- 1U.S. Army Corps of Engineers, Engineer Research and Development Center, Vicksburg, MS, United States
- 2U.S. Geological Survey, Mississippi Cooperative Fish and Wildlife Research Unit, Mississippi State University, Starkville, MS, United States
- 3U.S. Army Corps of Engineers Vicksburg District, Vicksburg, MS, United States
- 4College of Arts, Education, and Sciences, University of Louisiana at Monroe, Monroe, LA, United States
The effects of agriculture and flood control practices accrued over more than a century have impaired aquatic habitats and their fish communities in the Mississippi Alluvial Valley, the historic floodplain of the Lower Mississippi River prior to leveeing. As a first step to conservation planning and adaptive management, we developed and tested a conceptual model of how changes to this floodplain have affected stream environments and fish assemblages. The model is deliberately simple in structure because it needs to be understood by stakeholders ranging from engineers to farmers who must remain engaged to ensure effective conservation. Testing involved multivariate correlative analyses that included descriptors of land setting, water quality, and fish assemblages representing 376 stream samples taken over two decades and ranging in Strahler stream order from 1 to 8. The conceptual model was adequately corroborated by empirical data, but with unexplained variability that is not uncommon in field surveys where gear biases, temporal biases, and scale biases prevent accurate characterizations. Our conceptual model distinguishes three types of conservation actions relevant to large agricultural floodplains: reforestation of large parcels and riparian zone conservation, in-channel interventions and connectivity preservation, and flow augmentation. Complete restoration of the floodplain may not be an acceptable option to the agriculture community. However, in most cases the application of even the most basic measures can support the return of sensitive aquatic species. We suggest that together these types of conservation actions can bring improved water properties to impacted reaches, higher reach biodiversity, more intolerant species, and more rheophilic fishes.
1 Introduction
Streams in agricultural landscapes are impacted by a variety of anthropogenic disturbances such as land clearing, flood control, and hydrologic alterations. Conservation planning requires guidance on restoring fish communities including objective protocols for assessment of fishery resources, identification of specific management techniques, and an approach to test effectiveness of management actions. Conceptual models are a key element of resource conservation programs (Sanderson et al., 2002; Margoluis et al., 2009).
Conservation planning is complex and conceptual models simplify mental processes so that connections can be more easily abstracted to improve understanding and communication of conservation schemes among managers, researchers, and the public. Conceptual models are usually visually oriented illustrating the context within which a conservation program is operating and, in particular, the major forces influencing endpoints of concern (Knight et al., 2006). These models are hypotheses about how ecosystems are impacted by stressors and integrate contemporary understanding of ecosystem dynamics. A well-constructed conceptual model provides a scientific framework by identifying the drivers, linking stressors to their drivers, and delineating the effects of stressors and impacts on ecosystems and biota. A simple conceptual model can be a particularly useful tool when working at the landscape level with multiple and diverse agencies and stakeholder groups (Tarr, 2019).
Because of their natural fertility, floodplains in river basins in many parts of the world have been reclaimed for agriculture with ever more effective drainage and land engineering measures. Example river basins are the lower reaches of the Euphrates and Tigris, the Rhine, the Danube, the Yangtze, the Ganges, and the Mississippi, one of the largest agricultural floodplains in the world. Floodplains are some of the most engineered agricultural systems. To prevent flooding of floodplains set aside for agriculture, engineers have often built complex webs of dams, levees, and drainage ditches (Chen et al., 2016). The floodplains reclaimed often lose much of their original vegetation, wetlands, and flow patterns (Hughes and Vadas, 2021). Such modifications impact aquatic species adapted to the natural flow regime and specifically to flow and waterscape heterogeneity, leading to transformed biotic assemblages and reduced performance of ecosystem functions other than crop productivity (Tockner et al., 2008).
The historic floodplain of the Lower Mississippi River (Mississippi Alluvial Valley, MAV) lies between Cape Girardeau, Missouri and Baton Rouge, Louisiana (Figure 1). It is approximately 800 km long and up to 150 km wide and has an area of 86,635 km2 (Saucier, 1994), about the size of Portugal. The contemporary floodplain is a fraction of the historic floodplain as levees have greatly reduced flood expansion of the Mississippi River. Prior to European settlement, this region was largely covered by bottomland hardwood forests and was the second largest forested valley in the world, second only to the Amazon rainforest (Schoenholtz et al., 2001). Between the early 1800s and 1935, about one-half of the original bottomland forests in the MAV were cleared to grow cotton and other crops (Stanturf, 2006). Presently, the valley has been transformed from a bottomland hardwood ecosystem to a landscape with ~75% of the total area in croplands and remaining forests are highly fragmented (King and Keeland, 1999; Oswalt, 2013). Deforestation was followed by construction of various types of flood-control projects within and adjacent to streams channels to protect expanding croplands. Channel modifications have included clearing trees and snags along the riverbanks, enlarging cross sections with draglines or dredges, channelization, bendway cutoffs, diversions, and construction of weirs for channel maintenance.
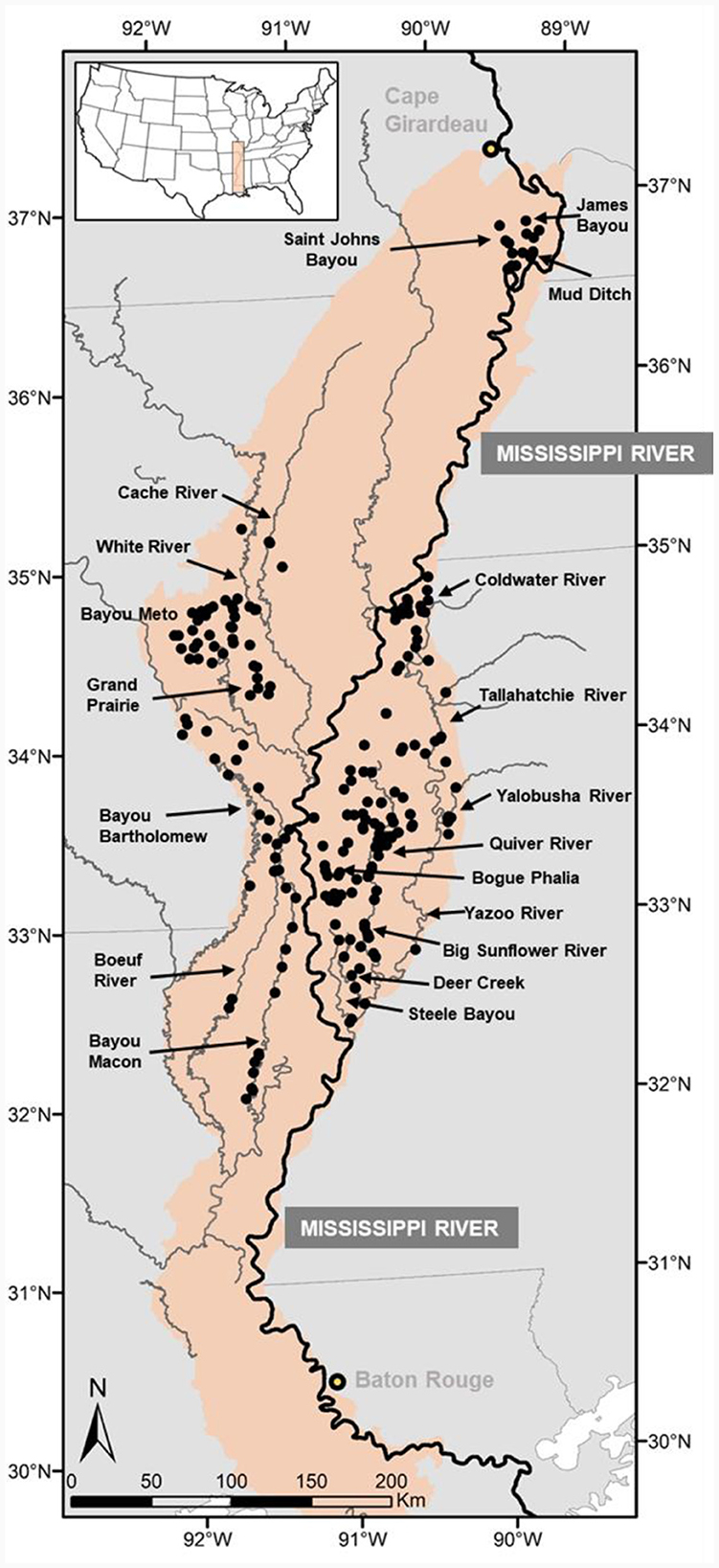
Figure 1. Sampling site locations in streams crossing the historic floodplain of the Lower Mississippi River. For reference, the Mississippi River is emphasized with a heavier line.
Agriculture and flood control practices in the MAV accrued over more than a century have impaired aquatic habitats and their fish communities (Bryant, 2010; Chen et al., 2016). Today, most streams in the MAV have soft, unconsolidated substrates consisting of silt and mud, depressed hydrographs due to groundwater depletion and water extractions for irrigation, as well as minimally forested riparian zones (Schoenholtz et al., 2001; Clark et al., 2011). The loss of forested riparian buffers, ensuing fine sediment accumulation in stream channels, and reduction of surface flows are the principal stressors to aquatic life in the low-gradient streams of the MAV. These stressors influence others (e.g., nutrients, dissolved oxygen) in a hierarchical arrangement of environmental influences that determine fish assemblage structuring (Dembkowski and Miranda, 2012; Miranda et al., 2014). Management of landuse disturbances can reverse and possibly improve or restore stream habitat condition (Flávio et al., 2017; Lenhart et al., 2018). Nevertheless, implementation of conservation practices in the MAV has been slow, partly because much of the land is privately owned and thus in most cases economics drive the decision-making process, and partly because of a lack of a largescale view of aquatic resources to guide conservation (Miranda et al., 2021).
