- 1Department of Plant and Environmental Sciences, Clemson University, Charleston, SC, United States
- 2Integral Consulting, Cary, NC, United States
- 3Environmental Science and Studies, Department of Biological Sciences, Towson University, Towson, MD, United States
It is widely recognized that populations of freshwater aquatic organisms are faced with a myriad of co-occurring stressors. These likely include manufactured chemicals, stressors due to climate change, habitat alterations, water quality parameters, etc. Importantly, these stressors are superimposed over “natural” stressors such as density of conspecifics. Density effects, in particular, are important and can result from resource competition or crowding; here we define crowding as high density but without resource limitation. Crowding has received less research attention despite its potential ecological importance and frequency of occurrence. In larval mosquitoes, for example, both physical and chemical components are important effects of crowding, which result in increased mortality, prolonged development, and reduced size. The objective of this research was to determine how different crowding conditions would affect subsequent insecticide sensitivity using the yellow-fever mosquito, Aedes aegypti. We hypothesized that stress due to crowding in the larval stage would increase insecticide sensitivity. Results showed that when larvae were reared at various crowding densities (without resource competition) but later exposed to a contact insecticide (permethrin) at equal densities they exhibited similar sensitivity. However, when larvae were reared at equal densities but exposed at various crowding densities there appeared to be a protective effect of crowding, as more densely crowded larvae were significantly less sensitive to the insecticide. Possible mechanisms for this protective effect were investigated. Induced detoxification enzymes do not appear to be a factor, but density-modified larval exposure is likely a mechanism. This research provides important insights into how mosquitoes may respond to control efforts as well as providing empirical recommendations on designing laboratory toxicity tests to better reflect ecological conditions in natural mosquito populations.
1 Introduction
It is well-recognized that naturally occurring populations of freshwater organisms are exposed to multiple, co-occurring stress factors (Ormerod et al., 2010 and related articles; Birk et al., 2020). Anthropogenic stressors that have received considerable attention include a wide array of manufactured chemicals (e.g., Busch et al., 2016), increasing salinity (Kaushal et al., 2021), altered water quality (Wantzen and Mol, 2013), and multiple factors related to climate change (Woodward et al., 2010). Importantly, these anthropogenic stress factors are operating in conjunction with inherent stress factors critical to population dynamics such as predator-prey interactions and density or resource effects. This multi-stressor environment has important implications for understanding, predicting and managing impacts to freshwater systems (Spears et al., 2021). An interesting subset of studies exploring impacts of multiple stressors can also have important implications for direct effects to humans. This is especially the case when mosquito species are the subject of multi-stressor research. Studies involving mosquito larvae have utility in advancing our understanding of stress ecology while also providing insights to facilitate management applications.
The Aedes aegypti mosquito is an important vector for diseases such as yellow fever, dengue, and chikungunya. Because of its public health importance, global efforts have been undertaken to control both larvae and adults. Understanding population dynamics of disease vector mosquitoes is essential in controlling mosquito populations as well as in assessing the impact of control efforts (Juliano, 2007; White et al., 2011). Like many aquatic invertebrates, for the larval (aquatic) stage of mosquitoes, density-dependent competition plays a central role in regulating populations by influencing survival (Maciá, 2009), development (Barbosa et al., 1972), adult size (Renshaw et al., 1994), egg hatching (Livdahl et al., 1984), female fecundity (Moore and Fisher, 1969), mating competitiveness (Ng'habi et al., 2005), dispersal capabilities (Mori, 1979), adult longevity (Hawley, 1985) and even oviposition behavior of gravid females (Wasserberg et al., 2014).
Density effects are often driven by competition for food but can also be caused by other physical and chemical factors (Roberts and Kokkinn, 2010), the latter having received less research attention despite its potential ecological importance. Density effects from high larval density can result from physical interference among larvae that disrupts normal feeding behavior (Roberts, 1998) or chemical effects such as accumulated chemical waste products (Bédhomme et al., 2005) and growth-regulating factors (Ikeshoji and Mulla, 1970; Silberbush et al., 2014). These chemical factors can be strain-specific (Dye, 1984) or species-specific (Silberbush et al., 2014). Chemical factors produced in crowded conditions have been shown to increase mortality, cause toxicity to 1st-instar larvae, delay or increase development rate, and produce morphological aberrations (Moore and Fisher, 1969; Ikeshoji and Mulla, 1970; Broadie and Bradshaw, 1991; Roberts, 1998; Silberbush et al., 2014). Here, we use “crowding” to describe the conditions whereby individuals are at high density but resources are not limited. We would expect under crowded conditions, that other stress responses (besides competition for resources) would manifest.
Based on the evidence for physically and chemically-driven crowding effects, it can be argued that larval crowding (without food limitation) can be a significant environmental stressor. In natural mosquito populations, sub-optimal conditions and environmental stress are common to most organisms and can include heat stress, freezing temperatures, desiccation, oxygen depletion, starvation, and pathogen exposure (Holmstrup et al., 2010). Environmental stress can also have ecological sources such as predation (Uitregt et al., 2012) and competition (Bedhomme et al., 2003). Furthermore, pesticide toxicity can be modified by co-occurring environmental and ecological factors such as temperature (Muturi et al., 2011), competition (Foit et al., 2012), predation (Relyea, 2003; Qin et al., 2011), pH (Lohner and Fisher, 1990), and resource conditions (Campero et al., 2008). Although the interaction of density and pesticide toxicity has been studied for decades (e.g., Forbes et al., 2001) it is still recognized as critically important for understanding and predicting effects of stressors at the population level (e.g., Accolla et al., 2021). Interestingly, the results of studies exploring the interaction of organismal density and toxicant exposure are varied and, thus far, have focused on resource competition as the density stress with release from competition as a driving factor for the results (Relyea and Hoverman, 2006). In contrast, we are not aware of any studies that have explored the interaction of crowding and pesticide toxicity in aquatic invertebrates.
There are a few existing studies that explore the influence of density on pesticide toxicity in mosquitoes, specifically. The efficacy of Bti, a widely used bacterial insecticide that must be ingested to activate, decreases with increasing larval density (Becker et al., 1992); presumably because it's availability can be limited similar to food. Contact insecticides, such as the organophosphate malathion, have decreased efficacy with increasing larval densities. This result only occurred at the high nutrient level and was most likely attributable to release from competition among survivors (Muturi et al., 2010). Contact insecticides—such as organophosphates, pyrethroids, and neonicotinoids—work by being absorbed through the cuticle and then interacting with nerve cells (Welling, 1977). Tolerance to contact insecticides can be modified by reduced exposure (Nkya et al., 2013) or increased metabolic detoxification (Hemingway et al., 2004).
Effects from larval crowding can manifest in at least two ways, which we describe as latent and active crowding. Latent crowding effects are those that have their origins in early development but are exhibited later in development (Pechenik, 2006). Specifically, to investigate latent crowding effects, newly hatched mosquito larvae could be reared and developed at varying densities prior to being exposed to an insecticide at equal larval densities. To investigate active crowding effects, larvae could be reared and developed at a single density prior to being distributed into different densities and exposed to the insecticide. The only other study we are aware of to test interacting effects of crowding and insecticide exposure was Edwards (1981), who found that cockroaches that were crowded during insecticide exposure were more sensitive than those exposed individually, possibly from the convulsions of one individual triggering convulsions in nearby individuals.
The overarching objective of this study was to determine how the environmental stress of different crowding conditions could modify permethrin sensitivity in Aedes aegypti larvae. We hypothesized that the physical and chemical factors of larval crowding, both latent and active, would increase sensitivity to permethrin, a contact insecticide. Additionally, the mechanism behind how crowding may modify toxicity was explored and the relationship between density and survival was modeled.
