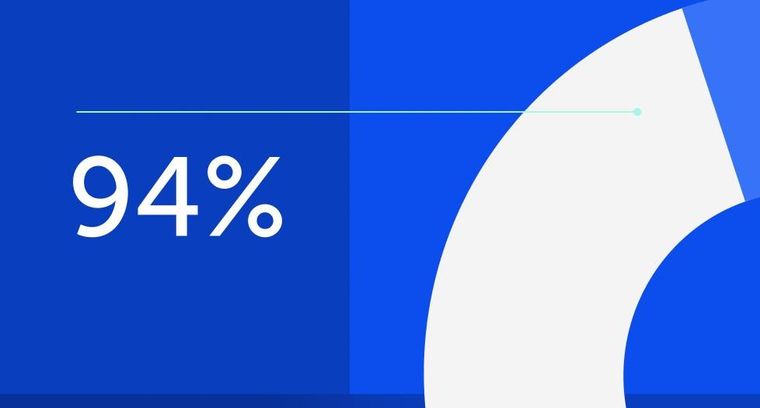
94% of researchers rate our articles as excellent or good
Learn more about the work of our research integrity team to safeguard the quality of each article we publish.
Find out more
ORIGINAL RESEARCH article
Front. Freshw. Sci., 05 July 2024
Sec. Freshwater Species Evolution and Ecology
Volume 2 - 2024 | https://doi.org/10.3389/ffwsc.2024.1398975
Introduction: Global change is a multi-faceted issue putting many species at risk. The broad range of potentially interacting environmental stressors is problematic for effective and efficient conservation and management. In freshwater systems, habitat degradation and introduced species have been repeatedly recognized for their extensive impacts on native ecosystems. However, the simultaneous impacts of these environmental stressors on naturally depauperate and inherently vulnerable communities are poorly understood.
Methods: In southern New Zealand, the fish communities in 14 tributaries of three lowland lakes were surveyed to quantify the within- and between-community changes along gradients of habitat complexity and abundance of introduced species, specifically brown trout (Salmo trutta Linnaeus) and redfin perch (Perca fluviatilis Linnaeus).
Results: Stable isotope analyses identified that trophic diversity increased with habitat complexity and an abundance of native eels (Anguilla spp.) but was unaffected by introduced species. Within each community, only perch exhibited distinct dietary shifts along all environmental gradients, whereas trout and the native fish had consistent, generalist diets. When supported with length–weight regressions, these impacts became increasingly size-dependent. For example, among the native fish, only the larger eels were unaffected by habitat and achieved greater body conditions with increased numbers of eels and perch; however, more trout were detrimental to eel body condition. In contrast, the smaller bodied natives, including elvers, all had improved body conditions from increased habitat complexity and reduced numbers of trout and perch. For the introduced species, perch weights were consistent regardless of the local environment due to their variable diet, but larger trout generally increased in weight with reduced habitat complexity and greater numbers of introduced fish, although high eel densities were detrimental.
Discussion: Overall, our results highlight how the responses to environmental stressors, even in depauperate communities, are complicated and generally species-specific. Nonetheless, habitat degradation had the most wide-ranging negative impacts on native fish, with perch numbers only affecting the smaller bodied natives and trout only affecting one native species. We conclude that focusing on habitat restoration in conservation strategies will provide the most efficient and effective use of resources, although the realized benefits for native species will be limited if introduced species are overly abundant.
Environmental degradation and invasive species are common issues for freshwater habitats that arise repeatedly under the umbrella of global change (Carpenter et al., 1992; Geist and Hawkins, 2016). Examples include widespread land-use modifications that have led to suitable habitats for fish being fragmented, reduced, or homogenized (Reid et al., 2019). The arrival of introduced fish, however, has resulted in native fish being suppressed or locally extirpated through increased competition and/or predation or both (Gallardo et al., 2016). These effects may interact, for example, introduced fish benefitting from degraded habitats, themselves elevating the level of environmental degradation, or some introduced fish benefitting from the presence of other introduced species (Moyle and Light, 1996; Strayer, 2010). Interactions between different stressors can make identifying how best to remedy affected freshwater habitats difficult (Dudgeon et al., 2006; Jackson et al., 2016). Consequently, attempts to rehabilitate or restore degraded freshwater habitats have often failed, with cited causes including, but not limited to, incorrect restorative action (i.e., misidentifying the underlying stressor or its impact) or working at the wrong scale (Geist and Hawkins, 2016; Reid et al., 2019). Decisions about whether to focus on restoring habitats before or after suppressing or removing invasive fish are difficult (Strayer and Dudgeon, 2010) and require an understanding of the independent and interactive effects of modified habitats and introduced fish so that appropriate actions can be taken.
The diversity of a resident community can provide resistance to unnatural changes, particularly to introduced species (Baltz and Moyle, 1993). For example, a community with many species will likely have less niche space available for novel species to occupy and establish a persistent population, conferring a greater resistance to introducing new species (Angermeier and Winston, 1998; Gido and Brown, 1999). Depauperate fish communities (i.e., low in species diversity) are inherently more vulnerable to introduced species. Freshwater fish communities can also become unnaturally depauperate because of environmental changes, such as habitat modification, leading to reduced diversity and increased susceptibility (Fausch et al., 1990; Aarts et al., 2004). For example, smaller or isolated habitats typically have low fish diversity due to the reduced space available to support a more diverse community (Martin-Smith and Laird, 1998; Scheffer et al., 2006). However, despite abundant habitats, isolated islands can also have naturally depauperate communities due to island biogeography and the connection between island size and local species richness (Font and Tate, 1994; McDowall, 2003; Levêque et al., 2008). These naturally depauperate communities may be more vulnerable to environmental stressors, but they also provide an opportunity to study multiple stressors in a relatively simple model system.
Native fish communities, even depauperate ones, may have some resistance to environmental changes (Comte and Olden, 2017). Typically, this resistance is focused on traits of the individual species by using described traits to assign sensitivities to the relevant stressors (Chessman, 2013; Kopf et al., 2017). For example, larger, competitively dominant fish are generally less susceptible to habitat degradation and introduced species than smaller bodied co-occurring species (van der Lee and Koops, 2015; Stefani et al., 2020). Larger, competitively dominant fish could also provide some indirect benefit to smaller co-occurring species by suppressing introduced species (Cucherousset and Olden, 2011). However, this is more complicated in species with notable ontogenetic shifts in habitat use, prey resources, or predation risks because smaller fish have different vulnerabilities compared to their larger conspecifics (King, 2004; Cantin and Post, 2018). When the described traits are not species-specific and are based on general assumptions for the largest size class, species with the greatest differences between larval and adult stages may become the most susceptible to misassigned tolerance to habitat degradation (Henderson and Johnston, 2010; Oele et al., 2019). Therefore, knowledge of size-related differences in species' sensitivity to environmental change is an integral part of species conservation and management, and failing to incorporate these differences into conservation strategies will increase the likelihood of long-term failure.
Changes to habitats or fish communities can lead to the normal available prey resources being reduced through displacement of or competition for resources (Dobson et al., 2006; Jackson et al., 2017). Affected species may alter foraging and feeding behaviors, rely on alternative prey, or increase their energy expenditure to persist in altered habitats (Sowersby et al., 2016; Jackson et al., 2017; da Silva Gonçalves et al., 2018). Changes to trophic interactions due to prey switching can be tracked through stable isotope analyses. The combined isotope ratios of nitrogen (15N:14N) and carbon (13C:12C) can highlight shifts in trophic positions and the origin of dietary resources, respectively, along an environmental gradient (Correa et al., 2012; Meijer et al., 2021). In contrast, shifts in foraging behaviors and potential costs through increased energy expenditure are ideally determined through carefully designed experiments (e.g., Nannini and Belk, 2006; Brand et al., 2021). However, length–weight regressions provide a simplistic way to infer whether environmental conditions are detrimental to the focal species (Cade et al., 2008; Zambrano et al., 2023). Specifically, by comparing individuals of similar length, a reduced weight indicates detrimental environmental conditions. Tracking changes in isotopic signatures and length–weight regressions along gradients of environmental change provides information for inferring how species persistence is likely affected by different environmental stressors.
Somewhat isolated in the South Pacific, New Zealand supports many examples of vulnerable and naturally depauperate freshwater fish communities. Dominated by diadromous fish, the local fish fauna is most abundant and diverse in lowland and coastal habitats, although the average species diversity remains low. For example, Jowett and Richardson (1996) found up to eight species per 50-m reach across 38 New Zealand rivers, which is approximately half the fish diversity found in southeastern Australia (up to 14 species per 50-m reach; Gehrke and Harris, 2000). Introduced salmonids, primarily brown trout (Salmo trutta Linnaeus) and rainbow trout (Oncorhynchus mykiss Walbaum), and percids (redfin perch, Perca fluviatilis Linnaeus) have established populations nationwide and are recognized internationally for their impacts on native fish (e.g., Morgan et al., 2003; McDowall, 2006; Sabetian et al., 2015). New Zealand lowland habitats invaded by these introduced fish have also undergone decades of environmental degradation, particularly habitat homogenisation, due to ongoing land-use intensification and management practices that maintain drainage (Young et al., 2004; Galbraith and Burns, 2007; Julian et al., 2017). Despite the prevalence of salmonids and percids, studies on their impacts in New Zealand have generally assessed either one species or family, occasionally including a specific environmental gradient (e.g., Ludgate and Closs, 2003; McIntosh et al., 2010; Boddy and McIntosh, 2017). Surprisingly, no studies have addressed how invaded fish communities have responded to the combination of salmonids and percids in tandem with ongoing habitat homogenisation. New Zealand provides an ideal place to assess how depauperate communities have responded to the simultaneous impacts of multiple environmental stressors.
