- 1Department of Biosciences and Bioengineering, Indian Institute of Technology (IIT), Roorkee, Roorkee, India
- 2Department of Biotechnology, Pandit S.N. Shukla University, Shahdol, India
Candida albicans is the predominant cause of systemic candidiasis, although other non albicans Candida species are progressively becoming more widespread nowadays. Candida auris has emerged as a deadly multidrug-resistant fungal pathogen, posing a significant threat to global public health. In the absence of effective antifungal therapies, the development of a vaccine against C. auris infections is imperative. Enolase, a key glycolytic enzyme, has emerged as a promising vaccine candidate due to its immunogenic properties and essential role in fungal virulence. Herein, full-length Enolase gene sequences from C. albicans and C. auris were cloned into suitable expression vector and transformed into Escherichia coli expression hosts. Recombinant Enolase proteins were successfully expressed and purified using affinity chromatography under native conditions, followed by SDS-PAGE characterization and Western blot analysis. CD spectroscopy verified the existence of expressed proteins in soluble native conformation. Preliminary in silico studies verified the immunogenicity of recombinant Enolase proteins isolated from both C. albicans and C. auris. Furthermore, bioinformatics analysis revealed conserved B-cell and T-cell epitopes across C. albicans and C. auris Enolase proteins, suggesting potential cross-reactivity and broad-spectrum vaccine efficacy. Our findings are anticipated to play a role in advancing therapeutic as well as diagnostic strategies against systemic candidiasis.
Introduction
Systemic candidiasis stands out as a prevalent nosocomial bloodstream infection on a global scale, affecting patients in intensive care units (ICUs) (Warnock, 2007; Soriano et al., 2023). Candida albicans has long been recognized as a predominant pathogen responsible for candidiasis. However, the emergence of non-albicans Candida (NAC) species, including C. auris, contributes to approximately 65% of Candida infections (Papon et al., 2013; Whibley and Gaffen, 2015). It has been estimated that over 250,000 individuals globally are affected by systemic candidiasis each year, which results in about 50,000 fatalities (Pappas et al., 2018). According to the Centers for Disease Control and Prevention (CDC), the global mortality rate for systemic candidiasis ranges from 30–70 percent, despite treatment with antifungal medications (Calandra et al., 2016). The emergence of antifungal drug resistance, coupled with high mortality rates and the increasing prevalence of infections caused by NAC species, especially multidrug resistant C. auris, has drawn urgent need for alternative immunotherapy approaches for the treatment of systemic candidiasis. Currently, there are no approved immunotherapeutic strategies available for the treatment of systemic candidiasis. Despite the identification of numerous anti-Candida vaccine candidates, only a small subset has undergone clinical trial evaluation thus far. Strategies for anti-Candida vaccines have included the investigation of many live attenuated strains, recombinant proteins, fungal cell wall polysaccharides, and/or glycoconjugates (Wan Tso et al., 2018). Among the leading vaccine candidates for systemic candidiasis, Enolase protein stands out as a frontrunner.
The Enolase 1 gene, also known as 2-phospho-D-glycerate hydrolase, belonging to the Enolase superfamily, has emerged as one of the leading vaccine candidates due to its well-established role in fungal virulence (Ko et al., 2013). This enzyme is highly expressed in the cytosol and plays a role in the glycolytic pathway, additionally showing transglutaminase activity (Reyna-Beltrán et al., 2018). Besides its role in glycolysis, Enolase has been implicated in various immunological processes and is considered a virulence factor (Lim et al., 2021). In addition to its cytosolic localization, it is secreted into the extracellular medium (He et al., 2022), released into extracellular vesicles (Karkowska-Kuleta et al., 2020), and is also anchored on the fungal cell surface (Satala et al., 2020a). It has been seen that Candida cell surface attachment of Enolase is mediated through Als3-binding (Karkowska-Kuleta et al., 2021), and cell wall Enolase further helps in fungal adhesion to various medical devices (Núñez-Beltrán et al., 2017). Exposed enolase on the surface of C. albicans cells has been reported to participate in binding human plasminogen, leading to increased invasion of human brain microvascular endothelial cells (Jong et al., 2003; Funk et al., 2016). Numerous research investigations have linked Enolase to its involvement in facilitating microbial attachment to host extracellular matrix proteins such as fibronectin, laminin, and vitronectin (Satala et al., 2020b). It plays a role in facilitating fungal adhesion to human tissues, activating fibrinolysis, and degrading the extracellular matrix (Jong et al., 2003; Silva et al., 2014).
Sundstorm et al., reported that Enolase acts as a prominent humoral immunogen, stimulating both humoral and cell-mediated responses in mice (Sundstrom et al., 1994). In mice, the passive transfer of antibodies against Enolase was seen to offer partial protection against systemic candidiasis (Van Deventer et al., 1996). Another study highlighted the immunogenicity of recombinant Enolase from C. albicans and its ability to provide protection against systemic candidiasis by reducing fungal burden and increasing survival in Enolase-immunized mice (Montagnoli et al., 2004). Along with increase in immunogenicity, the protection observed in Enolase vaccinated mice was attributed to induction of a Th1 mediated cellular immune response (Montagnoli et al., 2004). Li et al., demonstrated that immunization with purified recombinant Enolase from C. albicans conferred significant protection against tissue damage following challenge with two different strains of C. albicans. Protection in mice was associated with a reduced fungal burden, increased titers of Enolase-specific antibodies (IgG1 and IgG2a), increased secretion of Th1 (IL-12 and IL-8) and Th2 (IL-10) cytokines, and effective killing of phagocytosed yeasts by neutrophils (Li et al., 2011). Notably, oral immunization using Lactobacillus casei displaying Enolase 1 from C. albicans elicited a strong IgG response and increased the survival rate of the vaccinated mice against systemic candidiasis (Shibasaki et al., 2014). Recently, Leu et al., demonstrated improved survival rates, decreased fungal loads, and lowered levels of inflammatory cytokines in mice treated with a single-chain variable fragment monoclonal antibody (CaS1) targeting recombinant C. albicans Enolase during candidiasis (Leu et al., 2020).
The aim of this study was to identify and isolate the Enolase gene from C. auris, a multi drug resistant (MDR) strain, circulating in the Indian subcontinent, and compare it with the Enolase gene of C. albicans, as a reference strain. This study presents the molecular cloning followed by expression and purification of recombinant Enolase proteins of C. albicans (SC3514) and C. auris (South Asian clade) strains in a prokaryotic expression system. The recombinant Eno-albicans and Eno-auris proteins were purified under native soluble conditions and subsequently dialyzed for further analysis. Circular dichroism (CD) spectroscopy was performed to confirm the secondary structure of the Enolase proteins. Further, the B-cell and T-cell epitopes belonging to Eno-albicans and Eno-auris proteins were predicted using in silico immunoinformatics approaches, to compare their immunogenic and vaccine potential. Our results show that there is a high degree of homology between Enolase proteins belonging to C. albicans and C. auris species, and they can be used for the development of therapeutic as well as diagnostic strategies against systemic candidiasis.
Materials and methods
Chemicals and strains
C. albicans strain SC5314 (sourced from Prof. R. Prasad, Amity University, Gurgaon, India) and C. auris South Asian strain (Clade 1 CBS 470280) (sourced from Prof. M R Shivaprakash, PGIMER Chandigarh, India) were used for this work. The pQE30Xa vector (Qiagen, Germany) having an N-terminus 6-histidine tag was used for cloning of Enolase protein followed by its expression. Bacterial cultures were done using Luria-Bertani (LB) broth and agar supplemented with Ampicillin (100 μg/ml) and Kanamycin (25 μg/ml) antibiotics. Restriction enzymes used for cloning and amplification were obtained from NEB. The cloning and expression of both recombinant Enolase proteins were performed in E. coli XL1-blue and E. coli SG13009 strains, respectively. Ni-NTA affinity matrix for protein purification was procured from Qiagen. Sabouraud’s Dextrose broth (SAB) and agar media from HiMedia were utilized for routine Candida cultures.
Culture conditions
All fungal strains were stored at -80°C in SAB with 15% (vol/vol) glycerol. Glycerol stocks of C. albicans and C. auris strains were utilized for inoculation and subsequently cultured at 30°C and 37°C, respectively, in SAB broth for 48 hours with continuous shaking at 180 rpm (Chandley et al., 2022).
Cloning of recombinant enolase genes from C. albicans and C. auris
Genomic DNA was isolated from C. albicans and C. auris strains using the QIAamp DNA Mini Kit (Qiagen), according to manufacturer’s protocol. PCR amplification of Enolase gene (1323 bp, 440 aa) of C. albicans strain SC5314 was performed using gene-specific primers (Table 1) and Phusion DNA polymerase (NEB) (Shukla et al., 2021a). The reaction mixture, totaling 25 µL, comprised 5X GC Buffer, 0.2 µM of forward and reverse primers, 200 µM dNTP mix, 50 ng of C. albicans genomic DNA, and 2.5 Units of Phusion DNA polymerase. PCR reactions were conducted using the GeneAmp 2700 PCR system. PCR cycling involved an initial denaturation at 98°C for 2 minutes, followed by 30 cycles of denaturation at 98°C for 30 seconds, annealing at 57°C for 1 minute, and extension at 72°C for 1.5 minutes. Final extension was performed at 72°C for 10 minutes.
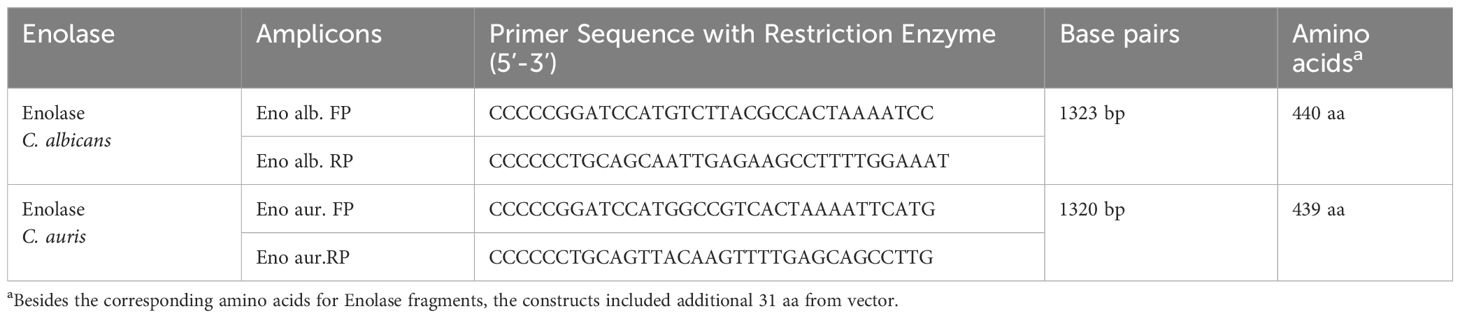
Table 1 Primers and restriction enzymes used for PCR amplification and expression cloning of Enolase fragments from C. albicans and C. auris.
PCR amplification of Enolase gene (1320 bp, 439 aa) of C. auris strain (Clade 1 CBS 470280) was performed using gene-specific primers (Table 1) and Ex-Taq DNA polymerase (Takara Bio). The similar PCR reaction including temperature cycling parameters was employed as those used for amplifying Enolase of C. albicans, except that instead of 5X GC Buffer and Phusion polymerase, 10X Ex-Taq buffer and 2.5 Units of Ex-Taq DNA polymerase was used. The Enolase fragments were purified and cloned into corresponding restriction sites of E. coli expression vector pQE30Xa (Qiagen). Transformants were screened on LB ampicillin plates and positive clones were verified through restriction digestion and confirmed by sequencing.
