- 1Commonwealth Scientific and Industrial Research Organisation (CSIRO), Brisbane, QLD, Australia
- 2Department of Agriculture and Fisheries, Biosecurity Queensland, Brisbane, QLD, Australia
- 3Department of Agriculture and Fisheries, Plant Pathology Herbarium, Brisbane, QLD, Australia
There are five closely related Sporobolus species, collectively known as weedy Sporobolus grasses (WSG) or the rat’s tail grasses. They are fast growing, highly competitive, unpalatable weeds of pastures, roadsides and woodlands. An effective biological control agent would be a welcomed alternative to successive herbicide application and manual removal methods. This study describes the initial exploratory phase of isolating and identifying native Australian microfungi associated with WSG, prior to evaluating their efficacy as inundative biological control agents. Accurate species-level identification of plant-pathogenic microfungi associated with WSG is an essential first step in the evaluation and prioritisation of pathogenicity bioassays. Starting with more than 79 unique fungal morphotypes isolated from diseased Sporobolus grasses in Queensland, Australia, we employed multi-locus phylogenetic analyses to classify these isolates into 54 fungal taxa. These taxa belong to 22 Ascomycete families (12 orders), of which the majority fall within the Pleosporales (>24 taxa in 7 families). In the next phase of the study, the putative species identities of these taxa will allow us to prioritise those which are likely to be pathogenic based on existing literature and their known ecological roles. This study represents the first step in a systematic, high-throughput approach to finding potential plant pathogenic biological control agents.
1 Introduction
Five species of Sporobolus grasses (S. africanus, S. fertilis, S. jacquemontii, S. natalensis, and S. pyramidalis) are collectively recognised as the weedy Sporobolus grasses (WSG) or rat’s tail grasses across Australia (Palmer, 2012; Biosecurity Queensland, 2018). These five species, together with the native Australian species S. blakei, S. creber, S. elongatus, S. laxus, and S. sessilis (Simon and Jacobs, 1999; Peterson et al., 2014), belong to the S. indicus complex (Hetherington and Irwin, 1999; Peterson et al., 2017). The WSG spread prolifically, are unpalatable and lack nutrition for grazing livestock, which reduces carrying capacity for graziers and outcompetes native plants for resources (Palmer, 2012; Ansong et al., 2015). The WSG are morphologically similar and difficult to distinguish from closely related native Sporobolus species (Hetherington and Irwin, 1999; Biosecurity Queensland, 2018). This complicates management strategies, particularly the targeted application of herbicides. In addition, areas requiring WSG management are often vast and in remote locations. WSG are prime targets for biological control (biocontrol) solutions, either separately or in combination with herbicide application and manual control (Yobo et al., 2009; Lawrie, 2011; Lock, 2018; Sutton, 2019). Of the five WSG, S. natalensis is the primary target for control in Queensland as it impacts agriculture, pasture and biodiversity, from the New South Wales border to the Cape York Peninsula (Business Queensland, 2020).
Inundative biocontrol of WSG requires the formulation of a mycoherbicide from fungal pathogens endemic to Australia, which can be applied at a high dose causing an artificial, localised infection (Templeton and Greaves, 1984; Charudattan, 1988; McRae and Auld, 2000; Morin, 2020). Examples of successful mycoherbicides include Colletotrichum gloeosporioides to control Aeschynomene virginica (Northern joint vetch) (Templeton and Greaves, 1984) and Nalanthamala diospyri (syn. Acremonium diospyri) for Diospyros virginiana (persimmon trees) (Auld, 2000) in the USA. In Australia, DiBak® Parkinsonia is the only registered mycoherbicide that has successfully navigated the framework of the Australian Pesticide and Veterinary Medicine Authority (APVMA). DiBak® Parkinsonia contains three fungi (Lasiodiplodia pseudotheobromae, Macrophomina phaseolina, and Neoscytalidium novaehollandiae) for the biocontrol of the invasive legume Parkinsonia aculeata (Galea, 2021).
Host-range testing, virulence and pathogenicity trials, and safety evaluations are resource intensive but essential for the regulatory approval of biocontrol agents. Several fungi have previously been tested as potential biocontrol agents for WSG, but none have been shown to be suitable, either due to their efficacy as pathogens, or their lack of host specificity. The fungi previously tested include Microdochium dawsoniorum, Neopestalotiopsis nebuloides, and Pestalotiopsis etonensis (Lock, 2018; Kukuntod, 2020; and this study); Nigrospora oryzae (Lawrie, 2011; Fletcher and Leemon, 2015); four Curvularia species including C. ravenelii (Hetherington, 1997; Hetherington and Irwin, 1999); and Ustilago sporoboli-indici (Yobo et al., 2009; Palmer, 2012; Vitelli et al., 2017; Rapley, 2020).
Observations of WSG populations across several years of surveys at multiple locations in Queensland, revealed evidence of S. natalensis dieback or die-off, reduced fecundity of plants, lesions, and other symptoms of fungal disease (Vitelli et al., 2017). As a result of these and other surveys, 79 fungal isolates were collected from symptomatic tissues of S. indicus complex grasses, and transferred onto artificial medium, with the goal of testing them as potential biocontrol agents for the WSG. Many of the isolates in the collection are certainly novel and/or cryptic species, based on based on multi-locus sequence analysis. This is the first step in prioritising these fungi for testing as potential biocontrol agents for WSG.
2 Methods
2.1 Sample collection and fungal isolation
Between early 2017 and mid-2021, plant tissue samples were collected from areas with infestations of WSG in Queensland (Table S1). Specifically, S. indicus complex grasses with disease symptoms (leaf chlorosis, leaf and stem lesions, and root death) were collected (Figure 1). Additional samples were collected from Sporobolus spp. cultivated in glasshouses at the Ecosciences Precinct, Dutton Park, QLD, Australia. A 5 cm sample of symptomatic plant material was surface sterilised by submersion in a solution of 70% v/v ethanol and 1% v/v sodium hypochlorite for 30 s, followed by 70% v/v ethanol, then rinsed twice in sterile distilled water, and dried in a laminar flow cabinet on sterile filter paper (as per Bills, 1996). Three to six leaf segments (~2 mm2) from each sample were placed on potato dextrose agar (PDA) amended with either streptomycin (sPDA; 50 mg L−1) or chloramphenicol (cPDA; 200 mg L-1) and incubated in the dark at room temperature (23−25 °C). As mycelia developed, isolates were sub-cultured onto fresh cPDA, followed by PDA plates for growth of pure cultures. Reference isolates generated in this study were deposited in the culture collection at the Queensland Plant Pathology Herbarium (BRIP), Dutton Park, QLD, Australia.
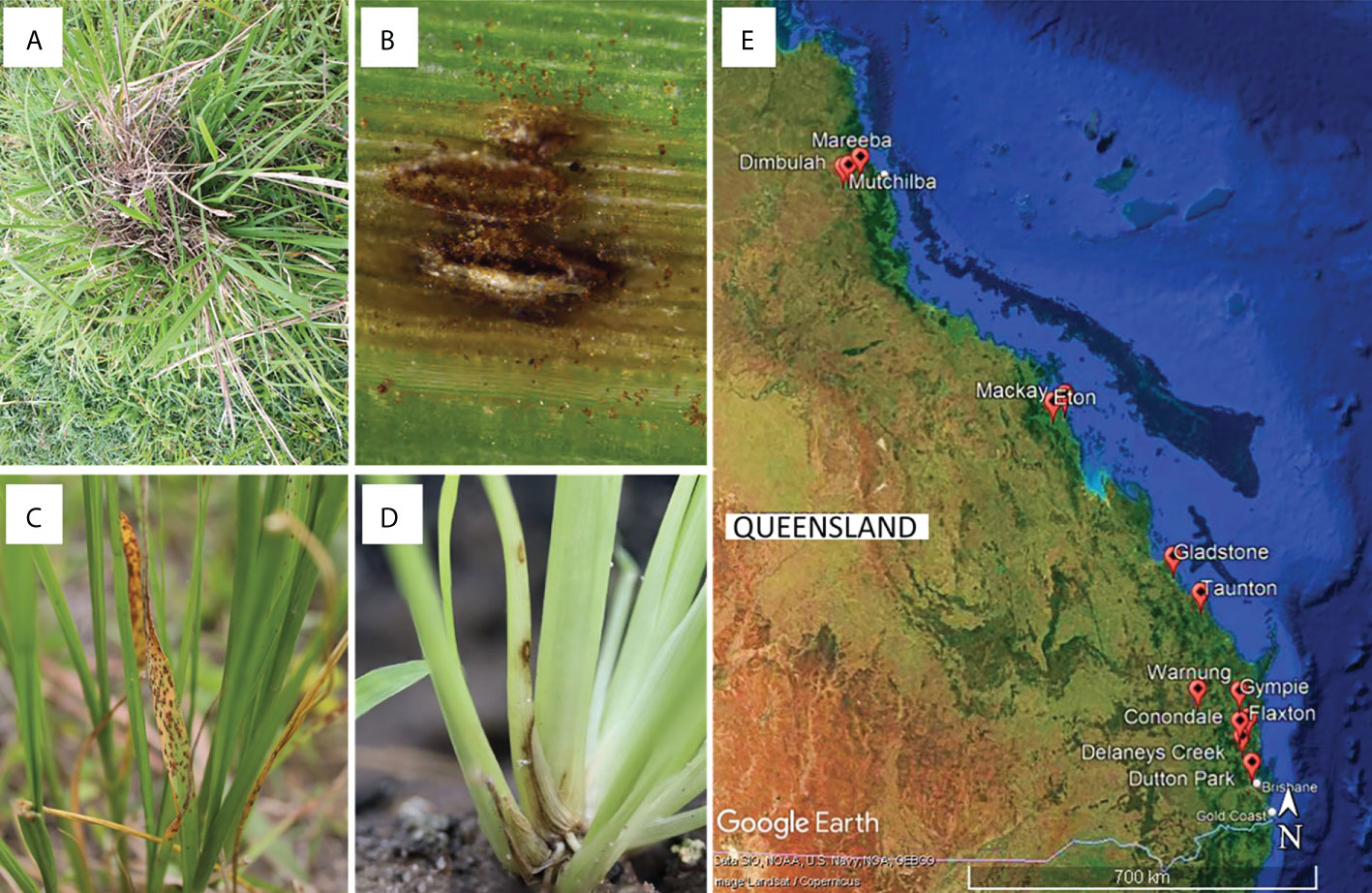
Figure 1 (A–D) Examples of disease symptoms on Sporobolus spp. Plants targeted for sampling, and (E) sampling sites for this study in Queensland, Australia generated using Google Earth Pro©.