As a first step to conservation planning and adaptive management, we developed a conceptual model of how changes to the MAV landscape may affect stream fish assemblages. The model is deliberately simple in structure because it needs to be understood by stakeholders ranging from engineers to farmers who must remain engaged to ensure effective management. It includes a general classification of the major drivers of landscape change and how they relate to stream fish assemblages at the landscape scale. Our goal in this article is to evaluate this framework with empirical data collected spatially as historical fish records pre-deforestation are not available in the MAV. Based on our analysis we suggest possible management actions to conserve or restore aquatic habitat quality in streams of this floodplain ecosystem by focusing on the principal environmental variables altered by agricultural practices.
2 The Mississippi Alluvial Valley
In 1820, the naturalist John J. Audubon was traveling on the Lower Mississippi River and recorded the following as he stopped near the mouth of the Yazoo River, one of the largest tributaries: “a beautiful stream of transparent water, covered with thousands of geese and ducks and filled with fish” (Rhodes, 2004). Many of the tributaries to the Lower Mississippi River in the early 1800s were likely tannin-stained, blackwater streams with sandy and gravel substrates, perennial flows, and bordered by extensive bottomland hardwood forest. Two centuries of landscape modifications have gravely transformed the MAV ecosystem.
Currently, slow turbid flows and seasonally stagnant water are typical of MAV streams. These circumstances are aggravated by a mild north-south landscape gradient of ~0.1 m/km (Saucier, 1994). In streams with extensive agricultural catchments, flows are further impacted by groundwater depletion, weirs, levees, and other water management structures. Consequently, streams often switch seasonally between flowing and non-flowing states. Water velocity and discharge are particularly important in structuring fish communities (Schlosser, 1985). The repeated onset and cessation of flow influence biotic communities by the advance and retreat of wetted fronts, attenuation of flow pulses, hydrological disconnection of persistent reaches and pools, and reduced flow permanence (Larned et al., 2009). Ironically, without weirs and other engineered structures many MAV streams may dry up completely during low-flow seasons because of a disconnection from the alluvial aquifer caused by excessive withdrawal for irrigation (Reba et al., 2017).
The accumulation of fine substrates in MAV streams and associated high turbidities correspond to long-term conversion of bottomland hardwood wetlands to cropland. Fine sediment deposition results in homogenization of substrates and the impoverishment of benthic aquatic communities (Wood and Armitage, 1997; Mathers et al., 2022). Forested riparian zones that filter runoff, increase bank stability, increase periodic food availability, contribute woody debris and leaf packs, and improve the structural complexity of stream channels (Rabeni, 1995; Benke and Wallace, 2003) are often absent in MAV streams, or greatly reduced or transformed by agricultural development. The apparent organization of fish assemblages along a gradient of environmental disturbances supports the notion that the combined loss of forests and accretion of sediment in stream channels impair lotic habitats and selected species assemblages.
Despite these environmental impacts, the various landscapes and waterscapes in the MAV provide habitat for a diverse fauna including about 40 species of mussels, 45 species of reptiles and amphibians, 50 species of mammals, and about 60% of all bird species in the contiguous United States (Brown et al., 2000; Jones et al., 2005). Moreover, nearly 200 fish species have been documented in the streams, lakes, and associated backwaters of the MAV (Baker et al., 1991; Dembkowski and Miranda, 2014; Schramm et al., 2016) making the MAV a principal center of biodiversity in North America (Burr and Page, 1986; Conner and Sutkus, 1986; Robison, 1986). Total number of fish species includes native fish at the periphery of their range and at least 17 non-native species that have established self-sustaining populations in one or more reaches of the Mississippi River (Schramm et al., 2016). Five families of fish taxonomically dominate the ichthyofauna of the MAV (Cross et al., 1986): Cyprinidae (N = 83 species), Percidae (N = 46), Centrarchidae (N = 22), Catostomidae (N = 21), and Ictaluridae (N = 19). These fish species have historically shared a common distribution throughout the MAV, but today, variation in occurrence, and abundance have been altered by landscape and riverscape engineering and ensuing environmental change (Baker et al., 1991; Schramm et al., 2016).
3 The conceptual model
We developed a conceptual model that relates landscape-level conditions on local-level stream water properties and fish assemblages in streams and bayous of the MAV. To frame the model, we drew from an extensive literature on stream impairment (e.g., Schlosser and Karr, 1981; Allan et al., 1997; Poff et al., 1997; Wang et al., 2002), from previous research we have conducted in the MAV (e.g., Killgore et al., 2008; Dembkowski and Miranda, 2012), and from over 30 years of practical experience studying fish in streams and backwaters in the MAV and adjacent regions. The model identifies agricultural development as the driver (Figure 2). Principal stressors influenced by agricultural development include deforestation, erosion, and water withdrawal. To keep the model simple, some stressors and effects were not considered individually because they are likely correlated with others. For example, mobilization of contaminants such as nutrients and pesticides were not included but are generally linked to the same processes that drive sediments (Bainbridge et al., 2009), and therefore increased sediment transport generally signals elevated nutrients. Therefore, variation due to attributes not included in the model is likely at least partially reflected by those included. Effects of stressors include loss of instream habitat diversity through sedimentation and increased turbidity, reductions in discharge resulting in intermittent flows that reduce water quality including higher temperatures and lower dissolved oxygen, as well as reductions in riparian stream cover and associated increases in temperature and declines in woody debris. Our conceptual model predicts that these effects alter fish assemblages in the MAV, leading to reductions in rheophilic species, substrate spawners, intolerant species, and various others leading to a reduction in biodiversity and fish assemblage integrity.
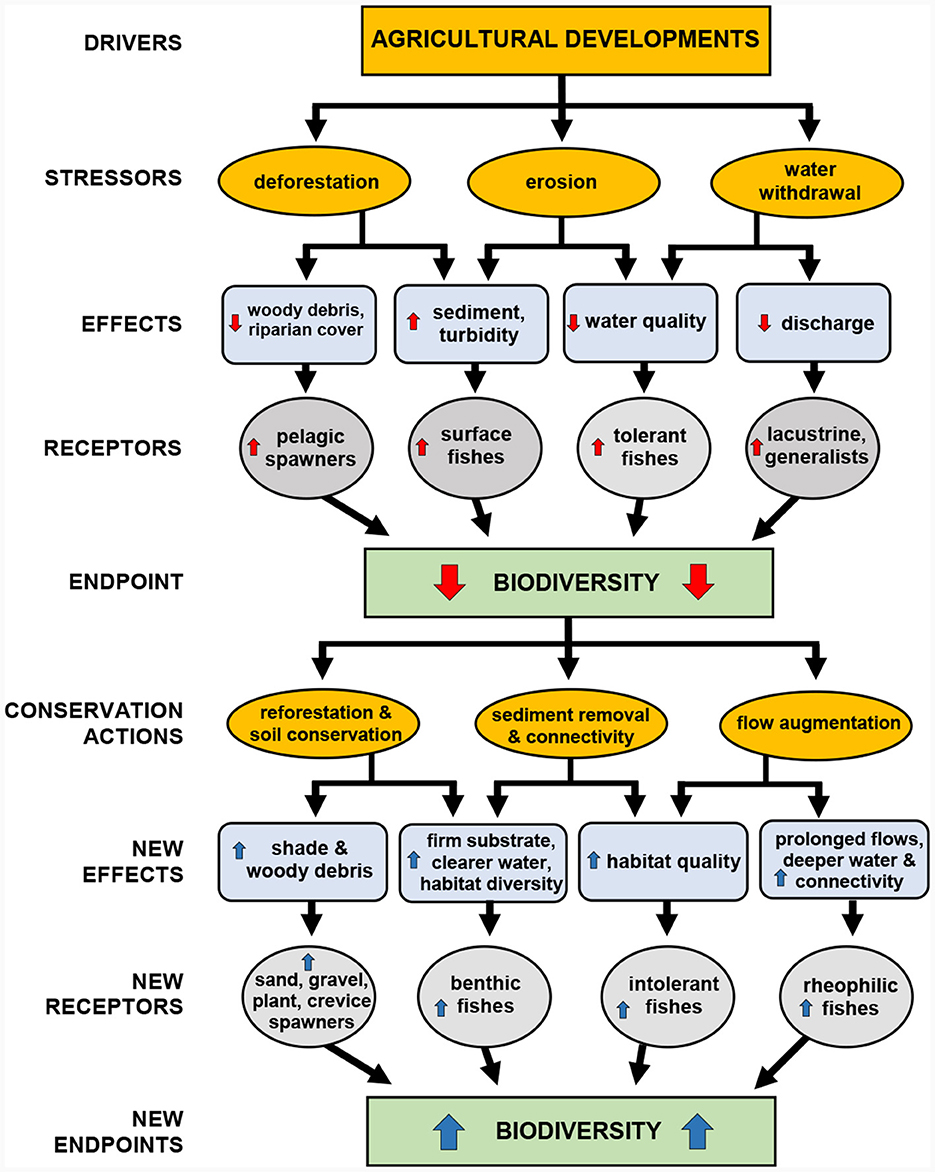
Figure 2. A conceptual model for fish conservation in streams of the Lower Mississippi River historic floodplain, identifying drivers, stressors, effects, receptors, endpoints and conservation actions. Upward arrows in red and blue indicate increase; downward indicate decrease.
4 Model testing
We used various metrics indicative of environmental stress to test the suitability of the conceptual model as a practical operational framework for fish conservation in the MAV. Metrics were recorded at diverse streams throughout the valley (Figure 1), although not all samples had a complete set of metrics. Testing involved multivariate correlative analyses to evaluate robustness of association among sets of stressors, effects, and receptors and their statistical significance.