Induction check of recombinant enolase proteins from C. albicans and C. auris
For protein induction and expression check, the Enolase gene constructs belonging to C. albicans and C. auris were re-transformed in E. coli strain SG13009 (Qiagen). The colonies obtained were proceeded with small scale bacterial cultures. Overnight grown primary cultures (1 ml) at 37°C and 220 rpm were used to inoculate secondary cultures (5 ml) the next day. The bacterial cultures were allowed to grow for 3 hours at 37°C and 220 rpm in orbital shaker. After the OD600 was obtained in the range of 0.4 - 0.6, protein expression was induced by adding 1.0 mM isopropyl-β-D-thiogalactopyranoside (IPTG) and kept for 8 hours at 18°C and 220 rpm in orbital shaker. After 8 hours, the induced cultures were centrifuged along with un-induced controls, and the pellets obtained were stored at -20°C. The un-induced and induced pellets were dissolved in SDS loading dye, heat-denatured and analyzed on SDS PAGE.
Expression and purification of recombinant enolase proteins from C. albicans and C. auris
The recombinant Enolase proteins from C. albicans and C. auris were purified under native conditions using Ni-NTA affinity chromatography following the manufacturer’s instructions provided by Qiagen. For large scale bacterial cultures, primary inoculation of positive colonies were performed in 5 ml of LB media with appropriate antibiotics at 37°C and 220 rpm (Shukla and Rohatgi, 2020). Next day, secondary culture was inoculated in 500 ml of LB medium containing 100 μg/ml ampicillin and 25 μg/ml kanamycin at 37°C and 220 rpm. Protein expression was induced by adding 1 mM IPTG to mid-logarithmic phase cultures (OD600 ~0.6) for 8 hours at 18°C and 220 rpm. Bacteria were harvested by centrifugation at 10,000 rpm for 60 min and pellets were weighed and stored at -80°C. After freeze-thawing, the pellets were resuspended in lysis buffer (pH 8.0, 10 mM imidazole), containing lysozyme. Cell lysates were obtained through 10 cycles of sonication, each lasting 20 seconds with a 20-second interval between pulses. Subsequently, the lysates underwent centrifugation at 10,000 rpm for 30 min at 4°C and the recombinant proteins were purified from the supernatants using Ni-NTA affinity chromatography as per the manufacturer’s instructions provided by Qiagen. Briefly, the supernatants were gently mixed with Ni-NTA slurry for 1 hour at 4°C, and loaded onto Ni-NTA columns. After flowthrough collection, the columns were passed with Wash buffer I (pH 8.0, 20 mM imidazole) and Wash buffer II (pH 8.0, 50 mM imidazole). Finally, the protein samples were eluted using Elution buffer I (pH 8.0, 100 mM imidazole) and Elution buffer II (pH 8.0, 250 mM imidazole). The eluted samples containing purified recombinant Enolase proteins were collected and stored at 4°C. Aliquots of the flowthrough, wash and elution fractions were heat-denatured and analyzed on SDS PAGE and Coomassie staining as per established protocols. The elution fractions having pure proteins (free from impurities) were pooled together and subjected to dialysis for 12 hours at 4°C for removing imidazole. The dialysis buffer was changed at an interval of 4 hours. The dialysed and un-dialysed protein fractions were further analyzed on SDS-PAGE.
Western blotting of recombinant enolase proteins from C. albicans and C. auris
The purified N-terminal histidine-tagged Enolase proteins were resolved on a 10% SDS-PAGE gel alongside a pre-stained protein marker (Bio Rad). His-tagged PspA protein was used as positive control along with BSA as negative control. After gel electrophoresis, they were transferred onto a nitrocellulose membrane (Bio-Rad). To prevent non-specific binding, the membrane was blocked with 3% (w/v) BSA in Tris-buffered saline (TBS) for 1 hour at room temperature. Subsequently, the membrane underwent four washes with TBS containing 0.05% Tween-20 (TBST). It was then incubated with an anti-His monoclonal antibody from Santa Cruz Biotechnology at a 1:1000 dilution for 1 hour at room temperature. After another four washes with TBST, the membrane was exposed to an HRP-conjugated goat anti-mouse IgG from Santa Cruz Biotechnology at a 1:5000 dilution for 1 hour at room temperature. After four washes with TBST and one wash with TBS, the membrane was exposed to a 3,3′-diaminobenzidine (DAB) substrate solution (0.5 mg/mL) at room temperature (Shukla et al., 2021a). The color development process was halted by rinsing with water. Subsequently, the blots were allowed to air-dry and scanned.
CD spectroscopy of recombinant enolase proteins from C. albicans and C. auris
To assess the structural composition of the recombinant Enolase proteins, the dialyzed proteins from both C. albicans and C. auris were subjected to circular dichroism (CD) analysis using a Chirascan Circular Dichroism Spectrometer manufactured by Applied Photophysics Ltd., Surrey, UK (Shukla et al., 2021a). CD spectra were acquired using a 1 mm quartz cell with continuous nitrogen purging, spanning wavelengths from 190 to 250 nm in 1 nm increments, with an average acquisition time of 2.0 seconds at 18°C. The protein samples, prepared at a concentration of 1.5 mg/mL, were analyzed in a buffer consisting of 10 mM Tris at pH 7.4 with 100 mM NaCl. Every sample underwent three scans, which was then averaged. Following that, the baseline spectrum of the relevant buffer was subtracted to acquire the ultimate averaged spectrum. Afterward, plots specific to secondary structure were created by graphing the molar ellipticity in relation to the wavelength.
Structural analysis of recombinant enolase proteins from C. albicans and C. auris
Tertiary 3D structure of Enolase protein from C. auris was modeled by using SWISS-MODEL server (https://swissmodel.expasy.org) based on available suitable homologous protein structure templates. The crystal structure of C. albicans Enolase protein (PDB ID: 7V67) was chosen as a template for modeling C. auris Enolase protein. The modeled 3D structure of C. auris Enolase protein and C. albicans Enolase protein were superimposed using PyMOL 3D structure visualization software (http://www.pymol.org/) for comparison of their respective secondary structure assessment (percentage of alpha helices, beta sheets and random coils) and surface accessibility (DeLano, 2002). The physical and chemical properties (including amino acid composition, molecular weight, theoretical isoelectric point, instability coefficient, aliphatic index and grand average of hydropathicity [GRAVY]) of both Enolase proteins were analyzed using ProtParam tool (http://web.expasy.org/protparam/). The alignment of the tertiary structures for the two proteins was conducted using PyMOL software, and the Root Mean Square Deviation (RMSD) values of the superimposed enolase proteins were assessed. The tertiary structures of Enolase proteins from both C. albicans and C. auris were validated through Ramachandran plots using the PROCHECK program available on the SAVES 6.0 structure validation server (https://saves.mbi.ucla.edu).
B-cell epitope analysis of recombinant enolase proteins from C. albicans and C. auris
Linear B cell epitopes of Enolase proteins from C. albicans and C. auris were predicted by using in silico approach on BepiPred Linear Epitope Prediction 2.0 tool (http://tools.iedb.org/bcell/) available at IEDB web server (Jespersen et al., 2017). Amino acid sequences of both the Enolase proteins were selected, analyzed and the scoring threshold was set to 0.50.
For prediction of discontinuous B cell epitopes, a structure-based antibody prediction tool, DiscoTope (http://tools.iedb.org/discotope/), available at IEDB web server, was used to predict conformational B cell epitopes (Kringelum et al., 2012). This tool was used tertiary structure of Enolase protein from C. albicans, downloaded from RCSB PDB web server in PDB (PDB ID: 7V67) format (https://www.rcsb.org/structure/7V67). For conformational B cell epitopes prediction in C. auris Enolase protein, we used modeled tertiary structure from SWISS-MODEL in PDB format. For this analysis, a threshold of -3.7 was utilized to determine surface epitopes on both the Enolase proteins.
T-cell epitope analysis of recombinant enolase proteins from C. albicans and C. auris
We used IEDB web server to predict T cell epitopes within Enolase proteins from C. albicans and C. auris for both the MHC class I and MHC class II alleles respectively.
For the prediction of MHC class I binding CD8 T-cell epitopes, we used IEDB-recommended 2023.09 NetMHCpan 4.1 EL method (http://tools.iedb.org/mhci/) (Jakhar and Gakhar, 2020). The MHC class I binding score >0.75 is regarded as excellent for T-cell epitopes (Jakhar and Gakhar, 2020; Shukla et al., 2022).
We also investigated the CD4 T-cell epitopes (MHC class II binding) in both Enolase proteins from C. albicans and C. auris respectively, using the IEDB-recommended 2023.09 NetMHCIIpan 4.1 EL tool available on (http://tools.iedb.org/mhcii/). It predicts the binding affinity of all possible epitopes with the available MHC II allele dataset. The predicted output is given in units of IC50 nM for combinatorial library and SMM align, where lower number indicates higher affinity. Predicted peptides with IC50 values <50 nM are considered high affinity, <500 nM intermediate affinity and <5000 nM low affinity (Shukla et al., 2022).
Antigenicity and allergenicity prediction of enolase epitopes
The antigenicity values of linear B-cell and T-cell epitopes identified in Eno-albicans and Eno-auris proteins was predicted using VaxiJen server (version 2.0), (http://www.ddg-pharmfac.net/vaxijen/VaxiJen/VaxiJen.html). The antigenicity scores were obtained by selecting fungal target organism and using a default threshold value of 0.5. Epitopes exhibiting values higher than 0.5 were considered antigenic. The antigenic values of epitopes having less than 6 amino acid residues were not computed by VaxiJen (Doytchinova and Flower, 2007).
For predicting in silico allergenicity of the linear B-cell and T-cell epitopes identified in recombinant Enolase proteins from C. albicans and C. auris, AllerTOP server (version 2.0), (https://www.ddgpharmfac.net/AllerTOP/method.html) was used, selecting default parameters (Dimitrov et al., 2014).
Results
Cloning of recombinant enolase proteins from C. albicans and C. auris
Genomic DNA of the Candida species, C. albicans strain SC5314 (ATCC MYA-2876), and C. auris South Asian strain (Clade 1 CBS 470280) were extracted using a commercial kit as per manufacturer’s instructions. Gene specific primers were designed for amplifying the full-length Enolase genes. The forward and reverse primers were incorporated with unique restriction enzymes (BamH1 and PstI, respectively) for ensuring directional cloning, as outlined in Table 1. PCR amplification of full-length Enolase genes was performed using genomic DNA of C. albicans and C. auris. The amplicon sizes obtained for C. albicans, and C. auris Enolase genes were 1323 bp and 1320 bp, respectively (Figure 1A, B). After PCR amplification, the resulting amplicons were digested using BamH1 and PstI restriction enzymes and cloned into double-digested pQE30Xa vector, ensuring compatibility for directional cloning. The ligated constructs were then transformed into competent cells of E. coli XL-1 Blue strain. Colonies obtained post-transformation were cultured for plasmid isolation, and positive clones were identified through restriction analysis and verified by sequencing.