2.2 DNA extraction, amplification, and sequencing
Genomic DNA was extracted with either the Isolate II Plant DNA Kit (Bioline) or the ZymoBIOMICS DNA Miniprep Kit (Zymo Research) as per the manufacturers’ instructions, from ~100 mg of mycelium scraped from agar plates. Oligonucleotide primers and Polymerase Chain Reaction (PCR) conditions used to amplify and sequence the targeted loci are listed in the Supplementary Material Table S2. PCRs were performed either with the MyTaq DNA Polymerase (Bioline) or with Phusion HF Master Mix (New England Biolab) according to manufacturer’s instructions, using 10 μmol of each primer and 2−3 μL neat DNA extract, to a total reaction volume of 25 μL per reaction. PCR products were purified with the ISOLATE II PCR and Gel Kit (Bioline) according to manufacturer’s instructions, eluted with sterile distilled H2O, and submitted to Macrogen Inc. (Seoul, South Korea) for bidirectional sanger sequencing.
2.3 Phylogenetic analysis
DNA sequence chromatograms of the sequenced loci were viewed, edited and assembled in Geneious Prime v. 11.1.2 (Biomatters Ltd., Auckland, New Zealand) and deposited in GenBank (Supplementary Material Table S1). DNA sequences were aligned with selected reference sequences downloaded from NCBI (Supplementary Material S3) using the MAFFT algorithm (Katoh et al., 2002) as implemented in Geneious. An initial two-loci phylogeny was constructed with a combined alignment of the internal transcribed spacer (ITS) region and 28S large subunit ribosomal RNA (LSU) sequences with Ustilago abaconensis ex-type CBS 8380 as the outgroup. Based on this initial identification, a more in-depth phylogenetic analysis was undertaken for each family based on DNA sequences from additional nuclear loci including the 18S small subunit ribosomal RNA (SSU), partial region of the glyceraldehyde-3-phosphate dehydrogenase (gapdh), RNA-directed polymerase II subunit 2 (rpb2), beta-tubulin (tub2), and translation elongation factor 1-α (tef1-α). All phylogenies were constructed using maximum likelihood (ML) with the RAxML v. 7.2.8 (Stamatakis, 2014) plug-in in Geneious starting from a random tree topology. The nucleotide substitution model used was General Time-Reversible (GTR) with a gamma-distributed rate variation. In addition, the Bayesian analysis was performed using the MrBayes v.3.2.1 (Huelsenbeck and Ronquist, 2001) plug-in in Geneious. To remove the need for a priori model testing, the Markov chain Monte Carlo (MCMC) analysis was set to sample across the entire GTR model space with a gamma-distributed rate variation across the nucleotide sites. Ten million random trees were generated using the MCMC procedure with four chains. The sample frequency was set at 2000 and the temperature of the heated chain was 0.1. “Burn-in” was set at 25%, after which the log-likelihood values were stationary.
3 Results
3.1 Phylogenetic analysis
In total, 79 fungal isolates were analysed in this study (Supplementary Material Table S1). Sequences from ITS and LSU resolved these isolates into 22 families representing 12 orders, all within the Ascomycota (Figure 2). Subsequent phylogenies used loci that were informative for each family.
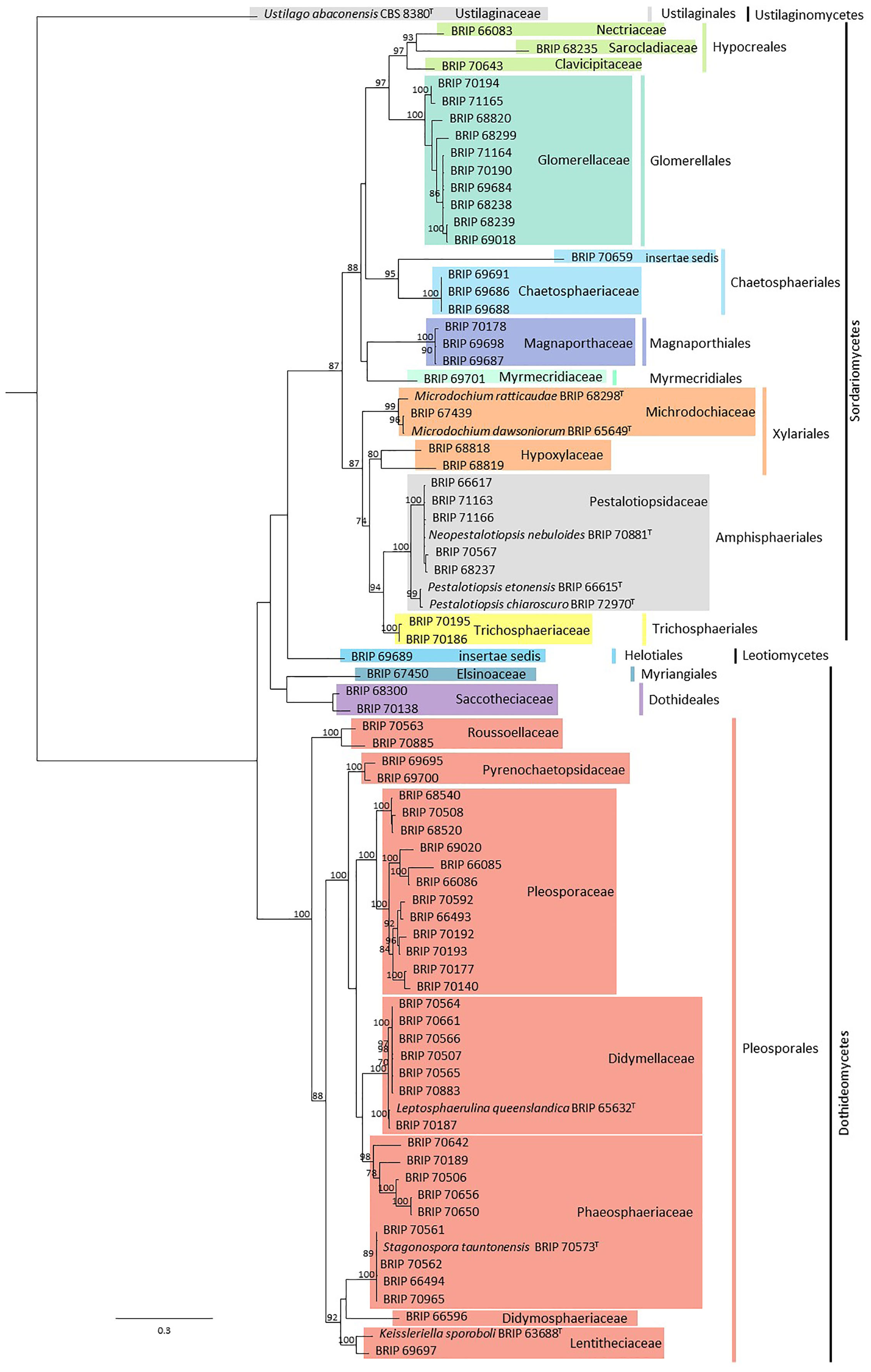
Figure 2 Phylogenetic tree inferred from a RAxML analysis based on a concatenated alignment of ITS and LSU sequences of the isolates in this study. RAxML bootstrap (bs) values greater than 70% are given at the nodes. In bold font are the isolates from this study and ex-type strains are indicated with T.
3.1.1 Amphisphaeriales
The phylogenetic analysis of four gene regions (ITS, LSU, tef1-α, and tub2) resolved seven isolates into Neopestalotiopsis and Pestalotiopsis (Pestalotiopsidaceae; Figure 3). Three isolates (BRIP 70567, BRIP 70881, and BRIP 71166) were identified as N. nebuloides, a species described from S. natalensis (Crous et al., 2020), and three isolates (BRIP 68236, BRIP 68237, and BRIP 71163) are likely a novel Neopestalotiopsis species. One isolate (BRIP 72970) represented the holotype of P. chiaroscuro (Crous et al., 2022).
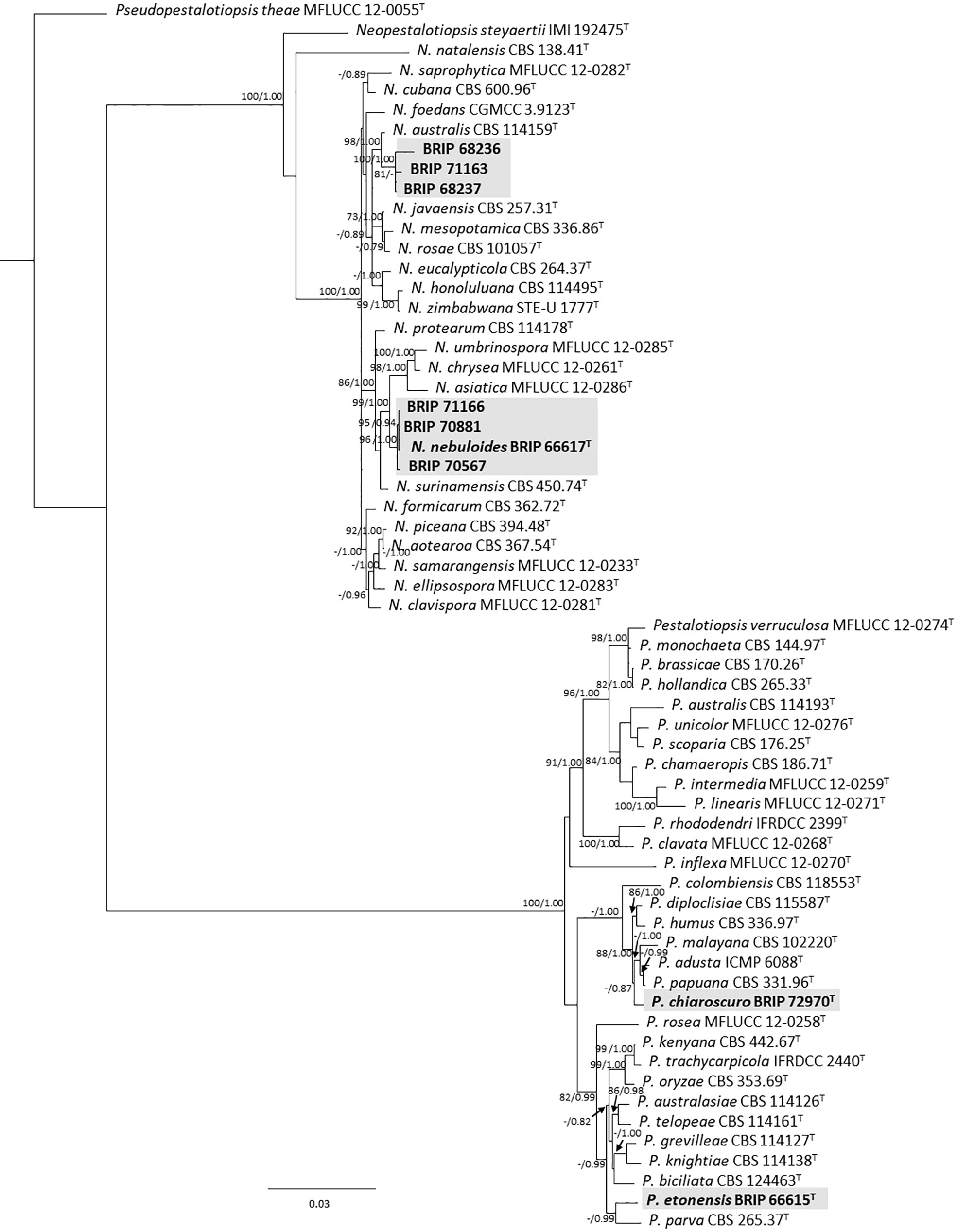
Figure 3 Phylogenetic tree inferred from a RAxML analysis based on a concatenated alignment of ITS, LSU, tef1-α and tub2 sequences of related genera from Pestalotopsidaceae (Amphisphaeriales). RAxML bootstrap (bs) values greater than 70% and Bayesian posterior probabilities (pp) greater than 0.8 are given at the nodes (bs/pp). In bold font are the isolates from this study, and ex-type strains are indicated with T.