For this study, we focused on fish communities of lowland freshwater habitats across the South Island of New Zealand to determine the resistance of naturally depauperate fish communities to environmental change, specifically habitat homogenisation and an increased abundance of introduced fish. We investigated (a) the potential interactions between and within resident fish, both native and introduced, as inferred from isotopic analyses and (b) the shifts in dietary proportions, individual conditions, and species abundance in response to environmental change.
For the potential interactions between and within resident fish, we hypothesize that although a potential overlap in isotopic signatures between species may exist, it will be lowest between pairs of native and introduced species. Once again, the assumption that introduced species were able to establish by occupying under-utilized or empty niches underpins this hypothesis. Regarding the shifts in response to environmental change, we hypothesize that native species will generally experience net negative effects from environmental change, compared to the net positive effects experienced by introduced species.
We used the New Zealand Freshwater Fish Database (National Institute of Water and Atmospheric Research, 2022) and extensive surveys (Meijer, 2024) to identify all freshwater habitats that potentially support populations of native eels (Anguilla spp.) coexisting with redfin perch and brown trout. Five potential locations were identified (see Supplementary Figure S1), but Waihola and Waipouri were eventually excluded due to the inability to access most of their tributaries for sampling. This left Māhinapua (four tributaries), a tannin-stained eutrophic lake in a mixed indigenous forest/agriculture catchment on the west coast; Te Waihora (eight tributaries), a supertrophic shallow lake in a mixed urban/agriculture catchment on the east coast; and Wairewa (two tributaries), a supertrophic shallow lake in a mixed indigenous forest/agriculture catchment on the east coast. Importantly, each lake is connected to tributaries that appeared to vary in habitat (based on personal observations) and have an abundance of the three focal predators.
For each accessible tributary, a 30-m reach was established in a wadable section of the stream, positioned as close as possible to the respective lake. Dissolved oxygen (YSI Ecosense ODO200 probe), pH, and specific conductivity (YSI Pro1030 probe) were measured to assess differences in general water chemistry between tributaries. Environmental gradients that may affect fish were measured at five sites within the reach: canopy cover, macrophyte cover, large woody debris (LWD), bank vegetation cover, and fine-sediment cover. The canopy cover was measured with a spherical convex densiometer held at hip height. Macrophyte cover, LWD, bank vegetation cover, and sediment cover were each estimated across a 5-m section of the stream centered on where the surveyor was standing. The same individual made all estimates to minimize bias. The average of these five measurements was used in all subsequent analyses. A Wolman pebble count (Wolman, 1954) was used to characterize substrate size distributions. The discharge was calculated from a transect across an unobstructed portion of the stream, with two additional transects (three total) used for stream width and depth measurements. Distance from the lake (to the nearest 0.1 km) was estimated from aerial imagery.
The environmental conditions within the selected 30-m wadable reaches did not necessarily reflect the general conditions of these habitats, especially the highly sedimented streams around Te Waihora. To overcome this, we also used observations from a 500-m streamside walk to broadly characterize the habitat complexity of all surveyed tributaries using a categorical approach. Five categories were used to assess overall complexity, with each category rated from 1 to 4 (see Supplementary Table S1). Categories were flow variability, habitat heterogeneity, stream shading, bank conditions, and instream features, with higher values representing increased complexity. An overall habitat complexity for each tributary was calculated as the cumulative scores of all categories. Three levels were used to define overall habitat complexity (see Supplementary Table S2); the four tributaries with the lowest scores (6–10) were classed “low complexity,” the four tributaries with the highest scores (14–17) were classed “high complexity,” and the remaining six tributaries (scored 11–13) were classed “moderate complexity.” Although unbalanced, these groupings were used to ensure distinction between the high- and low-complexity tributaries.
Fish were captured using one-pass electrofishing and stop nets, with efforts focused on highly vegetated or overhung bankside portions of a reach to ensure that fish, particularly eels, were not missed. We recorded the species present as individuals per square meter. Due to the inherent bias in fish capture from sampling a wadable section of each tributary (i.e., under-representation of fish associated with deeper water), we also set a series of six single-winged unbaited fyke nets [5 (l) × 0.7 (d) m, with a 5-m wing and 4-mm mesh] downstream of the sampling reach. The nets were used to capture fish not typically associated with shallow, faster flowing wadable sections of streams, namely, perch and smelt (Retropinna retropinna Richardson). Additionally, we caught larger perch using two angling methods, lure trolling and baited hooks. Consequentially, for fish captured with fyke nets or by angling, their abundance was recorded as catch per unit effort (CPUE; fish per fyke net or fish per 3-h angling, respectively). All fish were anesthetized in a stream water bath of Aqui-S (at 20 mg Aqui-S per liter of water), measured, and weighed. A subset of up to five individuals of each species from each tributary was selected for isotopic analysis, with these fish encapsulating the size range present within that tributary. Anguillid species were fin-clipped, using individuals >150 mm long, and then the equations of Hicks et al. (2022) were used to calculate the muscle tissue–equivalent isotopic values. However, due to difficulties of fin-clipping the smallest eels, one elver (< 150 mm) was euthanised at each tributary using a concentrated stream water bath of Aqui-S (at 200 mg Aqui-S per liter of water). For all other species, we euthanised individuals in this concentrated Aqui-S bath before individually sealing all fish and fin clips into vials or bags for transport back to the laboratory. For each tributary, the local invertebrates were sampled using a kick net, with three subsamples taken randomly across the reach by disturbing the substrate or plants immediately upstream of the net. For baseline comparisons with the freshwater invertebrates, lagoon invertebrates were collected from four locations spread across each lake and combined into one composite community. All collected samples were stored in the laboratory freezer (at −18°C) until isotope samples were prepared.
We inferred the trophic interactions within resident fish communities using isotope ratios of nitrogen (15N:14N) and carbon (13C:12C) from all fish and a subset of invertebrate species that were present, following standard isotope approaches (Vander Zanden et al., 1999; Layman et al., 2012) supplemented with stomach contents analysis. Dorsal muscle tissue from each euthanised fish was extracted by peeling the skin away to expose muscle along the backbone. The stomach contents of these 208 fish (inanga, bullies, perch, and trout) were removed and, as much as possible, identified as a fish species or an invertebrate family under a dissecting microscope. Fish were identified with online keys provided by the National Institute of Water and Atmospheric Research (https://niwa.co.nz/freshwater/nz-freshwater-fish-database/freshwater-fish-id-guideskeys/formal-identification-keys), and invertebrates were identified using keys in Winterbourn et al. (2006). Invertebrate samples were sorted by family into vials, with the most abundant taxa across all sites (i.e., Chironomidae larvae, Deleatidium nymphs, and assorted Crustacea) set aside for isotopic analysis, with the exact composition of invertebrate samples varying between tributaries. These invertebrates were kept separate for sample processing with the isotopic values combined when creating tributary-specific baselines. All tissue samples (i.e., dorsal muscle tissue, fin clips, and abundant invertebrate taxa) were dried for 3 days at 50°C, weighed and ground into a fine powder using a mortar and pestle, and 0.5 mg of material was sealed in tin capsules using a methodology adapted from Meijer et al. (2021). Prepared tin capsules were analyzed by the Institute of Geological and Nuclear Sciences Stable Isotope Laboratory using a Eurovector elemental analyser coupled to an IsoPrime mass spectrometer.
Isotopic results were expressed in per mil delta notation [i.e., δ15N or δ13C = (Rsample/Rstandard – 1) × 1,000, where R = 13C:12C or 15N:14N] relative to the laboratory standard (Leucine), which is calibrated to international standards (VPDB and N-Air). The analytical error associated with the sample analysis was 0.02‰ for δ13C and 0.03‰ for δ15N. Following Post et al. (2007), all samples were lipid-corrected using the formula Δδ13C = −3.32 + 0.99 × C:N.
All data analyses were run in R, version 4.2.1 (R Core Team, 2022), using the R-studio interface (Posit Team, 2022). For the multi-parameter models, partial regression model fits were extracted using the ‘effects' R package (Fox and Weisberg, 2018). For isotopic analyses, all fish signatures were standardized to the tributary-specific freshwater invertebrates to remove the potential effects of baseline shifts both within and between lake catchments. We assessed potential differences in the abundance of the common invertebrates using analyses of variance of dry-weight biomass values.
First, the “SIBER” R package (Jackson et al., 2011) was used to characterize the isotopic signatures of the fish community in each tributary using the six metrics described by Layman et al. (2007): the range of nitrogen values, the range of carbon values, the total isotopic area enclosed within the biplot, the mean distance to the center of the biplot, the mean distance to the nearest neighbor, and the standard deviation of the distance to the nearest neighbor. Using a series of linear mixed-effect models (“lme4” R package; Bates et al., 2015), the effect of categorized habitat complexity was assessed, as were densities of eels, trout, and perch on each of the metrics proposed by Layman et al. (2007), with “lake” included as the random effect. Due to differences in the scale of densities (or CPUE for perch) of the three predatory fish (see Supplementary Table S3), their densities were log-transformed for these models to ensure a consistent scale across species. For simplicity, we used perch CPUE values from fyke nets to define the perch gradient for all analyses. A significant effect would mean a change in an overall fish community food-web property across that respective gradient.