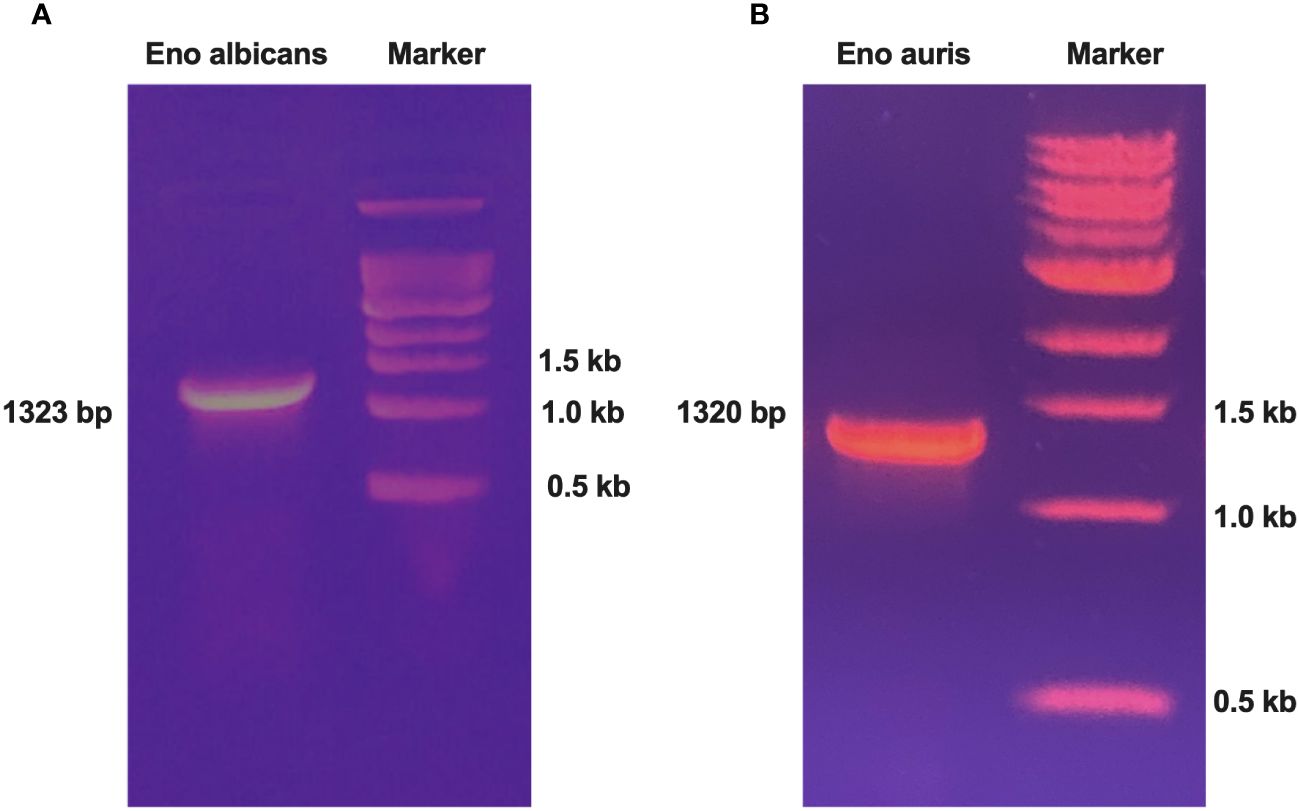
Figure 1 PCR amplification of Enolase genes from both C albicans and C auris strains. (A) PCR amplification of Enolase gene from C albicans (Eno-albicans: 1323 bp) and (B) PCR amplification of Enolase gene from C auris (Eno-auris: 1320 bp). Marker: 1 kb DNA ladder (last three bands of ladder are depicted).
Sequence alignment of recombinant enolase proteins from C. albicans and C. auris
The sequence alignment of Enolase proteins from C. albicans (Genbank accession number: AAB46358.1), C. auris (Genbank accession number KNE00479.1), C. tropicalis (Genbank accession number EER32738.1), C. parapsilosis (Genbank accession number CCE43078.1) and C. glabrata (Genbank accession number CAG59252.1) was performed using Clustal Omega and ESPRIPT 3. A high degree of homology was seen in Enolase sequences among these five Candida species. CLUSTAL alignment showed >80% identity between Enolase proteins between C. albicans and C. auris (Figure 2). Out of a total of 440 amino acids, 359 amino acids were identical between Eno-albicans and Eno-auris (81.6%), 29 amino acids were near identical (6.6%), and 52 amino acids were non-identical (11.8%).
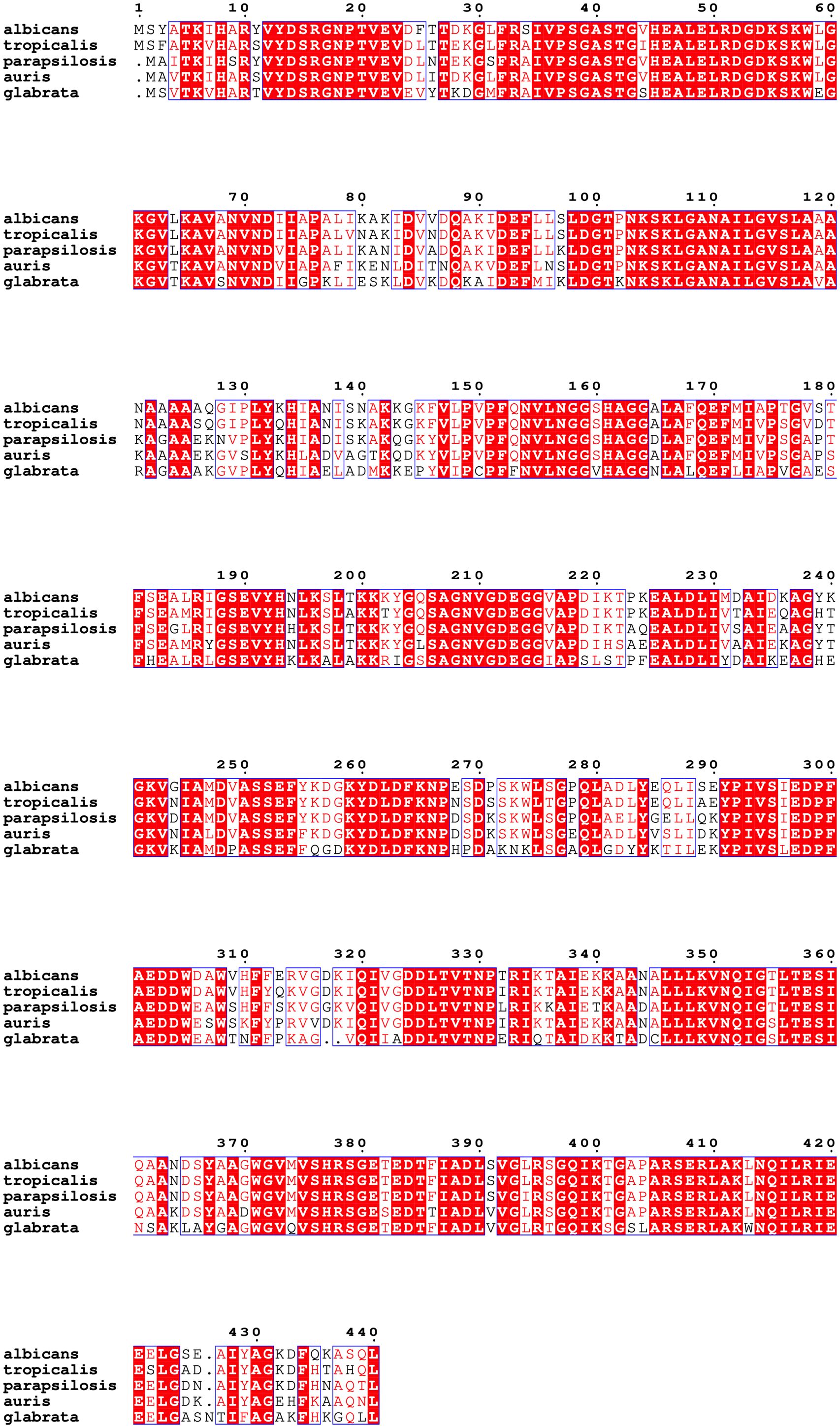
Figure 2 Multiple sequence alignment of full-length Enolase proteins from related Candida species. Amino acid sequences of Enolase from C. albicans (accession number: AAB46358.1) and C. auris (accession number: KNE00479.1) were aligned by Clustal Omega and are depicted using ESPript 3.0. Amino acid positions of Eno-albicans (1–440) and Eno-auris (1–439) are indicated and numbered according to Enolase of C. albicans. Universally conserved amino acid residues are depicted in white and marked with red highlights. Residues exhibiting a conservation rate exceeding 70% are enclosed within a blue box and indicated in red. Altered non-identical residues are unboxed and written in black.
Expression and purification of recombinant enolase proteins from C. albicans and C. auris
For protein expression, the histidine-tagged fusion protein constructs of Enolase belonging to C. albicans and C. auris were re-transformed in E. coli strain SG13009 (Qiagen). Small-scale E. coli bacterial cultures were then subjected to IPTG induction with continuous agitation using an orbital shaker. The optimal protein expression conditions were achieved at an IPTG concentration of 1 mM, a cell density (OD600) of 0.6 and an induction duration of 8 hours at 18°C. Following induction, both the un-induced and induced bacterial cultures were analyzed using SDS-PAGE electrophoresis. The induced Enolase proteins exhibited specific bands at approximately 51 kDa, consistent with the expected molecular weight of Enolase fusion proteins from both C. albicans and C. auris (Figure 3A, B).
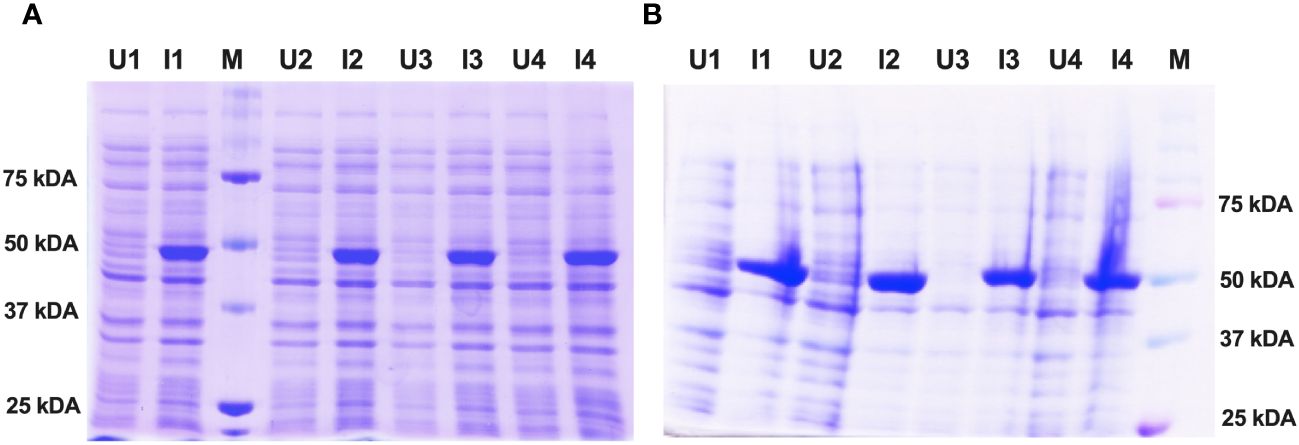
Figure 3 Expression and induction check of recombinant Enolase proteins from C albicans and C auris. (A) Induction of Eno-albicans protein: ~51 kDa; 440 aa. (B) Induction of Eno-auris protein: ~51 kDa; 439 aa. Lanes U: Uninduced samples from 4 bacterial colonies; Lanes I: Induced samples from 4 bacterial colonies; M: Protein Marker, Marker bands indicated on right.