3.1.2 Chaetosphaeriales
The phylogenetic analysis of three gene regions (ITS, LSU, and tef1-α) resolved four isolates into Dictyochaeta and Neoleptosporella (Figure 4). Three isolates isolated from S. natalensis roots were identified as phylogenetically close to Dictyochaeta assimica (Chaeotosphaeriaceae) (Figure 4A). Another isolate (BRIP 70659) is an undescribed species of Neoleptosporella (Chaetosphaeriales; Figure 4B). Neoleptosporella currently consists of three species associated with Clematis (Ranunculaceae; Phukhamsakda et al., 2020).
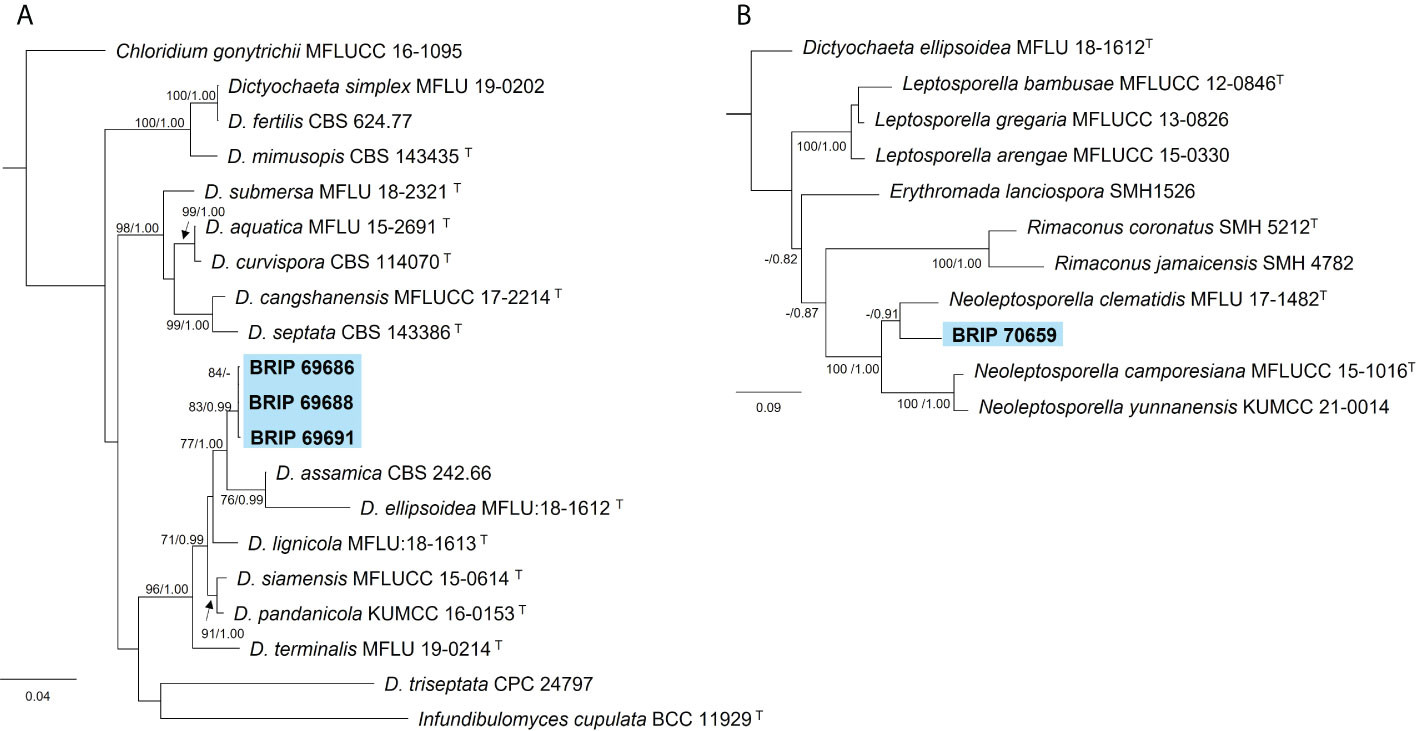
Figure 4 Phylogenetic trees inferred from RAxML analyses of strains in Chaetosphaeriales based on concatenated alignments of (A) ITS, LSU and tef1-α sequences of related species in Chaetosphaeriaceae; and (B) ITS and LSU sequences of related species in Neoleptoporella (incertae sedis). RAxML bootstrap (bs) values greater than 70% and Bayesian posterior probabilities (pp) greater than 0.8 are given at the nodes (bs/pp). In bold font are the isolates from this study, and ex-type strains are indicated with T.
3.1.3 Dothideales
The phylogenetic analysis of four gene regions (ITS, LSU, rpb2, and tub2) identified two isolates as Aureobasidium (Saccotheciaceae; Figure 5). One isolate (BRIP 70138) was isolated from the seed of S. natalensis and is closely related to the ubiquitous A. melanogeum. The other isolate (BRIP 68300) was isolated from leaf tissue of S. natalensis, and is a novel species sister to A. mangrovei, a species described from plant debris in a freshwater habitat in Oman (Alaraimi et al., 2019).
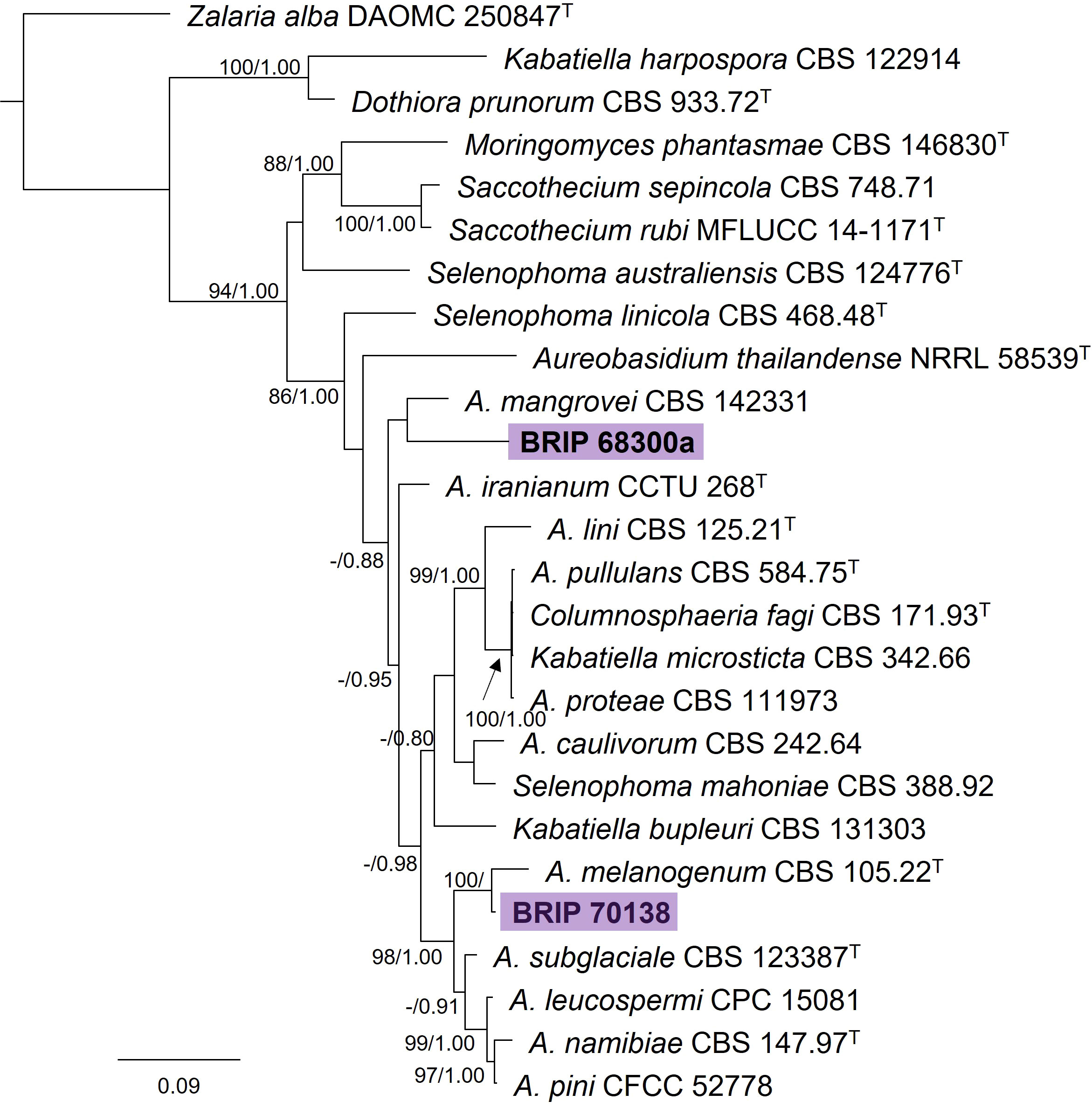
Figure 5 Phylogenetic tree inferred from a RAxML analysis based on a concatenated alignment of ITS, LSU, rpb2 and tub2 sequences of related genera in Saccotheciaceae (Dothideales). RAxML bootstrap (bs) values greater than 70% and Bayesian posterior probabilities (pp) greater than 0.8 are given at the nodes (bs/pp). In bold font are the isolates from this study, and ex-type strains are indicated by T.