Second, the methods of Stewart et al. (2022) were adapted and developed in a two end-member single isotope (δ13C) mixing model to quantify the proportional contribution of tributary- and lake-derived energy in fish diets (Equation 1). Due to their ecological similarities and limited distributional overlap, we combined longfin eel (Anguilla dieffenbachii Gray) and shortfin eel (Anguilla australis Richardson) into “eels” and common bully (Gobiomorphus cotidianus McDowall) and upland bully (Gobiomorphus breviceps Stokell) into “bullies.” This left six taxa sufficiently abundant for inclusion in these analyses: three predatory fish (eels, trout, and perch) alongside three small-bodied fish that may represent important prey (bullies, inanga [Galaxias maculatus Jenyns], and smelt).
where is the standardized carbon isotope for an individual fish, is the mean carbon isotope value for freshwater invertebrates collected in that tributary, and is the mean carbon isotope value for pooled invertebrates collected from the nearby lagoon environment. This model produced estimated dietary contributions between 0 (i.e., no lagoon resources in diets) and 1 (i.e., diets were exclusively lagoon resources) for each fish. However, due to using only a subset of invertebrates and, subsequently, composite isotopic signatures, 30 fish presented with lagoon-sourced dietary proportions < 0 and >1 (i.e., outside the bounds of the δ13C end-member values set by the selected invertebrate taxa). Nonetheless, we argue that any calculated proportion values outside the bounds of our proportion model represent either the absence of or exclusive dependence on lagoon-derived resources in diets. As such, these values were corrected to 0 and 1, respectively.
The limited overlap in body size ranges between some species meant any attempted model interactions involving body size could be misleading without prior transforming of data, but log-transforming these data was unsuccessful at removing these differences (see Supplementary Table S4). We settled on converting all body lengths into proportions of the largest taxon-specific size from our surveys (i.e., relative body sizes) to minimize differences between species. Using a linear mixed-effect model, we assessed the interaction between species identity and relative body size for estimating dietary proportions across the prey fish. Both relative body size and dietary proportions were logit-transformed to meet the assumptions of normality. “Tributary” was included as the random effect to account for any site-specific variation. This model was repeated for the three predatory fish.
We expanded on the two end-member mixing model by comparing potential niche overlap in isotopic space. To achieve this, linear mixed-effect models were used to estimate size-corrected δ15N and δ13C values for the three prey fish using an interaction between species identity and relative body size, with “tributary” included as the random effect. Differences between species, for these size-corrected isotopes, were tested using a permutational multivariate analysis of variance (PERMANOVA; “vegan” R package; Dixon, 2003), with a Euclidean distance coefficient. We used post hoc pairwise comparisons with a Bonferroni correction to identify prey species, if any, that were isotopically different from the others. These steps were repeated for the three predatory fish.
Next, we assessed how the isotopic signatures of different species responded to overall habitat complexity and predatory fish densities. At this point, the smelt were removed from further analyses due to their low sample size and variability of isotopic signatures, particularly the modeled estimates of dietary proportions. Taxon-specific PERMANOVAs were used to test the effects of habitat complexity and abundance of eels, trout, and perch, on size-corrected δ15N and δ13C values of the five remaining taxa (i.e., eels, inanga, bullies, perch, and trout). The densities of predatory fish were log-transformed. Partial regression plots were made by using a linear mixed-effect model each for δ15N and δ13C values. Permutational dispersion tests (PERMDISP, “vegan” R package) were done to assess if any species exhibited a change in isotopic variability in response to the predictors. For these PERMDISP analyses, the fish densities were converted to categorical ratings. Eel densities were classified as “low” (< 0.5 fish/m2), “medium” (0.5–1.0 fish/m2), or “high” (>1.0 fish/m2); trout densities were classified as “none” (0 fish/m2), “low” (0.01–0.05 fish/m2), or “high” (>0.05 fish/m2); and perch CPUE values were classified as “none” (0 fish per fyke net and 3-h angling), “low” (0.1–5 fish per fyke net or 3-h angling), or “high” (>5 fish per fyke net and 3-h angling).
Building on the isotopic analyses, the impact on individual conditions was inferred by assessing how length–weight regressions changed with habitat complexity and the abundance of eels, trout, and perch. For this, taxon-specific linear mixed-effect models were used to test for differences in length–weight regressions between levels of habitat complexity or the categorical ratings of predator abundances, with “lake” included as a random effect. For all species, we started with the full model (i.e., including interactions between length and all other predictors) and used backward elimination to remove all non-significant interactions until only significant interactions or no interactions remained. Importantly, all main effects remained in the final model. To capture the potential variation in differences in environmental effects on eels, due in part to their large size range and known cannibalistic and competitive interactions (see Jellyman, 1989), we separated individuals up to 150 mm long (hereafter referred to as “elvers”) from the larger congeners for this analysis. This specific size demarcation was chosen from observations that individuals < 150 mm long were never caught in fyke nets (that were set for perch) despite being caught nearby with electric fishing, whereas larger eels were successfully caught using either method.
Finally, the low sample size and limited number of species meant that we did not have the statistical power to reliably identify changes to the overall fish community along environmental gradients (i.e., using PERMANOVAs). Instead, changes in the abundances of five taxa (i.e., eels, inanga, bullies, perch, and trout) were assessed using linear mixed-effect models. These taxon-specific models identified how the abundance of each taxon was affected by the abiotic habitat and the abundance of the co-occurring fish. “Lake” was included as a random effect in these models. Proportional measurements (e.g., canopy cover) were logit-transformed, and non-proportional measurements (e.g. fish densities and substrate size) were log-transformed to rescale all predictors to a similar range of values. Due to the limited sample size (i.e., 14 tributaries) and the high number of possible predictors (see Supplementary Tables S3, S5), the appropriate predictors were selected for each species in a stepwise process. Starting with a maximal model using only abiotic predictors, all non-significant predictors were removed using backward elimination before the biotic predictors were added to the reduced model and then all non-significant biotic predictors from the model were removed using backward elimination again. Partial regression plots were constructed for these final taxon-specific models.
Water chemistry readings were somewhat variable between tributaries, but generally consistent within lakes (see Supplementary Table S5). Overall, dissolved oxygen ranged from 7.6 to 11.5 mg L−1, pH ranged from 5.1 to 8.1, and specific conductivity ranged from 26 to 285 μS cm−1. In comparison, the habitat measures (i.e., canopy, macrophyte, and bank vegetation cover) exhibited some lake-scale trends but were more variable than water chemistry (see Supplementary Table S5). Overall, canopy and macrophyte coverage ranged from no cover up to ~70%, and bank vegetation and fine sediment (silt) cover ranged from no cover to ~100%. The average substrate size was largest in the tributaries of Māhinapua but ranged from < 10 to >150 mm. The dry-weight biomass of the common invertebrates (i.e., Chironomidae larvae, Deleatidium nymphs, and assorted Crustacea) ranged from 61.4 to 142.7 mg m−2 and did not change significantly with habitat complexity, F2, 9.22 = 0.65, p = 0.547; eel density, F1, 12 = 1.96, p = 0.187; trout density, F1, 12 = 2.48, p = 0.141; or perch density, F1, 11.32 = 0.03, p = 0.871.
Overall, tributaries with low habitat complexity were characterized by steeper banks, more homogenous flows, low canopy cover, and the lack of instream structures. In contrast, tributaries with high habitat complexity were characterized by low-angle banks covered with trees or overhanging vegetation and variability in flow and instream microhabitats. Differences in habitat complexity were not tied to any specific category (e.g., stream shading), although stream shading and instream features played smaller roles in the overall grading (cf. bank stability, habitat heterogeneity, and flow variability; see Supplementary Figure S2).
Assessments of the whole-community distribution of isotopic signatures using the metrics proposed by Layman et al. (2007) indicated that only two metrics, the total hull area and the mean distance from centroid, differed significantly along one or more environmental gradients (Table 1). Specifically, the total hull area was significantly lower at low habitat complexity [mean 21.6, 95% CI (16.5, 28.2)], compared to medium [mean 39.0, 95% CI (29.3, 51.9)] and high complexity [mean 44.8, 95% CI (29.9, 67.1)], and increased with eel density [mean slope 1.59, 95% CI (1.34, 1.90)]. Similarly, the mean distance to the centroid was significantly lower with low habitat complexity [mean 2.56, 95% CI (2.10, 3.10)], compared to medium [mean 3.98, 95% CI (3.24, 4.89)] and high complexity [mean 4.45, 95% CI (3.32, 5.95)], and increased with eel density [mean slope 1.33, 95% CI (1.17, 1.51)]. Trout density and perch CPUE did not affect any of the six metrics.
Table 1. Significance results for the change in the whole-community metrics proposed by Layman et al. (2007) along each environmental gradient.
Plots of the isotopic signatures of the surveyed communities across the three lakes highlighted clear differences in baseline signatures, with isotopic signatures seemingly changing with body size and species identity (Figure 1). For the three prey species, changes in dietary proportions with increasing body size differed between species, F2, 105.4 = 3.12, p = 0.048. Smelt and bullies did not change their estimated diet proportions with body size, but inanga reduced their lagoon-derived dietary proportions with increasing body size (Figure 2A). For the three predatory species, size-related dietary shifts between freshwater and lagoon resources depended on the species, F2, 156.2 = 5.52, p = 0.005. Between the three predators, the proportion of lagoon diet was variable and unrelated to body size in trout but significantly increased with body size for eels and perch (Figure 2B). For perch and trout, these modeled shifts in dietary proportions were consistent with changes in stomach contents (see Supplementary Table S6).
Figure 1. Isotopic biplots of overall fish communities found in the tributaries of (A) Te Waihora, (B) Māhinapua, and (C) Wairewa. All fish were separated into common predatory species (blue hues), common prey species (green hues) and species found in only one lake catchment (red hues), with species/taxa distinguished by shape. Point size reflects the total length of individual fish. Invertebrates collected from freshwater (open triangle) and lagoon (open square) environments are depicted as mean ± standard deviation.