2 Materials and methods
2.1 Mosquitoes and chemicals
Larvae for experiments were obtained from a laboratory strain of Aedes aegypti (Liverpool strain) reared in standard insectary conditions (25 ± 1°C, 60–70% RH, photoperiod 14:10 L:D including 30 min of simulated dawn and 30 min of simulated dusk). Adult mosquitoes were bloodfed adult bovine blood (1:1 whole blood: alsever's solution; Lampire Biological Laboratories, USA) at least once a week and provided a 10% sugar solution ab libitum. Larvae used in experiments were hatched at 25°C from egg cards in plastic containers with ~1,500 mL moderately hard water (60 μg/l CaSO4, 60 μg/l, MgSO4, 4 μg/l, KCl, 98 μg/l NaHCO3; hereafter referred to as lab water). A pinch of yeast was added to reduce water oxygen content to induce hatching. Less than 24 h after hatching, larvae were distributed according to the specific experimental design (i.e., latent- or active-crowding effects, described in detail below) and experiments were conducted in an incubator at 25°C and a photoperiod of 12:12 (L:D).
The insecticide permethrin (98.3%; 26.7% cis-isomer, 71.6% trans-isomer) and the synergist piperonyl butoxide (99.0%) were both obtained from Sigma-Aldrich, Inc. (St. Louis, MO). Permethrin was used as a surrogate for the relevant contact insecticide class of pyrethroids and also has similar partitioning characteristics to temephos, a widely used organophosphate larvicide (Noble, 1993). Stock solutions of each were made with an acetone vehicle and stored at 5°C. Before beginning each bioassay, each stock solution was diluted in DI water to produce working solutions.
2.2 Experiment 1: latent crowding effects
Experiment 1 occurred in two phases. In the first phase, 1st-instar larvae were reared at different densities in the absence of pesticide. Once larvae reached the 4th-instar, they were then exposed to pesticides at equal densities. This design was chosen to evaluate effects of density during early development on subsequent pesticide sensitivity under low density conditions; these represent so-called latent effects (Pechenik, 2006).
The first phase of the experiment was initiated with larvae <24 h after hatching. 1st-instar larvae were distributed into containers representing three different densities, a “low” density of approximately 60 larvae in 300 mL lab water (0.20 larvae/mL), a “medium” density of approximately 175 larvae in 300 mL lab water (0.58 larvae/mL), and a “high” density” of approximately 250 larvae in 300 mL lab water (0.83 larvae/mL). Four replicates were used in both the medium and high-density treatments while five replicates were used in the low density treatment to ensure sufficient numbers of larvae for the bioassay. Larvae were provided abundant food consisting of ground TetraFin® goldfish flakes and a full water change was performed every day to maintain water quality. The feeding regime ensured larval crowding, whereby there was competition for space but not food.
After the majority of larvae in treatments developed to 4th-instar, the replicate rearing containers for each treatment were combined (within treatment) and 4th-instar larvae randomly selected for the second phase of the experiment involving pesticide exposure. All 4th-instar larvae from phase 1 were then included in a bioassay at the same larval density of 10 larvae in 100 mL lab water (0.1 larvae/mL). For each of the three density treatments in phase 1, the bioassays consisted of six concentrations (including one control) with four replicates. The concentrations used were 0.0, 0.5, 1.0, 1.5, 2.0, and 3.0 ppb permethrin. At 24-h, survival was recorded. Controls were provided an amount of acetone equivalent to the acetone concentration of the highest concentration permethrin exposure concentration and mortality never exceeded 8%, which is well below acceptable levels (generally, 20% or less). Larvae were not fed during phase 2 bioassays to avoid sorption of permethrin to food.
After each bioassay was initiated, 30- 4th-instar larvae from each of the three combined rearing density containers were removed and placed in emergence chambers to determine emergence rate and adult dry mass. Larvae were provided abundant ground TetraFin® goldfish flakes until emergence. Within 24-h of adults emerging, they were removed from the emergence chamber and stored at −25°C until all adults emerged. Adults were dried at 60°C for 48-h and then weighed to determine if larval rearing conditions had a significant impact on adult mosquito mass. Mosquito dry mass was measured using a Thermo Scientific Orion Cahn® C-33 microbalance.
2.3 Experiment 2: active crowding effects
Experiment 2 also occurred in two phases. First-instar larvae in this experiment were reared at equal initial densities but then exposed to pesticide at varying larval densities to determine if there was an interaction between concomitantly occurring density and pesticide exposure. In this design, any latent effects of different rearing densities (Experiment 1) were removed as potential factors.
Less than 24 h after hatching, Phase 1 of Experiment 2 was initiated with 1st-instar larvae that were evenly distributed into six new plastic containers each with ~340 larvae and 1,500 mL lab water (0.23 larvae/mL). Larvae were provided abundant food consisting of ground TetraFin® goldfish flakes and a full water change was performed every other day to maintain water quality. After the majority of larvae developed to 4th-instar, the separate rearing containers were combined and 4th-instar larvae were randomly selected for Phase 2 of Experiment 2.
In Phase II, the larvae from Phase I were separated into three different densities: a “low” density of 10 larvae in 250 mL lab water (0.04 larvae/mL); a “medium” density of 10 larvae in 100 mL lab water (0.1 larvae/mL); and a “high” density of 10 larvae in 25 mL lab water (0.4 larvae/mL). Three simultaneous toxicity bioassays were performed, one for each density, and each consisted of six concentrations of permethrin ranging from 0.0 ppb (control) to 4.5 ppb with four replicates. Based on the results of earlier pilot experiments in which density affected insecticide sensitivity, each bioassay included a specific range of concentrations in order to generate robust survival curves and toxicity estimates. For the low density, the bioassay concentrations were 0.0, 0.2, 0.35, 0.6, 1.0, 1.9, and 3.0 ppb permethrin. For the medium density, the bioassay concentrations were 0.0, 0.7, 1.0, 1.4, 1.9, 2.8, and 4.0 ppb permethrin. For the high density, the bioassay concentrations were 0.0, 1.0, 1.35, 1.9, 2.5, 3.35, and 4.5 ppb permethrin. At 24-h, survival and development were recorded. Larvae were not fed during the bioassays to avoid adsorption of permethrin to food.
A control was used for each density and was provided an amount of acetone equal to the highest permethrin exposure concentration. Control mortality never exceeded 5%.
2.4 Experiment 3: active crowding protective effects: role of metabolic tolerance?
The results from Experiments 2 indicated there was a protective effect of crowding in terms of pesticide sensitivity, a somewhat surprising result that we successfully repeated and confirmed (data not shown). To better understand a possible mechanism providing the protective effect of active larval crowding, a comparative toxicity test using piperonyl butoxide (PBO)—a synergist that inhibits cytochrome P-450 oxidase (CYP450)—was used to determine if induced detoxifying enzymes played a significant role in increasing insecticide tolerance for crowded larvae. CYP450s are an important enzyme class involved in metabolism of xenobiotics and pesticides and are one of the major mechanisms of insecticide resistance in insects (Hardstone et al., 2007). Expression and enzyme activity of CYP450 is induced by exposure to a wide-range of chemicals (Scott, 1999).
The experimental procedure was the same as for Experiment 2: Active Crowding Effects described above except that 1.5 ppm PBO was added to each treatment plus the controls and the permethrin concentrations were similar. For the low density treatment, the permethrin concentrations were 0.0, 0.05, 0.1, 0.17, 0.3, 0.6, and 1.0 ppb; for the medium density treatment, permethrin concentrations were 0.0, 0.01, 0.2, 0.4, 0.8, 1.1, and 1.5 ppb; for the high density treatment, the concentrations were 0.0, 0.25, 0.4, 0.6, 0.9, 1.3, and 2.0 ppb. At 24-h, survival and development were recorded. Controls were provided an amount of acetone equal to what the highest concentration permethrin exposure received and mortality never exceeded 1%. Larvae were not fed during the bioassay.
A synergism ratio (SR50) was determined for each density to better relate the role CYP450s play in the protective effect of non-latent larval crowding. Synergism ratios were obtained by calculating the ratio between each density's LC50 value with and without PBO. A larger SR50 value indicates a larger role for CYP450 metabolism in a density treatment (Riaz et al., 2013).