4.1 Sampling sites
In all, 376 samples were taken in MAV streams throughout the Mississippi Alluvial Plain and South Central Plains Level 3 ecoregions ranging in Strahler order from 1 to 8. The number of sites sampled and years of sampling varied by system, but all sites were sampled during summer and autumn (Julian Day range = 173–341). Systems sampled included the Yazoo River (12 years in 1993–2010) represented by the Yazoo River, Coldwater River, Tallahatchie River, Yalobusha River, Big Sunflower River, Quiver River, Bogue Phalia, Deer Creek, and Steele Bayou (N = 233 samples); the White River (6 years in 1996–2003) including the middle and lower White River, Cache River, and Grand Prairie Bayou (N = 59 samples); the Arkansas River (2 years, 1996 and 2000) including Bayou Meto (N = 23 samples); the lower Ouachita River (3 years, 2001–2003) including Bayou Bartholomew, Boeuf River, and Bayou Macon (N = 43 samples); the lower Red River (2 years, 2001–2002), (N = 21 samples); and the St. Johns—New Madrid system (1 year, 2007) including Mud Ditch, James Bayou and Saint Johns Bayou (N = 18 samples). Systems sampled were not randomized as collections were part of independent investigations spaced over two decades. Nevertheless, the methodology applied was standardized to collect uniform environmental and fish data across all study streams.
4.2 Collections
We recorded multiple metrics at each site reflecting the physicochemical environment including discharge, sediment depth, turbidity, temperature, dissolved oxygen, and pH (details about metrics and methodology given in Table 1). These metrics were selected because they have often been linked to environmental degradation. Offsite we used geographic information systems (GIS) tools and data (NLCD, 2016) to estimate the size of the catchment area above a sampling site and the percentage of croplands and forestlands in the catchment. We also used GIS tools to identify the Strahler stream order for the sampling site.
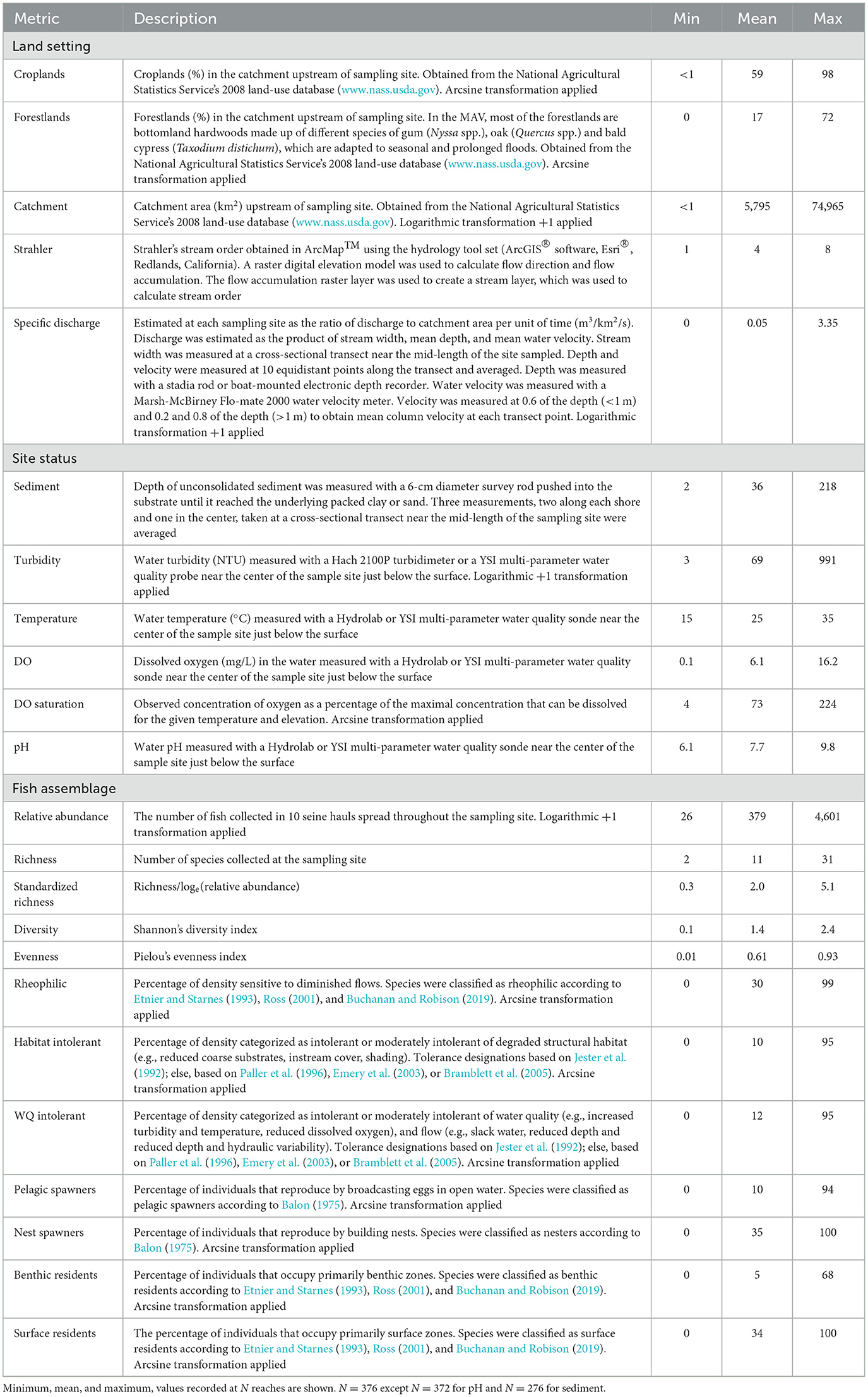
Table 1. Metrics represent land setting, site status, and fish assemblage characteristics in the Mississippi Alluvial Valley.
Fish were collected using a 3-m long by 2.4-m tall seine, with 5-mm mesh. Seining was chosen as the preferred gear because this technique can be employed in all sizes of streams and targets mostly small-body species and juveniles of large-body species. However, efficiency of the net may vary depending on stream size (more below). Ten seine hauls were equitably distributed among all apparent macrohabitats within the defined boundaries of the sampling reach. Fish collected by the 10 hauls were pooled and either identified to species, counted, and released or preserved in a formalin solution for laboratory processing (the majority). In the laboratory, they were rinsed, identified to species, counted, stored in alcohol, and vouchered. To avoid working with taxonomic classifications that have geographic specificity, we converted species designations into several non-taxonomic structural and functional metrics that span biogeographic zones and may more effectively reflect stream degradation in agrarian systems (Appendix 1). Moreover, collective properties of fish communities are likely more robust and responsive to long term environmental effects than individual species which may be subject to short-term fluctuations in population structure and abundance.
4.3 Data analysis
To identify effective management techniques that target habitat improvements for fish species assemblages of conservation needs, we asked the following question: Were widespread landscape modifications to support agriculture development correlated with local site status (i.e., abiotic environmental conditions), and if so, was that status correlated with fish metrics? To address this question canonical correlation analysis (CCA; SAS, 2015) was applied to measure the association between (1) land setting descriptors and site status, and in turn (2) site status and fish metrics. The land setting descriptors included landcover percentages of croplands and forestlands within the catchment area upstream of each site, as well as catchment area extent and stream order. These last two variables were included to account for inherent effects that stream size have on water properties and on fish assemblages. For example, discharge and turbidity characteristically change with catchment area and stream order. Similarly, species richness increases with catchment area and stream order resulting in shifting species compositions. Additionally, the efficiency of seine sampling is affected by stream size adding bias to assemblage descriptions. Including catchment area and steam order as covariates in the analysis allowed for a more direct connection to land cover and accounted for the bias introduced by decreased seining efficiency in larger streams.
To assess the relationship between two sets of variables, CCA reconstructs each set of variables into multiple pairs of synthetic variables. Each synthetic variable is generated to yield the largest possible canonical correlation between the two sets of variables. The canonical correlation is a Pearson r between a pair of synthetic variables. Any residual variance unexplained by the first pair of synthetic variables is used to create a second pair that is orthogonal (i.e., uncorrelated) with previous pairs. Analogous to a principal component analysis, this process repeats until all the variability is explained by subsequent pairs of synthetic variables. A major assumption of CCA is that all associations are linear. Large deviations from linearity could lead to reduced or no correlation. The linearity assumption was addressed for some variables with logarithmic transformations or arcsine transformations for percentage data (Table 1).
To appraise the resulting canonical correlations, we first evaluated the full canonical model to test if there was a relationship between the two sets of variables, and if so, we went further and examined relevant pairs of synthetic variables (i.e., those that explained the most amount of variance). The full canonical model was evaluated with Wilks's lambda (λ). This test statistic is used in multivariate procedures to measure the fraction of the variance in dependent variables unexplained by differences in levels of the independent variable. Therefore, 1 – λ is the proportion of variance explained by the full canonical model and, while not equivalent, can be interpreted like a multiple R2 in regression. Next, we evaluated each pair of synthetic variables generated by CCA. Each pair explains a decreasing amount of the overall variability until some of the pairs may not explain enough to warrant interpretation. Interpreting pairs of synthetic variables that account for only a small fraction of the overall association between the sets of variables risks interpreting an effect that may not be real. Lastly, we evaluated, in terms of directionality and intensity, which metrics were related to synthetic variables. For this we examined the sign and strength of the Pearson correlation between each synthetic canonical variable and each of its metrics.
5 Results
The 376 samples represented a gradient in physical habitat and water quality. Catchment areas ranged < 1 to 74,965 km2 and were drained with < 1 to 98% croplands and 0–72% forestlands (Table 1). Other than croplands and forestlands, wetlands including swamps, scrub-shrub wetlands, emergent wetlands, and abandoned river channels represented the third major land cover class. This broad diversity of land settings produced a broad range of physicochemical conditions ranging from no specific discharge to 3.35 m3/km2/s. Turbidity ranged from relatively clear to highly turbid and sediment from hard substrates to fine sediment deposits >2 m deep. Dissolved oxygen saturation at the sites ranged from about 10% to >200%, suggesting extreme environmental conditions that may fluctuate widely in diel cycles.