Figure 2. Changes in diet proportions with increasing body size for (A) prey fish and (B) predatory fish. Each point represents an individual fish. Model fits (solid line) and 95% confidence intervals (shaded area) for each species were extracted from the respective linear mixed-effect model with “tributary” included as the random effect.
After accounting for the size-dependent changes in isotopes, differences remained between prey fish for δ15N and δ13C values, pseudo-F2, 127 = 4.97, p = 0.006 (Figure 3A), driven by the separation between bullies and smelt, pseudo-F1, 71 = 6.78, p = 0.021, as inanga overlapped with bullies, pseudo-F1, 114 = 5.14, p = 0.084, and smelt, pseudo-F1, 69 = 2.02, p = 0.408. There were also differences in δ15N and δ13C values between the three predatory species, pseudo-F2, 170 = 14.67, p = 001 (Figure 3B), driven by the separation of eels from perch, pseudo-F1, 121 = 27.21, p = 0.003, and trout, pseudo-F1, 132 = 14.86, p = 0.003, with an isotopic overlap between trout and perch, pseudo-F1, 88 = 2.62, p = 0.291.
Figure 3. Isotopic biplots for the size-corrected isotopic signatures for (A) three prey fish and (B) three predatory fish standardized to the local freshwater prey signature. Each point represents the model prediction taken from a linear mixed-effect model that included species identity and relative size, with “tributary” added as a random effect. Ellipses denote 90% confidence intervals for each species. Species identity is shown by point shape and color. Lowercase letters denote PERMANOVA significance results.
Regarding the taxon-specific isotope changes due to the local environment, eel signatures did not change with habitat complexity, pseudo-F2, 78 = 0.34, p = 0.793 (Figure 4A); eel density, pseudo-F1, 78 = 2.15, p = 0.143 (Figure 4B); perch density, pseudo-F1, 78 = 0.03, p = 0.976 (Figure 4C); or trout density, pseudo-F1, 78 = 0.52, p = 0.527 (Figure 4D). However, eel isotopic signatures were more variable at low eel densities (cf. medium and high densities), pseudo-F2, 81 = 3.91, p = 0.024, but did not change with habitat complexity, pseudo-F2, 81 = 0.77, p = 0.465, and numbers of trout, pseudo-F2, 81 = 0.55, p = 0.581, or perch, pseudo-F2, 81 = 1.11, p = 0.335. Inanga isotopic signatures were associated with densities of eels, pseudo-F1, 51 = 5.94, p = 0.006 (Figure 4F), and perch, pseudo-F1, 51 = 4.83, p = 0.011 (Figure 4G). Specifically, greater eel numbers was associated with elevated carbon and reduced nitrogen values, whereas more perch was only associated with elevated nitrogen values (no change for carbon). Inanga isotopic signatures were not associated with habitat complexity, pseudo-F2, 51 = 0.91, p = 0.446 (Figure 4E) or trout density, pseudo-F1, 51 = 2.04, p = 0.153 (Figure 4H). Variability in inanga isotope values was not associated with habitat complexity, pseudo-F2, 54 = 0.33, p = 0.723; eel density, pseudo-F1, 54 = 0.20, p = 0.820; perch density, pseudo-F1, 54 = 0.13, p = 0.876; or trout density, pseudo-F1, 78 = 1.11, p = 0.338. Next, the isotopic signatures of bullies were not associated with habitat complexity, pseudo-F2, 53 = 0.40, p = 0.777 (Figure 4I); eel density, pseudo-F1, 53 = 3.51, p = 0.054 (Figure 4J); perch density, pseudo-F1, 53 = 1.83, p = 0.140 (Figure 4K); or trout density, pseudo-F1, 53 = 1.22, p = 0.277 (Figure 4L). However, the variability of bully isotopes was lower with low or medium habitat complexity (cf. high complexity), pseudo-F2, 56 = 6.51, p = 0.003; low eel numbers (cf. medium and high densities), pseudo-F2, 56 = 4.53, p = 0.015; and when any perch were present (cf. no perch), pseudo-F2, 56 = 3.23, p = 0.047. Trout density was not associated with the isotopic variability of bullies, pseudo-F2, 56 = 0.19, p = 0.831. The isotopic signatures of perch were associated with different levels of habitat complexity, pseudo-F2, 33 = 7.04, p = 0.001 (Figure 4M); densities of eels, pseudo-F2, 33 = 11.60, p = 0.001 (Figure 4N); perch CPUE, pseudo-F2, 33 = 8.65, p = 0.002 (Figure 4O); and trout density, pseudo-F2, 33 = 6.20, p = 0.004 (Figure 4P). Specifically, lower habitat complexity was associated with lower carbon and nitrogen values, and greater eel abundance was associated with elevated carbon and reduced nitrogen values. Greater trout and perch abundance were not associated with carbon values but were associated with elevated or reduced nitrogen values, respectively. Finally, isotopic signatures of trout were not associated with habitat complexity, pseudo-F2, 49 = 0.35, p = 0.764 (Figure 4Q); eel density, pseudo-F1, 49 = 1.67, p = 0.197 (Figure 4R); perch CPUE, pseudo-F1, 49 = 0.01, p = 0.985 (Figure 4S); or trout density, pseudo-F1, 49 = 2.16, p = 0.147 (Figure 4T). However, the variability of trout signatures was lowest with low or medium habitat complexity (cf. high complexity), pseudo-F2, 47 = 4.05, p = 0.024, and high eel densities (cf. low and medium densities), pseudo-F2, 47 = 6.44, p = 0.003. The isotopic variability of trout did not change with trout densities, pseudo-F2, 47 = 0.15, p = 0.698, or perch densities, pseudo-F2, 47 = 1.83, p = 0.171.
Figure 4. Independent effects of habitat complexity (green) and abundance of three different predatory fish (blue) on the isotopic signatures (δ15N and δ13C) of (A–D) eels, (E–H) īinanga, (I–L) bullies, (M–P) perch, and (Q–T) trout. Individual predictors are organized by columns, and taxon-specific responses are organized by row. Each point reflects the size-adjusted partial model fits taken from a linear mixed effect model that included all four environmental gradients as predictors, with “tributary” included as a random effect. Panels with significant changes in isotopic signatures (i.e., permutational multivariate analysis of variance) are marked with an asterisk (*), and panels with significant changes in isotopic variability (i.e., permutational dispersion tests) are marked with a diamond (♢). For habitat complexity, a lighter color reflects a lower level of complexity (more information available in Supplementary Tables S1, S2). For the three predatory fish, point size reflects local densities for eels and trout (fish per m2), and relative abundance for perch (fish per fyke net).
When inferring body condition, the weight of large eels (>150 mm long) was not associated with habitat complexity, F2, 158.22 = 0.41, p = 0.661 (Figure 5A), but was affected by the interactions of length and eel density, F2, 186.38 = 3.46, p = 0.033 (Figure 5B); length and perch density, F2, 187.69 = 3.36, p = 0.037 (Figure 5C); and length and trout density, F2, 187.04 = 4.92, p = 0.008 (Figure 5D). Specifically, weight gains with increasing body length were greatest under high densities of eels and perch and either high densities or an absence of trout. Elvers, however, responded differently to these same environmental gradients. Although there was no interaction with the main effect of length, F1, 79.70 = 471.22, p < 0.001, elvers had greater weights, at any given length, with a medium or high habitat complexity (cf. low habitat complexity), F2, 68.41 = 12.10, p < 0.001 (Figure 5E); low numbers of eels (cf. medium and high densities), F2, 62.04 = 10.26, p < 0.001 (Figure 5F); and no perch (cf. high densities), F2, 65.12 = 3.21, p = 0.046 (Figure 5G). Elver weights were not associated with trout densities, F2, 61.97 = 0.50, p = 0.608 (Figure 5H). Inanga weights were affected by the interactions between length and habitat complexity, F2, 144.05 = 40.05, p < 0.001 (Figure 5I), and length and trout density, F2, 135.21 = 8.19, p < 0.001 (Figure 5L), as well as the main effect of perch density, F2, 52.73 = 4.45, p = 0.004 (Figure 5K). Eel density did not impact inanga weights, F2, 42.73 = 0.14, p = 0.872 (Figure 5J). Bullies were affected in a similar pattern to inanga, with body weights affected by the interactions of length and habitat complexity, F2, 772.29 = 43.41, p < 0.001 (Figure 5M); length and perch density, F2, 772.14 = 18.06, p < 0.001 (Figure 5O); and length and trout density, F2, 772.26 = 14.40, p < 0.001 (Figure 5P), although eel density were not associated, F2, 725.36 = 1.41, p = 0.246 (Figure 5N). Specifically, for bullies, weight gains with increasing body length were the greatest under high or low habitat complexity (cf. medium habitat complexity), high densities of trout (cf. low densities or absence of trout), and the presence of perch at any densities (cf. absence of perch). Unlike all other fish, perch weights were not associated with habitat complexity, F2, 123.50 = 1.28, p = 0.438 (Figure 5Q), and the densities of eels, F2, 123.45 = 1.13, p = 0.469 (Figure 5R); perch, F1, 123.18 = 0.17, p = 0.710 (Figure 5S); and trout, F2, 123.49 = 0.77, p = 0.566 (Figure 5T), with their weights solely determined by body length, F2, 123.50 = 5122.83, p < 0.001). In contrast to perch, trout weights were affected by interactions between length and habitat complexity, F2, 62.06 = 3.91, p = 0.025 (Figure 5U); length and eel densities, F2, 62.49 = 16.45, p < 0.001 (Figure 5V); length and perch densities, F2, 62.52 = 22.73, p < 0.001 (Figure 5W); and length and trout densities, F1, 62.10 = 24.02, p < 0.001 (Figure 5X). Specifically, for trout, weight gains with increasing body length were greatest under medium densities of eels (cf. high and low densities of eels), high densities or an absence of perch (cf. low densities of perch), and low densities of trout (cf. high densities of trout). However, across all levels of habitat complexity, smaller trout had similar weights, but larger trout were heavier with decreasing levels of complexity.