The Enolase proteins from both C. albicans and C. auris were subsequently purified using Ni-NTA affinity chromatography following the manufacturer’s instructions (Qiagen) under soluble, native conditions. SDS PAGE analysis revealed fractions of the purified Eno-albicans protein obtained after passing the Ni-NTA column with wash (W1 and W2) and elution (E1 and E2) buffers (Figure 4A, B). The eluates of the purified Eno-auris protein recovered after passing the Ni-NTA column with wash (W1 and W2) and elution (E1 and E2) buffers was also analyzed on SDS PAGE (Figure 4C, D). The purified his-tagged Eno-albicans and Eno-auris proteins were obtained at approximately 51 kDa.
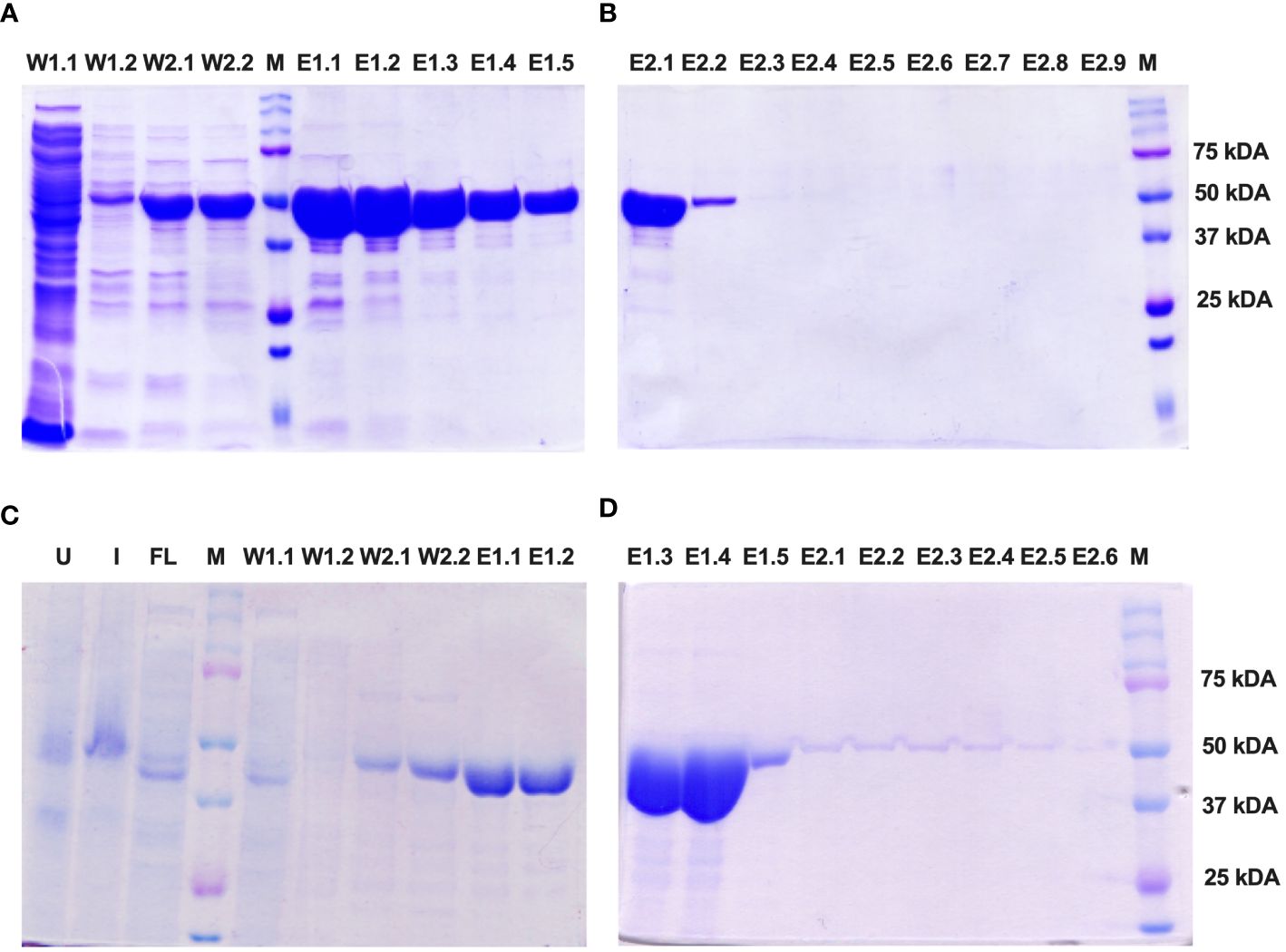
Figure 4 SDS-PAGE analysis of purified His-tagged Enolase proteins used in the study. Protein fractions obtained under native conditions (50 mM NaH2PO4, 300 mM NaCl, pH 8.0) with different concentrations of imidazole: 10 mM (Lysis), 20 mM (Wash 1), 50 mM (Wash 2), 100 mM (Elution 1) and 250 mM (Elution 2). (A, B) Fractions of Eno-albicans protein (440 aa; ~51 kDa). (C, D) Fractions of Eno-auris protein (439 aa; ~51 kDa). Lane U: Uninduced sample; Lane I: Induced sample; Lane FL: Flow through; Lanes W1-W2: Wash fractions; Lanes E1.1 to E1.5: five sequentially eluted fractions with E1 buffer; Lanes E2.1 to E2.5: five sequentially eluted fractions with E2 buffer; M: Protein Marker, Marker bands indicated on right.
Western blotting of recombinant enolase proteins from C. albicans and C. auris
The purified Enolase protein samples belonging to C. albicans and C. auris were stored at 4°C and subjected to dialysis for 12 hours at 4°C with an interval of 4 hours for changing the dialysis buffer. After dialysis, the purified Enolase fusion proteins (~51 kDa) were analyzed on SDS PAGE by Coomassie Blue staining (Figure 5A, C), transferred on nitrocellulose membranes, and confirmed through Western blot analysis using the anti-His antibody along with appropriate negative (BSA) and positive (PspA) controls (Figure 5B, D).
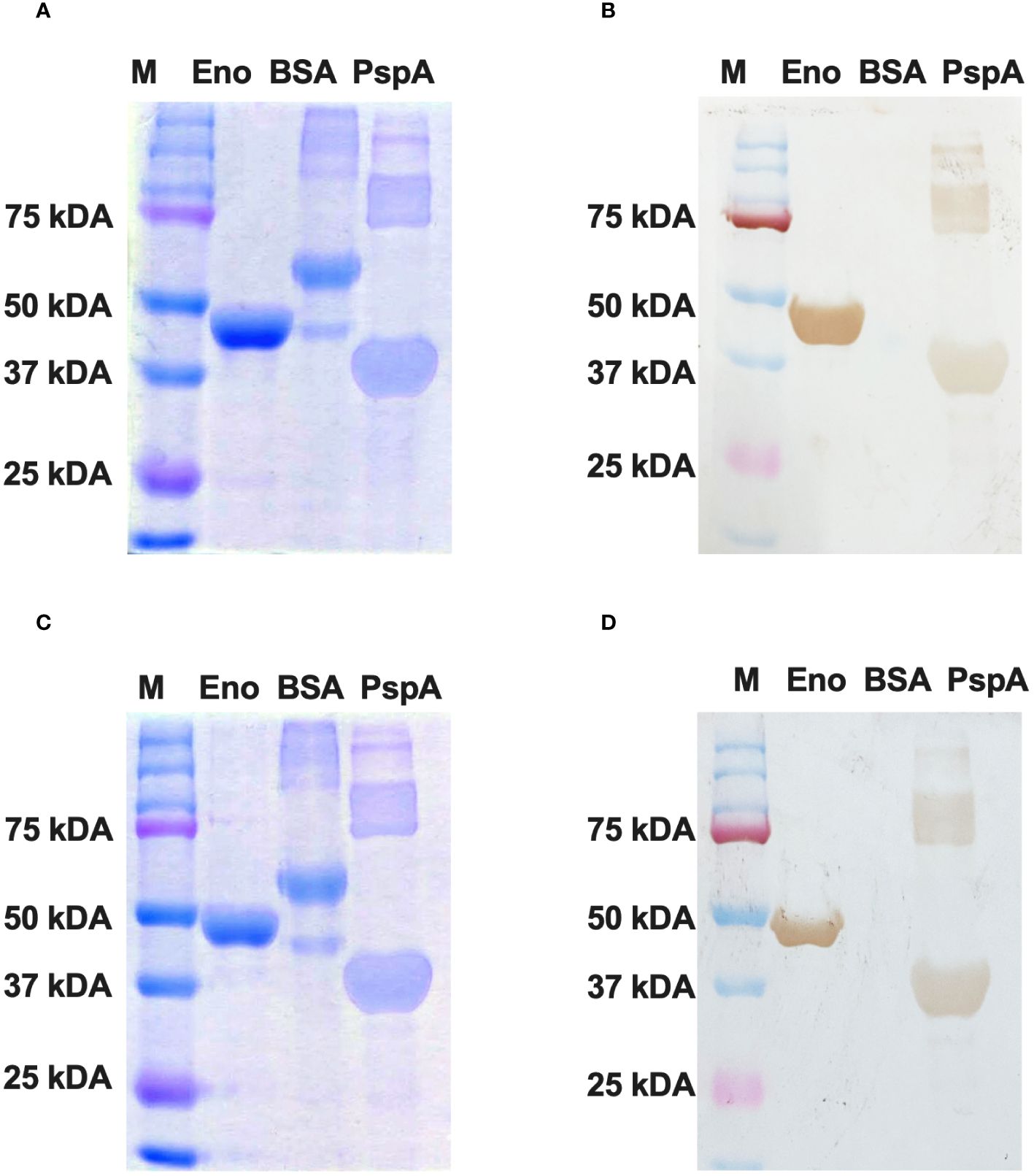
Figure 5 Western blotting of recombinant Enolase proteins used in the study. (A) SDS PAGE using Coomassie Brilliant Blue staining of Enolase protein from C albicans. (B) Western Blot of Eno-albicans protein using DAB substrate. (C) SDS PAGE using CBR staining of Enolase protein from C auris. (B) Western Blot of Eno-auris protein using DAB substrate. Histidine-tagged Eno-albicans and Eno-auris proteins obtained at ~51 kDa. PspA and BSA proteins are used as positive and negative controls, respectively. Protein Marker bands are indicated on right.