A total of 142,452 fish representing 116 species and 21 families were collected in the MAV (Appendix 1). Our species list is comparable to zoogeographic accounts by Conner and Sutkus (1986) and Cross et al. (1986) who report five families dominate the taxonomic diversity of the ichthyofauna of the MAV. The most species-rich families in our study were: Cyprinidae (N = 35 species), Percidae (N = 21 species), Centrarchidae (N = 16 species), Catostomidae (N = 9 species), and Ictaluridae (N = 9 species). Overall, Western mosquitofish Gambusia affinis (19.7%), bluegill Lepomis macrochirus (9.2%), blacktail shiner Cyprinella venusta (8.4%), orangespotted sunfish Lepomis humilis (6.6%), and bullhead minnow Pimephales vigilax (4.0%) were the most common species. Ten species were collected in the transitional zone between upland (Ozark Highlands and Ouachita River Ecoregions) and lowlands in the MAV, and may be rare or absent in the Mississippi Alluvial Plain Ecoregion (Appendix 1). Of the 116 species collected, only four species were introduced including the invasive silver carp Hypophthalmichthys molitrix (Appendix 1). However, invasive species comprised < 1% of the total number collected with seines possibly due to the resilience of high native species richness.
The biodiversity and structural and functional fish assemblage metrics further illustrate site and faunal diversity in the MAV. Most of the diversity metrics varied greatly among sites with species richness ranging from 2 to 31 and evenness from near 0 to 0.93 (out of a 0–1 possible range). Similarly, most of the functional metrics ranged from 0 to about 100%. This broad array of biodiversity and functional metrics coupled with the diversified land setting and site status provided a suitable backdrop for testing the conceptual model.
The CCA revealed various associations between land setting, site status, and fish metrics. For the land setting—site status CCA the full model across all functions was statistically significant [λ = 0.34, F(24, 859) = 12.9, P < 0.001]. Likewise, for the site status—fish metrics CCA the full model across all functions was statistically significant [λ = 0.21, F(72, 1241) = 5.8, P < 0.001]. Because Wilks's λ represents the variance unexplained by the model, 1– λ yields the full model effect size in an R2 metric. Thus, the full model accounted for 66% of the variance shared by the land setting variables and the site status variables (Figure 3). Similarly, the full model accounted for 79% of the variance shared by the site status variables and the fish metrics (Figure 4).
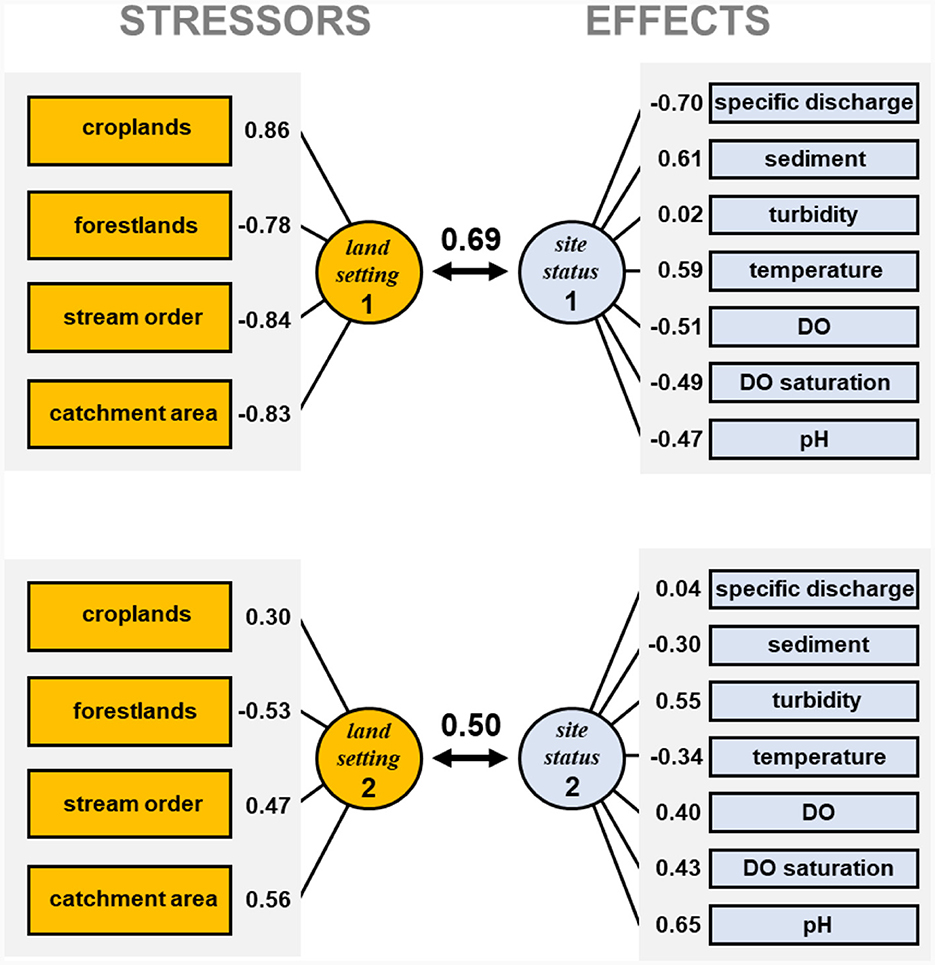
Figure 3. Canonical correlations between stressors and effects. Canonical correlations between the first two synthetic variables are shown (0.69 and 0.50, respectively), along with the correlation between each variable and corresponding synthetic variable. Definitions of each stressor and effect are listed in Table 1. Land setting 1 represented increasing croplands and decreasing forestlands with decreasing stream sizes, an effect related to croplands more closely bordering small streams than large ones. Site status 1 represented reduced DO and pH and increased temperature in streams with low specific discharge and high sediment. In contrast, land setting 2 emphasized increasing croplands in large streams rather than small streams. Site status 2, had a positive correlation with turbidity, DO, DO saturation, and pH, but a negative correlation with temperature and sediment, suggesting that increases in croplands in large streams do not result in the heavy sediment accumulations as in smaller streams, yet large streams are more turbid and are able to stay cooler and maintain higher DO and pH.
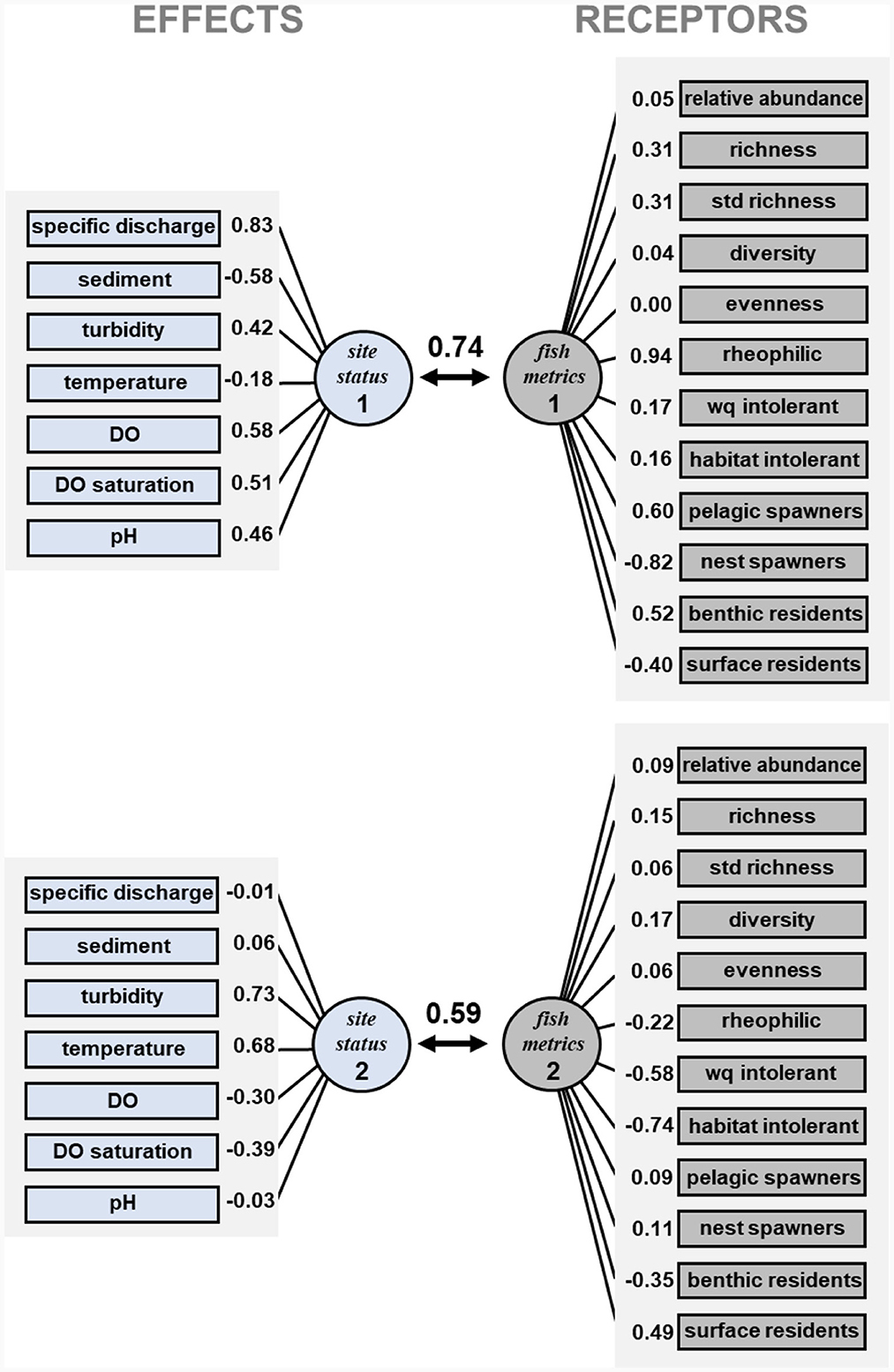
Figure 4. Canonical correlations between effects and receptors. Canonical correlations between the first two synthetic variables are shown (0.74 and 0.59, respectively), along with the correlation between each variable and corresponding synthetic variable. Definitions of each effect and receptor are listed in Table 1. The first canonical function represented a positive effect of discharge on key fish biodiversity metrics, but a negative effect on nest spawners and surface residents which are usually associated with lentic waters. The second canonical function represented the negative effect that high stream turbidity and temperature, and low dissolved oxygen, exert over species intolerant of reduced water quality, altered substrates, and overall channel degradation. The second canonical function also reflects how these changes in environmental quality, particularly increases in turbidity and temperature, diminish intolerant species, and promote development of surface-oriented fish assemblages that can better withstand precarious environmental conditions that may shift daily and seasonally.