2.5 Experiment 4: characterizing the density-survival relationship
Larvae at a range of different densities were exposed to two concentrations of permethrin to understand and characterize the relationship between larval density and survival. The higher number of density treatments and fewer permethrin exposure concentrations allowed us to create more robust density-dependent relationships.
Larvae were reared to 4th-instar as described above in Experiment 2: Active Crowding Effects. Once at 4th-instar, larvae were separated into eight densities and exposed to a concentration of 1.5 or 2.0 ppb permethrin. These concentrations were chosen because they permitted sufficient survival/mortality for a wide range of densities. Each glass container had ten larvae and the volumes of 1.5 and 2.0 ppb permethrin solutions were varied to achieve densities of 2.0, 1.0, 0.71, 0.50, 0.29, 0.20, 0.10, and 0.05 larvae/mL. Each larval density treatment had four replicates and a control was used for densities 2.0, 0.29, and 0.05 larvae/mL, which corresponded to a low, medium, and high density. Controls were provided an amount of acetone equal to the highest permethrin exposure concentration and mortality never exceeded 1%. At 24-h, survival and development were recorded.
2.6 Statistical analysis
The R 3.0.2 statistical programming environment (R Core Team, 2023) was used for all analyses and alpha equaled 0.05. Dose-response curves were created using a log-logistic function. Effect levels (e.g., LC50) along with corresponding 95% confidence intervals and standard errors were obtained using the drm() function in R within the drc package (Ritz and Streibig, 2005). Dose-response curves and LC50 values were compared using 95% confidence intervals and standard errors. Lack of an overlap in 95% confidence intervals or lack of an overlap in standard errors indicated that any observed differences in LC50 values were significantly different (Payton et al., 2003). SR50 values were also compared using standard error overlap.
Survival differences within latent crowding treatment groups at 1.00ppb were analyzed by one-way analysis of variance (ANOVA), with density as a factor. Adult mass was also analyzed using ANOVA, with density as a factor. Post hoc analysis for all ANOVAs included Tukey's HSD. Response variables were assessed for assumptions of normality and homogeneity of variances prior to analysis. Results were considered significant at the p ≤ 0.05 level.
3 Results
3.1 Latent crowding effects
In experiment 1: latent crowding effects, crowding early in development did not significantly impact permethrin sensitivity, despite increased rearing densities resulting in significantly decreased adult mass. LC50 values, 95% confidence intervals, and standard errors were calculated for each rearing density. LC50 values for the rearing densities 0.19, 0.59, and 0.84 larvae/mL were 1.237, 1.391, and 1.485 ppb, respectively. Overlapping 95% confidence intervals are typically used to determine if values are significantly different from each other, but overlapping standard errors can be used and is more stringent, although overly conservative (Payton et al., 2003). 95% confidence intervals overlapped for all three latent rearing densities, indicating similar LC50 values for each (Table 1). The dose-response curve for the low rearing density (0.19 larvae/ml) showed an unusual relationship, where at low permethrin concentrations there was less survival compared to the medium and high densities. However, at high permethrin concentrations there was higher survival in the low rearing density compared to the medium and high densities (Figure 1). LC50 values between the rearing densities were not significantly different from each other, but at 1.00 ppb permethrin the low rearing density had lower survival than the medium and high rearing density treatments (F2,27 = 7.923, p < 0.05).
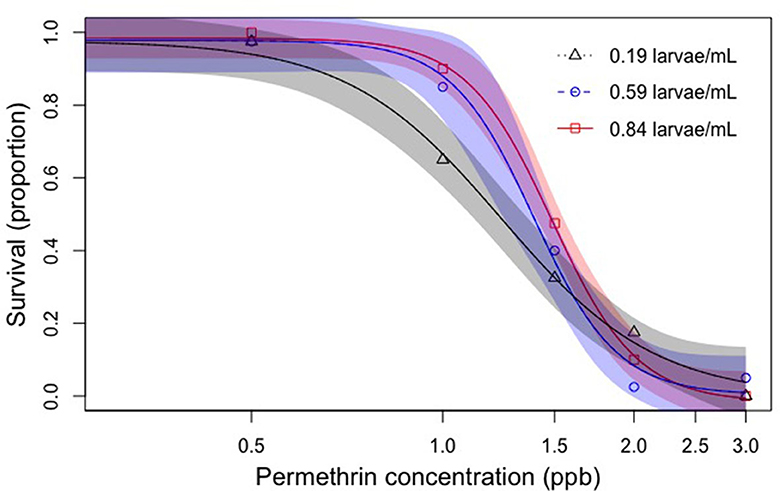
Figure 1. Effects of latent crowding on insecticide sensitivity. A dose-response curve and 95% confidence intervals were generated for each of the three rearing densities exposed to permethrin and LC50 values were calculated. Standard error values and 95% CI for each LC50 value (Table 1) were calculated. Confidence intervals overlapped for each rearing density LC50. Standard errors overlapped for the medium and high densities indicating no significant difference in permethrin sensitivity (p > 0.05). At the 1.0 ppb permethrin concentration the low rearing density had significantly less survival than the medium and high rearing densities (F2, 27 = 7.923, p < 0.05). See Table 1 for specific values.
At permethrin concentrations ≤1.5 ppb there was an apparent trend in that mosquito larvae at the higher rearing density showed an increased tolerance to subsequent permethrin exposure, but this trend did not hold for concentrations ≥1.5 ppb.
Latent effects of different larval rearing densities resulted in significant impacts on adult mass (Figure 2). Female mosquitoes reared at high densities (0.84 larvae/mL) weighed significantly less than larvae reared at low densities (0.19 larvae/mL) (F2,27 = 6.539, p = 0.004). Male mosquitoes reared at high densities (0.84 larvae/mL) weighed significantly less than male mosquitoes reared at medium densities (0.59 larvae/mL) (F2,21= 4.546, p = 0.013).
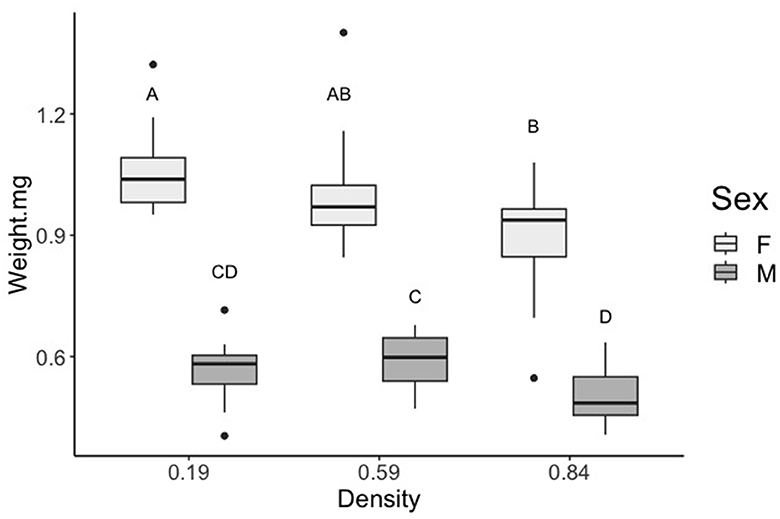
Figure 2. Effect of rearing density on adult dry mass. Rearing densities used to determine latent crowding effects had a significant impact on adult mass. Means with different letters are significantly different (Tukey's HSD, p < 0.05). Standard error bars are shown.
3.2 Active crowding effects
The active crowding effects described here included larvae reared from 1st-instar at equal densities (0.22 larvae/mL) in the absence of permethrin. When larvae developed to 4th-instar, they were divided into a low, medium, and high density treatment (0.04, 0.1, 0.4 larvae/mL, respectively) before being exposed to permethrin. A 24-h bioassay was conducted to determine dose-response curves (Figure 3) and LC50 values (Table 1). LC50 values for the exposure densities 0.04, 0.1, 0.4 larvae/mL were 0.928, 1.196, and 2.484 ppb, respectively. We found that active crowding effects had a significant impact on permethrin sensitivity and that increasing larval density resulted in increased larval tolerance (Figure 3). The 95% confidence intervals for each of the low, medium, and high densities did not overlap indicating significant differences (Payton et al., 2003).
