al., 2014). The brackish C. aspera and C. tomentosa had relatively more positive δ13C values than freshwater charophytes, indicating the uptake of different DIC species for photosynthesis: primarily the use of in the brackish Baltic Sea and CO2 in freshwater lakes. Furthermore, we found that the studied freshwater lakes have significantly higher pCO2 concentrations than the brackish Baltic Sea.
Seljajärv's average δ13C value of −9.24‰ suggests an enriched δ13C signature, likely due to significant bicarbonate contributions from limestone or other mineral sources, and perhaps a minimized role of organic matter decomposition. In contrast, Äntu Sinijärv's δ13C value of −12.93‰ indicates a more depleted δ13C, pointing toward a greater impact from organic decomposition or atmospheric CO2 mixing into the lake's carbon pool. Notably, bicarbonate from mineral sources, as documented in literature (Ott, 2011), contributes to both lakes, suggesting a common geochemical influence. The disparity in δ13C between the charophytes and their respective lake's DIC could illuminate the effectiveness of the CCMs in harnessing available carbon. More proficient CCMs are expected to show minimal deviation from the ambient DIC δ13C, indicating a strategic adaptation to local carbon sources. Therefore, the reason for the different carbon uptake mechanisms between brackish and freshwater charophytes is driven by the environments where they grow—the freshwater charophytes probably do not need to use energetically expensive since the availability of energetically inexpensive CO2 is in abundance.
Also, the P vs. DIC experiment results show the freshwater charophytes are likely carbon-saturated, meaning their photosynthetic processes are operating at their maximum efficiency due to the availability of DIC in their environment. In Seljajärv, the DIC concentration (3,112 ± 55 μmol kg−1) is close to the K0.5 for C. aspera (3,090 ± 350 μM) but significantly lower than the K0.5 for C. tomentosa (5,311 ± 920 μM). This suggests that C. aspera is likely well-adapted to the available DIC levels in Seljajärv, while C. tomentosa might be less efficient due to its higher K0.5. In the brackish Baltic Sea, the K0.5 values for both species (C. aspera: 3,410 ± 740 μM, C. tomentosa: 2,820 ± 400 μM) are higher than the DIC concentration (1,582 ± 40 μmol kg−1). This suggests both species might be operating below their optimal DIC saturation levels, indicating potential limitations in their carbon uptake efficiency in this environment. C. tomentosa has a lower K0.5 than C. aspera, indicating a higher affinity for DIC, but given that both K0.5 values exceed the available DIC, both species may still face DIC limitation, with C. tomentosa being slightly more efficient under these suboptimal conditions.
The majority of papers suggest the genus Chara ability to use for photosynthesis (Price et al., 1985; Van den Berg et al., 2002; Ray et al., 2003). Van den Berg et al. (1998) found that C. aspera had a high photosynthetic rate over a wide range of concentrations, suggesting efficient bicarbonate use. Pronin et al. (2016) studied seven different lakes in Poland and found C. tomentosa carbon isotope values varied on average between −15‰ to −21‰, suggesting is the main source of carbon for C. tomentosa. Furthermore, Osmond et al. (1981) found that at pH 7.0 and 7.5, and high CO2 levels the submerged macrophyte Stuckenia pectinata L. stable carbon isotope values were −25.0‰ and −25.7‰. However, in shallow lakes when CO2 concentration approaches zero, under such conditions, Stuckenia pectinata relies solely on , uptake as inferred from pH-drift experiments under comparable conditions (Sand-Jensen, 1983). In the recent study by Pronin et al. (2023) they examined the δ13C values of the charophyte Nitella flexilis in both softwater and hardwater lakes and reported the δ13C values did not significantly differ between the two types of lakes but showed more variation in softwater lakes. They concluded that the variability is attributed to different proportions of carbon forms (CO2 and ) available for photosynthesis, influenced by pH. These results indicate high variability of carbon uptake mechanisms between different growth environments of the same species. Based on this study, we cannot exclude the possibility that the studied freshwater charophytes have physiological mechanisms available for the operation of CCM when the concentration of CO2 is too low for photosynthesis. However, this needs further investigation using different experimental approaches.