5.1 Stressors—Effects correlation
The CCA created four canonical functions for the land setting and site status correlation, but only the first two functions were statistically significant (P < 0.05). The canonical coefficient for the first canonical function was 0.69. In the first canonical function the land setting synthetic variable (land setting 1, Figure 3) had a positive correlation with croplands and a negative correlation with forestlands, stream order, and catchment area. Thus, land setting 1 represented increasing croplands and decreasing forestlands with decreasing stream sizes, an effect related to croplands more closely bordering small streams than large ones. The site status synthetic variable (site status 1, Figure 3) had negative correlations with specific discharge, DO, DO saturation, and pH but positive correlations with sediment and temperature. Thus, site status 1 represented reduced DO and pH and increased temperature in streams with low specific discharge and high sediment. High percentages of croplands were most associated with smaller streams and coincided with reduced specific discharge, increased sediment, higher temperatures, and reduced DO and pH.
The canonical coefficient for the second land setting and site status canonical function was 0.50. The second synthetic variable land setting 2, like land setting 1, had a positive correlation with croplands and a negative correlation with forestlands, but unlike land setting 1, land setting 2 had a positive correlation with stream order and catchment area (Figure 3). Thus, in contrast to land setting 1, land setting 2 emphasized increasing croplands in watersheds of larger streams rather than smaller streams. The site status synthetic variable for the second canonical function (site status 2, Figure 3) had a positive correlation with turbidity, DO, DO saturation, and pH but a negative correlation with temperature and sediment. This correlation structure suggests that increases in croplands in watersheds of larger streams do not result in the heavy sediment accumulations seen in smaller streams, yet large streams are more turbid and are able to stay cooler and maintain higher DO and pH.
5.2 Effects—Receptors correlation
The CCA created six canonical functions for the site status and fish metrics correlation, and the first two functions were statistically significant (P < 0.05). The canonical coefficient for the first canonical function was 0.74. In this first canonical function the site status synthetic variable (site status 1, Figure 4) had a strong positive correlation with specific discharge and with turbidity, DO and pH, and a negative correlation with sediment. The first fish metrics synthetic variable (fish metrics 1, Figure 4) had positive correlations with species richness, rheophilic species, pelagic spawners, and benthic residents. Moreover, it had negative correlations with nest spawners and surface residents. Thus, the first canonical function represented a positive effect of discharge on key fish biodiversity metrics, but a negative effect on nest spawners and surface residents which are usually associated with lentic waters.
The canonical coefficient for the second site status and fish metrics canonical function was 0.59. The second synthetic variable site status 2 had positive correlations with turbidity and temperature and negative correlations with the two dissolved oxygen variables (Figure 4). Thus, in contrast to site status 1, site status 2 emphasized water quality characteristics rather than streamflow. The fish assemblage synthetic variable (fish metrics 2) had negative correlation with water quality and habitat intolerance and with benthic fishes, but a positive correlation with surface fishes. Thus, it appears that the second canonical function represented the negative effect that high stream turbidity and temperature, and low dissolved oxygen, exert over species intolerant of reduced water quality, altered substrates, and overall channel degradation. The second canonical function also reflects how these changes in environmental quality promote development of surface-oriented fish assemblages that can better withstand precarious environmental conditions that may shift daily and seasonally.
6 Discussion
We developed a conceptual model linking agricultural development in the historic floodplain of the Lower Mississippi River to stream environmental properties and fish species assemblages. To test our conceptual model, we used an extensive dataset collected over two decades throughout various stream systems in the MAV. We estimated canonical correlations between metrics descriptive of land setting, site status, and fish assemblages. Our findings are consistent with previous studies that have found negative effects of agricultural development on stream ecosystems (e.g., Lasne et al., 2007; Schürings et al., 2022), but patterns unique to floodplain ecosystems were apparent.
The impacts of agriculture on site status and fish assemblages depended on stream size. Generally, the percentage of croplands was inversely related to stream size as croplands encroached closer to stream channels. In contrast, high order streams tend to be better buffered by forested wetlands that are generally too costly to transform to cropland and many forested wetlands have been set aside as conservation easements. Lower order streams surrounded by high percentages of croplands reflected reduced specific discharge creating intermittent flows, deep sediment, high temperature, and reduced DO and pH. These alterations to environmental conditions, along with others unavailable to include in our study, diminish biodiversity of the fish assemblages likely due to reduction of rheophilic species, intolerant species, benthic species, and pelagic spawners, leaving a high representation of nest spawners and surface residents.
Sensitive taxa tend to decline with agricultural impact, while tolerant taxa tend to benefit (Schürings et al., 2022). In our study, nest spawners were commonly represented by small centrarchids including bluegill, orangespotted sunfish, green sunfish Lepomis cyanellus, and juvenile white crappie Pomoxis annularis. These species can tolerate environmental extremes and survive in streams where conditions are degraded, they have high reproductive potential, and they are often the species that first recolonize recently inundated areas. Surface residents included principally the Western mosquitofish, a live-bearer, surface feeder capable of surviving extreme hypoxia by extracting oxygen from the interface between water and air, and capable of surviving high temperatures (Hoover and Killgore, 1998). The physiological adaptations of these and other tolerant species result in a depleted assemblage dominated by a few tolerant species.
Increases in croplands and reductions in forestlands in smaller streams were correlated with heavy sediment accumulations, but not higher turbidity, as suggested by the first land setting canonical axis. Moreover, temperature increased and dissolved oxygen decreased. These effects are likely related to lower base flows in smaller streams that mitigate the effects of croplands on turbidity. Also, irrigation return water into small streams during the summer has low turbidity and cooler water. Increased baseflows in larger streams with larger catchment areas were in fact associated with increased turbidity as suggested by the second land setting canonical axis. The increase in turbidity in larger steams was associated with increases in rheophilic species, pelagic spawners, and species richness as suggested by the first site status canonical axis. However, these increases are probably an effect of stream size rather than turbidity. The second site status canonical axis confirmed the reduction of intolerant species under turbid conditions.
Our analyses suggested relationships between forestlands and site status, and between site status and fish assemblage metrics. Forestlands were associated with enhancement of discharges, oxygen, and pH and reductions in sediment and temperature. The positive effects of forestlands on aquatic environments have been well-documented in the literature (e.g., Schlosser, 1991; Harding et al., 1998; Sutherland et al., 2002). However, forestlands in floodplains are different. Most of the forests in the MAV are bottomland hardwoods, which are forested wetlands that are seasonally flooded or covered with water much of the year (Schoenholtz et al., 2001). Alternating wet and dry periods are controlled by elevation and determine the fish species assemblages that develop, supporting biodiversity. These floodplain forests have persisted mostly because they are in tracts unsuitable for conversion to cropland. Forestlands in floodplains can help augment flows, cleanse water that eventually may be added to streams, supplement aquatic habitats and refugia available to stream species, provide rearing habitats for juvenile stream fish, and contribute wetland species to stream faunas. Various species in our data set were positively correlated with forestlands including the cyprinids Mississippi silvery minnow Hybognathus nuchalis, mimic shiner Notropis volucellus, bluntface shiner Cyprinella camura, and Yazoo shiner Notropis rafinesquei; the percids saddleback darter Percina vigil, slough darter Etheostoma gracile, and logperch Percina caprodes; and the centrarchid spotted bass Micropterus punctulatus.
7 Conservation implications
Basin-wide restoration of the MAV aquatic ecosystem may not be an acceptable option to the agriculture community. In most cases, however, the application of even the most basic measures, such as buffer strips, can support the return of key aquatic species by providing benefits such as shade, woody debris, bank stability, flow regulation, and runoff purification. To this end we distinguish three types of conservation actions relevant to the MAV and potentially other large agricultural floodplains with demonstrated benefits to ecologically-important fish assemblages: (1) reforestation of large parcels, riparian zone protection, and soil conservation; (2) Sediment removal and management to preserve connectivity and improve benthic habitats, and (3) flow augmentation. We suggest that together these types of conservation actions can bring improved environmental quality to impacted reaches, higher reach biodiversity, more intolerant species, and more rheophilic species.