3.1.4 Glomerellales
The phylogenetic analysis of three gene regions (ITS, gapdh, and tub2) identified ten isolates as Colletotrichum (Glomerellaceae; Figure 6). Six of these isolates (BRIP 68238, BRIP 68239, BRIP 69018, BRIP 69684, and BRIP 70190) clustered in the same clade as the ex-type strain of Co. karsti, whilst two isolates (BRIP 70194, and BRIP 71165) clustered in the same clade as the ex-type strain of Co. gigasporum. Two isolates are likely to represent taxonomic novelties, one (BRIP 68820) in the Co. gloeosporioides species complex, and the other (BRIP 68299) in the Co. graminicola species complex.
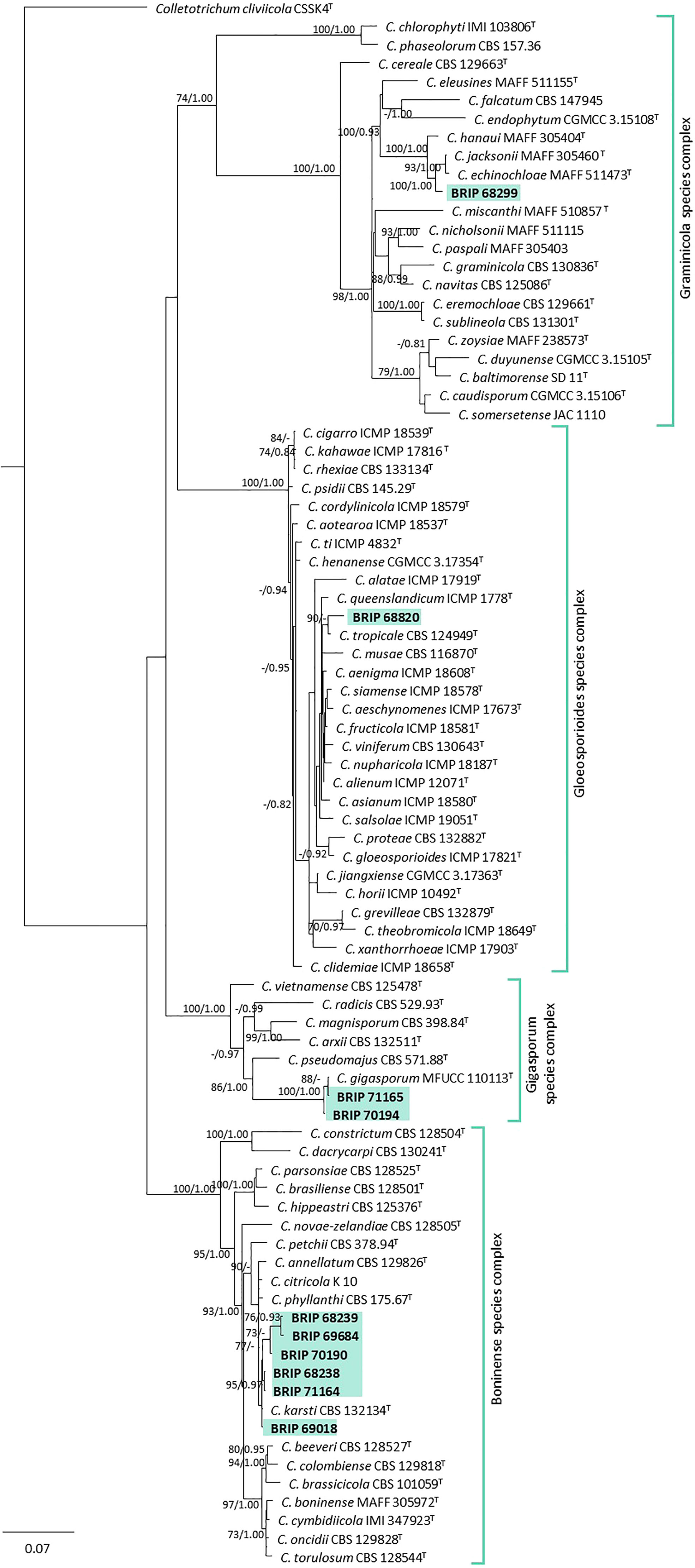
Figure 6 Phylogenetic tree inferred from a RAxML analysis based on a concatenated alignment of ITS, tub2 and gapdh sequences of related genera from the Colletotrichumgleosporioides, Co. graminicola, and Co. boninense species complexes in Glomerellaceae (Glomerellales). RAxML bootstrap (bs) values greater than 70% and Bayesian posterior probabilities (pp) greater than 0.8 are given at the nodes (bs/pp). In bold font are the isolates from this study, and ex-type strains are indicated by T.
3.1.5 Helotiales
The phylogenetic analysis of four gene regions (ITS, LSU, SSU, and rpb2) identified one isolate (BRIP 69689) isolated from the roots of S. natalensis as sister to the yellow rot fungus Scytalidium sphaerosporum (Helotiales; Figure 7).
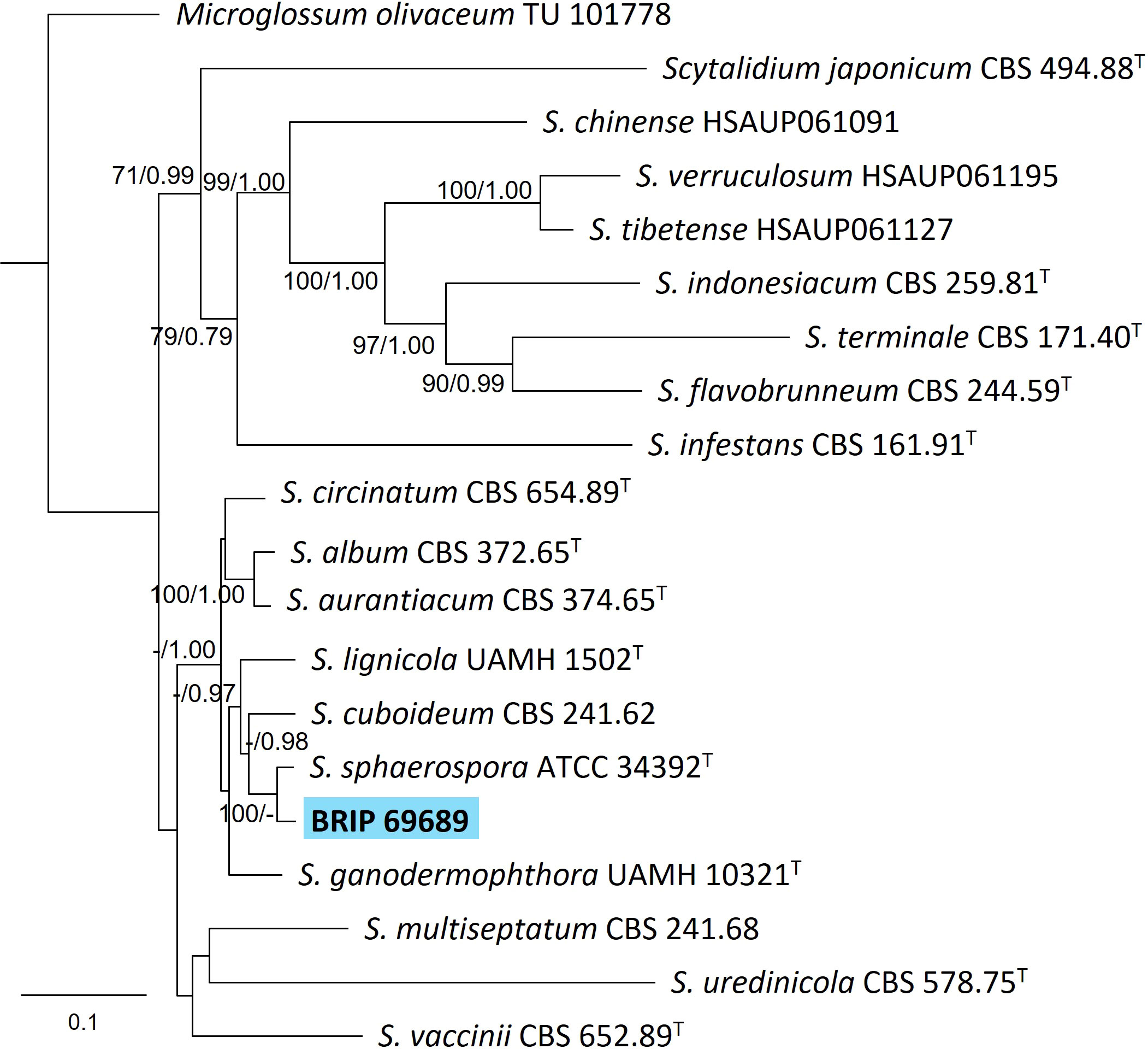
Figure 7 Phylogenetic tree inferred from a RAxML analysis based on a concatenated alignment of ITS, LSU, rpb2 and SSU sequences of related genera from Scytalidium (Helotiales). RAxML bootstrap (bs) values greater than 70% and Bayesian posterior probabilities (pp) greater than 0.8 are given at the nodes (bs/pp). In bold font is the isolate from this study, and ex-type strains are indicated with T.