Studies have shown that macrophytes have different types of CCMs with varying energetic efficiencies (Giordano et al., 2005; Hepburn et al., 2011; Cornwall et al., 2017). The sheltered nature of charophyte habitats likely plays an important role in DIC acquisition. Our measurements in the brackish Baltic Sea indicate that CO2 concentration is virtually absent during the active growth period in charophyte habitats. Therefore, the studied charophytes have likely developed an effective CCM, enabling them to raise the pH above 10 in the Väike Strait. However, based on our P vs. DIC experiment results the brackish Baltic Sea C. tomentosa and C. aspera might be limited by current DIC availability (~1.5 mM). Furthermore, brackish C. tomentosa Pmax was significantly higher compared to freshwater charophytes indicating an effective DIC uptake. This is consistent with Pajusalu et al. (2015) who found that C. tomentosa exhibited a significant increase in net primary production rates when the CO2 concentration was elevated until 2,000 μatm. Also, C. aspera showed only a slight response to elevated pCO2, possibly due to differences in the ecophysiology and life strategy of the species (Pajusalu et al., 2015). Furthermore, our P vs. DIC experiment results refer to a possible scenario when the atmospheric CO2 concentration increases by 150–250% predicted by IPCC (2022), the brackish Baltic Sea C. tomentosa and C. aspera might be able to downregulate CCM operation e.g., switch from an to CO2 based metabolism. This could mean that lowered pH will have a positive impact on sheltered parts of the Baltic Sea where charophytes grow.
Another possible explanation why the brackish water charophytes could be DIC limited might be related to the extent of calcification. Calcite-encrusted charophytes are usually associated with base-rich waters associated with limestone in the catchment (Coletta et al., 2001). In this study, we found that alkalinity is much higher in freshwater lakes compared to brackish water. Charophytes are generally thought to be efficient calcifiers in freshwater (McConnaughey and Whelan, 1997; Lucas and Berry, 1985), which ensures the conversion of to free CO2 for continued photosynthesis without increasing pH to inhibiting levels (Ca2+ + 2 → CaCO3 + CO2; Andersen et al., 2017). However, in the northern Baltic Sea, C. tomentosa has much thinner CaCO3 incrustations than in freshwater lakes close to the Baltic Sea coast (Ray et al., 2003). use without calcification increases pH ( → CO2 + OH−) which may inhibit photosynthesis (Andersen et al., 2017). There is a lack of research available about the mechanisms and peculiarities of Chara calcification in the brackish Baltic Sea compared to freshwater environments, however, the species calcification in the Baltic Sea could be minor due to low calcium conditions.
4.1 Summary statement
The study showed that freshwater and brackish water C. aspera and C. tomentosa likely use different carbon uptake mechanisms. Our results indicated that freshwater charophytes preferentially use CO2 and brackish water charophytes , likely due to their local acclimatization to different growth environments. At present, the high pCO2 concentrations in Äntu Sinijärv and Seljajärv indicate that these lakes might be functioning as CO2 sources rather than sinks. Therefore, the freshwater C. tomentosa and C. aspera are likely carbon saturated in these lakes and probably will not gain photosynthetic advantages from acidification. However, the predicted increase in CO2 concentration may positively affect the growth of the charophytes in the brackish Baltic Sea.
5 Future research needs
This study opened an understanding of the carbon uptake strategies of C. aspera and C. tomentosa growing in the brackish Baltic Sea and in the freshwater lakes of Estonia. The comparison underscores the importance of considering both biological and geochemical perspectives to fully appreciate the nuances of aquatic plant ecology and the carbon cycle. However, for a more detailed understanding of the CCM operation in these species, the next step will be to carry out the pH banding experiments e.g., identifying the alkaline and acid zones to determine the level of transport. Additionally, gathering more background information about the freshwater lakes will be important. This includes measuring daily natural fluctuations of pH levels, photosynthetically active radiation (PAR), and oxygen levels among the vegetation, similar to the studies carried out in the brackish Baltic Sea.
Data availability statement
The original contributions presented in the study are included in the article/supplementary material, further inquiries can be directed to the corresponding author.