Figure 5. Length-weight regressions reflecting the independent effects of habitat complexity (green) and abundance of three different predatory fish (blue) on (A–D) large eels, (E–H) elvers, (I–L) inanga, (M–P) bullies, (Q–T) perch, and (U–X) trout. Each point reflects the partial fits taken from a linear mixed-effect model that included all four environmental gradients alongside relative body size as predictors, with “tributary” included as a random effect. Partial model fits (lines) and 95% confidence intervals (CI; shaded areas) were also extracted from this global model. Note that in models where the environmental gradient was not significant, we present the underlying change with body length (gray area for 95% CI). For habitat complexity, color reflects the ascribed level of complexity (more information available in Supplementary Tables S1, S2). For the predatory fish, color reflects categories for densities of eels, trout, and perch (more information available in Supplementary Table S3). Note that the axes are presented on a logarithmic scale.
For shifts in the abundance of individual fish groups, inanga abundance was negatively associated with increasing bank cover, F1, 9 = 8.50, p = 0.017 (Figure 6A); canopy cover, F1, 9 = 21.78, p = 0.001 (Figure 6A); and trout abundance, F1, 9 = 71.82, p < 0.001 (Figure 6B), but positively associated with eel density, F1, 9 = 40.78, p < 0.001 (Figure 6B). Interestingly, the abundance of the other common prey species, bullies, was not associated with any predictor, although the random effect ‘lake' explained 91.6% of the variation in abundance. Among the predator taxa, eels were more abundant with increased canopy cover, F1, 9 = 19.68, p < 0.001 (Figure 6C), and greater numbers of inanga, F1, 9 = 13.17, p = 0.005 (Figure 6D); bullies, F1, 9 = 22.67, p < 0.001 (Figure 6D); and trout, F1, 9 = 48.37, p < 0.001 (Figure 6D). Trout densities were negatively associated with increasing bank cover, F1, 9 = 9.67, p = 0.013 (Figure 6E); canopy cover, F1, 9 = 35.85, p < 0.001 (Figure 6E); and inanga abundance, F1, 9 = 71.82, p < 0.001 (Figure 6F), but positively associated with eel abundance, F1, 9 = 88.26, p < 0.001 (Figure 6F). Like bullies, perch CPUE was not associated with any predictors, although the random effect of “lake” explained very little (10.4%) of the variation in abundance.
Figure 6. Partial regression plots for density changes in (A, B) inanga, (C, D) eels, and (E, F) brown trout in response to habitat shifts and altered abundances of co-occurring species. Each point reflects the partial model fits taken from a linear mixed-effect model that included only significant environmental gradients and fish densities as predictors, with “lake” included as a random effect. Partial model fits (lines) and 95% confidence intervals (CI; shaded areas) were also extracted from this global model. Note that bullies and perch did not respond to any predictors, so are not shown here, and that the axes are presented on a logarithmic scale.
When managing vulnerable naturally depauperate communities, implementing effective conservation strategies is necessary to mitigate the multi-faceted impacts of global change. However, attributing causality between different potential stressors is required before interventions that maximize the return on investment are designed. Here, we show how the freshwater fish communities of New Zealand found in coastal lakes have responded to the simultaneous effects of habitat degradation and two introduced predatory species, redfin perch and brown trout. Overall, the introduced species did not add to the trophic diversity of the community; instead, they occupied isotopic space that native fish would otherwise have likely used. Only the largest eels benefitted from these introduced fish, although trout and perch were using the productive lagoon environment at earlier life stages than the native eels. Trout were synonymous with the largest impact on any one species, a decline in inanga in association with increasing trout density, although perch and habitat degradation were associated with similar effects on the body conditions across the smaller bodied fish. Suppressing or controlling these introduced fish would have considerable benefits for native fish, but because their use of riverine and lagoon habitats and resources is extensive, efforts to limit their numbers would be problematic. We conclude that an overarching focus on improving habitat complexity in these lowland environments is likely to produce the biggest response in the native fish community for the investments made (i.e., time and money). However, working in catchments with already low numbers of introduced fish would further increase the potential benefits for native fish.
Within a naturally depauperate community, low diversity is assumed to leave niche spaces open for introduced species to occupy and establish new populations (Angermeier and Winston, 1998; Gido and Brown, 1999). Consequently, these new species would alter the trophic diversity of the recipient community. We found the opposite, however, with the overall trophic diversity of the community unchanged with increasing abundance of perch or trout because both species had isotopic signatures within the already occupied range. This consistency in trophic diversity implies that these introduced species were replacing native species within these depauperate communities. However, the isotopic overlap was not with the larger growing eels (i.e., the top native predator) because perch and trout are feeding at a lower trophic level than eels when dietary proportions overlapped (i.e., at similar δ13C signatures). Moreover, introduced fish seemed to avoid direct competition with eels by consuming lagoon-derived resources at earlier life stages. Therefore, we suggest that the introduced species, particularly the larger size classes of perch, are effectively monopolizing the resources provided by the more productive lagoon environment, but their interactions with eels may lessen their impacts on other riverine fish.
Across all resident fish, only the native top predator and largest growing species, the larger competitively dominant eels, generally benefitted from greater numbers of all other fish. Underpinning these positive responses is their generalist, opportunistic diet (see Jellyman, 1989), whereby larger eels consume a range of prey, including smaller perch and trout (see Jellyman, 1996; Stewart et al., 2023). As we predicted, the negative interactions from increasing eel numbers were mostly with the introduced species, such as the reduced body conditions of trout and the increasingly lagoon-supported diets of perch. In fact, the only negative impact that eels had on the native fish was intra-specific competition with smaller conspecifics, which is likely a density-dependent population regulation (see Noth et al., 2008). The presence of larger eels does trigger innate predator avoidance behavior in potential prey species, including elvers, bullies, and inanga (see Glova, 2001; McLean et al., 2007; Jellyman et al., 2012). However, for these smaller fish, similar anti-predator responses are typically only observed in introduced fish once they have been learned through experience (see Kristensen and Closs, 2004; McLean et al., 2007; Milano et al., 2010). Therefore, these innate behaviors, or a lack of them, in response to predator detection likely underpin the negative impacts, such as reduced abundance or body condition, associated with increasing predator abundance.
Small fish were negatively associated with greater perch densities, although the exact mechanisms differed slightly between species. Consistent with previous work, riverine fish featured most heavily in the diets of mid-size perch (10–20 cm long), with larger perch (>20 cm long) feeding exclusively within lakes or lagoons (see Ludgate and Closs, 2003; Morgan et al., 2003; Wedderburn and Barnes, 2016). In terms of specific-specific responses to greater perch abundance, inanga exhibited reduced body condition and elevated nitrogen signatures, which we suggest could reflect reduced foraging activity and, potentially, periods of starvation when faced with predation risk from perch (see Casini et al., 2016; Doi et al., 2017). Under these same conditions (i.e., high perch numbers), bullies exhibited a reduction in diet variability, with fewer individuals feeding on lagoon-derived resources, although the impact on overall body condition was minimal. Therefore, perch were also excluding smaller fish from the productive lagoon environments, and the removal of perch would benefit most, if not all, native species because of their ubiquitous predation of all smaller fish.
In contrast to size-mediated impacts from perch, which had similar effects across multiple species, impacts of trout were more variable. First, trout exhibited the single largest effect that we detected, with near-complete removal of inanga in the presence of trout, a pattern consistent across many species of galaxiid (see McDowall, 2006; McIntosh et al., 2010). However, bullies were unaffected by trout, and only the largest eels exhibited a loss of body condition with increased trout abundance, a potential indication of increased competitive interactions (see Irons et al., 2007; Smith et al., 2017). In fact, the competitive interactions between trout and eels, in which trout are also suppressed, likely underly the positive relationship between densities of eels and inanga.
Perch and trout are recognized internationally for their invasiveness and impacts on recipient communities (see Morgan et al., 2003; McDowall, 2006), but these predatory fish responded very differently to environmental change. Perch exhibited clear dietary shifts, with increasing body size and along measured gradients of environmental change. These dietary shifts likely underpin their consistent body condition (i.e., consistent length–weight regressions) regardless of the local environmental conditions. In contrast, trout exhibited highly variable diets that did not change with body size and most environmental changes, aside from a reduction in diet variability in response to high eel numbers and reduced habitat complexity. Although trout did not have consistent body conditions like perch, their body conditions notably increased with greater numbers of perch and trout and reduced habitat complexity. In addition to benefitting from environmental degradation and introduced species, perch and trout were effective at using the riverine and lagoon environments for feeding and development, which makes efforts to control or reduce their numbers problematic.
Previous attempts to remove or suppress introduced trout or perch highlight the need for repeated treatments across large spatial scales (Peterson et al., 2008). For example, Shepard et al. (2014) used as many as 14 rounds of electrofishing removal treatments to successfully eradicate introduced brook trout from four mountain streams in Montana, USA, at a cost of between US$3,500 to US$9,000 per kilometer depending on the of the need for additional vegetation clearing. Despite these investments, the chances of failure remain high, and reinvasion is always possible (see Ludgate and Closs, 2003; Caudron and Champigneulle, 2011). Therefore, unless working in catchments (e.g., tributaries or isolated streams) where introduced species are already low in abundance, reducing their numbers to sufficient levels to benefit native species would be a large undertaking in time and money.