CD spectroscopy of recombinant enolase proteins from C. albicans and C. auris
CD spectroscopy was used to assess the secondary structure of the dialyzed Enolase proteins. The CD spectral data underwent analysis and deconvolution utilizing an online CD spectra algorithm. The CD spectra obtained for dialysed forms of the Enolase proteins from C. albicans (Figure 6A) and C. auris are shown (Figure 6B). Molar ellipticity is illustrated on the y-axis, while the wavelength is depicted on the x-axis. The CD spectra of yeast Enolase is characterized by double minimum (209 nm, 222 nm) and maximum (192 nm) peaks. The CD spectra analysis (Figure 6) of the dialyzed Enolase protein samples show presence of dip at around 209 nm and 220 nm, indicating existence of secondary structure elements, which are primarily α-helical. Overall, the CD spectra curves of Enolase proteins exhibits signature of an α-helix protein.
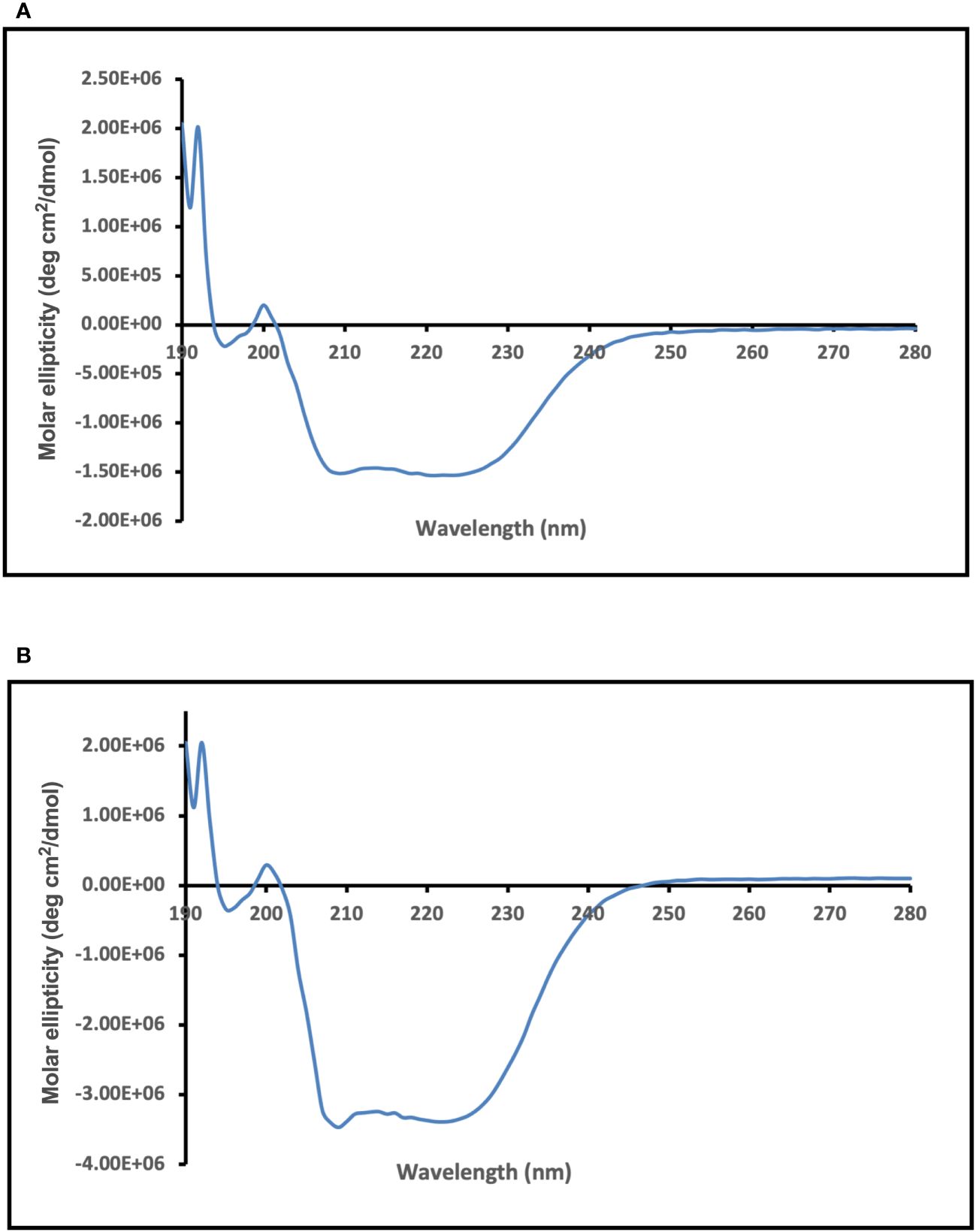
Figure 6 CD spectra analysis of purified and dialyzed recombinant Enolase protein samples used in the study. CD spectra obtained for (A) Eno-albicans and (B) Eno-auris proteins is shown. Molar ellipticity is depicted on the y-axis with wavelength on x-axis.
Structural analysis of recombinant enolase proteins from C. albicans and C. auris
The primary structures of both the recombinant Enolase proteins from C. albicans and C. auris were analyzed, and different parameters were computed using ExPasy ProtParam tool (Table 2). Amino acid sequence analysis provided valuable insights of amino acid composition, stability, hydrophobicity, and immunogenic properties of Enolase proteins from C. albicans and C. auris respectively. Upon comparing the physicochemical properties of both Enolase proteins, we observed minor differences in their isoelectric point (pI), instability index, aliphatic index and GRAVY score. The theoretical isoelectric point (pI) was found to be lower for C. auris Enolase protein (5.44) when compared to C. albicans Enolase protein (5.54). Additionally, C. auris Enolase protein (29.12) was classified as more stable than C. albicans Enolase protein (32.91) due to its low instability index. Aliphatic index is associated with increase in the thermostability of proteins, a higher aliphatic index value indicating more stability. As per their aliphatic index values, Eno-auris (93.83) protein was found to be more thermostable than the Eno-albicans (92.09) protein. Furthermore, we used GRAVY score to compare the hydrophobicity of both the Enolase proteins. We found negative GRAVY values for both Enolase proteins from C. albicans and C. auris, indicating their hydrophilic nature. Nevertheless, Eno-auris (-0.195) protein exhibits lower hydrophobicity score than the Eno-albicans (-0.192) protein, suggesting its higher probability of being exposed on the protein surface, and may be more accessible to antibodies and immune cells. The 3D structure of Enolase protein from C. auris was generated using SWISS-MODEL server. The tertiary structure of both Eno-albicans and Eno-auris proteins were visualized using PyMol software (Figure 7A, B). It was observed that Enolase protein from C. albicans is predominantly alpha helical, exhibiting the following composition: alpha helix (50.45%), beta sheets (16.36%) and random coils (33.18%). The secondary structure of Enolase protein from C. auris was similar to that of Eno-albicans, having the following composition: alpha helix (43.50%), beta sheets (21.64%), and random coils (34.85%) (Table 2). Both Eno-albicans and Eno-auris proteins had predominantly alpha helices, (indicated in cyan coloration) in their structures. Beta sheets (depicted in red), also contributes to the protein’s overall structural stability. The random coils regions, (depicted in magenta), connected the alpha helices and beta sheets. The tertiary structures of the two Enolase proteins were superimposed to assess homology (Figure 7C). The superimposed tertiary structures of the two Enolase proteins were aligned using PyMOL, revealing a RMSD value of 0.054 Å. This value indicates a relatively close structural similarity between the proteins (Kosloff and Kolodny, 2008). The tertiary structures of the two proteins were validated using PROCHECK analysis. The PROCHECK analysis produced Ramachandran plots for both Eno-albicans and Eno-auris proteins (Figure 7D, E). The plot statistics revealed that 91.3% and 91.4% of the residues were localized in the most favored regions [A, B, L] for Eno-albicans and Eno-auris, respectively. Additionally, 8.7% and 8.1% of residues were situated in the additional allowed regions [a, b, l, p] for Eno-albicans and Eno-auris, respectively. Ideally, a good quality model is expected to have over 90% of its residues in the most favored regions (Laskowski et al., 1996).
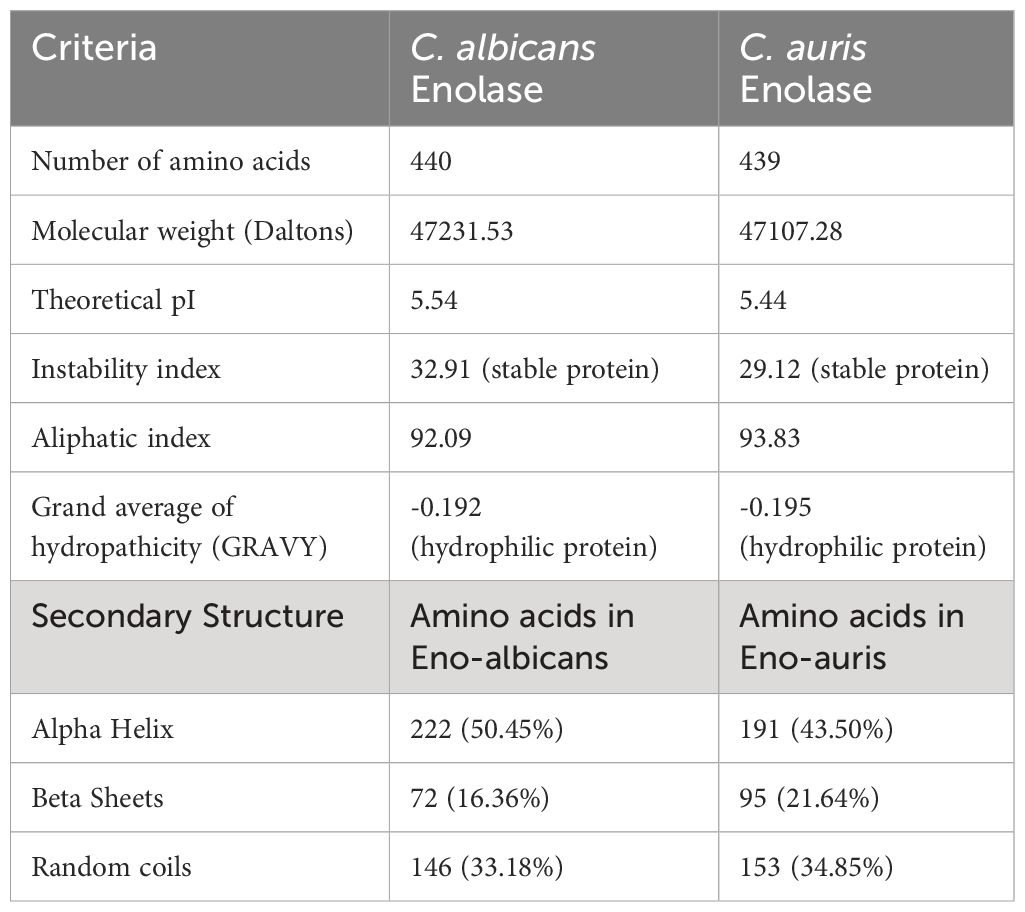
Table 2 Analysis of physicochemical properties of C. albicans and C. auris Enolase proteins using ProtParam server.