Author contributions
GA: Formal analysis, Investigation, Visualization, Writing – original draft, Writing – review & editing. LP: Conceptualization, Data curation, Supervision, Writing – review & editing. DP: Formal analysis, Methodology, Writing – review & editing. CH: Conceptualization, Writing – review & editing. KT: Conceptualization, Investigation, Writing – review & editing. TP: Conceptualization, Investigation, Writing – review & editing. AP: Data curation, Formal analysis, Writing – review & editing. GM: Funding acquisition, Supervision, Writing – review & editing.
Funding
The author(s) declare that financial support was received for the research, authorship, and/or publication of this article. Funding for this study was provided through the basic research and infrastructure fund of the University of Tartu Estonian Marine Institute.
Acknowledgments
The authors would like to acknowledge those who have aided us in the completion of this work.
Conflict of interest
The authors declare that the research was conducted in the absence of any commercial or financial relationships that could be construed as a potential conflict of interest.
Publisher's note
All claims expressed in this article are solely those of the authors and do not necessarily represent those of their affiliated organizations, or those of the publisher, the editors and the reviewers. Any product that may be evaluated in this article, or claim that may be made by its manufacturer, is not guaranteed or endorsed by the publisher.
References
Albert, G., Hepburn, C. D., Pajusalu, L., Paalme, T., Pritchard, D. W., and Martin, G. (2020). Could ocean acidification influence epiphytism? A comparison of carbon-use strategies between Fucus vesiculosus and its epiphytes in the Baltic Sea. J. Appl. Phycol. 32, 2479–2487. doi: 10.1007/s10811-019-01953-z
Alin, S. R., and Johnson, T. C. (2007). Carbon cycling in large lakes of the world: a synthesis of production, burial, and lake-atmosphere exchange estimates. Glob. Biogeochem. Cycl. 21:2881. doi: 10.1029/2006GB002881
Andersen, M. R., Kragh, T., and Sand-Jensen, K. (2017). Extreme diel dissolved oxygen and carbon cycles in shallow vegetated lakes. Proc. Royal Soc. B 284:20171427. doi: 10.1098/rspb.2017.1427
Atilla, N., McKinley, G. A., Bennington, V., Baehr, M., Urban, N., DeGrandpre, M., et al. (2011). Observed variability of Lake Superior pCO2. Limnol. Oceanogr. 56, 775–786. doi: 10.4319/lo.2011.56.3.0775
Bates, D., Maechler, M., Bolker, B., and Walker, S. (2015). lme4: Linear Mixed-Effects Models Using Eigen and S4. Available at: https://cran.r-project.org/ (accessed March 10, 2020).
Beardall, J., and Raven, J. A. (2004). The potential effects of global climate change on microalgal photosynthesis, growth and ecology. Phycologia 43, 26–40. doi: 10.2216/i0031-8884-43-1-26.1
Beardall, J., and Roberts, S. (1999). Inorganic carbon acquisition by two Antarctic macroalgae, Porphyra endiviifolium (Rhodophyta: Bangiales) and Palmaria decipiens (Rhodophyta: Palmariales). Polar Biol. 21, 310–315. doi: 10.1007/s003000050367
Bennington, V., McKinley, G. A., Urban, N. R., and McDonald, C. P. (2012). Can spatial heterogeneity explain the perceived imbalance in Lake Superior's carbon budget? A model study. J. Geophys. Res. 117:1895. doi: 10.1029/2011JG001895
Blindow, I., and van de Weyer, K. (ed.), (2016). “Ökologie der Characeen,” in Armleuchteralgen (Berlin; Heidelberg: Springer Spektrum) 79–95.