An alternative pathway for conservation lies in the loss of habitat complexity due to widespread environmental degradation. Like perch, the loss of habitat complexity caused consistent impacts on all smaller fish. Lower habitat complexity led to poorer body conditions for small-bodied fish, an increased proportion of river-derived resources in perch diets, and better body conditions in trout. We conclude that these results derive from the predative interactions between the introduced fish and the native prey, particularly because the biomass of common invertebrates did not change. In homogeneous, less complex environments, prey species would be expected to expend more energy in predator avoidance, and predators would expend less energy in the search for prey (see Claireaux and Lefrançois, 2007; Tamburello et al., 2015). Therefore, restoring habitat complexity could be the most cost-efficient way to benefit most, if not all, native fish by mitigating the impacts from introduced species while minimizing the realized benefits to those introduced species.
In summary, our results show how a combination of dietary shifts, and competitive and predatory interactions underpin the changes in depauperate riverine fish communities when simultaneously exposed to multiple environmental stressors. Although the introduced fish minimized direct competition with the native top predators, neither trout nor perch increased overall trophic diversity due to their suppression of the smaller fish. The eradication or ongoing suppression of introduced fish such as trout can quickly become a costly endeavor. However, we have shown how increased habitat complexity provides a more efficient way to benefit native species. Although the total benefits will be smaller than direct control of the introduced fish, improvements to habitat complexity (e.g., increased flow heterogeneity or canopy cover, instream additions, or bank reforming) could lead to increasing benefits when applied at larger scales (see Jansson et al., 2007; Miller et al., 2010; Stoffers et al., 2022). Furthermore, the need for ongoing maintenance with habitat modifications would be diminished, if not removed, in a relatively short time (see Iversen et al., 1993; Saunders et al., 2002). Focusing on habitat restoration instead of directly working on controlling introduced species, therefore, provides a scope to work at larger scales, with multiple benefits across all native species while reducing ongoing costs and increasing the likelihood of successful outcomes.
The original contributions presented in the study are included in the article/Supplementary material, further inquiries can be directed to the corresponding author.
All sampling procedures were approved by the University of Canterbury Animal Ethics Committee (permit number 2021/19R), with sampling involving sports fish approved by North Canterbury Fish and Game (permit number 22-1). The study was conducted in accordance with the local legislation and institutional requirements.
CM: Conceptualization, Formal analysis, Investigation, Methodology, Writing – original draft, Writing – review & editing. MH: Conceptualization, Investigation, Methodology, Supervision, Writing – review & editing. DG: Conceptualization, Supervision, Writing – review & editing. DS: Conceptualization, Supervision, Writing – review & editing.
The author(s) declare that financial support was received for the research, authorship, and/or publication of this article. CM was supported by scholarship jointly funded by University of Canterbury and Environment Canterbury. DS was financially supported by the NZ Ministry of Business, Innovation and Employment (C01X1615; https://app.dimensions.ai/details/grant/grant.7565396). The funders had no role in study design, data collection and analysis, decision to publish, or preparation of the manuscript.
We are grateful to Ben Crichton, Nixie Boddy, Channell Thoms, Evie Garrod, Saskia Brown, Rory Lennox, Ignacio R. Sainz, and Zoe Hamilton for extensive field assistance; Jan McKenzie for laboratory assistance; the Marine Ecology Research Group for discussion and feedback on this manuscript; and Sefeti Erasito (Taumutu Rūnanga), Miriam Clark (Taumutu Rūnanga) and the Mana Moana Environmental Pou (Wairewa Rūnanga) for advice and support regarding indigenous knowledge. We would also like to thank Environment Canterbury and the University of Canterbury for financial and logistical support.
The authors declare that the research was conducted in the absence of any commercial or financial relationships that could be construed as a potential conflict of interest.
All claims expressed in this article are solely those of the authors and do not necessarily represent those of their affiliated organizations, or those of the publisher, the editors and the reviewers. Any product that may be evaluated in this article, or claim that may be made by its manufacturer, is not guaranteed or endorsed by the publisher.
The Supplementary Material for this article can be found online at: https://www.frontiersin.org/articles/10.3389/ffwsc.2024.1398975/full#supplementary-material
Aarts, B. G., Van Den Brink, F. W., and Nienhuis, P. H. (2004). Habitat loss as the main cause of the slow recovery of fish faunas of regulated large rivers in Europe: the transversal floodplain gradient. River Res. Appl. 20, 3–23. doi: 10.1002/rra.720
Angermeier, P. L., and Winston, M. R. (1998). Local vs. regional influences on local diversity in stream fish communities of Virginia. Ecology 79, 911–927. doi: 10.1890/0012-9658(1998)079[0911:LVRIOL]2.0.CO;2
Baltz, D. M., and Moyle, P. B. (1993). Invasion resistance to introduced species by a native assemblage of California stream fishes. Ecol. Appl. 3, 246–255. doi: 10.2307/1941827
Bates, D., Mächler, M., Bolker, B., and Walker, S. (2015). Fitting linear mixed-effects models using lme4. J. Stat. Softw. 67, 1–48. doi: 10.18637/jss.v067.i01
Boddy, N. C., and McIntosh, A. R. (2017). Temperature, invaders and patchy habitat interact to limit the distribution of a vulnerable freshwater fish. Austral Ecol. 42, 456–167. doi: 10.1111/aec.12463
Brand, J. A., Martin, J. M., Tan, H., Mason, R. T., Orford, J. T., Hammer, M. P., et al. (2021). Rapid shifts in behavioural traits during a recent fish invasion. Behav. Ecol. Sociobiol. 75:134. doi: 10.1007/s00265-021-03077-2
Cade, B. S., Terrell, J. W., and Porath, M. T. (2008). Estimating fish body condition with quantile regression. North Am. J. Fish. Manag. 28, 349–359. doi: 10.1577/M07-048.1
Cantin, A., and Post, J. R. (2018). Habitat availability and ontogenetic shifts alter bottlenecks in size-structured fish populations. Ecology 99, 1644–1659. doi: 10.1002/ecy.2371
Carpenter, S. R., Fisher, S. G., Grimm, N. B., and Kitchell, J. F. (1992). Global change and freshwater ecosystems. Annu. Rev. Ecol. Syst. 23, 119–139. doi: 10.1146/annurev.es.23.110192.001003
Casini, M., Käll, F., Hansson, M., Plikshs, M., Baranova, T., Karlsson, O., et al. (2016). Hypoxic areas, density-dependence and food limitation drive the body condition of a heavily exploited marine fish predator. R. Soc. Open Sci. 3:160416. doi: 10.1098/rsos.160416
Caudron, A., and Champigneulle, A. (2011). Multiple electrofishing as a mitigate tool for removing nonnative Atlantic brown trout (Salmo trutta L.) threatening a native Mediterranean brown trout population. Eur. J. Wildl. Res. 57, 575–583. doi: 10.1007/s10344-010-0468-8
Chessman, B. C. (2013). Identifying species at risk from climate change: traits predict the drought vulnerability of freshwater fishes. Biol. Conserv. 160, 40–49. doi: 10.1016/j.biocon.2012.12.032
Claireaux, G., and Lefrançois, C. (2007). Linking environmental variability and fish performance: integration through the concept of scope for activity. Philos. Trans. R. Soc. B Biol. Sci. 362, 2031–2041. doi: 10.1098/rstb.2007.2099
Comte, L., and Olden, J. D. (2017). Climatic vulnerability of the world's freshwater and marine fishes. Nat. Clim. Change 7, 718–722. doi: 10.1038/nclimate3382
Correa, C., Bravo, A. P., and Hendry, A. P. (2012). Reciprocal trophic niche shifts in native and invasive fish: salmonids and galaxiids in Patagonian lakes. Freshw. Biol. 57, 1769–1781. doi: 10.1111/j.1365-2427.2012.02837.x
Cucherousset, J., and Olden, J. D. (2011). Ecological impacts of nonnative freshwater fishes. Fisheries 36, 215–230. doi: 10.1080/03632415.2011.574578
da Silva Gonçalves, C., De Souza Braga, F. M., and Casatti, L. (2018). Trophic structure of coastal freshwater stream fishes from an Atlantic rainforest: evidence of the importance of protected and forest-covered areas to fish diet. Environ. Biol. Fishes 101, 933–948. doi: 10.1007/s10641-018-0749-8
Dixon, P. (2003). VEGAN, a package of R functions for community ecology. J. Veg. Sci. 14, 927–930. doi: 10.1111/j.1654-1103.2003.tb02228.x
Dobson, A., Lodge, D., Alder, J., Cumming, G. S., Keymer, J., Mcglade, J., et al. (2006). Habitat loss, trophic collapse, and the decline of ecosystem services. Ecology 87, 1915–1924. doi: 10.1890/0012-9658(2006)87[1915:HLTCAT]2.0.CO;2
Doi, H., Akamatsu, F., and González, A. L. (2017). Starvation effects on nitrogen and carbon stable isotopes of animals: an insight from meta-analysis of fasting experiments. R. Soc. Open Sci. 4:170633. doi: 10.1098/rsos.170633
Dudgeon, D., Arthington, A. H., Gessner, M. O., Kawabata, Z.-I., Knowler, D. J., Lévêque, C., et al. (2006). Freshwater biodiversity: importance, threats, status and conservation challenges. Biol. Rev. 81, 163–182. doi: 10.1017/S1464793105006950
Fausch, K. D., Lyons, J., Karr, J. R., and Angermeier, P. L. (1990). “Fish communities as indicators of environmental degradation,” in American fisheries society symposium (Bethesda), 123–144.