7.1 Reforestation, riparian zone protection, soil conservation
Whereas, a large number of conservation practices have been applied on agricultural floodplains (Faulkner et al., 2011), reforestation of large sections of the MAV has been the ultimate goal for many years, but with climate uncertainties reforestation of agricultural lands has also become an international issue (Hanberry et al., 2012). The impetus for this effort has focused on the restoration of habitat for migratory birds, yet these efforts benefit water resources through increased floodwater retention and reduced sediment loads. To preserve agriculture interests, reforestation often takes the form of conversion of marginal cropland to timber plantations, bottomland hardwoods set asides, and riparian forest buffers that include mainly lower-lying areas that are difficult to farm because of inadequate drainage or flood control. Agroforestry, defined as the integration of trees into productive agricultural systems, may provide additional options to expand reforestation into higher areas (Dosskey et al., 2012). Agroforestry plantings can be located and designed to provide key ecological attributes normally provided by bottomland hardwood forests. Reforestation in the region is driven primarily by actions of federal and state agencies and by incentive programs that buy conservation easements (Stanturf et al., 2000). As an example, the Conservation Reserve Program of the U.S. Department of Agriculture Farm Service Agency incentivizes maintenance of perennial cover in farms across the MAV. This incentive to return croplands to forestlands stops the tillage of soils in areas enrolled in the program and greatly reduces agrichemical applications.
In certain scenarios faster progress can be made with riparian forest buffers. These are areas adjacent to a stream, oxbow lake, or wetland that contains a combination of trees, shrubs, and/or other perennial plants and are managed primarily to provide conservation benefits adjacent to agricultural lands. Buffers have multiple objectives including (1) improve habitat for aquatic species by creating shade to lower water temperatures while providing a source of detritus and large woody debris; (2) create wildlife habitat and establish wildlife corridors; (3) block excess amounts of sediment, organic material, nutrients, and pesticides in surface runoff; (4) restore natural riparian plant communities; and (5) increase carbon storage in plant biomass and soils. The width of buffers along streams depends on the purpose of the project (Fischer and Fischenich, 2000). If the purpose is to provide shade along the littoral zone and input of organic debris, only a narrow (e.g., 10 m) strip may be sufficient. Lovisa et al. (2019) developed the concept of “Ecologically Functional Riparian Zones” suggesting that a 3 m wide buffer zone acts as a basic nutrient filter. However, to maintain a high floral diversity, a 24 m buffer zone is required, while a 144 m buffer is needed to preserve bird diversity. Riparian buffers cannot supply the benefits of largescale reforestation projects but can provide minor reach-scale improvements that in small building blocks further biodiversity conservation. Development of riparian buffers relies on landowner cooperation which is incentivized by a wide variety of state and federal programs that support the installation of riparian forest buffers on public and private lands.
7.2 Sediment removal and connectivity preservation
Excessive sediment is a major concern in river systems that flow through agricultural catchments. Fine sediment accumulation influence hydrology and substrates, directly impacting benthic species and indirectly the entire biota. In streams that flow through a floodplain, the riverbanks are often at higher elevation than surrounding lands, so runoff moves from agricultural fields into ditches or small tributaries before it reaches streams. This path presents various opportunities for implementing structural management practices, such as drop pipes, that aim to reduce sediment transport (Kroger et al., 2012; Kroll and Oakland, 2019; Antolini et al., 2020). Direct removal of sediment in selected reaches may be required to return degraded streams already saturated with fines and unlikely to self-cleanse to a closer approximation of their natural hydrology (Naden et al., 2016; Langland et al., 2020; McMahon et al., 2021). Removal of soft sediments often leads to re-colonization of benthic fish (e.g., darters, madtoms) and increased diversity of nest-building species (e.g., sunfishes).
Connectivity with abandoned channels and neighboring wetlands is a key feature of streams in floodplain ecosystems but is impaired by the documented reductions in discharge, perching of wetlands, and excessive sedimentation. Connectivity performs several functions including refill of lateral waters that recharge groundwater and sustain river baseflows; retention and transformation of nutrients, sediment, and pesticides; storage and subsequent release of floodwaters; and provision of spawning and protective habitats for stream species (Fritz et al., 2018; Lane et al., 2018). Increases in sediment associated with agriculture disrupt the balance between a stream's sediment load and discharge, channel slope, and channel dimensions (Ferguson and Hoy, 2008). Sedimentation often blocks low-water connections and fish access between the stream and wetlands. Attempts to regain balance through engineering solutions such as leveeing (prevents bank overflow) and channelization (reworks discharge, channel slope, and dimensions lowering stream bed) can further degrade connectivity. Identifying the factors that facilitate or limit connectivity is critical to effectively managing stream habitat and fish assemblages.
7.3 Flow augmentation
Though rainfall in the MAV is abundant, its timing and quantity often do not coincide with crop needs. Thus, agriculture has increasingly turned to irrigation to optimize yields and mitigate risks associated with drought (Reba et al., 2017). Extensive irrigation coupled with the region's geology have led to significant declines in aquifers over various segments of the Mississippi embayment aquifer system, producing precarious low flows during low-rainfall months. Engineered structures such as low-crest weirs or rock ramps that consider periodic fish passage opportunities (Kapitzke, 2010) can maintain pools, avoid desiccation of freshwater mussel beds, and create tailwaters during the low-water season. Riprap used in weir construction and adjacent bank stabilization provides several benefits in MAV streams where hard substrates may be limited. Rock substrates provide sites for attachment of invertebrates that fish feed upon, and riprap creates interstitial spaces and bathymetric diversity used by fish for predator avoidance and spawning crevices (Dardeau et al., 1995). Pools above weirs provide surrogate habitat conditions for specialized wetland fishes that have declined region-wide due to habitat loss. Flowing tailwaters below weirs are comparable to riffles in streams and become colonized by rheophilic fish. Although weirs provide benefits, during low water stages they do convert lotic to lentic habitat and can be an impediment to fish passage. Other options for environmental flows include augmentation from storage reservoirs, pumping from re-charged aquifers, or inter-basin transfers. Currently, the Yazoo Mississippi Delta Joint Water Management District operates several wells near the Mississippi River, where the aquifer is recharged annually, to supplement seasonal flows in the Big Sunflower River.
8 Conclusions
Deforestation, agriculture, and hydrological modifications that sustain agriculture have severely transformed the historic floodplain of the Lower Mississippi River. Managing the waterscape of a historic floodplain as large as that of the Lower Mississippi River can be daunting. The conceptual model described here was designed to facilitate this task by helping to focus communication among scientists, managers, farmers, and policy makers through the visual depiction of linkages between major system drivers, stressors, effects, receptors, and ecological endpoints important to the region's fish assemblage. The conceptual model was adequately corroborated by empirical data, but there was substantial unexplained variability that is not uncommon in field surveys where gear biases, temporal biases, scale biases, and other biases prevent accurate characterization of stream reaches. Moreover, our analysis could not possibly account for all potential stressors and effects that directly or indirectly impact receptors. Nevertheless, given the statistically significant association observed with landscape variables and site status, we suggest the model is suitable as a first step to conservation planning and adaptive management. Our conceptual model outlines a long-term approach for restoring degraded habitats and improving biodiversity in the Lower Mississippi River historical floodplain by re-establishing riparian corridors, managing sediment input, and improving base flows.
Data availability statement
The original contributions presented in the study are included in the article/Supplementary material, further inquiries can be directed to the corresponding author.
Ethics statement
The animal study was conducted under the Institute of Animal Care Committee at the Engineer Research and Development Center, and accredited by the Care and Use of Laboratory Animals in DOD Programs. The study was conducted in accordance with the local legislation and institutional requirements.
Author contributions
KK: Data curation, Funding acquisition, Investigation, Methodology, Project administration, Resources, Supervision, Writing—original draft, Writing—review & editing. JH: Conceptualization, Investigation, Methodology, Resources, Supervision, Visualization, Writing—review & editing, Writing—original draft. LM: Conceptualization, Formal analysis, Methodology, Validation, Visualization, Writing—original draft, Writing—review & editing. WS: Data curation, Funding acquisition, Investigation, Project administration, Resources, Supervision, Writing—review & editing. DJ: Conceptualization, Formal analysis, Investigation, Methodology, Writing—review & editing. ND: Data curation, Investigation, Methodology, Resources, Writing—review & editing.
Funding
The author(s) declare financial support was received for the research, authorship, and/or publication of this article. Funding was provided by the U.S. Army Engineer Vicksburg District managed by Kent Parrish and the Ecosystem Management and Restoration Research Program of the Engineer Research and Development Center.
Acknowledgments
We are grateful for the field assistance of many people: Reid Adams, Joseph Beard, Krista Boysen, Jay Collins, Steven George, Sherry Harrell, Bill Lancaster, Bradley Lewis, Catherine E. Murphy, and Larry Sanders all with ERDC (now or previously) accounted for most collections. Field collections were curated by Dr. Neil Douglas into the collections of the University of Louisiana at Monroe Museum of Natural History. In 2019, these collections were transferred to the Mississippi Museum of Natural Science in Jackson, Mississippi. Michael Moore, USGS Iowa Cooperative Fish and Wildlife Research Unit, provided a constructive review. This study was partially performed under the auspices of ERDC protocol # EL-FR-2019-3. Any use of trade, firm, or product names is for descriptive purposes only and does not imply endorsement by the U.S. Government.
Conflict of interest
The authors declare that the research was conducted in the absence of any commercial or financial relationships that could be construed as a potential conflict of interest.
Publisher's note
All claims expressed in this article are solely those of the authors and do not necessarily represent those of their affiliated organizations, or those of the publisher, the editors and the reviewers. Any product that may be evaluated in this article, or claim that may be made by its manufacturer, is not guaranteed or endorsed by the publisher.