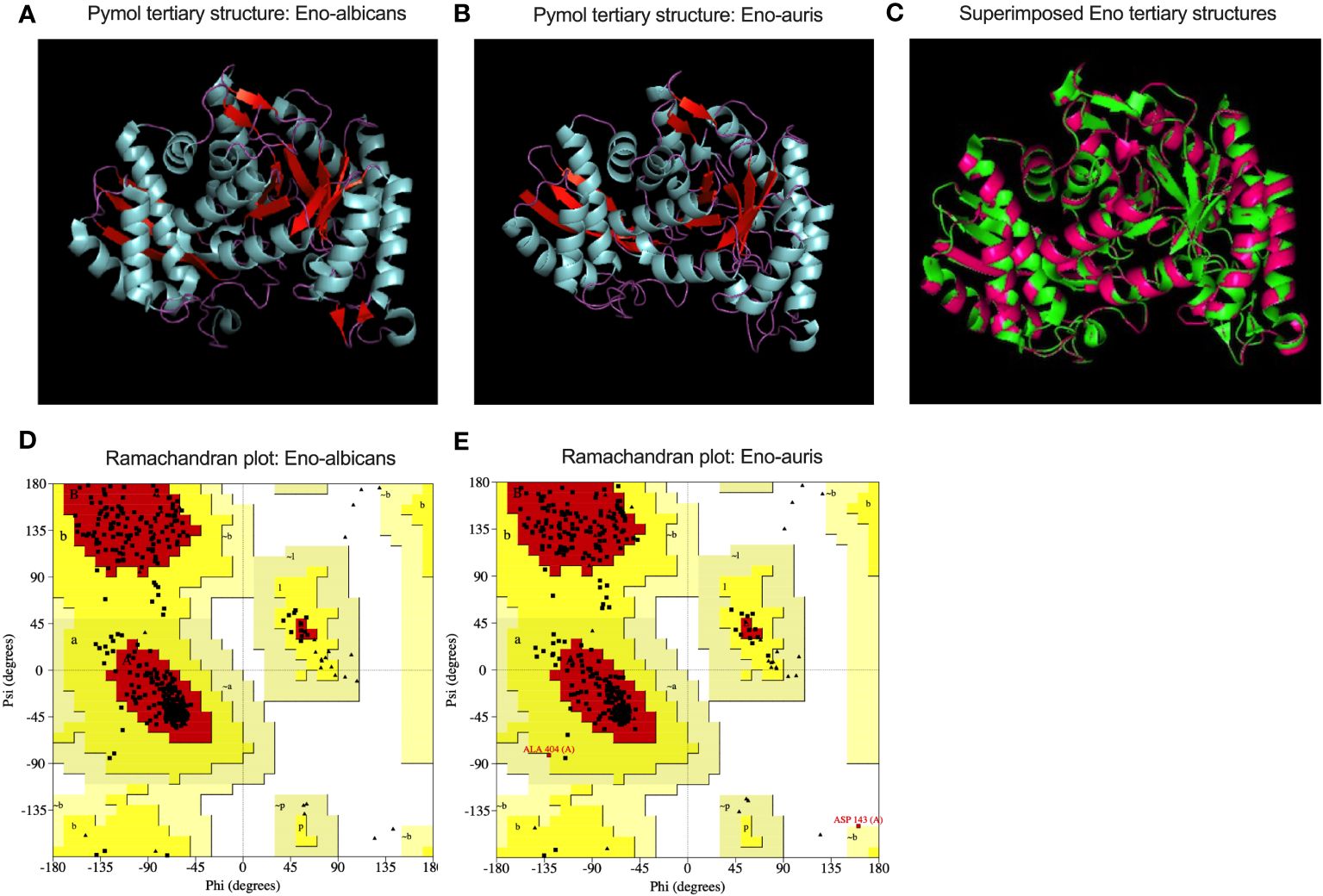
Figure 7 A cartoon view of the tertiary structural models of (A) Eno-albicans and (B) Eno-auris proteins is shown using Pymol. The amino acid residues corresponding to alpha helix, beta sheets and random coils are shown in cyan, red, and magenta, respectively, for both the Enolase proteins. (C) Superimposed tertiary structures of Eno-albicans and Eno-auris. (D) Ramachandran plot for Eno-albicans (E) Ramachandran plot for Eno-auris.
B-cell epitope analysis of recombinant enolase proteins from C. albicans and C. auris
The amino acid sequences of both C. albicans and C. auris Enolase proteins were computed for prediction of linear sequential B cell epitopes on BepiPred 2.0 server, using a threshold value of 0.5. The same threshold value was applied for the prediction of linear B-cell epitopes on the BepiPred-2.0 server for both the C. albicans Enolase protein and C. auris Enolase protein, resulting in the identification of 16 epitopes for each protein (Table 3). The analysis provided length and position of each linear B cell epitopes, which are outlined in Table 3. Antigenicity analysis of linear B-cell epitopes conducted via the VaxiJen server identified 8 epitopes in the Enolase protein from C. albicans and 7 epitopes in the Enolase protein from C. auris. Additionally, the assessment of linear B-cell epitope allergenicity using the AllerTOP server predicted 3 allergenic epitopes in the Enolase protein from C. albicans and 4 allergenic epitopes in the Enolase protein from C. auris.
Conformation-based B-cell epitopes were computed on DiscoTope 2.0 server available at IEDB web server, using tertiary structure of both the Enolase proteins. A threshold value of − 3.7 was chosen for the computation, which corresponds to default value. The analysis provided residue ID, residue name, contact number, propensity score, and DiscoTope score for each amino acid meeting the threshold value (Table 4). This tool predicted 23 and 16 discontinuous B cell epitopes in C. albicans Enolase and C. auris Enolase proteins, respectively (Table 4).
T-cell epitope analysis of recombinant enolase proteins from C. albicans and C. auris
Because MHC binding is essential for T-cell-mediated immune responses to antigenic peptides, we employed it as a parameter for forecasting potential antigenic peptides within recombinant Enolase proteins from C. albicans and C. auris, in our study.
To identify the CD8 T-cell epitopes binding to human MHC class I alleles, we employed the NetMHCpan EL 4.1 method (http://tools.iedb.org/mhci/, version 2023.09), as recommended by IEDB to forecast the MHC class I binding affinity of T-cell epitopes for the human MHC I alleles accessible on the IEDB server. This tool predicts the binding affinity of each peptide with various MHC alleles. We found 23,301 and 11,546 CD8 T-cell potential peptides in Enolase proteins from C. albicans and C. auris, respectively for 22 MHC I alleles. According to published data, binding score >0.75 for the MHC class I in T-cell epitopes is regarded as strong binders (Jakhar and Gakhar, 2020; Shukla et al., 2022). We identified 38 and 42 potential MHC I binding CD8 T-cell epitopes in Enolase proteins from C. albicans and C. auris, respectively, whose score value was >0.75 (Table 5). The Enolase protein from C. auris exhibits a higher number of CD8 T-cell epitopes for MHC I alleles compared to C. albicans Enolase protein. This finding indicates that the Enolase protein from C. auris might possess more antigenic properties or a higher immunogenicity potential for MHC I presentation than the Enolase protein from C. albicans. Evaluation of CD8 T-cell epitope antigenicity through the VaxiJen server revealed 16 epitopes in the Enolase protein from C. albicans and 19 epitopes in the Enolase protein from C. auris. Meanwhile, CD8 T-cell epitope allergenicity analysis conducted via the AllerTOP server predicted 21 allergenic epitopes in the Enolase protein from C. albicans and 23 allergenic epitopes in the Enolase protein from C. auris.
For identifying CD4 T-cell epitopes binding to MHC II alleles, we performed in silico analysis of both C. albicans and C. auris Enolase proteins using IEDB-recommended NetMHCIIpan 4.1 EL tool available on (http://tools.iedb.org/mhcii/, version 2023.09). The server predicted a total of 1477 and 1436 T-cell peptides in C. albicans and C. auris Enolase proteins, respectively for 13 MHC II binding alleles. By establishing a threshold below 50 nM IC50, we detected a combined total of 129 and 121 possible MHC II binding CD4 T-cell epitopes in C. albicans and C. auris Enolase proteins respectively, with good binding affinities (Table 6). The server prediction indicates that the CD4 T-cell epitopes for MHC II alleles were found to be comparable in both the Enolase proteins from C. albicans and C. auris. Such comparability in MHC II epitopes may imply similarities in immune responses or interactions with host immune cells mediated by MHC II presentation, between C. albicans and C. auris infections. Evaluation of CD4 T-cell epitope antigenicity through the VaxiJen server revealed 77 epitopes in the Enolase protein from C. albicans and 69 epitopes in the Enolase protein from C. auris. Meanwhile, CD8 T-cell epitope allergenicity analysis conducted via the AllerTOP server predicted 58 allergenic epitopes in the Enolase protein from C. albicans and 37 allergenic epitopes in the Enolase protein from C. auris.
Discussion
This research work focuses on the molecular cloning, protein expression, followed by purification of recombinant Enolase proteins from both C. albicans and C. auris, along with in silico prediction and identification of B-cell and T-cell epitopes. Our study contributes to the ongoing efforts to develop effective vaccines for preventing and controlling infections caused by the multidrug-resistant C. auris infections. Further, our results may also help in developing improved diagnostic strategies for C. auris infections. C. auris has emerged as a significant concern due to its ability to cause systemic infections, its propensity for spreading within healthcare settings and is associated with high morbidity and mortality across the globe (Chowdhary et al., 2023). Since its initial discovery in Japan in 2009, C. auris has rapidly spread across the globe, causing outbreaks in healthcare facilities in various countries (Spivak and Hanson, 2018). It exhibits significant genetic diversity, with six distinct clades namely South Asian (I), East Asian (II), African (III), South American (IV), Iran (V) and Singapore (VI) identified across different geographic regions (Narayanan et al., 2022; Spruijtenburg et al., 2022; Suphavilai et al., 2023). Unlike other Candida species, C. auris is resistant to multiple classes of antifungal treatments including azoles, echinocandins, and amphotericin B, making it difficult to treat. Identifying C. auris infections can be challenging, as it may be misidentified as other Candida species using conventional laboratory methods, lead to delayed diagnosis and inappropriate treatment, potentially exacerbating the spread of the infection. Despite advancements in understanding host defense mechanisms, invasive infections caused by C. auris remain poorly understood. Improved understanding of host immune responses during C. auris infections has important clinical implications, including the development of immunotherapeutic associated with systemic candidiasis (Pechacek and Lionakis, 2023).
Currently, there are no approved immunotherapeutic strategies/vaccines specifically designed for the treatment of systemic candidiasis, including those caused by multidrug-resistant C. auris. Of note, host-directed therapy and vaccine development (NDV-3) for C. auris mediated systemic candidiasis is still in progress (Singh et al., 2019). Akhtar et al. used bioinformatics tools and databases to identify potential antigenic epitopes from agglutinin-like sequence-3 (Als3) proteins expressed by C. auris (Akhtar et al., 2021). In addition to Als3, the Enolase protein exhibits significant conservation across various Candida species, positioning it as a prominent vaccine candidate for systemic candidiasis. This recognition makes it a promising focus for the development of broad-spectrum vaccines (Shukla et al., 2021b). Xin et al. conducted a study demonstrating the creation of synthetic glycopeptide vaccines that combine beta-mannan with peptide epitopes derived from the Enolase protein of C. albicans. These vaccines were shown to provide protection against systemic candidiasis in a mouse model (Xin et al., 2008). Besides being considered a potential vaccine candidate, Enolase protein also has a role in diagnosing systemic candidiasis infections (He et al., 2015). The immunogenic ability of Enolase is well documented and several studies have investigated the use of recombinant Enolase protein from C. albicans for diagnostic potential (Laín et al., 2007; Pitarch et al., 2014). Detection of Enolase-specific antibodies has been used in the diagnosis of systemic candidiasis in multiple reports (van Deventer et al., 1994; Li et al., 2013). As such, recombinant Enolase protein belonging to Candida species exhibits both diagnostic and therapeutic potential against systemic Candida infections.