Font, W. F., and Tate, D. C. (1994). Helminth parasites of native Hawaiian freshwater fishes: an example of extreme ecological isolation. J. Parasitol. 80, 682–688. doi: 10.2307/3283246
Fox, J., and Weisberg, S. (2018). Visualizing fit and lack of fit in complex regression models with predictor effect plots and partial residuals. J. Stat. Softw. 87, 1–27. doi: 10.18637/jss.v087.i09
Galbraith, L. M., and Burns, C. W. (2007). Linking land-use, water body type and water quality in southern New Zealand. Landsc. Ecol. 22, 231–241. doi: 10.1007/s10980-006-9018-x
Gallardo, B., Clavero, M., Sánchez, M. I., and Vilà, M. (2016). Global ecological impacts of invasive species in aquatic ecosystems. Glob. Chang. Biol. 22, 151–163. doi: 10.1111/gcb.13004
Gehrke, P. C., and Harris, J. H. (2000). Large-scale patterns in species richness and composition of temperate riverine fish communities, south-eastern Australia. Mar. Freshw. Res. 51, 165–182. doi: 10.1071/MF99061
Geist, J., and Hawkins, S. J. (2016). Habitat recovery and restoration in aquatic ecosystems: current progress and future challenges. Aquat. Conserv.: Mar. Freshw. Ecosyst. 26, 942–962. doi: 10.1002/aqc.2702
Gido, K. B., and Brown, J. H. (1999). Invasion of North American drainages by alien fish species. Freshw. Biol. 42, 387–399. doi: 10.1046/j.1365-2427.1999.444490.x
Glova, G. J. (2001). Effects of the presence of subadult longfinned eels (Anguilla dieffenbachii) on cover preferences of juvenile eels (Anguilla spp.) in replicate channels. N. Z. J. Mar. Freshw. Res. 35, 221–233. doi: 10.1080/00288330.2001.9516993
Henderson, A., and Johnston, C. (2010). Ontogenetic habitat shifts and habitat use in an endangered minnow, Notropis mekistocholas. Ecol. Freshw. Fish 19, 87–95. doi: 10.1111/j.1600-0633.2009.00392.x
Hicks, B. J., Smith, D. R., Pingram, M. A., Kelly, D. J., and Fraley, K. M. (2022). Conservation of freshwater eels in food-web studies: non-lethal stable isotope analyses substitute fin for muscle tissue with lipid correction. Ecol. Freshw. Fish 31, 515–528. doi: 10.1111/eff.12647
Irons, K. S., Sass, G. G., Mcclelland, M. A., and Stafford, J. D. (2007). Reduced condition factor of two native fish species coincident with invasion of non-native Asian carps in the Illinois River, U.S.A. Is this evidence for competition and reduced fitness? J. Fish Biol. 71, 258–273. doi: 10.1111/j.1095-8649.2007.01670.x
Iversen, T. M., Kronvang, B., Madsen, B. L., Markmann, P., and Nielsen, M. B. (1993). Re-establishment of Danish streams: restoration and maintenance measures. Aquat. Conserv.: Mar. Freshw. Ecosyst. 3, 73–92. doi: 10.1002/aqc.3270030203
Jackson, A. L., Inger, R., Parnell, A. C., and Bearhop, S. (2011). Comparing isotopic niche widths among and within communities: SIBER–Stable Isotope Bayesian Ellipses in R. J. Anim. Ecol. 80, 595–602. doi: 10.1111/j.1365-2656.2011.01806.x
Jackson, M., Wasserman, R., Grey, J., Ricciardi, A., Dick, J. T., Alexander, M., et al. (2017). “Novel and disrupted trophic links following invasion in freshwater ecosystems,” in Advances in Ecological Research, eds. D. A. Bohan, A. J. Dumbrell, and F. Massol (Amsterdam: Elsevier), 55–97. doi: 10.1016/bs.aecr.2016.10.006
Jackson, M. C., Loewen, C. J., Vinebrooke, R. D., and Chimimba, C. T. (2016). Net effects of multiple stressors in freshwater ecosystems: a meta-analysis. Glob. Chang. Biol. 22, 180–189. doi: 10.1111/gcb.13028
Jansson, R., Nilsson, C., and Malmqvist, B. (2007). Restoring freshwater ecosystems in riverine landscapes: the roles of connectivity and recovery processes. Freshw. Biol. 52, 589–596. doi: 10.1111/j.1365-2427.2007.01737.x
Jellyman, D. J. (1989). Diet of two species of freshwater eel (Anguilla spp.) in Lake Pounui, New Zealand. N. Z. J. Mar. Freshw. Res. 23, 1–10. doi: 10.1080/00288330.1989.9516334
Jellyman, D. J. (1996). Diet of longfinned eels, Anguilla dieffenbachii, in Lake Rotoiti, Nelson Lakes, New Zealand. N. Z. J. Mar. Freshw. Res. 30, 365–369. doi: 10.1080/00288330.1996.9516723
Jellyman, D. J., Crow, S. K., and Robinson, P. (2012). Impacts of longfin eels (Anguilla dieffenbachii) on the behaviour of common bullies (Gobiomorphus cotidianus) held in captivity. Ecol. Freshw. Fish 21, 57–63. doi: 10.1111/j.1600-0633.2011.00523.x
Jowett, I. G., and Richardson, J. (1996). Distribution and abundance of freshwater fish in New Zealand rivers. N. Z. J. Mar. Freshw. Res. 30, 239–255. doi: 10.1080/00288330.1996.9516712
Julian, J. P., De Beurs, K. M., Owsley, B., Davies-Colley, R. J., and Ausseil, A.-G. E. (2017). River water quality changes in New Zealand over 26 years: response to land use intensity. Hydrol. Earth Syst. Sci. 21, 1149–1171. doi: 10.5194/hess-21-1149-2017
King, A. (2004). Ontogenetic patterns of habitat use by fishes within the main channel of an Australian floodplain river. J. Fish Biol. 65, 1582–1603. doi: 10.1111/j.0022-1112.2004.00567.x
Kopf, R. K., Shaw, C., and Humphries, P. (2017). Trait-based prediction of extinction risk of small-bodied freshwater fishes. Conserv. Biol. 31, 581–591. doi: 10.1111/cobi.12882
Kristensen, E. A., and Closs, G. P. (2004). Anti-predator response of naïve and experienced common bully to chemical alarm cues. J. Fish Biol. 64, 643–652. doi: 10.1111/j.1095-8649.2004.00328.x
Layman, C. A., Araujo, M. S., Boucek, R., Hammerschlag-Peyer, C. M., Harrison, E., Jud, Z. R., et al. (2012). Applying stable isotopes to examine food-web structure: an overview of analytical tools. Biol. Rev. 87, 545–562. doi: 10.1111/j.1469-185X.2011.00208.x
Layman, C. A., Arrington, D. A., Montaña, C. G., and Post, D. M. (2007). Can stable isotope ratios provide for community-wide measures of trophic structure? Ecology 88, 42–48. doi: 10.1890/0012-9658(2007)88[42:CSIRPF]2.0.CO;2
Levêque, C., Oberdorff, T., Paugy, D., Stiassny, M., and Tedesco, P. A. (2008). “Global diversity of fish (Pisces) in freshwater,” in Freshwater animal diversity assessment, eds. E.V. Balian, C. Lévêque, H. Segers, and K. Martens (Dordrecht: Springer), 545–567. doi: 10.1007/978-1-4020-8259-7_53
Ludgate, B., and Closs, G. (2003). Responses of fish communities to sustained removals of perch (Perca fluviatilis). Sci. Conserv. 210, 1–38.
Martin-Smith, K., and Laird, L. (1998). Depauperate freshwater fish communities in Sabah: the role of barriers to movement and habitat quality. J. Fish Biol. 53, 331–344. doi: 10.1111/j.1095-8649.1998.tb01035.x
McDowall, R. (2003). Hawaiian biogeography and the islands' freshwater fish fauna. J. Biogeogr. 30, 703–710. doi: 10.1046/j.1365-2699.2003.00851.x
McDowall, R. M. (2006). Crying wolf, crying foul, or crying shame: Alien salmonids and a biodiversity crisis in the southern cool-temperate galaxioid fishes? Rev. Fish Biol. Fish 16, 233–422. doi: 10.1007/s11160-006-9017-7
McIntosh, A. R., Mchugh, P. A., Dunn, N. R., Goodman, J. M., Howard, S. W., Jellyman, P. G., et al. (2010). The impact of trout on galaxiid fishes in New Zealand. N. Z. J. Ecol. 34, 195–206.
McLean, F., Barbee, N. C., and Swearer, S. E. (2007). Avoidance of native versus non-native predator odours by migrating whitebait and juveniles of the common galaxiid, Galaxias maculatus. N. Z. J. Mar. Freshw. Res. 41, 175–184. doi: 10.1080/00288330709509906
Meijer, C. G. (2024). Transitory use of lowland lakes in southern New Zealand by īnanga (Galaxias maculatus) and implications for management (Unpublished Ph.D thesis). University of Canterbury.