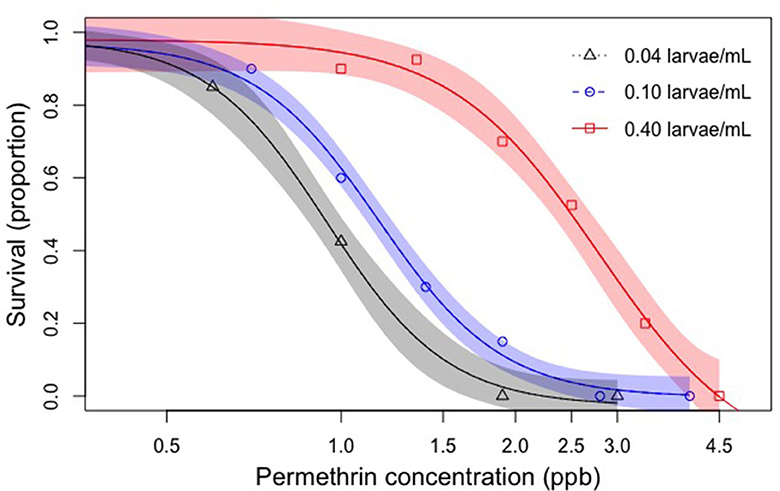
Figure 3. Effects of active crowding on insecticide sensitivity. A dose-response curve and associated 95% confidence intervals were generated for each of the three non-latent exposure densities and LC50 values for permethrin exposure were calculated. 95% C.I. for each LC50 values were calculated (Table 1). There was no overlap between any of the 95% CI values, indicating that permethrin sensitivities were significantly different between all three non-latent crowding densities (p < 0.05) (Payton et al., 2003). See Table 1 for specific values.
3.3 Active crowding protective effects: eliminating the possible role of metabolic tolerance
To investigate a potential mechanism for the protective effect of non-latent crowding, the synergist piperonyl butoxide (PBO) was used to inhibit and assess the role of CYP450 detoxifying enzymes in permethrin toxicity. A 24-hr bioassay was conducted similar to the previous non-latent crowding experiment, except for the addition of PBO. LC50 values for the permethrin+PBO exposure densities 0.04, 0.1, 0.4 larvae/mL were 0.465, 0.608, 1.143 ppb, respectively. A synergism ratio (SR50) was obtained for each density by calculating the ratio between LC50 values with and without PBO and the SR50 values were compared to determine the role of CYP450s among density treatments (Table 2). We found that the synergism ratios among density treatments were not significantly different from each other and that standard error values overlapped for each SR50 (Payton et al., 2003).
3.4 Modeled density-survival relationship
To better understand the relationship between larval density and survival, and to facilitate eventual incorporation into population dynamics models, a mathematical relationship was generated. The density-survival relationship for 1.5 ppb was similar as for 2.0 ppb permethrin (except for slightly more survival overall).
3.5 Toxicity under a range of larval densities
To provide additional context for the observed results and our argument that density is an important consideration when conducting toxicity studies, we conducted a simple experiment where 4 densities of larval mosquitoes were exposed to the same 2.0 ppb permethrin. The four densities were in units of larvae/ml: 30/360, 10,100, 20/100, and 25/50. Larvae were reared to 4th-instar and separated into the four different density treatments with four replicates per treatment. After addition of 2.0 ppb permethrin, 24-hr survival was recorded. Data were log transformed and a one-way analysis of variance and a Tukey post hoc test were used to compare proportion survival across treatments.
4 Discussion
We examined the effects of two different crowding conditions on pesticide sensitivity, latent and active, on Aedes aegypti larvae and hypothesized that increased crowding would increase sensitivity to a contact insecticide, permethrin. Latent crowding effects were those where density effects were present early in development but not during permethrin exposure and active crowding effects were those where crowding was only present at the time of permethrin exposure. Contrary to our hypothesis, we found no significant difference among permethrin sensitivity for latent crowding and decreased permethrin sensitivity in actively crowded larvae. Actively crowded larvae displayed a very strong protective effect and increased crowding resulted in increased pesticide tolerance. Induced metabolic detoxification was investigated as a possible mechanism for the protective effect but proved to be an unlikely source of the increased tolerance. This is among the only studies to investigate the role that crowding plays in toxicant sensitivity and yielded some interesting and unexpected insights.
Environmental stressors such as temperature, pH, resource conditions, competition and predation are common occurrences to most organisms and have been found to modify pesticide toxicity when they co-occur (e.g., Holmstrup et al., 2010; Qin et al., 2011). Non-food limiting larval-crowding is likely an important environmental stressor for treehole mosquitoes that breed in small, ephemeral pools and other aquatic species (invertebrates, fish, amphibians) because of the physical and chemical factors that alter significant life history traits such as development rate and survival (Moore and Fisher, 1969; Ikeshoji and Mulla, 1970; Broadie and Bradshaw, 1991; Roberts, 1998; Silberbush et al., 2014).
Despite no significant difference among acute toxicity in the three latent crowding treatments, the fitness-related effects of latent crowding were evident, as manifested in reduced adult dry mass. Increased latent rearing densities resulted in a significant reduction in adult mass for both male and female mosquitoes (Figure 2). The effects of intraspecific competition on adults have been described previously (Haramis, 1983; Renshaw et al., 1994; Reiskind and Lounibos, 2009) and were sex-specific (Bedhomme et al., 2003). Male and females each maintain the more important life-history trait, which is development rate for males and body size for females. Our result, that females had decreased body size with increased density, indicates that females were negatively impacted by crowding despite ample food, but were unaffected in terms of insecticide sensitivity.
While the effects of latent crowding on sensitivity overall were minimal, at a single, low concentration (1.00 ppb) the low rearing density treatment was more sensitive to permethrin than the medium and high densities. This result was related to the flatter dose-response curve of the low rearing density treatment. This curve intersected the other two curves, with mosquitoes from the low rearing treatment showing greater sensitivity to permethrin at lower concentrations but less sensitivity at higher concentrations. This is a peculiar response but the lack of significant differences indicates that additional experiments are needed to truly understand what, if any effect, is occurring. Future work should incorporate a wider range and greater number of latent rearing densities, especially at a lower density range (0.05–0.40 larvae/ml) to better understand the subtle underlying factors that may be impacting permethrin sensitivity for latent crowded larvae.
In the active crowding experiment, sensitivities to permethrin were significantly different among different density treatments. Increasing larval density during exposure resulted in higher pesticide tolerance. This result was unanticipated. If active crowding was, in fact, a stressor then insecticide sensitivity would be expected to increase with increased crowding, as seen in other reports of multiple stressors and synergism (e.g., Holmstrup et al., 2010). Instead, the opposite was observed, indicating a strongly protective effect of crowding in the presence of pesticide exposure. To better understand the relationship between active crowding and pesticide tolerance, the mechanism driving this protective effect should be further explored. Two possibilities include an induction of protective enzymes associated with crowding or a diminishment in per-individual exposure to insecticide (a “dilution” effect).