3.1.6 Hypocreales
Three isolates isolated from the leaves of S. natalensis were identified to belong to three families within Hypocreales. The phylogenetic analysis of three gene regions (LSU, rpb2, and tef1-α) identified one isolate (BRIP 70643) as a novel genus within Clavicipitaceae (Figure 8A). The phylogenetic analysis of four genes regions (ITS, LSU, rpb2, and tef1-α) identified one isolate (BRIP 66083) in the same clade as the ex-type strain of Fusarium proliferatum (Nectriaceae; Figure 8B). The phylogenetic analysis of three gene regions (ITS, LSU, and tub2) identified isolate BRIP 68235 as a novel species in Parasarcocladium (Sarocladiaceae; Figure 8C).
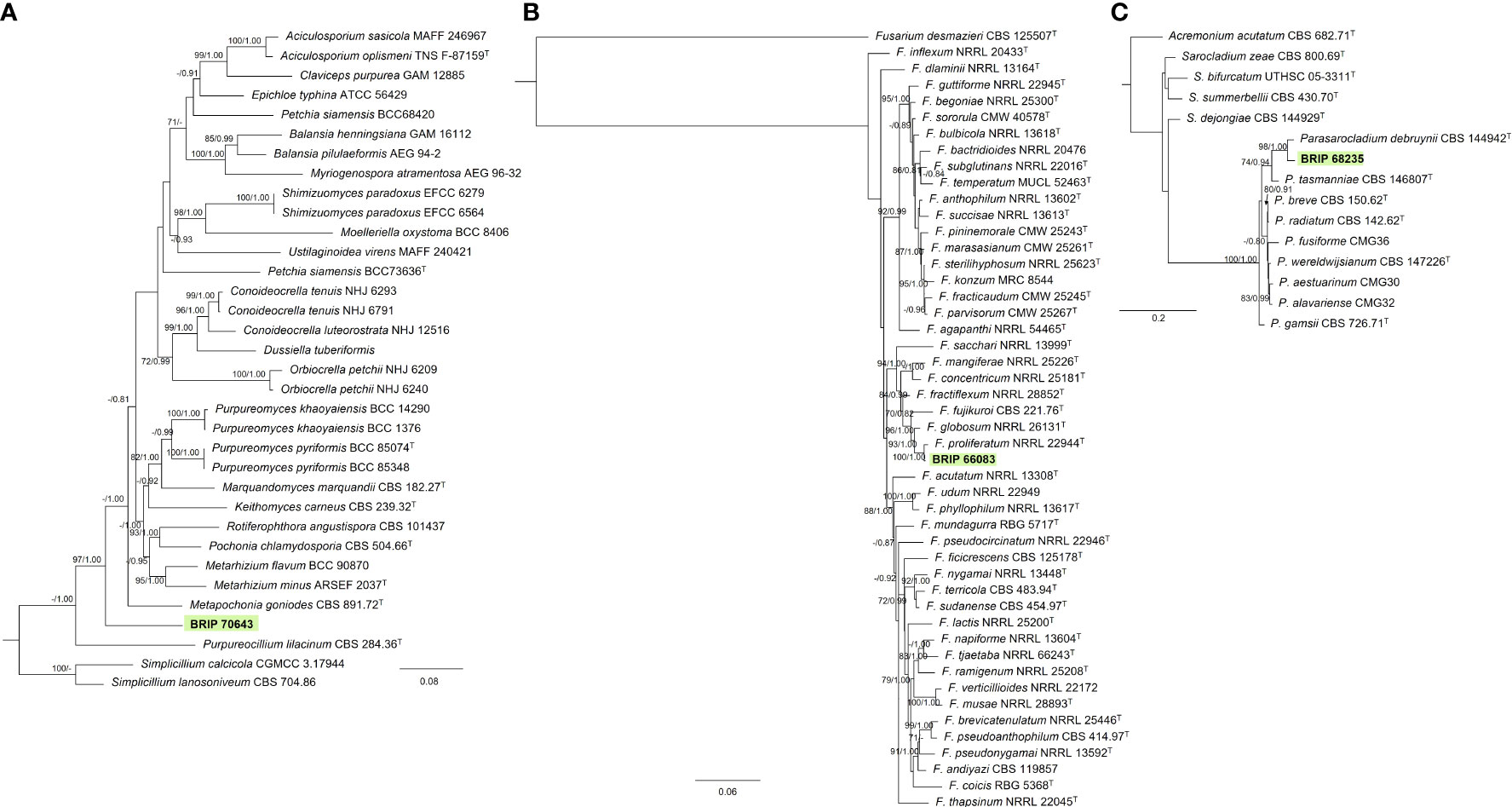
Figure 8 Phylogenetic trees inferred from RAxML analyses of strains in Hypocreales based on concatenated alignments of (A) rpb2, tef1-α and LSU sequences of related genera from Clavicipitaceae; (B) ITS, LSU, rpb2, tef1-α and tub2 sequences of related species from the Fusarium fujikuroi species complex (Nectriaceae); and (C) ITS, LSU, tub2 sequences of related genera from Sarocladiaceae (Hypocreales). RAxML bootstrap (bs) values greater than 70% and Bayesian posterior probabilities (pp) greater than 0.8 are given at the nodes (bs/pp). In bold font is the isolate from this study, and ex-type strains are indicated with T.
3.1.7 Myriangiales
The phylogenetic analysis of four genes regions (ITS, LSU, rpb2, and tef1-α) identified one isolate (BRIP 67450) as a novel species in Elsinoë (Elsinoaceae; Figure 9).
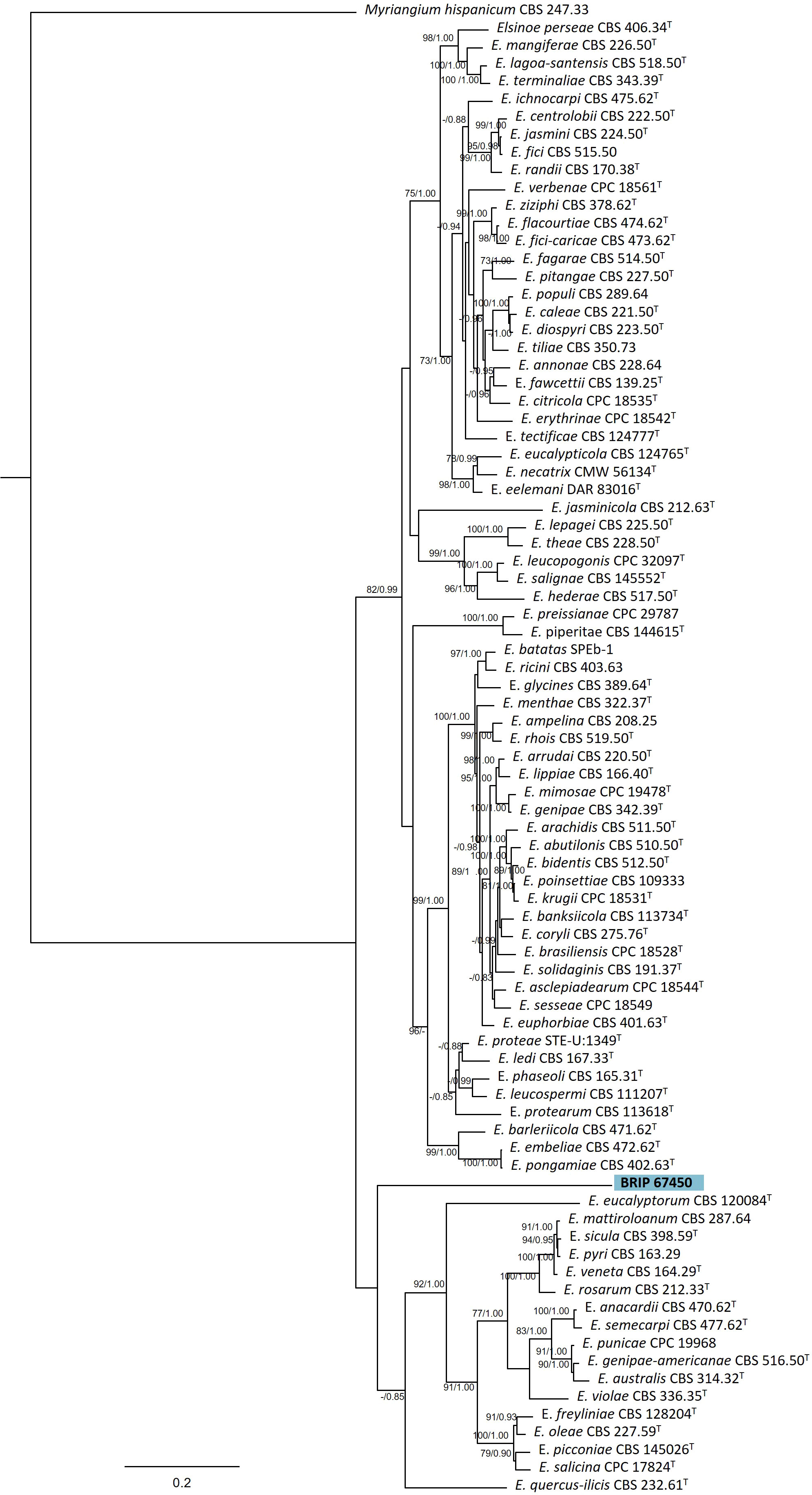
Figure 9 Phylogenetic tree inferred from a RAxML analysis based on a concatenated alignment of ITS, LSU, rpb2 and tef1-α sequences of related genera from Elsinoaceae (Myriangiales). RAxML bootstrap (bs) values greater than 70% and Bayesian posterior probabilities (pp) greater than 0.8 are given at the nodes (bs/pp). In bold font is the isolate from this study, and ex-type strains are indicated with T.
3.1.8 Pleosporales
Most of the isolates examined in this study belonged to six families within Pleosporales. The phylogenetic analysis of two gene regions (ITS and rpb2) identified three isolates to belong to Didymellaceae (Figure 10A). One species (BRIP 70507, BRIP 70661, BRIP 70883, and BRIPs 70564-70566) is a novel Epicoccum, isolated from stem, roots and leaves of S. elongatus and S. natalensis. This species was identified as closely related to E. multiceps. One isolate (BRIP 70187) was phylogenetically close to Leptospherulina argentinensis and L. gaeumannii. Another isolate (BRIP 65632) was identified as L. queenslandica (Crous et al., 2022).