Cole, J. J., Prairie, Y. T., Caraco, N. F., McDowell, W. H., Tranvik, L. J., Striegl, R. G., et al. (2007). Plumbing the global carbon cycle: integrating inland waters into the terrestrial carbon budget. Ecosystems 10, 172–185. doi: 10.1007/s10021-006-9013-8
Coletta, P., Pentecost, A., and Spiro, B. (2001). Stable isotopes in charophyte incrustations: relationships with climate and water chemistry. Palaeogeogr. Palaeoclimatol. Palaeoecol. 173, 9–19. doi: 10.1016/S0031-0182(01)00305-4
Cornwall, C. E., Revill, A. T., Hall-Spencer, J. M., Milazzo, M., Raven, J. A., and Hurd, C. L. (2017). Inorganic carbon physiology underpins macroalgal responses to elevated CO2. Sci. Rep. 7:46297. doi: 10.1038/srep46297
Doney, S. C., Busch, D. S., Cooley, S. R., and Kroeker, K. J. (2020). The impacts of ocean acidification on marine ecosystems and reliant human communities. Annu. Rev. Environ. Resour. 45, 83–112. doi: 10.1146/annurev-environ-012320-083019
Eadie, B. J., and Robertson, A. (1976). An IFYGL carbon budget for Lake Ontario. J. Great Lakes Res. 2, 307–323. doi: 10.1016/S0380-1330(76)72295-0
Einsele, G., Yan, J., and Hinderer, M. (2001). Atmospheric carbon burial in modern lake basins and its significance for the global carbon budget. Glob. Planet. Change 30, 167–195. doi: 10.1016/S0921-8181(01)00105-9
Emeis, K. C., Neumann, T., Endler, R., Struck, U., Kunzendorf, H., and Christiansen, C. (2014). Stable carbon isotopes in the Baltic Sea. Biogeosciences 11, 2301–2315. doi: 10.5194/bg-11-2301-2014
Gattuso, J., Epitalon, J., Lavigne, H., and Orr, J. (2022). Seacarb: Seawater Carbonate Chemistry. R Package Version 3.3.1. Available at: https://CRAN.R-project.org/package=seacarb (accessed September 15, 2023).
Gattuso, J., and Hansson, L. (2011). Ocean Acidification (New York, NY: Oxford University Press Inc.), 326.
Giordano, M., Beardall, J., and Raven, J. A. (2005). CO2 concentrating mechanisms in algae: mechanisms, environmental modulation, and evolution. Annu. Rev. Plant Biol. 56, 99–131. doi: 10.1146/annurev.arplant.56.032604.144052
Hanson, P. C., Pollard, A. L., Bade, D. L., Predick, K., Carpenter, S. R., and Foley, J. A. (2004). A model of carbon evasion and sedimentation in temperate lakes. Glob. Change Biol. 10, 285–298. doi: 10.1111/j.1529-8817.2003.00805.x
HELCOM (2016). Draft Guidelines for Determination of Total Alkalinity. State and Conservation 4-2016 2MA-6. Available at: https://helcom.fi/
Hepburn, C. D., Pritchard, D. W., Cornwall, C. E., McLeod, R. J., Beardall, J., Raven, J. A., et al. (2011). Diversity of carbon use strategies in a kelp forest community: implications for a high CO2 ocean. Glob. Change Biol. 17, 2488–2497. doi: 10.1111/j.1365-2486.2011.02411.x
Herbst, A., and Schubert, H. (2018). Age and site-specific pattern on encrustation of charophytes. Bot Stud. 59:31. doi: 10.1186/s40529-018-0247-5
Hothorn, T., Bretz, F., and Westfall, P. (2008). Simultaneous inference in general parametric models. Biometr. J. 50, 346–363. doi: 10.1002/bimj.200810425
IPCC (2022). “Climate change 2022: impacts, adaptation, and vulnerability,” in Contribution of Working Group II to the Sixth Assessment Report of the Intergovernmental Panel on Climate Change, eds. H. O. Pörtner, D. C. Roberts, M. Tignor, E. S. Poloczanska, K. Mintenbeck, A. Alegría, et al. Cambridge: Cambridge University Press.
Johnson, K. A., and Goody, R. S. (2011). The original michaelis constant: translation of the 1913 Michaelis-Menten paper. Biochemistry 50, 8264–8269. doi: 10.1021/bi201284u
Krause, W. (1997). Charales (Charophyceae). Süßwasserflora von Mitteleuropa [Charophytes (Charophyceae). Freshwater Flora of Central Europe]. Bd. 18. Jena: G. Fischer.