We empirically explored one possible mechanism supporting the protective effect of larval crowding by testing whether there was an increased role of CYP450 metabolic detoxification associated with crowding, as this enzyme family can be induced by permethrin and is also a major detoxification pathway of permethrin (Kasai et al., 1998; Poupardin et al., 2008; Liu et al., 2011). A wide variety of chemicals have been shown to induce CYP450s, such as plant chemicals, fungal metabolites, hormones, pesticides, and metals (Feyereisen, 1999; Riaz et al., 2009). With the evidence for chemical factors (e.g., growth factors, waste metabolites) in larval crowding (Ikeshoji and Mulla, 1970; Bédhomme et al., 2005; Silberbush et al., 2014) there was the potential that chemical factors from conspecifics could induce CYP450s thereby playing a role in permethrin detoxification as well. Our experiments utilizing the synergist piperonyl butoxide to inhibit CYP450s demonstrated that CYP450 detoxification is likely not playing a role in the active crowding protective effect. Inhibiting CYP450s roughly doubled the sensitivity to permethrin for each density treatment but the synergism ratios calculated to estimate the importance of metabolism were not significantly different among the density treatments. As CYP450s are the metabolic enzyme most strongly linked to pyrethroid detoxification (Kasai et al., 2014), metabolism likely does not play a significant role in the protective effect of crowding. However, future work should also investigate esterases and glutathione-S-transferases (GSTs), two potentially important detoxification enzyme systems (Hemingway et al., 2004; Li et al., 2007).
Given the lack of an apparent effect of crowding on enzyme systems, an alternate and likely mechanism that would explain the protective effect of active crowding is modified exposure. In this scenario, crowded larvae competitively absorb the available insecticide so that the exposure each individual receives is reduced to the point of being less or non-toxic. This is substantiated by earlier studies that have demonstrated the mosquito larvae propensity for bioaccumulation of xenobiotics. Henry et al. (1971) found that 4th-instar mosquito larvae concentrated Abate (temephos) up to 1ppm per larva, which was 100 times the concentration of the surrounding water used to kill the larvae. Another study found the absorption of Abate into A. aegypti larvae was extremely rapid, with over 99% being absorbed within 1 h after exposure (Leesch and Fukuto, 1972). Previous studies also indicate that mosquito larvae are particularly efficient at absorbing xenobiotics relative to other aquatic organisms. Culex quinquefasciatus larvae accumulate DEHP (di-2-ethylhexyl phthalate; resembles DDT in rate of uptake and storage) to a much higher level than other aquatic organisms investigated (guppy, snail, Daphnia, Elodea, Culex pupae) (Metcalf et al., 1973). Compared to brine shrimp and silverside fish, A. aegypti larvae showed the highest tendency to accumulate TCDD, DDT, mexacarbate and γ-BHC. Furthermore, the level of bioaccumulation in fish decreased because of the competitive pickup of available xenobiotics by the A. aegypti larvae (Matsumara, 1977). The high degree of pesticide partitioning to mosquito larvae is likely related to their exceptionally high lipid content, which can facilitate survival up to 15 days without food (Timmermann and Briegel, 1999).
It is generally accepted that insecticide penetration occurs through the cuticle and into the hemolymph and is then dissolved and stored in lipid-rich regions such as the fat body (Welling, 1977; Arrese and Soulages, 2010). Compared to other mosquito species, pesticide partitioning may be more pronounced for A. aegypti because of their exponential, and non-linear accumulation of lipids throughout the larval period (Timmermann and Briegel, 1999). For lipophilic and low water soluble compounds such as permethrin, temephos, and DDT, partitioning capacity is best predicted using log KOW values, since octanol represents a good substitute for biotic lipids (Chessels et al., 1991). Temephos (under the trade name Abate®) is the third most widely used larvicide after methoprene and Bti, and is the only product specifically designed for application to tire piles (USEPA United States Environmental Protection Agency, 2015). The experiments conducted here utilized permethrin, but because of their very similar log KOW values (5.84 for trans-permethrin, 6.24 for cis-permethrin (our sample was 72% trans), 5.96 for temephos; Noble, 1993) their partitioning behavior in water systems is likely similar (Noble, 1993). Thus, permethrin is a good proxy for other contact insecticides, specifically temephos.
While we have not identified other studies that explored the effects of non-food limited larval crowding on sensitivity to contact insecticides, similar questions have been investigated but often involve other factors such as food levels. Muturi et al. (2010) found that at high nutrients and low malathion concentrations, survival was enhanced with increasing larval densities. However, these effects were attributed to release from competition among survivors after mortality from malathion. In this overcompensatory model of larval control, larviciding high-density populations can be counterintuitive (Agudelo-Silva and Spielman, 1984; Juliano, 2007). Our results add an additional layer of nuance to the process of density-mediated toxicant responses.
We have shown that high-density Aedes populations are more tolerant to insecticide exposure than low density populations, possibly due to modified exposure. The effects of density on insecticide tolerance can occur with seemingly innocuous changes in density, but we have shown that even relatively small changes in density, especially at the low to moderate density range (0–0.5 larvae/ml), can have significant impacts on survival (depicted in density-survival model, Figure 4). To add further context for our observed results, we conducted another experiment where we analyzed toxicity differences among four different density regimes found in the ecotoxicity literature that have been used in testing. Most studies appear to somewhat arbitrarily choose larval densities for toxicity experiments such as 20 larvae in 250 ml (Baruah, 2004), 20 larvae in 50 ml (Kasai et al., 2014), 30 larvae in 360 ml (Muturi et al., 2011), 20 larvae in 100 ml (Mulla et al., 1982), among others. We experimentally compared several representative densities and found significant differences in mortality of larvae exposed to the same 2.0 ppb permethrin (Figure 5). These results confirm our main finding that higher larval density can increase insecticide tolerance and further indicate that results from toxicity assays can differ widely depending on rearing density. We suspect the effect of density (in the absence of food limitation) has not been seriously considered when designing toxicity bioassays but perhaps should.
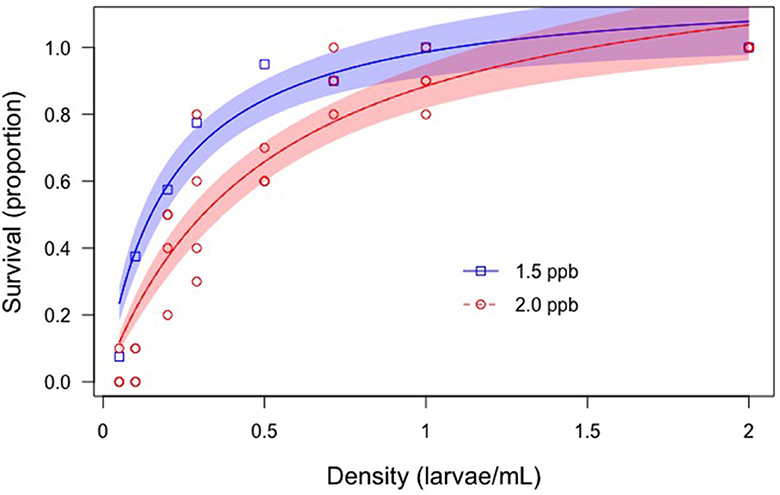
Figure 4. Relationship between larval density and survival. Two concentrations of permethrin (1.5 and 2.0 ppb) were used to test larval survival at eight different densities. The density-survival relationship is shown. Means along with 95% confidence intervals are shown. Control survival was >0.98 and is not shown here.

Figure 5. Comparison of insecticide sensitivity across a range of representative rearing densities found in published studies. A single concentration of permethrin, 2.00 ppb, was used to compare survival differences among four different larval density regimes (i.e., # of larvae and amount of water used). Density treatments are in order of increasing density (larvae/ml). Significant differences among samples were detected using one-way analysis of variance (ANOVA; F3, 336 = 36.12, p < 0.0001). A Tukey post hoc test detected significant differences among all samples except for between the two lowest densities, 30/360 and 10/100 larvae/mL (Tukey HSD, p < 0.05). Treatments that share a letter are not significantly different from each other. Data were log-transformed to meet assumptions. Means and standard error bars are shown.