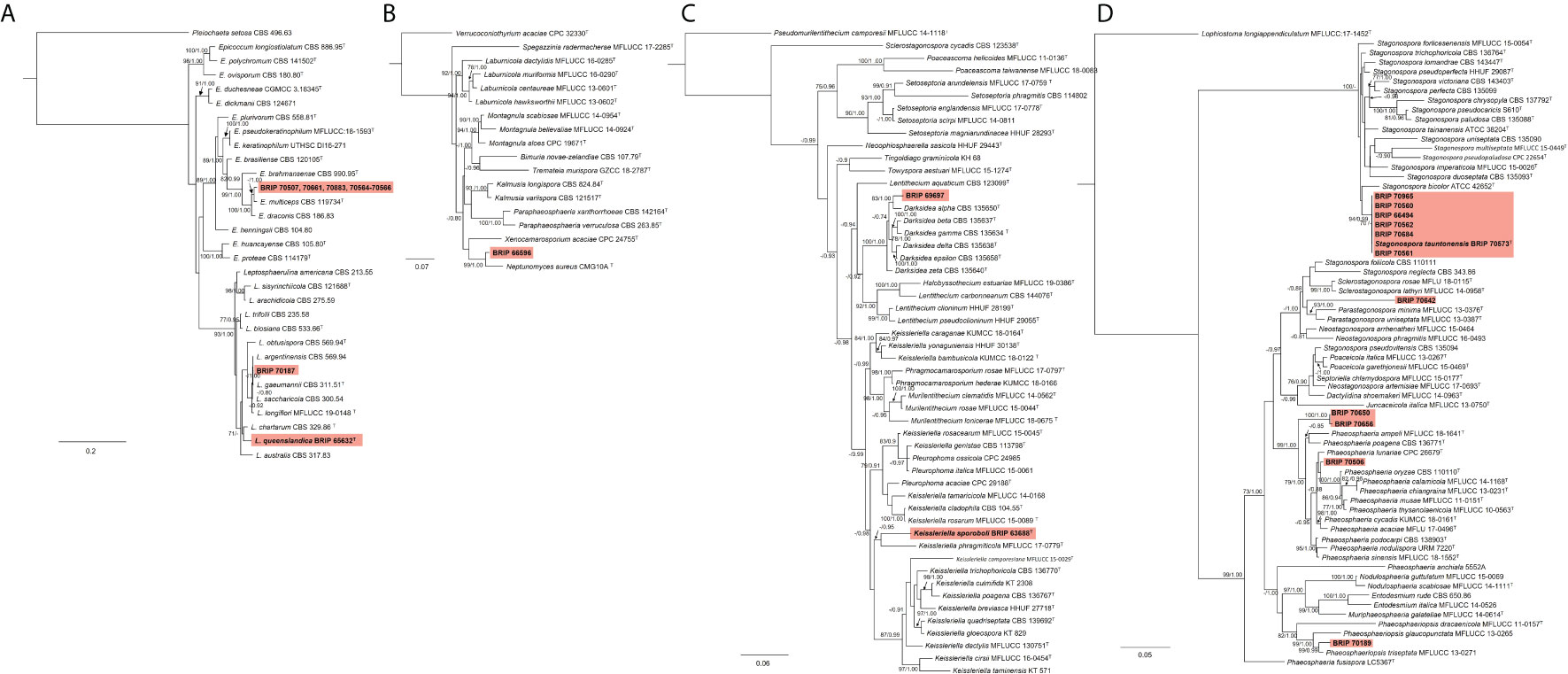
Figure 10 Phylogenetic trees inferred from RAxML analyses of strains in Pleosporales based on concatenated alignments of (A) ITS and rpb2 sequences of related genera from Didymellaceae; (B) ITS, LSU, SSU and tub2 sequences of related genera from Didymosphaeriaceae; (C) ITS, LSU and tef1-α sequences of related genera from Lentitheciaceae; and (D) ITS, LSU, SSU and tef1-α sequences of related genera from Phaeosphaeriaceae. RAxML bootstrap (bs) values greater than 70% and Bayesian posterior probabilities (pp) greater than 0.8 are given at the nodes (bs/pp). In bold font are the isolates from this study, and ex-type strains are indicated with T.
The phylogenetic analysis of four gene regions (ITS, LSU, SSU, and tub2) identified one isolate (BRIP 66596) as a novel species in Neptunomyces (Didymosphaeriaceae; Figure 10B).
Two isolates to belonged to Lentitheciaceae based on three gene regions (ITS, LSU, and tef1-α; Figure 10C). One isolate (BRIP 63688) was identified as Keissleriella sporoboli (Crous et al., 2022), and another isolate (BRIP 69697) represents a novel Darksidea.
The phylogenetic analysis of four gene regions (ITS, LSU, SSU, tef1-a) resolved 11 isolates into five species within Phaeosphaeriaceae (Figure 10D). Three isolates (BRIP 70506, BRIP 70650, and BRIP 70656) represent two novel species in Phaeosphaeria. One isolate (BRIP 70642) is a novel species in Parastagonospora, and another isolate (BRIP 70189) is a novel species in Phaeosphaeriopsis. Interestingly, during this study, 30 isolates representing Stagonospora tauntonensis (Crous et al., 2022) were isolated from multiple Sporobolus spp. collected across the state of Queensland. Six of these isolates were included in the phylogeny.
The phylogenetic analysis of the rDNA (ITS and LSU) found 12 isolates belonged to Pleosporaceae (Figure 11A). Three isolates (BRIP 68520, BRIP 68540, and BRIP 70508) clustered within Alternaria sect. alternata. Additional phylogenetic analysis based on three gene regions (gapdh, rpb2, and tef1-α) resolved the other nine Pleosporeaceae isolates into three genera, Bipolaris, Curvularia, and Exserohilum (Figure 11B). Two isolates (BRIP 70140 and BRIP 70177) represent a novel Exserohilum species. One isolate (BRIP 69020) is phylogenetically closely related to Cu. lunata, and two isolates (BRIP 66085 and BRIP 66086) are phylogenetically close to Cu. ravenelii. Curvularia ravenelii had been previously shown to infect S. indicus (Luttrell, 1976). It was assessed, but ultimately rejected, as a biocontrol agent against WSG in Australia (Hetherington and Irwin, 1999).
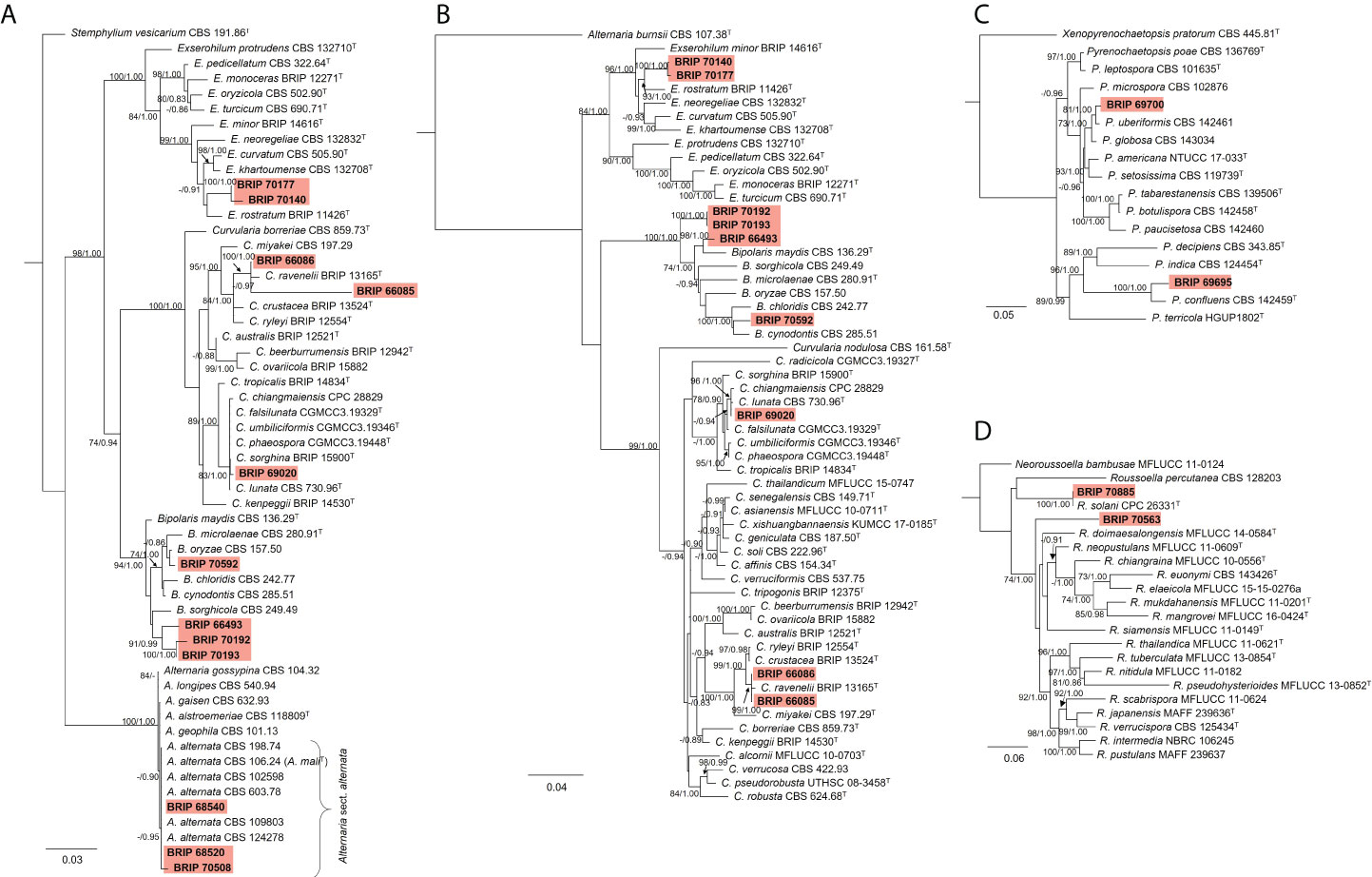
Figure 11 Phylogenetic trees inferred from RAxML analyses of strains in Pleosporales based on concatenated alignments of (A) ITS and LSU sequences of related species in Alternaria, Bipolaris, Curvularia, and Exserohilum (Pleosporaceae); (B) gapdh, rpb2 and tef1-α sequences of related species in the Bipolaris, Curvularia, and Exserohilum (Pleosporaceae); (C) LSU, ITS, rpb2 and tub2 sequences of related species in Pyrenochaetopsidaceae; and (D) ITS, LSU, rpb2 and tef1-α sequences of related species from Roussoellaceae. RAxML bootstrap (bs) values greater than 70% and Bayesian posterior probabilities (pp) greater than 0.8 are given at the nodes (bs/pp). In bold font are the isolates from this study, and ex-type strains are indicated with T.