Meijer, C. G., Warburton, H. J., and McIntosh, A. R. (2021). Disentangling the multiple effects of stream drying and riparian canopy cover on the trophic ecology of a highly threatened fish. Freshw. Biol. 66, 102–113. doi: 10.1111/fwb.13620
Milano, D., Lozada, M., and Zagarese, H. E. (2010). Predator-induced reaction patterns of landlocked Galaxias maculatus to visual and chemical cues. Aquatic Ecol 44, 741–748. doi: 10.1007/s10452-010-9312-1
Miller, S. W., Budy, P., and Schmidt, J. C. (2010). Quantifying macroinvertebrate responses to in-stream habitat restoration: applications of meta-analysis to river restoration. Restor. Ecol. 18, 8–19. doi: 10.1111/j.1526-100X.2009.00605.x
Morgan, D. L., Hambleton, S. J., Gill, H. S., and Beatty, S. J. (2003). Distribution, biology and likely impacts of the introduced redfin perch (Perca fluviatilis) (Percidae) in Western Australia. Mar. Freshw. Res. 53, 1211–1221. doi: 10.1071/MF02047
Moyle, P. B., and Light, T. (1996). Fish invasions in California: do abiotic factors determine success? Ecology 77, 1666–1670. doi: 10.2307/2265770
Nannini, M., and Belk, M. C. (2006). Antipredator responses of two native stream fishes to an introduced predator: does similarity in morphology predict similarity in behavioural response? Ecol. Freshw. Fish 15, 453–463. doi: 10.1111/j.1600-0633.2006.00177.x
National Institute of Water and Atmospheric Research (2022). New Zealand Freshwater Fish Database [Online]. Available online at: https://niwa.co.nz/information-services/nz-freshwater-fish-database (accessed May 5, 2022).
Noth, E. G., Francis, R. I., and Jellyman, D. (2008). Factors influencing juvenile eel (Anguilla spp.) survival in lowland New Zealand streams. N. Z. J. Mar. Freshw. Res. 42, 153–172. doi: 10.1080/00288330809509945
Oele, D. L., Gaeta, J. W., Rypel, A. L., and Mcintyre, P. B. (2019). Growth and recruitment dynamics of young-of-year northern pike: implications for habitat conservation and management. Ecol. Freshw. Fish 28, 285–301. doi: 10.1111/eff.12453
Peterson, D. P., Fausch, K. D., Watmough, J., and Cunjak, R. A. (2008). When eradication is not an option: modeling strategies for electrofishing suppression of nonnative brook trout to foster persistence of sympatric native cutthroat trout in small streams. North Am. J. Fish. Manag. 28, 1847–1867. doi: 10.1577/M07-174.1
Posit Team (2022). RStudio: Integrated development environment for R. Boston, MA: Posit Software, Pbc.
Post, D. M., Layman, C. A., Arrington, D. A., Takimoto, G., Quattrochi, J., Montaña, C. G., et al. (2007). Getting to the fat of the matter: models, methods and assumptions for dealing with lipids in stable isotope analyses. Oecologia 152, 179–189. doi: 10.1007/s00442-006-0630-x
R Core Team (2022). R: A Language and Environment for Statistical Computing. Vienna, Austria: R Foundation for Statistical Computing.
Reid, A. J., Carlson, A. K., Creed, I. F., Eliason, E. J., Gell, P. A., Johnson, P. T., et al. (2019). Emerging threats and persistent conservation challenges for freshwater biodiversity. Biol. Rev. 94, 849–873. doi: 10.1111/brv.12480
Sabetian, A., Trip, E. D. L., Wheeler, P., Sands, L., Wakefield, S., Visconti, V., et al. (2015). Biological plasticity of non-native European perch (Perca fluviatilis) populations and the implications for management in northern New Zealand. N. Z. J. Mar. Freshw. Res. 49, 119–131. doi: 10.1080/00288330.2014.958089
Saunders, D. L., Meeuwig, J. J., and Vincent, A. C. (2002). Freshwater protected areas: strategies for conservation. Conserv. Biol. 16, 30–41. doi: 10.1046/j.1523-1739.2002.99562.x
Scheffer, M., Van Geest, G. J., Zimmer, K., Jeppesen, E., Søndergaard, M., Butler, M. G. L., et al. (2006). Small habitat size and isolation can promote species richness: second-order effects on biodiversity in shallow lakes and ponds. Oikos 112, 227–231. doi: 10.1111/j.0030-1299.2006.14145.x
Shepard, B. B., Nelson, L. M., Taper, M. L., and Zale, A. V. (2014). Factors influencing successful eradication of nonnative brook trout from four small rocky mountain streams using electrofishing. North Am. J. Fish. Manag. 34, 988–997. doi: 10.1080/02755947.2014.942042
Smith, A. D., Houde, A. L. S., Neff, B., and Peres-Neto, P. R. (2017). Effects of competition on fitness-related traits. Oecologia 183, 701–713. doi: 10.1007/s00442-017-3816-5
Sowersby, W., Thompson, R., and Wong, B. (2016). Invasive predator influences habitat preferences in a freshwater fish. Environ. Biol. Fishes 99, 187–193. doi: 10.1007/s10641-015-0466-5
Stefani, F., Schiavon, A., Tirozzi, P., Gomarasca, S., and Marziali, L. (2020). Functional response of fish communities in a multistressed freshwater world. Sci. Total Environ. 740:139902. doi: 10.1016/j.scitotenv.2020.139902
Stewart, C., Harper, B., Couper, J., Bury, S. J., and Sabadel, A. (2023). Can non-native perch (Perca fluviatilis) support native eel populations in a wetland complex? Ecol. Freshw. Fish 33, 1−15. doi: 10.1111/eff.12749
Stewart, S. D., Holmes, R., Vadeboncoeur, Y., Bury, S. J., and Crump, S. (2022). Sea to the mountains: quantifying freshwater eel and trout diet reliance on marine subsidies from upstream migrating fish. N. Z. J. Mar. Freshw. Res. 56, 466–490. doi: 10.1080/00288330.2022.2101482
Stoffers, T., Buijse, A., Geerling, G., Jans, L., Schoor, M., Poos, J., et al. (2022). Freshwater fish biodiversity restoration in floodplain rivers requires connectivity and habitat heterogeneity at multiple spatial scales. Sci. Total Environ. 838:156509. doi: 10.1016/j.scitotenv.2022.156509
Strayer, D. L. (2010). Alien species in fresh waters: ecological effects, interactions with other stressors, and prospects for the future. Freshw. Biol. 55, 152–174. doi: 10.1111/j.1365-2427.2009.02380.x
Strayer, D. L., and Dudgeon, D. (2010). Freshwater biodiversity conservation: recent progress and future challenges. J. North Am. Benthol. Soc. 29, 344–358. doi: 10.1899/08-171.1
Tamburello, N., Côté, I. M., and Dulvy, N. K. (2015). Energy and the scaling of animal space use. Am. Nat. 186, 196–211. doi: 10.1086/682070
van der Lee, A. S., and Koops, M. A. (2015). Are small fishes more sensitive to habitat loss? A generic size-based model. Can. J. Fish. Aquat. Sci. 73, 716−726. doi: 10.1139/cjfas-2015-0026
Vander Zanden, M. J., Casselman, J. M., and Rasmussen, J. B. (1999). Stable isotope evidence for the food web consequences of species invasions in lakes. Nature 401, 464–467. doi: 10.1038/46762
Wedderburn, S. D., and Barnes, T. C. (2016). Piscivory by alien redfin perch (Perca fluviatilis) begins earlier than anticipated in two contrasting habitats of Lake Alexandrina, South Australia. Aust. J. Zool. 64, 1–7. doi: 10.1071/ZO15083
Winterbourn, M. J., Gregson, K. L. D., and Dolphin, C. H. (2006). Guide to the Aquatic Insects of New Zealand, 4th Edn. Christchurch, NZ: Entomological Society of New Zealand.
Wolman, M. G. (1954). A method of sampling coarse river-bed material. EOS Trans. Am. Geophys. Union 35, 951–956. doi: 10.1029/TR035i006p00951
Young, R. G., Keeley, N. B., Shearer, K. A., and Crowe, A. L. (2004). Impacts of diquat herbicide and mechanical excavation on spring-fed drains in Marlborough, New Zealand. Sci. Conserv. 240, 1–36.
Zambrano, M., Bonifacio, A., Brito, J., Rautenberg, G., and Hued, A. (2023). Length–weight relationships and body condition indices of a South American bioindicator, the native neotropical fish species, Cnesterodon decemmaculatus (Poeciliidae). J. Ichthyol. 63, 930–936. doi: 10.1134/S0032945223050156
Keywords: New Zealand, conservation, multiple stressors, modified habitats, fresh water, lowland environments, introduced species
Citation: Meijer CG, Hickford MJH, Gray DP and Schiel DR (2024) Disentangling the simultaneous effects of habitat degradation and introduced species on naturally depauperate riverine fish communities. Front. Freshw. Sci. 2:1398975. doi: 10.3389/ffwsc.2024.1398975
Received: 11 March 2024; Accepted: 19 June 2024;
Published: 05 July 2024.
Edited by:
Carmen Montaña, Stephen F. Austin State University, United StatesReviewed by:
Gabriela Echevarría, University of the Americas, EcuadorCopyright © 2024 Meijer, Hickford, Gray and Schiel. This is an open-access article distributed under the terms of the Creative Commons Attribution License (CC BY). The use, distribution or reproduction in other forums is permitted, provided the original author(s) and the copyright owner(s) are credited and that the original publication in this journal is cited, in accordance with accepted academic practice. No use, distribution or reproduction is permitted which does not comply with these terms.
*Correspondence: Christopher G. Meijer, bWVpamVyY2gxM0BnbWFpbC5jb20=
Disclaimer: All claims expressed in this article are solely those of the authors and do not necessarily represent those of their affiliated organizations, or those of the publisher, the editors and the reviewers. Any product that may be evaluated in this article or claim that may be made by its manufacturer is not guaranteed or endorsed by the publisher.
Research integrity at Frontiers
Learn more about the work of our research integrity team to safeguard the quality of each article we publish.