The protective effect of crowding that we have shown here can have important implications for mosquito control programs, in designing and comparing toxicity tests (such as resistance monitoring), and in developing population models for use in management and risk assessment. High density larval populations encountered in the field may be tolerant or less receptive to insecticide treatment (such as temephos) if larval densities are not taken into consideration. Failure to compensate for high densities may lead to unsuccessful reduction of mosquitoes and may also permit the development of resistance if 100% mortality is not being achieved and the most tolerant individuals are able to survive (Hamdan et al., 2005). It is also very important in toxicity testing of mosquito larvae to consider the densities being used. The WHO has issued multiple recommendations for bioassay experimental design. In their instructions for determining susceptibility or resistance of larvae to insecticides (1981), they recommend a density of 20 or 25 larvae in a 250 ml solution, which correspond to densities of 0.08 and 0.1 larvae/ml, respectively. If applied to our model (Figure 2), these density differences result in survival differences of 11% and 18% for 0.08 and 0.1 larvae/ml, respectively. In the WHO guidelines for laboratory and field-testing of mosquito larvicides (2005), they recommend a density of 25 larvae in 100 or 200 ml of solution. In our model, these density differences resulted in 46% survival and 25% survival, respectively. The effect that small density differences have on toxicity makes it critical that toxicity data only be compared when the exact same density regimes are used. Ideally, comparative toxicity tests should all be conducted concurrently: same day, same time and same larvae population. For determining toxicant sensitivity in resistance monitoring it is crucial to perform the same toxicity test on a susceptible population [as advised in World Health Organization (2005)] and to not rely only on a lethal concentration value, as larval crowding can easily influence them.
Additionally, when assessing impacts or potential impacts of toxicants on exposed populations, our results have clear and potentially meaningful applications beyond mosquito control. As indicated, understanding the manifestation of density-dependent effects is critically important for assessing impacts of anthropogenic stressors on populations (Accolla et al., 2021). For example, Woo et al. (2020), using Daphnia magna as the research model, demonstrated the importance of resource levels as well as phase of the population cycle and density in contributing to response and recovery from pesticide exposure. In essence, while it is widely recognized that density-dependent effects are critical for understanding and predicting population-level effects of anthropogenic stressors, more research is needed to continue to advance the science and to improve management of impacted freshwater systems.
This study demonstrates the importance of crowding in mosquito larval tolerance to contact insecticides. Latent effects of crowding in this study did not exhibit a significant overall impact on insecticide sensitivity but active crowding (when crowding co-occurred with insecticide exposure) resulted in a strongly protective effect against the insecticide with the likely mechanism occurring via a modification of exposure. These results stress the importance of larval crowding in the design and comparison of susceptibility and resistance testing as well as highlight the need to consider larval density when carrying out mosquito control efforts in the field and when considering population-level effects of anthropogenic stressors.
Data availability statement
The raw data supporting the conclusions of this article will be made available by the authors, without undue reservation.
Author contributions
TB: Conceptualization, Data curation, Formal analysis, Investigation, Methodology, Validation, Visualization, Writing – original draft, Writing – review & editing. DD: Conceptualization, Formal analysis, Investigation, Methodology, Validation, Visualization, Writing – review & editing. CS: Conceptualization, Formal analysis, Methodology, Project administration, Resources, Supervision, Writing – review & editing.
Funding
The author(s) declare that no financial support was received for the research, authorship, and/or publication of this article.
Acknowledgments
This research was conducted at Texas Tech Univesity and we would like to thank Texas Tech University and The Institute of Environmental and Human Health (TIEHH) for funding for TB. This research was also supported by a grant from the Helen DeVitt Jones Graduate Fellowship. CS was supported by U.S. Environmental Protection Agency Science to Achieve Results grant (8358001), Additional thanks to Dr. Todd Anderson and Dr. Jon Maul for fruitful discussions. We thank two reviewers whose comments improved the manuscript.
Conflict of interest
DD is employed by the company Integral Consulting.
The remaining authors declare that the research was conducted in the absence of any commercial or financial relationships that could be construed as a potential conflict of interest.
The author CS declared that they were an editorial board member of Frontiers, at the time of submission. This had no impact on the peer review process and the final decision.
Publisher's note
All claims expressed in this article are solely those of the authors and do not necessarily represent those of their affiliated organizations, or those of the publisher, the editors and the reviewers. Any product that may be evaluated in this article, or claim that may be made by its manufacturer, is not guaranteed or endorsed by the publisher.
References
Accolla, C., Vaugeois, M., Grimm, V., Moore, A. P., Rueda-Cediel, P., Schmolke, A., et al. (2021). A review of key features and their implementation in unstructured, structured, and agent-based population model for ecological risk assessment. Environm. Toxicol. Chem. 17, 521–540. doi: 10.1002/ieam.4362
Agudelo-Silva, F., and Spielman, A. (1984). Paradoxical effects of simulated larviciding on production of adult mosquitoes. Am. J. Trop. Med. Hyg. 33, 1267–1269. doi: 10.4269/ajtmh.1984.33.1267
Arrese, E. L., and Soulages, J. L. (2010). Insect fat body: energy, metabolism and regulation. Annu. Rev. Entomol. 55, 207–225. doi: 10.1146/annurev-ento-112408-085356
Barbosa, P., Peters, T. M., and Grenough, N. C. (1972). Overcrowding of mosquito populations responses of larval Aedes aegypti to stress. Environ. Entomol. 1, 89–93. doi: 10.1093/ee/1.1.89
Baruah, K. (2004). Laboratory bio-assay of Temephos and Fenthion against some vector species of public health importance. J. Commun. Dis. 36, 100–104.
Becker, N., Zgomba, M., Ludwig, M., Petric, D., and Rettich, F. (1992). Factors influencing the activity of Bacillus thuringiensis var. israelensis treatments. J. Am. Mosquito Control Assoc. 8, 285−289
Bedhomme, S., Agnew, P., Sidobre, C., and Michalakis, Y. (2003). Sex-specific reaction norms to intraspecific larval competition in the mosquito Aedes aegypti. J. Evol. Biol. 16, 721–730. doi: 10.1046/j.1420-9101.2003.00576.x
Bédhomme, S., Agnew, P., Sidobre, C., and Michalakis, Y. (2005). Pollution by conspecifics as a component of intraspecific competition among Aedes aegypti larvae. Ecol. Entomol. 30, 1–7. doi: 10.1111/j.0307-6946.2005.00665.x
Birk, S., Chapman, D., Carvalho, L., Spears, B. M., Andersen, H. E., et al. (2020). Impacts of multiple stressors on freshwater biota across spatial scales and ecosystems. Nat. Ecol. Evol. 4, 1060–1068. doi: 10.1038/s41559-020-1216-4
Broadie, K. S., and Bradshaw, W. E. (1991). Mechanisms of interference competition in the tree hole mosquito, Aedes sierrensis. Ecol. Entomol. 16, 145–154. doi: 10.1111/j.1365-2311.1991.tb00203.x
Busch, W., Schmidt, S., Kühne, R., Schulze, T., Krauss, M., and Altenburger, R. (2016). Micropollutants in European rivers: a mode of action survey to support the development of effect-based tools for water monitoring. Environm. Toxicol. Chem. 35, 1887–1899. doi: 10.1002/etc.3460
Campero, M., De Block, M., Ollevier, F., and Stoks, R. (2008). Correcting the short-term effect of food deprivation in a damselfly: mechanisms and costs. J. Anim. Ecol. 77, 66–73. doi: 10.1111/j.1365-2656.2007.01308.x
Chessels, M., Hawker, D. W., and Connell, D. W. (1991). Critical evaluation of the measurement of the 1-octanol/water partition coefficient of hydrophobic compounds. Chemosphere 22, 1175–1190. doi: 10.1016/0045-6535(91)90213-W
Dye, C. (1984). Competition amongst larval Aedes aegypti: the role of interference. Ecol. Entomol. 9, 355–357. doi: 10.1111/j.1365-2311.1984.tb00859.x
Edwards, A. J. (1981). Effects of carbon dioxide anaestesia and crowding on the susceptibility of cockroaches (Periplaneta americana L.) to insecticides. Entomol. Exp. Appl. 29, 339–344.