The phylogenetic analysis of four gene regions (LSU, ITS, rpb2, and tub2) identified two isolates (BRIP 69695 and BRIP 69700) as a novel species in Pyrenochaetopsis (Pyrenochaetopsidaceae; Figure 11C). The phylogenetic analysis of four gene regions (LSU, ITS, rpb2, and tef1-α) found two isolates belong to Roussoella (Roussoellaceae; Figure 11D). One isolate (BRIP 70885) was identified as R. solani, while the other isolate (BRIP 70563) is a novel species.
3.1.9 Magnaporthales
The phylogenetic analysis of four gene regions (ITS, LSU, SSU, and tef1-α) identified three isolates (BRIP 69687, BRIP 69698, and BRIP 70178) as Magnaporthiopsis meyeri-festucae (Figure 12). Magnaporthiopsis meyeri-festucae was associated with summer patch-like disease symptoms in Festuca spp. (Poaceae) in the USA (Luo et al., 2017). This study represents a new host record for M. meyeri-festucae, and the first report in Australia (Wong et al., 2022).
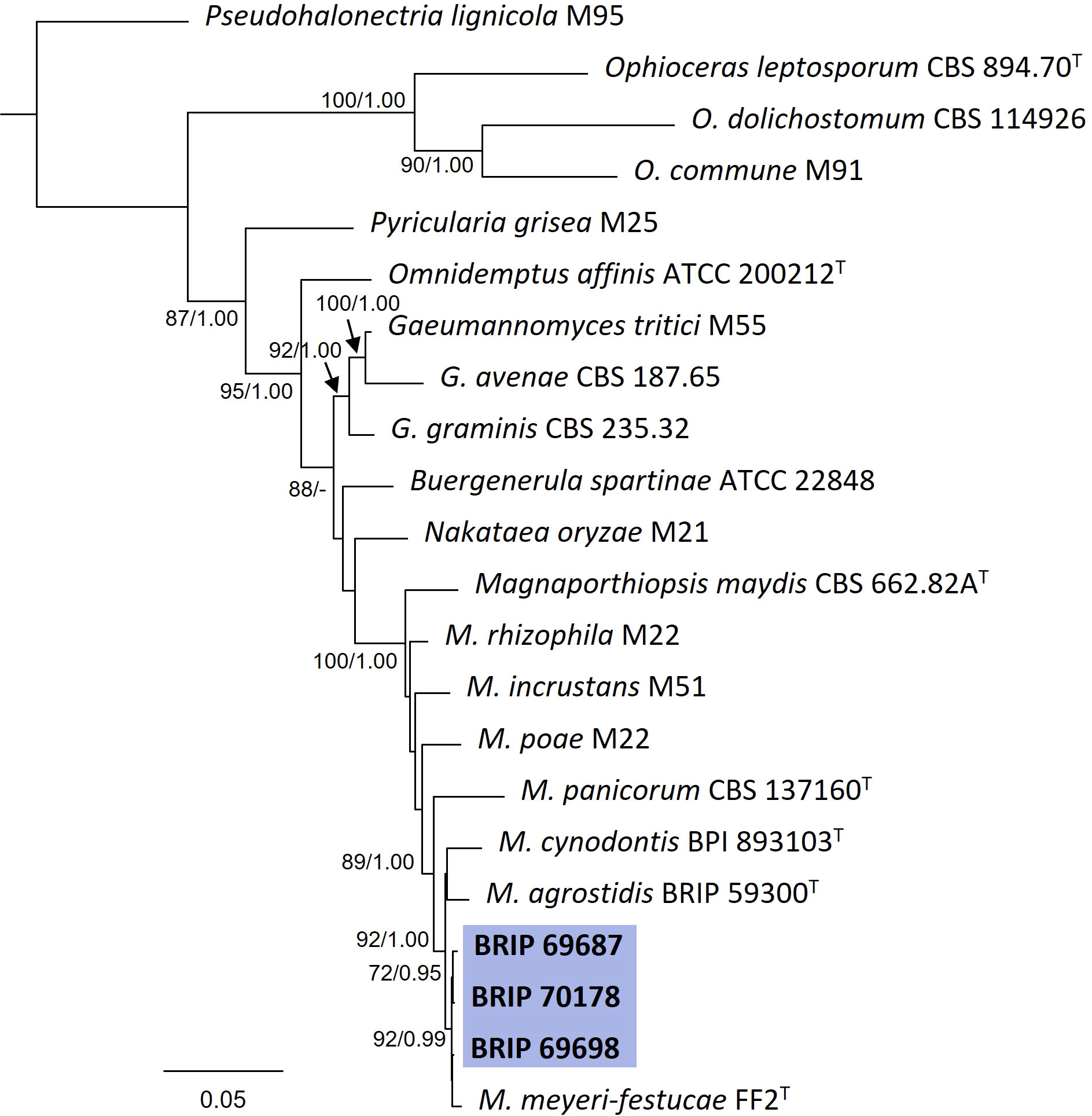
Figure 12 Phylogenetic tree inferred from a RAxML analysis based on a concatenated alignment of ITS, LSU, SSU and tef1-α sequences of related genera from Magnaporthaceae (Magnaporthiales). RAxML bootstrap (bs) values greater than 70% and Bayesian posterior probabilities (pp) greater than 0.8 are given at the nodes (bs/pp). In bold font are the isolates from this study, and ex-type strains are indicated with T.
3.1.10 Myrmecridiales
The phylogenetic analysis of four gene regions (ITS, LSU, SSU, and rpb2) identified one isolate (BRIP 69701) as a novel species of Myrmecridium (Myrmecridiaceae; Figure 13).
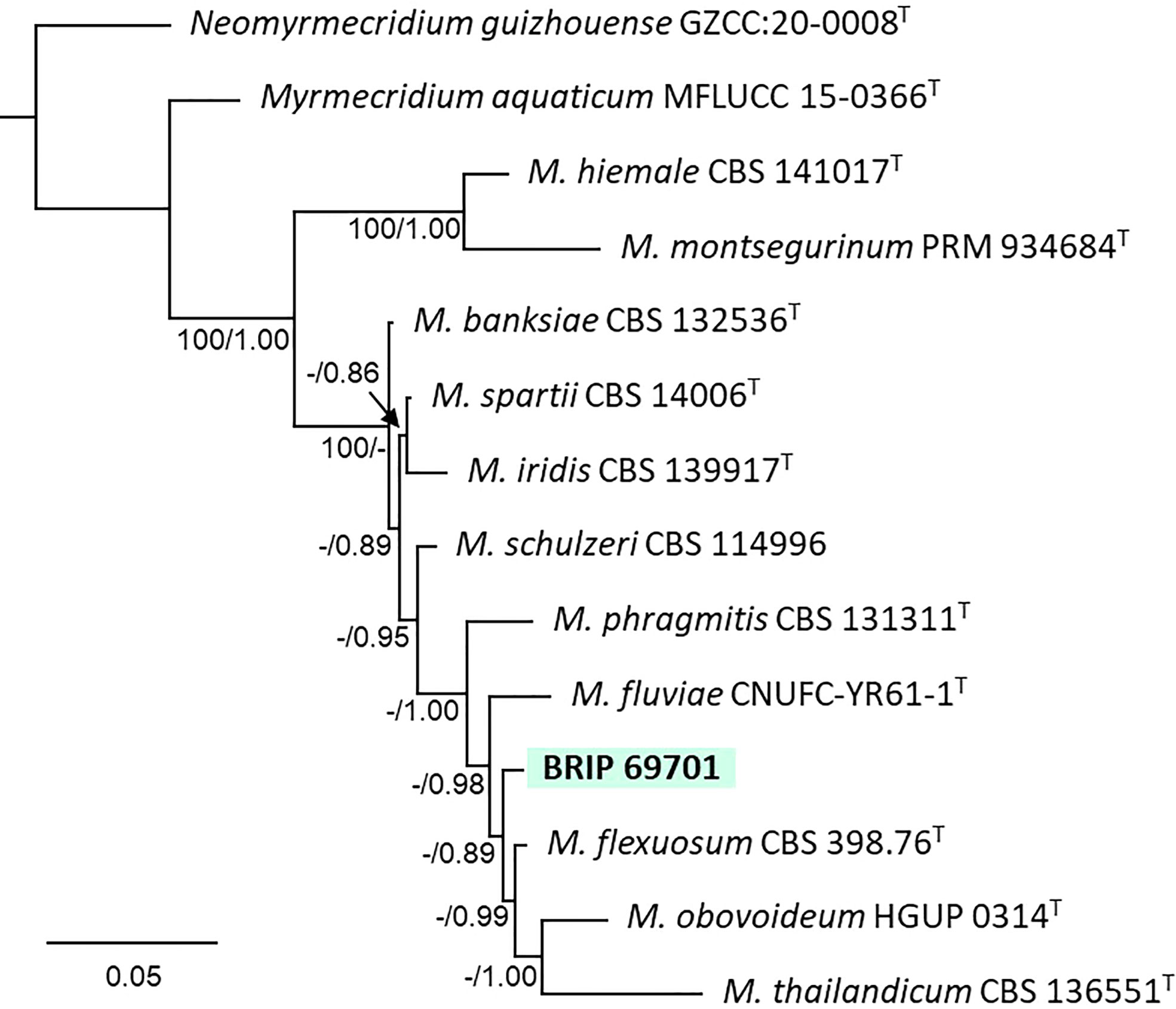
Figure 13 Phylogenetic tree inferred from a RAxML analysis based on a concatenated alignment of ITS, LSU, SSU and rpb2 sequences of related species in Myrmecridiaceae (Myrmecridiales). RAxML bootstrap (bs) values greater than 70% and Bayesian posterior probabilities (pp) greater than 0.8 are given at the nodes (bs/pp). In bold font are the isolates from this study, and ex-type strains are indicated with T.