Kuznetsov, I., and Neumann, T. (2013). Simulation of carbon dynamics in the Baltic Sea with a 3D model. J. Mar. Syst. 111–112, 167–174. doi: 10.1016/j.jmarsys,.2012.10.011
Laumets, L., Kalm, V., Poska, A., Kele, S., Lasberg, K., and Amon, L. (2014). Palaeoclimate inferred from δ18O and palaeobotanical indicators in freshwater tufa of Lake Äntu Sinijärv, Estonia. J. Paleolimnol. 51, 99–111. doi: 10.1007/s10933-013-9758-y
Lewis, E., and Wallace, D. W. R. (1998). CO2SYS-Program Developed for the CO2 System Calculations. Oak Ridge, TN: Carbon Dioxide Information Analysis Center, Oak Ridge National Laboratory, U.S. Department of Energy.
Lucas, W. J., and Berry, J. A. (1985). Inorganic carbon transport in aquatic photosynthetic organisms. Physiol. Plant. 65, 539–543. doi: 10.1111/j.1399-3054.1985.tb08687.x
Lucas, W. J., and Smith, F. A. (1973). The formation of alkaline and acid regions at the surface of Chara corallina cells. J. Exp. Bot. 24, 1–14. doi: 10.1093/jxb/24.1.1
Maberly, S. C. (1990). Exogenous sources of inorganic carbon for photosynthesis by marine macroalgae. J. Phycol. 26, 439–449. doi: 10.1111/j.0022-3646.1990.00439.x
Maberly, S. C., and Spence, D. H. N. (1983). Photosynthetic inorganic carbon use by freshwater plants. J. Ecol. 71, 705–724. doi: 10.2307/2259587
McConnaughey, T. A., and Falk, R. H. (1991). Calcium-proton exchange during algal calcification. Biol. Bull. 180, 185–195. doi: 10.2307/1542440
McConnaughey, T. A., LaBaugh, J. W., Rosenberry, D., Striegl, R. G., Reddy, M. M., Schuster, P. F., et al. (1994). Carbon budget for a groundwater-fed lake: calcification supports summer photosynthesis. Limnol. Oceanogr. 39, 1319–1332. doi: 10.4319/lo.1994.39.6.1319
McConnaughey, T. A., and Whelan, J. F. (1997). Calcification generates protons for nutrient and bicarbonate uptake. Earth Sci. Rev. 42, 95–117. doi: 10.1016/S0012-8252(96)00036-0
McKinley, G. A., Urban, N., Bennington, V., Pilcher, D., and McDonald, C. (2011). Preliminary carbon budgets for the Laurentian Great Lakes. OCB News 4, 1–7.
Melzner, F., Thomsen, J., Koeve, W., Oschlies, A., Gutowska, M. A., Bange, H. W., et al. (2013). Future ocean acidification will be amplified by hypoxia in coastal habitats. Mar. Biol. 160, 1875–1888. doi: 10.1007/s00227-012-1954-1
Mercado, J. M., and Gordillo, F. J. L. (2011). Inorganic carbon acquisition in algal communities: are the laboratory data relevant to the natural ecosystems? Photosynth. Res. 109:257. doi: 10.1007/s11120-011-9646-0
Nõges, P., Nõges, T., and Laas, A. (2010). Climate-related changes of phytoplankton seasonality in large shallow temperate lakes. Hydrobiologia 646, 81–94. doi: 10.1007/s10750-010-0178-y
Omstedt, A., Edman, M., Anderson, L., and Laudon, H. (2010). Factors influencing the acid-base (pH) balance in the Baltic Sea: a sensitivity analysis. Tellus B 62, 280–295. doi: 10.1111/j.1600-0889.2010.00463.x
Omstedt, A., Edman, M., Claremar, B. J., Frodin, P., Gustafsson, E., Humborg, C., et al. (2012). Future changes in the Baltic Sea acid-base (pH) and oxygen balances. Tellus B 64:19586. doi: 10.3402/tellusb.v64i0.19586
Osmond, C. B., Valaane, N., Haslam, S. M., Uotila, P., and Roksandic, Z. (1981). Comparisons of δ13C values in leaves of aquatic macrophytes from different habitats in Britain and Finland; some implications for photosynthetic processes in aquatic plants. Oecologia 50, 117–124. doi: 10.1007/BF00378804
Ott, I. (2011). Eesti väikejärvede seire 2011. a. Eesti Maaülikooli põllumajandus- ja keskkonnainstituut. Tartu. Available at: envir.ee (accessed April 10, 2011).