Feyereisen, R. (1999). Insect P450 Enzymes. Annu. Rev. Entomol. 44, 507–533. doi: 10.1146/annurev.ento.44.1.507
Foit, K., Kaske, O., and Liess, M. (2012). Competition increases toxicant sensitivity and delays the recovery of two interacting populations. Aquatic Toxicol. 106–107, 25–31. doi: 10.1016/j.aquatox.2011.09.012
Forbes, V. E., Sibly, R. M., and Calow, P. (2001). Toxicant impacts on density-limited populations: a critical review of theory, practice, and results. Ecol. Appl. 11, 1249–1257. doi: 10.1890/1051-0761(2001)011(1249:TIODLP)2.0.CO;2
Hamdan, H., Sofian-Azirun, M., Ahmad, N. W., and Lim, L. H. (2005). Insecticide resistance development in Culex quinquefasciatus (Say), Aedes aegypti (L.) and Aedes albopictus (Skuse) larvae against malathion, permethrin and temephos. Trop. Biomed. 22, 45–52.
Haramis, L. (1983). Increased adult size correlated with parity in Aedes triseriatus. Mosq. News 43, 77–79.
Hardstone, M. C., Leichter, C., Harrington, L. C., Kasai, S., Tomita, T., and Scott, J. G. (2007). Pesticide biochemistry and physiology. 89, 175–184. doi: 10.1016/j.pestbp.2007.06.006
Hawley, W. A. (1985). The effect of larval density on adult longevity of a mosquito, Aedes sierrensis: epidemiological consequences. 54, 955–964. doi: 10.2307/4389
Hemingway, J., Hawkes, N. J., McCarroll, L., and Ranson, H. (2004). The molecular basis of insecticide resistance in mosquitoes. Insect Biochem. Mol. Biol. 34, 653–665. doi: 10.1016/j.ibmb.2004.03.018
Henry, R. A., Schmit, J. A., Dieckman, J. F., and Murphey, F. J. (1971). Combines high speed liquid chromatography and bioassay for the evaluation and analysis of an organophosphorous larvicide. Anal. Chem. 43, 1053–1057. doi: 10.1021/ac60303a019
Holmstrup, M., Bindesbol, A. M., Oostingh, G. H., Duschl, A., Scheil, V., Kohler, H. R., et al. (2010). Interactions between effects of environmental chemicals and natural stressors: a review. Sci. Total Environm. 408, 3746–3762. doi: 10.1016/j.scitotenv.2009.10.067
Ikeshoji, T., and Mulla, M. S. (1970). Overcrowding factors of mosquito larvae. J. Econ. Entomol. 63, 90–96. doi: 10.1093/jee/63.1.90
Juliano, S. A. (2007). Population dynamics. J. Am. Mosq. Control Assoc. 23, 265–275. doi: 10.2987/8756-971X(2007)23(265:PD)2.0.CO;2
Kasai, S., Komagata, O., Itokawa, K., Shono, T., Ng, L. C., Kobayashi, M., and Tomita, T. (2014). Mechanisms of pyrethroid resistance in the Dengue mosquito vector, Aedes aegypti: Target site insensitivity, penetration, and metabolism. PLoS Negl. Trop. Dis. 8, e2984. doi: 10.1371/journal.pntd.0002948
Kasai, S., Weerashinghe, I. S., and Shono, T. (1998). P450 monooxygenases are an important mechanism of permethrin resistance in Culex quinquefasciatus Say larvae. Arch. Insect Biochem. Physiol. 37, 47–56.
Kaushal, S. S., Likens, G. E., Pace, M. L., Reimer, J. E., Maas, C. M., et al. (2021). Freshwater salinization syndrome: from emerging global problem to managing risks. Biogeochemistry 154, 225–292. doi: 10.5194/egusphere-egu21-16299
Leesch, J. G., and Fukuto, T. R. (1972). The metabolism of Abate in mosquito larvae and houseflies. Pestic. Biochem. Physiol. 2, 223–235. doi: 10.1016/0048-3575(72)90025-9
Li, X., Schuler, M. A., and Berenbaum, M. R. (2007). Molecular mechanisms of metabolic resistance to synthetic and natural xenobiotics. Ann. Rev. Entomol. 52, 231–253. doi: 10.1146/annurev.ento.51.110104.151104
Liu, N., Li, T., Reid, W. R., Yang, T., and Zhang, L. (2011). Multiple cytochrome P450 genes: their constitutive overexpression and permethrin induction in insecticide resistant mosquitoes, Culex quinquefasciatus. PLoS ONE 6, e23403. doi: 10.1371/journal.pone.0023403
Livdahl, T. P., Koenekoop, R. K., and Futterweit, S. G. (1984). The complex hatching response of Aedes eggs to larval density. Ecol. Entomol. 9, 437–442. doi: 10.1111/j.1365-2311.1984.tb00841.x
Lohner, T. W., and Fisher, S. W. (1990). Effects of pH and temperature on the acute toxicity and uptake of carbaryl in the midge, Chironomus riparius. Aquatic Toxicol. 16, 335–354. doi: 10.1016/0166-445X(90)90045-Q
Maciá, A. (2009). Effects of larval crowding on development time, survival and weight at metamorphosis in Aedes aegypti (Diptera: Culicidae). Revista de la Sociedad Entomolo gica Argentina 68, 107−114.
Matsumara, F. (1977). Absorption, accumulation, and elimination of pesticides by aquatic organisms. Environm. Sci. Res. 10, 77–105. doi: 10.1007/978-1-4684-2868-1_5
Metcalf, R. L., Booth, G. M., Schuth, C. K., Hansen, D. J., and Lu, P. Y. (1973). Uptake and fate of di-2-ethylhexyl phthalate in aquatic organisms and in a model ecosystem. Environ. Health Perspect. 4, 27–34. doi: 10.1289/ehp.730427
Moore, C. G., and Fisher, B. R. (1969). Competition in mosquitoes. Density and species ratio effects growth, mortality, fecundity and production of growth retardant. Ann. Entomol. Soc. Am. 62, 1325–1331. doi: 10.1093/aesa/62.6.1325
Mori, A. (1979). Effects of larval density and nutrition on some attribute of immature and adult Ae. albopictus. Trop. Med. 21, 85–103.
Mulla, M. S., Darwaseh, H. A., and Ede, L. (1982). Evaluation of new pyrethroids against immature mosquitoes and their effects on nontarget organisms. Mosq. News 42, 583–590.
Muturi, E. J., Costanzo, K., Kesavaraju, B., Lampman, R., and Alto, B. W. (2010). Interactions of a pesticide and larval competition on life history traits of Culex pipiens. Acta Trop. 116, 141–146. doi: 10.1016/j.actatropica.2010.07.003
Muturi, E. J., Lampman, R., Costanzo, K., and Alto, B. W. (2011). Effect of temperature and insecticide stress on life-history traits of Culex restuans and Aedes albopictus (Diptera: Culicidae). J. Med. Entomol. 48, 243–250. doi: 10.1603/ME10017
Ng'habi, K. R., John, B., Nkwengulila, G., Knols, B. G. J., Killeen, G. F., and Ferguson, H. M. (2005). Effect of larval crowding on mating competitiveness of Anopheles gambiae mosquitoes. Malar. J. 4:49. doi: 10.1186/1475-2875-4-49
Nkya, T. E., Akhouayri, I., Kisinza, W., and David, J. P. (2013). Impact of environment on mosquito response to pyrethroid insecticides: facts, evidences and prospects. Insect Biochem. Mol. Biol. 43, 407–416. doi: 10.1016/j.ibmb.2012.10.006
Noble, A. (1993). Partition coefficients (n-octanol-water) for pesticides. J. Chromatogr. 642, 3–16. doi: 10.1016/0021-9673(93)80072-G
Ormerod, S. J., Dobson, M., Hildrew, A. G., and Townsend, C. F. (2010). Multiple stressors in freshwater ecosystems. Freshwater Biol. 55, 104. doi: 10.1111/j.1365-2427.2009.02395.x
Payton, M. E., Greenstone, M. H., and Schenker, N. (2003). Overlapping confidence intervals or standard error intervals: What do they mean in terms of statistical significance? J. Insect Sci. 3, 34. doi: 10.1673/031.003.3401
Pechenik, J. A. (2006). Larval experience and latent effects—metamorphosis is not a new beginning. Integr. Comp. Biol. 46, 323–333. doi: 10.1093/icb/icj028
Poupardin, R., Reynaud, S., Strode, C., Ranson, H., Vontas, J., and David, J. P. (2008). Cross-induction of detoxification genes by environmental xenobiotics and insecticides in the mosquito Aedes aegypti: impact on larval tolerance to chemical insecticides. Insect Biochem. Mol. Biol. 38, 540–551. doi: 10.1016/j.ibmb.2008.01.004
Qin, G., Presley, S. M., Anderson, T. A., Gao, W., and Maul, J. D. (2011). Effects of predator cues on pesticide toxicity: toward an understanding of the mechanism of the interaction. Environm. Toxicol. Chem. 30, 1926–1934. doi: 10.1002/etc.575
R Core Team (2023). R: A Language and Environment for Statistical Computing. Vienna, Austria: R Foundation for Statistical Computing.