In this study, we successfully cloned and expressed recombinant Enolase proteins from South Asian strain of C. auris (clade I CBS 470280) and C. albicans reference strain (SC5314) in E. coli. The recombinant Enolase protein from C. albicans has previously been cloned and expressed using prokaryotic (Mason et al., 1993) and eukaryotic expression systems (Franklyn and Warmington, 1993) and characterized (Sandini et al., 1999). Another study has characterized the sequence and structural features of the C. krusei Enolase protein using in silico methods (Gandhi et al., 2008). We performed structural and immunoinformatics analysis (involving in silico B-cell and T-cell epitope analysis) for comparing the two proteins. In silico immunoinformatics analysis indicated that the B-cell and T-cell epitopes were comparable in both the Enolase proteins from C. albicans and C. auris. We used BepiPred and DiscoTope servers available at IEDB to predict the linear and discontinuous B-cell epitopes, respectively. The antigenicity and allergenicity analyses for the identified epitopes were performed using VaxiJen and AllerTOP servers, respectively (Mir et al., 2022). Enolase protein from C. auris was observed to have less discontinuous epitopes than the Enolase protein of C. albicans in this study. It can be explained by the fact that their precise number and locations cannot be determined due to the lack of experimental structural data of this protein. We used modeled tertiary structure of the Enolase protein from C. auris using the Swiss Model server with an 82% template similarity with C. albicans Enolase protein, offering valuable insights into potential epitope distribution. However, the exact disposition of discontinuous epitopes remains uncertain without experimental validation. We used NetMHCpan 4.1 EL and NETMHCIIpan 4.1 EL prediction tools available at IEDB for predicting the Enolase CD8 and CD4 T-cell epitopes, respectively. While both the total number and antigenic CD8 and CD4 T-cell epitopes were comparable between Eno-albicans and Eno-auris proteins, the Enolase protein from C. auris exhibited lesser number of allergenic epitopes compared to the protein from C. albicans. The crystal structure of Enolase protein from C. albicans is available and provides valuable insights into its pathogenesis, virulence, and potential as a therapeutic vaccine candidate (Li et al., 2022). The study by Langenhorst et al. highlighted the immunostimulatory and therapeutic potential of Enolase protein from C. albicans on human and mouse immune cells (Langenhorst et al., 2023). Another study demonstrated direct modulation of T cell responses against C. albicans infections (Daud et al., 2023). Our results suggest that Enolase epitopes augers well for designing an improved anti-Candida vaccine. However, a multi-epitope vaccine comprising additional epitopes from various other immunogenic proteins would enhance vaccine efficacy. Along with Enolase, we recommend specific proteins such as secreted aspartyl proteinase (Sap2), agglutinin like sequence gene (Als3), heat shock protein (Hsp90), hyphally-regulated protein (Hyr1), hyphal wall protein (Hwp1), phospholipase (PLB), pyruvate kinase (Pk), fructose bisphosphate aldolase (Fba1), superoxide dismutase gene (Sod5), malate dehydrogenase (Mdh1), etc. and their epitopes for inclusion in such a combination approach, based on their known immunogenicity and relevance to Candida infection (Shukla et al., 2021b).
Enolase protein holds promise as both a diagnostic marker and a therapeutic target against multidrug resistance Candida infections. Further research into the development of Enolase protein based diagnostic assays, and multi-epitope vaccines in preclinical and clinical studies may contribute to improved strategies for diagnosing, preventing, and treating Candida infections in clinical settings. Overall, this study lays the groundwork for further investigation into the immunogenicity and protective efficacy of Enolase-based vaccines against Candida infections. These findings underscore the potential of Enolase as a versatile vaccine candidate for combating Candida-associated diseases and highlight the importance of molecular approaches in vaccine development against fungal pathogens. The emergence of Covid associated fungal infections and global warming increasing C. auris infections has provided momentum to fungal vaccine research. The availability of an improved multivalent and/or multi-epitope vaccine for pan-fungal infections is on the horizon, within next 10 years, hopefully.
Data availability statement
The original contributions presented in the study are included in the article/supplementary material. Further inquiries can be directed to the corresponding author.
Author contributions
MS: Data curation, Formal analysis, Investigation, Methodology, Visualization, Writing – original draft. RS: Data curation, Formal analysis, Investigation, Visualization, Writing – review & editing. PC: Data curation, Investigation, Visualization, Writing – review & editing. SR: Conceptualization, Formal analysis, Methodology, Project administration, Resources, Supervision, Validation, Writing – review & editing.
Funding
The author(s) declare financial support was received for the research, authorship, and/or publication of this article. The authors acknowledge UGC-JRF Fellowship to RS and ICMR-JRF Fellowship to PC.
Acknowledgments
The authors acknowledge all the facilities at IIT-Roorkee and PTSNS University.
Conflict of interest
The authors declare that the research was conducted in the absence of any commercial or financial relationships that could be construed as a potential conflict of interest.
Publisher’s note
All claims expressed in this article are solely those of the authors and do not necessarily represent those of their affiliated organizations, or those of the publisher, the editors and the reviewers. Any product that may be evaluated in this article, or claim that may be made by its manufacturer, is not guaranteed or endorsed by the publisher.
References
Akhtar N., Joshi A., Kaushik V., Kumar M., Mannan M. A. (2021). In-silico design of a multivalent epitope-based vaccine against Candida auris. Microb. Pathog. 155, 104879. doi: 10.1016/j.micpath.2021.104879
Calandra T., Roberts J. A., Antonelli M., Bassetti M., Vincent J. L. (2016). Diagnosis and management of invasive candidiasis in the ICU: an updated approach to an old enemy. Crit. Care 20, 125. doi: 10.1186/s13054-016-1313-6
Chandley P., Subba P., Rohatgi S. (2022). A soluble tetrazolium-based reduction assay to evaluate the effect of antibodies on candida tropicalis biofilms. J. Visual. Exper. 10, 187. doi: 10.3791/64425
Chowdhary A., Jain K., Chauhan N. (2023). Candida auris genetics and emergence. Annu. Rev. Microbiol. 77, 583–602. doi: 10.1146/annurev-micro-032521-015858
Daud M., Dasari P., Adelfinger M., Langenhorst D., Lother J., Slavkovic-Lukic D., et al. (2023). Enolase 1 of Candida albicans binds human CD4+ T cells and modulates naïve and memory responses. Eur. J. Immunol. 53, e2250284. doi: 10.1002/eji.202250284
DeLano W. L. (2002). Pymol: An open-source molecular graphics tool. CCP4 Newsl Protein Crystallogr. 40, 82–92.
Dimitrov I., Bangov I., Flower D. R., Doytchinova I. (2014). AllerTOP v.2–a server for in silico prediction of allergens. J. Mol. Model. 20, 2278. doi: 10.1007/s00894-014-2278-5
Doytchinova I. A., Flower D. R. (2007). VaxiJen: a server for prediction of protective antigens, tumour antigens and subunit vaccines. BMC Bioinf. 8, 4. doi: 10.1186/1471-2105-8-4
Franklyn K. M., Warmington J. R. (1993). Cloning and nucleotide sequence analysis of the Candida albicans enolase gene. FEMS Microbiol. Lett. 111, 101–107. doi: 10.1111/fml.1993.111.issue-1
Funk J., Schaarschmidt B., Slesiona S., Hallström T., Horn U., Brock M. (2016). The glycolytic enzyme enolase represents a plasminogen-binding protein on the surface of a wide variety of medically important fungal species. Int. J. Med. Microbiol. 306, 59–68. doi: 10.1016/j.ijmm.2015.11.005
Gandhi N. S., Young K., Warmington J. R., Mancera R. L. (2008). Characterization of sequence and structural features of the Candida krusei enolase. Silico Biol. 8, 449–460.