Supplementary material
The Supplementary Material for this article can be found online at: https://www.frontiersin.org/articles/10.3389/ffwsc.2024.1365691/full#supplementary-material
References
Allan, J. D., Erickson, D. L., and Fay, J. (1997). The influence of catchment land use on stream integrity across multiple spatial scales. Freshw. Biol. 37, 149–161. doi: 10.1046/j.1365-2427.1997.d01-546.x
Antolini, F., Tate, E., Dalzell, B., Young, N., Johnson, K., and Hawthorne, P. (2020). Flood risk reduction from agricultural best management practices. J. Am. Water Resour. Assoc. 56, 161–179. doi: 10.1111/1752-1688.12812
Bainbridge, Z., Faithful, J., Lewis, S., and Brodie, J. (2009). Identifying the land-based sources of suspended sediments, nutrients and pesticides discharged to the Great Barrier Reef from the Tully basin, Queensland, Australia. Mar. Freshw. Res. 60, 1081–1090. doi: 10.1071/MF08333
Baker, J. A., Killgore, K. J., and Kasul, R. L. (1991). Aquatic habitats and fish communities in the Lower Mississippi River. Rev. Aquat. Sci. 3, 313–414.1
Balon, E. K. (1975). Reproductive guilds of fishes: a proposal and definition. J. Fish. Res. Board Can. 32, 821–864. doi: 10.1139/f75-110
Benke, A. C., and Wallace, J. B. (2003). Influence of wood on invertebrate communities in streams and rivers. Am. Fish. Soc. Symp. 37, 149–167. doi: 10.47886/9781888569568.ch8
Bramblett, R. G., Johnson, T. R., Zale, A. V., and Heggem, D. G. (2005). Development and evaluation of a fish assemblage index of biotic integrity for northwestern Great Plains streams. Trans. Am. Fish. Soc. 134, 624–640. doi: 10.1577/T04-051.1
Brown, C. R., Baxter, C., and Pashley, D. N. (2000). “The ecological basis for the conservation of migratory birds in the Mississippi Alluvial Valley,” in Strategies for Bird Conservation: the Partners in Flight Planning Process, eds R. Bonney, D. N. Pashley, R. J. Cooper, and L. Niles (Ogden: U.S. Forest Service, Rocky Mountain Research Station, RMRS-P-16), 4–7.
Bryant, M. D. (2010). Past and Present Aquatic Habitats and Fish Populations of the Yazoo-Mississippi Delta. Asheville, NC: US Department of Agriculture, Forest Service, Southern Research Station, General Technical Report SRS−130, 36.
Buchanan, T. M., and Robison, H. W. (2019). Fishes of Arkansas, 2nd Edn.. Fayetteville: University of Arkansas Press.
Burr, B. M., and Page, L. M. (1986). “Zoogeography of fishes in the lower Ohio-upper Mississippi basin,” in The Zoogeography of North American Fishes, eds C. H. Hocutt, and E. O. Wiley (New York, NY: Wiley), 287–325.
Chen, Y., Daniels, M., Reba, M., Bouldin, J., Henry, C., Daniel, P., et al. (2016). Agriculture in the Mississippi River basin: effects on water quality, aquatic biota, and watershed conservation. Am. Fish. Soc. Symp. 84, 293–309. doi: 10.47886/9781934874448.ch14
Clark, K. R., Rheannon, M. H., and Gurdak, J. J. (2011). Groundwater availability of the Mississippi embayment. Professional Paper 1785. Reston, VA: U. S. Geological Survey, 62.
Conner, J. V., and Sutkus, R. D. (1986). “Zoogeograpghy of freshwater fishes of the Western Gulf Slope,” in The Zoogeography of North American Fishes, eds C. H. Hocutt, and E. O. Wiley (New York, NY: Wiley), 413–456.
Cross, F. B., Mayden, R. L., and Stewart, J. D. (1986). “Fishes in the western Mississippi drainage,” in The Zoogeography of North American Fishes, eds C. H. Hocutt, and E. O. Wiley (New York, NY: Wiley-Interscience), 363–412.
Dardeau, T., Killgore, K. J., and Miller, A. C. (1995). “Using riprap to create or improve riverine Habitat,” in River, Coastal and Shoreline Protection: Erosion Control using Riprap and Armourstone, ed C. R. Thorne (Sussex: Wiley), 609–620.
Dembkowski, D. J., and Miranda, L. E. (2012). Hierarchy in factors affecting fish biodiversity in floodplain lakes of the Mississippi Alluvial Valley. Environ. Biol. Fishes 93, 357–368. doi: 10.1007/s10641-011-9923-y
Dembkowski, D. J., and Miranda, L. E. (2014). Environmental variables measured at multiple spatial scales exert uneven influence on fish assemblages of floodplain lakes. Hydrobiologia 721, 129–144. doi: 10.1007/s10750-013-1655-x
Dosskey, M. G., Bentrup, G., and Schoeneberger, M. (2012). A role for agroforestry in forest restoration in the Lower Mississippi alluvial valley. J. For. 110, 48–55. doi: 10.5849/jof.10-061
Emery, E. B., Simon, T. P., McCormick, F. H., Angermeier, P. L., Deshon, J. E., Yoder, C. O., et al. (2003). Development of a multimetric index for assessing the biological condition of the Ohio River. Trans. Am. Fish. Soc. 132, 791–808. doi: 10.1577/T01-076
Etnier, D. A., and Starnes, W. C. (1993). Fishes of Tennessee. Knoxville, TN: University of Tennessee Press.
Faulkner, S., Barrow, W. Jr., Keeland, B., Walls, S., and Telesco., D. (2011). Effects of conservation practices on wetland ecosystem services in the Mississippi Alluvial Valley. Ecol. Appl. 21, S31–S48. doi: 10.1890/10-0592.1
Ferguson, R., and Hoy, T. (2008). “Effects of tributaries on main-channel geomorphology,” in River Confluences, Tributaries and the Fluvial Network, eds S. P. Rice, A. G. Roy, and B. L. Roads (Chischester: Wiley), 183–208.
Fischer, R., and Fischenich, C. (2000). Design recommendations for riparian corridors and vegetated buffer strips. EMRRP Technical Notes, TN-EMRRP-SR-24. Vicksburg, MS: U.S. Army Engineer Research and Development Center, 17.
Flávio, H. M., Ferreira, P., Formigo, N., and Svendsen, J. C. (2017). Reconciling agriculture and stream restoration in Europe: a review relating to the EU Water Framework Directive. Sci. Total Environ. 576, 378–395. doi: 10.1016/j.scitotenv.2017.04.057
Fritz, K. M., Schofield, K. A., Alexander, L. C., McManus, M. G., Golden, H. E., Lane, C. R., et al. (2018). Physical and chemical connectivity of streams and riparian wetlands to downstream waters: a synthesis. J. Am. Water Resour. Assoc. 54, 323–345. doi: 10.1111/1752-1688.12632
Hanberry, B. B., Kabrick, J. M., He, H. S., and Palik, B. J. (2012). Historical trajectories and restoration strategies for the Mississippi River Alluvial Valley. Forest Ecol. Manag. 280, 103–111. doi: 10.1016/j.foreco.2012.05.033
Harding, J. S., Benfield, E. F., Bolstad, P. V., Helfman, G. S., and Jones, E. B. D. (1998). Stream biodiversity: the ghost of land use past. Proc. Natl. Acad. Sci. U. S. A. 95, 14843–14847. doi: 10.1073/pnas.95.25.14843
Hoover, J. J., and Killgore, K. J. (1998). “Fish communities,” in Southern Forested Wetlands, eds M. G. Messina, and W. H. Conner (Boca Raton, FL: Lewis Publishers), 237–260.
Hughes, R. M., and Vadas, R. L. Jr. (2021). Agricultural effects on streams and rivers: a western USA focus. Water 13:1901. doi: 10.3390/w13141901
Jester, D. B., Echelle, A. A., Matthews, W. J., Pigg, J., Scott, C. M., and Collins, K. D. (1992). The fishes of Oklahoma, their gross habitats, and their tolerance of degradation in water quality and habitat. Proc. Okla. Acad. Sci. U. S. A. 72, 7–19.
Jones, R. L., Slack, W. T., and Hartfield, P. D. (2005). The freshwater mussels (Mollusca: Bivalvia: Unionidae) of Mississippi. Southwest. Nat. 4, 77–92. doi: 10.1656/1528-7092(2005)004(0077:TFMMBU)2.0.CO;2
Kapitzke, R. (2010). Culvert Fishway Planning and Design Guidelines, Part H – Rock Ramp Fishways for Open Channels, Version 2. James Cook University School of Engineering and Physical Sciences, 40. Available online at: https://www.usgs.gov/centers/eros/science/national-land-cover-database (accessed January, 2021).
Killgore, K. J., Hoover, J. J., Murphy, C. E., Parrish, K. D., Johnson, D. R., and Myers, K. F. (2008). Restoration of Delta streams: a case history and conceptual model. EMRRP Technical Notes, EMRRP-ER-08. Vicksburg, MS: U.S. Army Engineer Research and Development Center.
King, S. L., and Keeland, B. D. (1999). Evaluation of reforestation in the Lower Mississippi River Alluvial Valley. Restor. Ecol. 7, 348–359. doi: 10.1046/j.1526-100X.1999.72029.x
Knight, A. T., Cowling, R. M., and Campbell, B. M. (2006). An operational model for implementing conservation action. Conserv. Biol. 20, 408–419. doi: 10.1111/j.1523-1739.2006.00305.x
Kroger, R., Perez, M., Walker, S., and Sharpley, A. (2012). Review of best management practice reduction efficiencies in the Lower Mississippi Alluvial Valley. J. Soil Water Conserv. 67, 556–563. doi: 10.2489/jswc.67.6.556
Kroll, S. A., and Oakland, H. C. (2019). A review of studies documenting the effects of agricultural best management practices on physiochemical and biological measures of stream ecosystem integrity. Nat. Areas J. 39, 58–77. doi: 10.3375/043.039.0105
Lane, C. R., Leibowitz, S. G., Autrey, B. C., LeDuc, S. D., and Alexander, L. C. (2018). Hydrological, physical, and chemical functions and connectivity of non-floodplain wetlands to downstream waters: a review. J. Am. Water Resour. Assoc. 54, 346–371. doi: 10.1111/1752-1688.12633
Langland, M. J., Duris, J. W., Zimmerman, T. M., and Chaplin., J. J. (2020). Effects of legacy sediment removal on nutrients and sediment in Big Spring Run, Lancaster County, Pennsylvania, 2009–15. Reston: U.S. Geological Survey Scientific Investigations Report 2020-5031.