Reiskind, M. H., and Lounibos, L. P. (2009). Effects of intraspecific larval competition on adult longevity in the mosquitoes Aedes aegypti and Aedes albopictus. Med. Vet. Entomology 23, 62–68. doi: 10.1111/j.1365-2915.2008.00782.x
Relyea, R., and Hoverman, J. (2006). Assessing the ecology in ecotoxicology: a review and synthesis in freshwater systems. Ecol. Lett. 9, 1157–1171. doi: 10.1111/j.1461-0248.2006.00966.x
Relyea, R. A. (2003). Predator cues and pesticides: A double dose of danger for amphibians. Ecol. Appl. 13, 1515–1521. doi: 10.1890/02-5298
Renshaw, M., Service, M. W., and Birley, M. H. (1994). Size variation and reproductive successs in the mosquito Aedes cantans. Med. Vet. Entomol. 8, 179–186. doi: 10.1111/j.1365-2915.1994.tb00161.x
Riaz, M. A., Chandor-Proust, A., Dauphin-Villemant, C., Poupardin, R., Jones, C. M., Strode, C., et al. (2013). Molecular mechanisms associated with increased tolerance to the neonicotinoids insecticide imidacloprid in the dengue vector Aedes aegypti. Aquatic Toxicol. 126, 326–337. doi: 10.1016/j.aquatox.2012.09.010
Riaz, M. A., Poupardin, R., Reynaud, S., Strode, C., Ranson, H., and David, J. P. (2009). Impact of glyphosate and benzo[a]pyrene on the tolerance of mosquito larvae to chemical insecticides. Role of detoxification genes in response to xenobiotics. Aquatic Toxicol. 93, 61–69. doi: 10.1016/j.aquatox.2009.03.005
Ritz, C., and Streibig, J. C. (2005). Bioassay analysis using R. J. Statist. Software 12, 5. doi: 10.18637/jss.v012.i05
Roberts, D. (1998). Overcrowding of Culex sitiens (Diptera: Culicidae) Larvae: population Regulation by Chemical Factors or Mechanical Interference. J. Med. Entomol. 35, 665–669. doi: 10.1093/jmedent/35.5.665
Roberts, D., and Kokkinn, M. (2010). Larval crowding effects on the mosquito Culex quinquefasciatus: physical or chemical? Entomol. Exp. Appl. 135, 271–275. doi: 10.1111/j.1570-7458.2010.00993.x
Scott, J. G. (1999). Cytochromes P450 and insecticide resistance. Insect Biochem. Mol. Biol. 29, 757–777. doi: 10.1016/S0965-1748(99)00038-7
Silberbush, A., Tsurim, I., Rosen, R., Margalith, Y., and Ovadia, O. (2014). Species-specific non-physical interference competition among mosquito larvae. PLoS ONE 9, e88650. doi: 10.1371/journal.pone.0088650
Spears, B. M., Chapman, D. S., Carvalho, L., Field, C. K., Gessner, M. O., et al. (2021). Making waves. Bridging theory and practice towards multiple stressor management of freshwater ecosystems. Water Res. 196, 116981. doi: 10.1016/j.watres.2021.116981
Timmermann, S. E., and Briegel, H. (1999). Larval growth and biosynthesis of reserves in mosquitoes. J. Insect Physiol. 45, 461–470. doi: 10.1016/S0022-1910(98)00147-4
Uitregt, V. O., Hurst, T. P., and Wilson, R. S. (2012). Reduced size and starvation resistance in adult mosquitoes, Aedes notoscriptus, exposed to predation cues as larvae. J. Anim. Ecol. 81, 108–115. doi: 10.1111/j.1365-2656.2011.01880.x
USEPA United States Environmental Protection Agency (2015). RED Reregistration Eligibility Decisions Facts – Temephos. Available online at: https://webharvest.gov/peth04/20041120005945/http://www.epa.gov/oppsrrd1/REDs/temephos_red.htm (accessed April 9, 2015).
Wantzen, K. M., and Mol, J. H. (2013). Soil erosion from agriculture and mining: a threat to tropical stream ecosystems. Agriculture 3, 660–683. doi: 10.3390/agriculture3040660
Wasserberg, G., Bailes, N., Davis, C., and Yeoman, K. (2014). Hump-shaped density-dependent regulation of mosquito oviposition site-selection by conspecific immature stages: theory, field test with aedes albopictus, and a meta-analysis. PLoS ONE 9, e92658. doi: 10.1371/journal.pone.0092658
Welling, W. (1977). Dynamic aspects of insect-insecticide interactions. Annu. Rev. Entomol. 22, 53–78. doi: 10.1146/annurev.en.22.010177.000413
White, M. T., Griffin, J. T., Churcher, T. S., Ferguson, N. M., Basanez, M. G., and Ghani, A. C. (2011). Modelling the impact of vector control interventions on Anopheles gambiae population dynamics. Paras. Vectors 4, 153. doi: 10.1186/1756-3305-4-153
Woo, T. J., East, A., and Salice, C. J. (2020). Intraspecific interactions affect outcomes of pulse toxicity at different Daphnia magna population phases. Environ. Pollut. 267:115398. doi: 10.1016/j.envpol.2020.115398
Woodward, G., Perkins, D. M., and Brown, L. E. (2010). Climate change and freshwater ecosystems: impacts across multiple levels of organization. Phil. Trans. R. Soc. B 365:2093–2106. doi: 10.1098/rstb.2010.0055
Keywords: ephemeral, density-mediated effects, toxicant, crowding, aquatic invertebrate
Citation: Bilbo TR, Dawson DE and Salice CJ (2024) The challenge of assessing multiple stressors in freshwater ecosystems: nonintuitive interactions between pesticide exposure and larval crowding. Front. Freshw. Sci. 1:1302240. doi: 10.3389/ffwsc.2023.1302240
Received: 26 September 2023; Accepted: 29 November 2023;
Published: 17 January 2024.
Edited by:
Theodore Henry, Heriot-Watt University, United KingdomReviewed by:
Silvia Quadroni, University of Insubria, ItalySylvia Anton, Institut National de recherche pour l'agriculture, l'alimentation et l'environnement (INRAE), France
Copyright © 2024 Bilbo, Dawson and Salice. This is an open-access article distributed under the terms of the Creative Commons Attribution License (CC BY). The use, distribution or reproduction in other forums is permitted, provided the original author(s) and the copyright owner(s) are credited and that the original publication in this journal is cited, in accordance with accepted academic practice. No use, distribution or reproduction is permitted which does not comply with these terms.
*Correspondence: Christopher J. Salice, Y3NhbGljZSYjeDAwMDQwO3Rvd3Nvbi5lZHU=