Ott, I., and Kõiv, T. (1999). Primary production and phytoplankton in Lake Peipsi-Pihkva. Limnologica 29, 35–44.
Pajusalu, L., Albert, G., Fachon, E., Hepburn, C. D., Kotta, J., Liversage, K., et al. (2020). Ocean acidification may threaten a unique seaweed community and associated industry in the Baltic Sea. J. Appl. Phycol. 32, 2469–2478. doi: 10.1007/s10811-019-01935-1
Pajusalu, L., Martin, G., P?llum?e, A., Torn, K., and Paalme, T. (2015). Direct effects of increased CO2 concentrations in seawater on the net primary production of charophytes in a shallow, coastal, brackish-water ecosystem. Boreal Environ. Res. 20, 413–422.
Phillips, J. C., McKinley, G. A., Bennington, V., Bootsma, H. A., Pilcher, D. J., Sterner, R. W., and Urban, N. R. (2015). The potential for CO2-induced acidification in freshwater: a Great Lakes case study. Oceanography 28, 136–145. doi: 10.5670/oceanog.2015.37
Price, G. D., Badger, M. R., Bassett, M. E., and Whitecross, M. I. (1985). Involvement of plasmalemmasomes and carbonic anhydrase in photosynthetic utilization of bicarbonate in Chara corallina. Funct. Plant Biol. 12, 241–256. doi: 10.1071/PP9850241
Pronin, E., Banaś, K., Chmara, R., Ronowski, R., Merdalski, M., Santoni, A-L., et al. (2023). Do stable carbon and nitrogen isotope values of Nitella flexilis differ between softwater and hardwater lakes?. Aquat. Sci. 85:79. doi: 10.1007/s00027-023-00976-6
Pronin, E., Pełechaty, M., Apolinarska, K., Pukacz, A., and Frankowski, M. (2016). Sharp differences in the δ13C values of organic matter and carbonate encrustations but not in ambient water DIC between two morphologically distinct charophytes. Hydrobiologia 773, 177–191. doi: 10.1007/s10750-016-2698-6
R Core Team (2017). R: A Language and Environment for Statistical Computing. Vienna: R Foundation for Statistical Computing. Available at: https://www.R-project.org/
Raven, J. A., Ball, L. A., Beardall, J., Giordano, M., and Maberly, S. C. (2005). Algae lacking carbon-concentrating mechanisms. Can. J. Bot. 83, 879–890. doi: 10.1139/b05-074
Raven, J. A., Johnston, A. M., Kübler, J. E., Korb, R., McInroy, S. G., Handley, L. L., et al. (2002). Mechanistic interpretation of carbon isotope discrimination by marine macroalgae and seagrasses. Funct. Plant Biol. 29, 355–378. doi: 10.1071/PP01201
Ray, S., Klenell, M., Choo, K. S., Pedersén, M., and Snoeijs, P. (2003). Carbon acquisition mechanisms in Chara tomentosa. Aquat. Bot. 76, 141–154. doi: 10.1016/S0304-3770(03)00035-4
Rolff, C., and Almesjö, K. (2009). The influence of riverine inputs on the carbon and nitrogen isotopic composition of particulate organic matter in the Baltic Sea. Mar. Chem. 114, 160–174.
Saarse, L., and Liiva, A. (1995). Geology of the Äntu group of lakes. Proc. Eston. Acad. Sci. Geol. 44, 119–132. doi: 10.3176/geol.1995.2.05
Sand-Jensen, K. (1983). Photosynthetic carbon sources of stream macrophytes. J. Exp. Bot. 34, 198–210. doi: 10.1093/jxb/34.2.198
Schubert, H., and Blindow, I. (2003). Charophytes of the Baltic Sea. The Baltic Marine Biologists Publication No. 19 (Ruggell: ARG Gantner Verlag Kommanditgesellschaft), 326.
Schubert, H., and Yousef, M. A. M. (2001). Charophytes in the Baltic Sea- threats and conservation. Schriftenr Landschaftspflege Naturschutz 72, 7–8.