Larned, S. T., Datry, T., Arscott, D. B., and Tochner, K. (2009). Emerging concepts in temporary-river ecology. Freshw. Biol. 55, 717–738. doi: 10.1111/j.1365-2427.2009.02322.x
Lasne, E., Lek, S., and Laffaille, P. (2007). Patterns in fish assemblages in the Loire floodplain: the role of hydrological connectivity and implications for conservation. Biol. Conserv. 139, 258–268. doi: 10.1016/j.biocon.2007.07.002
Lenhart, C. F., Smith, D. J., Lewandowski, A., Belmont, P., Gunderson, L., and Nieber, J. L. (2018). Assessment of stream restoration for reduction of sediment in a large agricultural watershed. J. Water Resour. Plan. Manag. 144:908. doi: 10.1061/(ASCE)WR.1943-5452.0000908
Lovisa, L., Hasselquista, E. M., and Laudona, H. (2019). Towards ecologically functional riparian zones: a meta-analysis to develop guidelines for protecting ecosystem functions and biodiversity in agricultural landscapes. J. Environ. Manag. 249:109391. doi: 10.1016/j.jenvman.2019.109391
Margoluis, R., Stern, C., Salafsky, N., and Brown, M. (2009). Using conceptual models as a planning and evaluation tool in conservation. Eval. Program Plann. 32, 138–147. doi: 10.1016/j.evalprogplan.2008.09.007
Mathers, K., Doretto, A., Fenoglio, S., and Wood, P. J. (2022). Temporal effects of fine sediment deposition on benthic macroinvertebrate community structure, function and biodiversity likely reflects landscape setting. Sci. Total Environ. 829:154612. doi: 10.1016/j.scitotenv.2022.154612
McMahon, P., Beauchamp, V. B., Casey, R. E., Salice, C. J., Bucher, K., Marsh, M., et al. (2021). Effects of stream restoration by legacy sediment removal and floodplain reconnection on water quality. Environ. Res. Lett. 16, 1–13. doi: 10.1088/1748-9326/abe007
Miranda, L. E., Andrews, C. S., and Kroger, R. (2014). Connectedness of land use, nutrients, primary production, and fish assemblages in oxbow lakes. Aquat. Sci. 76, 41–50. doi: 10.1007/s00027-013-0310-y
Miranda, L. E., Rhodes, M. C., Allen, Y., and Killgore, K. J. (2021). An inventory and typology of permanent floodplain lakes in the Mississippi Alluvial Valley: a first step to conservation planning. Aquat. Sci. 83:20. doi: 10.1007/s00027-020-00775-3
Naden, P. S., Murphy, J. F., Olda, G. H., Newman, J., Scarlett, P., Harman, M., et al. (2016). Understanding the controls on deposited fine sediment in the streams of agricultural catchments. Sci. Total Environ. 547, 366–381. doi: 10.1016/j.scitotenv.2015.12.079
NLCD (2016). U.S. Geological Survey National Land Cover Database 2016. National Landcover Database. Available online at: https://safe.menlosecurity.com/doc/docview/viewer/docN23E9DD66A219a9983a342d09486f0aeb0e41852d20106955d2f64e40c7bfae4e6e057f3592a8 (accessed Septamber, 2023).
Oswalt, S. N. (2013). Forest resources of the Lower Mississippi Alluvial Valley. General Technical Report SRS-177. Asheville, NC: U.S. Department of Agriculture Forest Service, Southern Research Station, 29.
Paller, M. H., Reichert, M. J. M., and Dean, J. M. (1996). Use of fish communities to assess environmental impacts in South Carolina Coastal Plain streams. Trans. Am. Fish. Soc. 125, 633–644. doi: 10.1577/1548-8659(1996)125<0633:UOFCTA&>2.3.CO;2
Poff, N. L., Allan, J. D., Bain, M. B., Karr, J. R., Prestgaard, K. L., Richter, B. D., et al. (1997). The natural flow regime: a paradigm for river conservation and restoration. BioScience 47, 769–784. doi: 10.2307/1313099
Rabeni, C. F. (1995). Effects of siltation on stream fishes and the potential mitigating role of the buffering riparian zone. Hydrobiologia 303, 211–219. doi: 10.1007/978-94-017-3360-1_20
Reba, M. L., Massey, J. H., Adviento-Borbe, M. A., Leslie, D., Yaeger, M. A., Anders, M., et al. (2017). Aquifer deple-tion in the lower Mississippi River Basin: Challenges and solutions. JCWRE 162, 128–139. doi: 10.1111/j.1936-704X.2017.03264.x
Rhodes, R. (2004). John James Audubon, the Making of an American. New York, NY: Alfred A. Knopf Publishers.
Robison, H. W. (1986). “Zoogeographic implications of the Mississippi River basin,” in The Zoogeography of North American Fishes, eds C. H. Hocutt, and E. O. Wiley (New York, NY: Wiley-Interscience), 267–286.
Sanderson, E. W., Redford, K. H., Vedder, A., Coppolillo, P., and Ward, S. E. (2002). A conceptual model for conservation planning based on landscape species requirements. Landsc. Urban Plan. 58, 41–56. doi: 10.1016/S.0169-2046(01)00231-6
Saucier, R. T. (1994). Geomorphology and quaternary geologic history of the Lower Mississippi Valley. Vicksburg, MS: U. S. Army Engineer Waterways Experiment Station, 414.
Schlosser, I. J. (1985). Flow regime, juvenile abundance, and the assemblage structure of stream fishes. Ecology 66, 1484–1490. doi: 10.2307/1938011
Schlosser, I. J. (1991). Stream fish ecology: a land- scape perspective. BioScience 41, 704–712. doi: 10.2307/1311765
Schlosser, I. J., and Karr, J. R. (1981). Riparian vegetation and channel morphology impact on spatial patterns of water quality in agricultural watersheds. Environ. Manage. 5:233–243 doi: 10.1007/BF01873282
Schoenholtz, S. H., James, J. P., Kaminski, R. M., Leopold, B. D., and Ezell, A. W. (2001). Afforestation of bottomland hardwoods in the Lower Mississippi alluvial valley: status and trends. Wetlands 21, 602–613. doi: 10.1672/0277-5212(2001)021(0602:AOBHIT02.0.CO;2
Schramm, H. L., Hatch, J. T., Hrabik, R. A., and Slack, W. T. (2016). Fishes of the Mississippi River. Am. Fish. Soc. Symp. 84, 53–57. doi: 10.47886/9781934874448.ch3
Schürings, C., Feld, C. K., Kail, J., and Hering, D. (2022). Effects of agricultural land use on river biota: a meta-analysis. Environ. Sci. Europe 34:124. doi: 10.1186/s12302-022-00706-z
Stanturf, J. A. (2006). Can we bring back Faulkner's Big Woods? Compass Issue 6. Asheville, NC: Southern Research Station, USDA Forest Service, 1-5.
Stanturf, J. A., Gardiner, E. S., Hamel, P. B., Devall, M. S., Leininger, T. D., and Warren, M. E. (2000). Restoring bottomland hardwood ecosystems in the Lower Mississippi Alluvial Valley. J. For. 98, 10–16. doi: 10.1093/jof/98.8.10
Sutherland, A. B., Meyer., J. L, and Gardiner., E. P. (2002). Effects of land cover on sediment regime and fish assemblage structure in four southern Appalachian streams. Freshw. Biol. 47, 1791–1805. doi: 10.1046/j.1365-2427.2002.00927.x
Tarr, N. M. (2019). Demonstrating a conceptual model for multispecies landscape pattern indices in landscape conservation. Landsc. Ecol. 34, 2133–2147. doi: 10.1007/s10980-019-00888-7
Tockner, K., Bunn, S. E., Gordon, C., Naiman, R. J., Quinn, G. P., and Standford, J. A. (2008). “Flood plains: critically threatened ecosystems,” in Aquatic Ecosystems, ed N. V. C. Polunin (Cambridge: Cambridge University Press), 45–61.
Wang, L., Lyons, J., and Kanehl, P. (2002). Effects of watershed best management practices on habitat and fish in Wisconsin streams. J. Am. Water Resour. Assoc. 38, 663–680. doi: 10.1111/j.1752-1688.2002.tb00988.x
Keywords: restoration, E-flows, sediment management, riparian buffers, fish diversity
Citation: Killgore KJ, Hoover JJ, Miranda LE, Slack WT, Johnson DR and Douglas NH (2024) Fish conservation in streams of the agrarian Mississippi Alluvial Valley: conceptual model, management actions, and field verification. Front. Freshw. Sci. 2:1365691. doi: 10.3389/ffwsc.2024.1365691
Received: 04 January 2024; Accepted: 31 January 2024;
Published: 16 February 2024.
Edited by:
James E. Garvey, Southern Illinois University Carbondale, United StatesReviewed by:
Laura Davalos-Lind, Baylor University, United StatesMustafa Ceylan, Isparta University of Applied Sciences, Türkiye
Copyright © 2024 Killgore, Hoover, Miranda, Slack, Johnson and Douglas. This is an open-access article distributed under the terms of the Creative Commons Attribution License (CC BY). The use, distribution or reproduction in other forums is permitted, provided the original author(s) and the copyright owner(s) are credited and that the original publication in this journal is cited, in accordance with accepted academic practice. No use, distribution or reproduction is permitted which does not comply with these terms.
*Correspondence: Kenneth J. Killgore, SmFjay5raWxsZ29yZSYjeDAwMDQwO3VzYWNlLmFybXkubWls