He Z. X., Chen J., Li W., Cheng Y., Zhang H. P., Zhang L. N., et al. (2015). Serological response and diagnostic value of recombinant candida cell wall protein enolase, phosphoglycerate kinase, and β-glucosidase. Front. Microbiol. 6, 920. doi: 10.3389/fmicb.2015.00920
He Z., Piao J., Qiu Y., Lei D., Yang Y., Shi L., Wang F. (2022). Investigation of the location and secretion features of Candida albicans enolase with monoclonal antibodies. Ann. Microbiol. 72, 25. doi: 10.1186/s13213-022-01682-8
Jakhar R., Gakhar S. K. (2020). An immunoinformatics study to predict epitopes in the envelope protein of SARS-CoV-2. Can. J. Infect. Dis. Med. Microbiol. 2020, 7079356. doi: 10.1155/2020/7079356
Jespersen M. C., Peters B., Nielsen M., Marcatili P. (2017). BepiPred-2.0: improving sequence-based B-cell epitope prediction using conformational epitopes. Nucleic Acids Res. 45, W24–W29. doi: 10.1093/nar/gkx346
Jong A. Y., Chen S. H. M., Stins M. F., Kim K. S., Tuan T. L., Huang S. H. (2003). Binding of Candida albicans enolase to plasmin(ogen) results in enhanced invasion of human brain microvascular endothelial cells. J. Med. Microbiol. 52, 615–622. doi: 10.1099/jmm.0.05060-0
Karkowska-Kuleta J., Kulig K., Karnas E., Zuba-Surma E., Woznicka O., Pyza E., et al. (2020). Characteristics of Extracellular Vesicles Released by the Pathogenic Yeast-Like Fungi Candida glabrata, Candida parapsilosis and Candida tropicalis. Cells 9, 1722. doi: 10.3390/cells9071722
Karkowska-Kuleta J., Wronowska E., Satala D., Zawrotniak M., Bras G., Kozik A., et al. (2021). Als3-mediated attachment of enolase on the surface of Candida albicans cells regulates their interactions with host proteins. Cell Microbiol. 23, e13297. doi: 10.1111/cmi.13297
Ko H. C., Hsiao T. Y., Chen C. T., Yang Y. L. (2013). Candida albicans ENO1 null mutants exhibit altered drug susceptibility, hyphal formation, and virulence. J. Microbiol. 51, 345–351. doi: 10.1007/s12275-013-2577-z
Kosloff M., Kolodny R. (2008). Sequence-similar, structure-dissimilar protein pairs in the PDB. Proteins 71, 891–902. doi: 10.1002/prot.21770
Kringelum J. V., Lundegaard C., Lund O., Nielsen M. (2012). Reliable B cell epitope predictions: impacts of method development and improved benchmarking. PloS Comput. Biol. 8, e1002829. doi: 10.1371/journal.pcbi.1002829
Laín A., Moragues M. D., Ruiz J. C., Mendoza J., Camacho A., Del Palacio A., et al. (2007). Evaluation of a novel enzyme-linked immunosorbent assay to detect immunoglobulin G antibody to enolase for serodiagnosis of invasive candidiasis. Clin. Vaccine Immunol. 14, 318–319. doi: 10.1128/CVI.00396-06
Langenhorst D., Fürst A. L., Alberter K., Vilhena C., Dasari P., Daud M., et al. (2023). Soluble Enolase 1 of Candida albicans and Aspergillus fumigatus Stimulates Human and Mouse B Cells and Monocytes. J. Immunol. 211, 804–815. doi: 10.4049/jimmunol.2200318
Laskowski R. A., MacArthur M. W., Moss D. S., Thornton J. M. (1996). PROCHECK-a program to check the stereochemical quality of protein structures. J. Appl. Cryst. 26, 283–291. doi: 10.1107/S0021889892009944
Leu S. J., Lee Y. C., Lee C. H., Liao P. Y., Chiang C. W., Yang C. M., et al. (2020). Generation and Characterization of Single Chain Variable Fragment against Alpha-Enolase of Candida albicans. Int. J. Mol. Sci. 21, 2903. doi: 10.3390/ijms21082903
Li W.q., Hu X.c., Zhang X., Ge Y., Zhao S., Hu Y., et al. (2011). Immunisation with the glycolytic enzyme enolase confers effective protection against Candida albicans infection in mice. Vaccine 29, 5526–5533. doi: 10.1016/j.vaccine.2011.05.030
Li L., Lu H., Zhang X., Whiteway M., Wu H., Tan S., et al. (2022). Baicalein Acts against Candida albicans by Targeting Eno1 and Inhibiting Glycolysis. Microbiol. Spectr. 10, e0208522. doi: 10.1128/spectrum.02085-22
Li F. Q., Ma C. F., Shi L. N., Lu J. F., Wang Y., Huang M., et al. (2013). Diagnostic value of immunoglobulin G antibodies against Candida enolase and fructose-bisphosphate aldolase for candidemia. BMC Infect. Dis. 13, 253. doi: 10.1186/1471-2334-13-253
Lim S. J., Mohamad Ali M. S., Sabri S., Muhd Noor N. D., Salleh A. B., Oslan S. N. (2021). Opportunistic yeast pathogen Candida spp.: Secreted and membrane-bound virulence factors. Med. Mycol. 59, 1127–1144. doi: 10.1093/mmy/myab053
Mason A. B., Buckley H. R., Gorman J. A. (1993). Molecular cloning and characterization of the Candida albicans enolase gene. J. Bacteriol. 175, 2632–2639. doi: 10.1128/jb.175.9.2632-2639.1993
Mir S. A., Alaidarous M., Alshehri B., Bin Dukhyil A. A., Banawas S., Madkhali Y., et al. (2022). Immunoinformatics-based identification of B and T cell epitopes in RNA-dependent RNA polymerase of SARS-cov-2. Vaccines (Basel). 10, 1660. doi: 10.3390/vaccines10101660
Montagnoli C., Sandini S., Bacci A., Romani L., La Valle R. (2004). Immunogenicity and protective effect of recombinant enolase of Candida albicans in a murine model of systemic candidiasis. Medical Mycology. 42 (4) 319–24. doi: 10.1080/13693780310001644653
Narayanan A., Selvakumar P., Siddharthan R., Sanyal K. (2022). ClaID: a rapid method of clade-level identification of the multidrug resistant human fungal pathogen candida auris. Microbiol. Spectr. 10, e0063422. doi: 10.1128/spectrum.00634-22
Núñez-Beltrán A., López-Romero E., Cuéllar-Cruz M. (2017). Identification of proteins involved in the adhesion of Candida species to different medical devices. Microb. Pathog. 107, 293–303. doi: 10.1016/j.micpath.2017.04.009
Papon N., Courdavault V., Clastre M., Bennett R. J. (2013). Emerging and emerged pathogenic Candida species: beyond the Candida albicans paradigm. PloS Pathog. 9, e1003550. doi: 10.1371/journal.ppat.1003550
Pappas P. G., Lionakis M. S., Arendrup M. C., Ostrosky-Zeichner L., Kullberg B. J. (2018). Invasive candidiasis. Nat. Rev. Dis. Prim. 4, 1–20. doi: 10.1038/nrdp.2018.26
Pechacek J., Lionakis M. S. (2023). Host defense mechanisms against Candida auris. Expert Rev. Anti Infect. Ther. 21, 1087–1096. doi: 10.1080/14787210.2023.2264500
Pitarch A., Nombela C., Gil C. (2014). Serum antibody signature directed against Candida albicans Hsp90 and enolase detects invasive candidiasis in non-neutropenic patients. J. Proteome Res. 13, 5165–5184. doi: 10.1021/pr500681x
Reyna-Beltrán E., Iranzo M., Calderón-González K. G., Mondragón-Flores R., Labra-Barrios M. L., Mormeneo S., et al. (2018). The Candida albicans ENO1 gene encodes a transglutaminase involved in growth, cell division, morphogenesis, and osmotic protection. J. Biol. Chem. 293, 4304–4323. doi: 10.1074/jbc.M117.810440
Sandini S., Melchionna R., Arancia S., Gomez M. J., La Valle R. (1999). Generation of a highly immunogenic recombinant enolase of the human opportunistic pathogen Candida albicans. Biotechnol. Appl. Biochem. 29, 223–227. doi: 10.1111/j.1470-8744.1999.tb00553.x
Satala D., Karkowska-Kuleta J., Zelazna A., Rapala-Kozik M., Kozik A. (2020a). Moonlighting proteins at the candidal cell surface. Microorganisms 8, 1–25. doi: 10.3390/microorganisms8071046
Satala D., Satala G., Karkowska-Kuleta J., Bukowski M., Kluza A., Rapala-Kozik M., et al. (2020b). Structural insights into the interactions of candidal enolase with human vitronectin, fibronectin and plasminogen. Int. J. Mol. Sci. 21, 7843. doi: 10.3390/ijms21217843
Shibasaki S., Karasaki M., Tafuku S., Aoki W., Sewaki T., Ueda M. (2014). Oral Immunization Against Candidiasis Using Lactobacillus casei Displaying Enolase 1 from Candida albicans. Sci. Pharm. 82, 697–708. doi: 10.3797/(ISSN)0036-8709
Shukla M., Chandley P., Kaur H., Ghosh A. K., Shivaprakash M. R., Rohatgi S. (2021a). Expression and purification along with evaluation of serological response and diagnostic potential of recombinant Sap2 protein from C. parapsilosis for use in systemic candidiasis. J. Fungi 7, 999. doi: 10.3390/jof7120999
Shukla M., Chandley P., Rohatgi S. (2021b). The role of B cells and antibodies against candidate vaccine antigens in invasive candidiasis. Vaccines 9, 1159. doi: 10.3390/vaccines9101159
Shukla M., Chandley P., Tapryal S., Kumar N., Mukherjee S. P., Rohatgi S. (2022). Expression, purification and refolding of Chikungunya virus full length envelop E2 protein along with B-cell and T-cell epitope analysis using immuno-informatics approaches. ACS Omega 7, 3491–3513. doi: 10.1021/acsomega.1c05975
Shukla M., Rohatgi S. (2020). Vaccination with secreted aspartyl proteinase 2 protein from Candida parapsilosis can enhance survival of mice during Candida tropicalis mediated systemic candidiasis. Infect. Immun. 88, e00312. doi: 10.1128/IAI.00312-20
Silva R. C., Padovan A. C. B., Pimenta D. C., Ferreira R. C., da Silva C. V., Briones M. R. S. (2014). Extracellular enolase of Candida albicans is involved in colonization of mammalian intestinal epithelium. Front. Cell. Infect. Microbiol. 4, 1–9. doi: 10.3389/fcimb.2014.00066
Singh S., Uppuluri P., Mamouei Z., Alqarihi A., Elhassan H., French S., et al. (2019). The NDV-3A vaccine protects mice from multidrug resistant Candida auris infection. PloS Pathog. 15, e1007460. doi: 10.1371/journal.ppat.1007460
Soriano A., Honore P. M., Puerta-Alcalde P., Garcia-Vidal C., Pagotto A., Gonçalves-Bradley D. C., et al. (2023). Invasive candidiasis: current clinical challenges and unmet needs in adult populations. J. Antimicrobial. Chemother. 78, 1569–1585. doi: 10.1093/jac/dkad139
Spivak E. S., Hanson K. E. (2018). Candida auris: an emerging fungal pathogen. J. Clin. Microbiol. 56, e01588–e01517. doi: 10.1128/JCM.01588-17
Spruijtenburg B., Badali H., Abastabar M., Mirhendi H., Khodavaisy S., Sharifisooraki J., et al. (2022). Confirmation of fifth Candida auris clade by whole genome sequencing. Emerg. Microbes Infect. 11, 2405–2411. doi: 10.1080/22221751.2022.2125349
Sundstrom P., Jensen J., Balish E. (1994). Humoral and cellular immune responses to enolase after alimentary tract colonization or intravenous immunization with candida albicans. J. Infect. Dis. 170, 390–395. doi: 10.1093/infdis/170.2.390
Suphavilai C., Ko K., Lim K., Tan M., Boonsimma P., Chu J., et al. (2023). Discovery of the sixth Candida auris clade in Singapore. medRxiv, 23293435. doi: 10.1101/2023.08.01.23293435
Van Deventer H. J., Goessens W. H., Van Vliet A. J., Verbrugh H. A. (1996). Anti-enolase antibodies partially protective against systemic candidiasis in mice. Clin. Microbiol. Infect. 2, 36–43. doi: 10.1111/j.1469-0691.1996.tb00198.x
van Deventer A. J., van Vliet H. J., Hop W. C., Goessens W. H. (1994). Diagnostic value of anti-Candida enolase antibodies. J. Clin. Microbiol. 32, 17–23. doi: 10.1128/jcm.32.1.17-23.1994
Wan Tso G. H., Reales-Calderon J. A., Pavelka N. (2018). The Elusive Anti-Candida Vaccine: Lessons from the past and opportunities for the future. Front. Immunol. 9. doi: 10.3389/fimmu.2018.00897
Warnock D. W. (2007). Trends in the epidemiology of invasive fungal infections. Jpn. J. Med. Mycol. 48, 1–12. doi: 10.3314/jjmm.48.1
Whibley N., Gaffen S. L. (2015). Beyond Candida albicans: mechanisms of immunity to non-albicans Candida species. Cytokine 76, 42–52. doi: 10.1016/j.cyto.2015.07.025
Keywords: Candida auris, systemic candidiasis, vaccine candidate, enolase, epitopes, Candida albicans
Citation: Shukla M, Singh R, Chandley P and Rohatgi S (2024) Molecular cloning, expression, and purification, along with in silico epitope analysis of recombinant enolase proteins (a potential vaccine candidate) from Candida albicans and Candida auris. Front. Fungal Biol. 5:1399546. doi: 10.3389/ffunb.2024.1399546
Received: 12 March 2024; Accepted: 16 May 2024;
Published: 31 May 2024.
Edited by:
Srivastava Vartika, Cleveland Clinic, United StatesReviewed by:
Thomas Dandekar, Julius Maximilian University of Würzburg, GermanyPriya P, National Institute of Technology Warangal, India
Copyright © 2024 Shukla, Singh, Chandley and Rohatgi. This is an open-access article distributed under the terms of the Creative Commons Attribution License (CC BY). The use, distribution or reproduction in other forums is permitted, provided the original author(s) and the copyright owner(s) are credited and that the original publication in this journal is cited, in accordance with accepted academic practice. No use, distribution or reproduction is permitted which does not comply with these terms.
*Correspondence: Soma Rohatgi, c29tYS5yb2hhdGdpQGJ0LmlpdHIuYWMuaW4=