Shao, C., Chen, J., Stepien, C. A., Chu, H., Ouyang, Z., Bridgeman, T. B., et al. (2015). Diurnal to annual changes in latent, sensible heat, and CO2 fluxes over a Laurentian Great Lake: a case study in Western Lake Erie. J. Geophys. Res. 120, 1587–1604. doi: 10.1002/2015JG003025
Simm, M., Ott, I., and Kukk, H. (2006). The influence of environmental factors on the spatial distribution of charophytes in a shallow lake (Võrtsjärv, Estonia). Annales Botanici Fennici 43, 195–204.
Spear, D. G., Barr, J. K., and Barr, C. E. (1969). Localization of hydrogen ion and chloride ion fluxes in Nitella. J. Gen. Physiol. 54, 397–414. doi: 10.1085/jgp.54.3.397
Stets, E. G., Striegl, R. G., Aiken, G. R., Rosenberry, D. O., and Winter, T. C. (2009). Hydrologic support of carbon dioxide flux revealed by whole-lake carbon budgets. J. Geophys. Res. 114:783. doi: 10.1029/2008JG000783
Stumm, W., and Morgan, J. J. (1970). Aquatic Chemistry: Chemical Equilibria and Rates in Natural Waters. New York, NY: Wiley-Interscience.
Terhaar, J., Kwiatkowski, L., and Bopp, L. (2020). Emergent constraint on Arctic Ocean acidification in the twenty-first century. Nature 582, 379–383. doi: 10.1038/s41586-020-2360-3
Torn, K., Kovtun-Kante, A., Herkül, K., Martin, G., and Mäemets, H. (2015). Distribution and predictive occurrence model of charophytes in Estonian waters. Aquat. Bot. 120, 142–149. doi: 10.1016/j.aquabot.2014.05.005
Torn, K., Martin, G., Kukk, H., and Trei, T. (2004). Distribution of charophyte species in Estonian coastal water (NE Baltic Sea). Scimar 68, 129–136. doi: 10.3989/scimar.2004.68s1129
Van den Berg, M. S., Coops, H., Meijer, M.-L., Scheffer, M., and Simons, J. (1998). “Clear water associated with a dense Chara vegetation in the shallow and turbid Lake Veluwemeer, The Netherlands,” in The Structuring Role of Submerged Macrophytes in Lakes, eds. E. Jeppesen, M. A. S?ndergaard, M. O. S?ndergaard, and K. Christoffersen (Springer), 339–352. doi: 10.1007/978-1-4615-4370-1_28
Van den Berg, M. S., Coops, H., Simons, J., and Pilon, J. (2002). A comparative study of the use of inorganic carbon resources by Chara aspera and Potamogeton pectinatus. Aquat. Bot. 72, 219–233. doi: 10.1016/S0304-3770(01)00202-9
Keywords: charophytes, carbon physiology, ocean acidification, macrophytes, increasing
Citation: Albert G, Pajusalu L, Pritchard DW, Hepburn CD, Torn K, Paalme T, Põllumäe A and Martin G (2024) Comparison of carbon uptake strategies between Chara aspera and Chara tomentosa growing in the brackish Baltic Sea and in the freshwater lakes of Estonia. Front. Freshw. Sci. 2:1421114. doi: 10.3389/ffwsc.2024.1421114
Received: 21 April 2024; Accepted: 10 September 2024;
Published: 27 September 2024.
Edited by:
Peter Goethals, Ghent University, BelgiumReviewed by:
Evangeline Fachon, Woods Hole Oceanographic Institution, United StatesLeandro E. (Steve) Miranda, US Geological Survey and Mississippi State University, United States
Copyright © 2024 Albert, Pajusalu, Pritchard, Hepburn, Torn, Paalme, Põllumäe and Martin. This is an open-access article distributed under the terms of the Creative Commons Attribution License (CC BY). The use, distribution or reproduction in other forums is permitted, provided the original author(s) and the copyright owner(s) are credited and that the original publication in this journal is cited, in accordance with accepted academic practice. No use, distribution or reproduction is permitted which does not comply with these terms.
*Correspondence: Gerli Albert, gerli.albert@ut.ee