3.1.11 Trichosphaeriales
Two Trichosphaeriaceae isolates (BRIP 70186 and BRIP 70195) were identified using sequences from three gene regions (ITS, tef1-α and tub2; Figure 14) as the generalist plant pathogen Nigrospora sphaerica (Trichosphaeriaceae) (Wang et al., 2017; Hao et al., 2020).
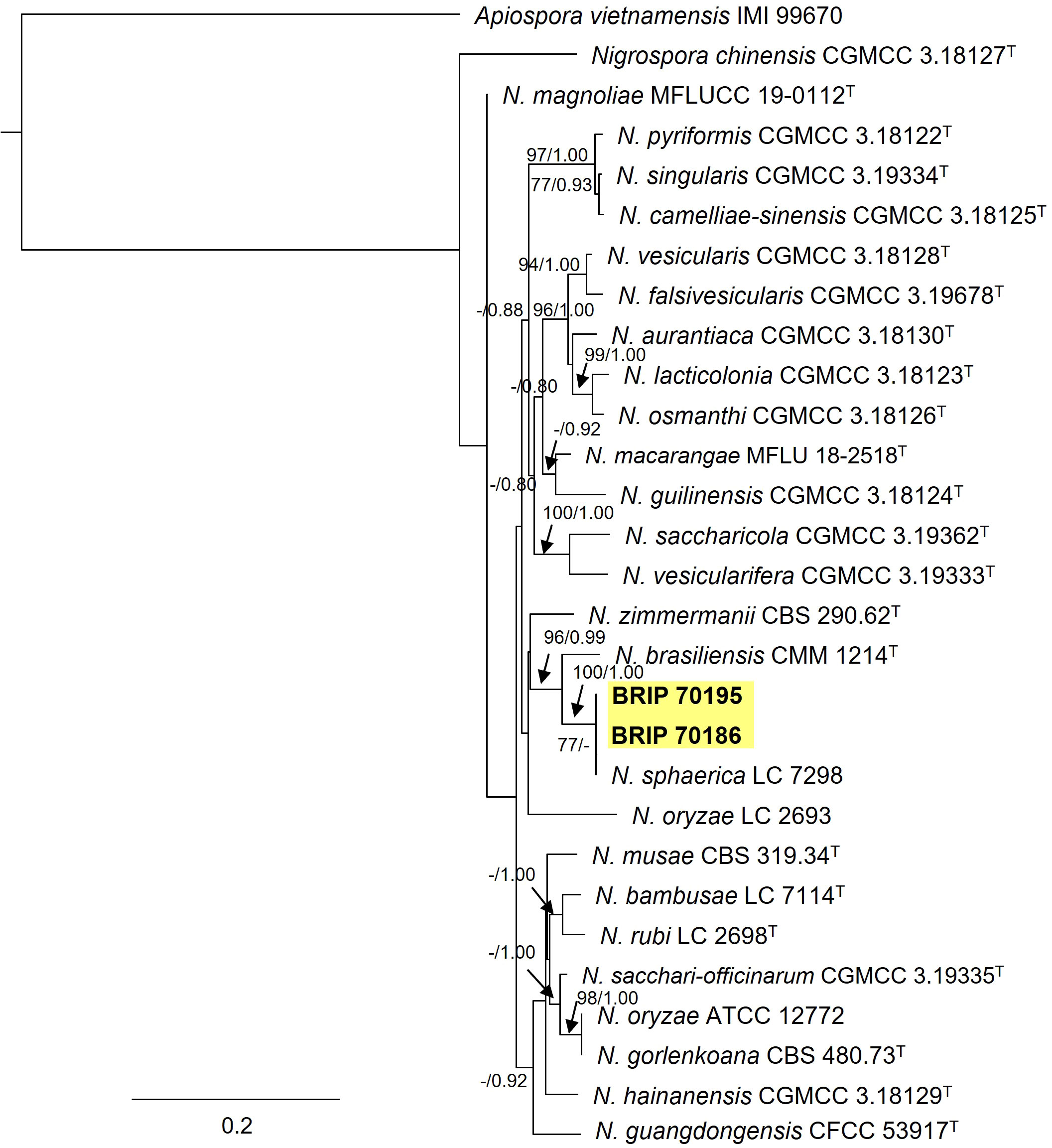
Figure 14 Phylogenetic tree inferred from a RAxML analysis based on a concatenated alignment of ITS, LSU and tef1-α sequences of related species in Trichosphaeriaceae (Trichosphaeriales). RAxML bootstrap (bs) values greater than 70% and Bayesian posterior probabilities (pp) greater than 0.8 are given at the nodes (bs/pp). In bold font are the isolates from this study, and ex-type strains are indicated with T.
3.1.12 Xylariales
The phylogenetic analysis of four gene regions (ITS, LSU, rpb2, and tub2) identified two isolates (BRIP 68818 and BRIP 68819) as two novel species of Hypoxylon (Hypoxylaceae; Figure 15A). The phylogenetic analysis of three gene regions (ITS, LSU, and rpb2; Figure 15B) identified three isolates to belong to Microdochium (Microdochiaceae). One isolate (BRIP 65649) represents the holotype of M. dawsoniorum (Crous et al., 2020), and the other (BRIP 68298) represent the holotype of M. ratticaudae (Crous et al., 2021).
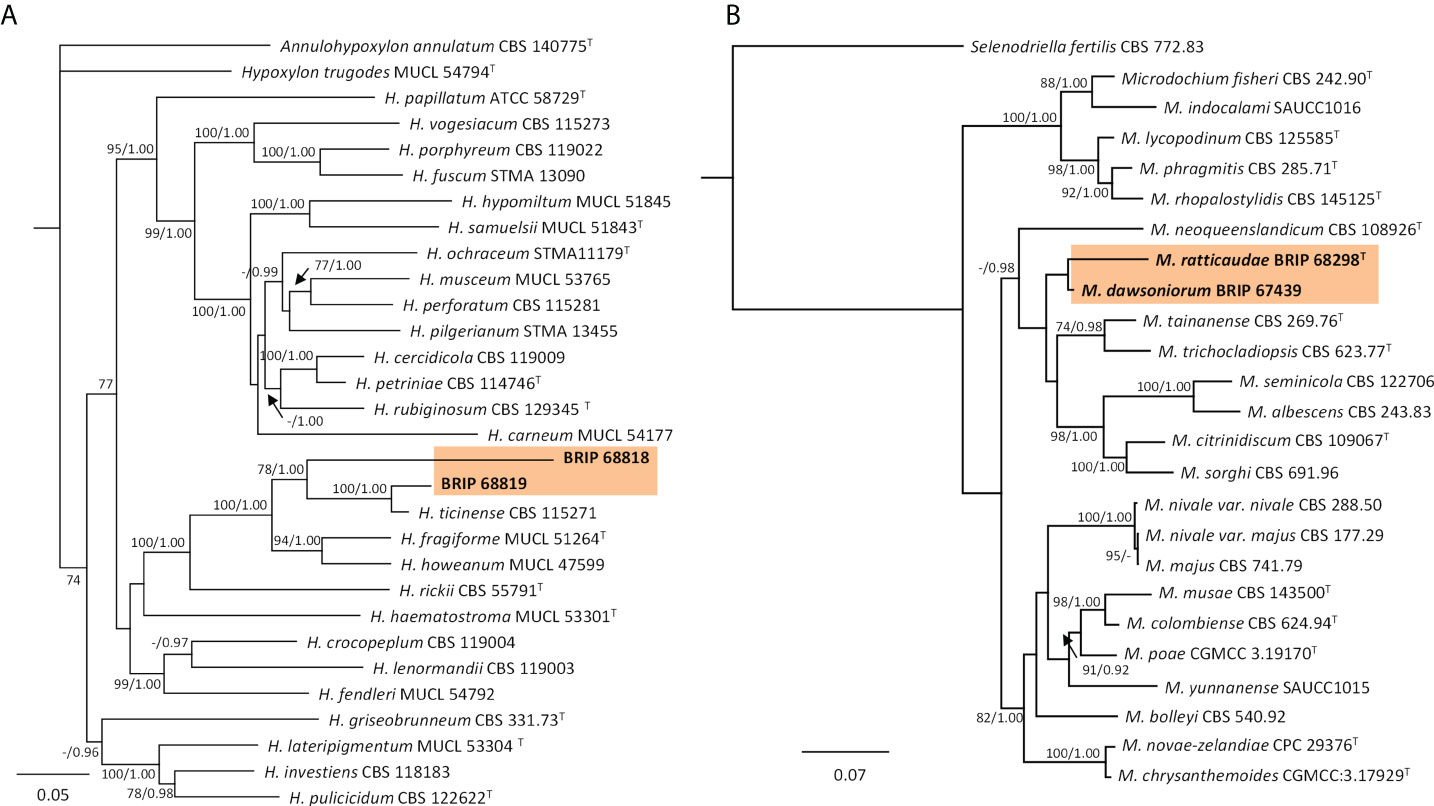
Figure 15 Phylogenetic trees inferred from RAxML analyses of strains in Xylariales based on concatenated alignments of (A) ITS, LSU, rpb2 and tef1-α sequences of related species in Hypoxylaceae; and (B) ITS, LSU and rpb2 sequences of related species in Microdochiaceae. RAxML bootstrap (bs) values greater than 70% and Bayesian posterior probabilities (pp) greater than 0.8 are given at the nodes (bs/pp). In bold font are the isolates from this study, and ex-type strains are indicated with T.
4 Discussion
Grass-associated endophytes have been used for the biocontrol of insects and weeds in various agricultural and environmental systems. Neotyphodium spp. (Clavicipitaceae) has been applied to tall fescue (Festuca arundinacea) and perennial ryegrass (Lolium perenne) for the biocontrol of herbivorous insect pests, e.g., the root aphid Aploneura lentisci and wheat sheath miner Cerodontha australis (Saikkonen et al., 2006; Young et al., 2013). Other entomopathogenic fungi, including Beauveria (Cordycipitaceae; Mascarin and Jaronski, 2016), Parametarhizium (Clavicipitaceae; Gao et al., 2021) and Clonostachys (Bionectriaceae; Vega et al., 2008) have been used successfully to control insect pests. The fungal pathogen, Stagonospora convolvuli, produces metabolites that are toxic to crop pathogens (Boss et al., 2007a) and weeds (Boss et al., 2007b). There is evidence that endophyte-containing pasture grasses are more competitive against invading weeds (Saikkonen et al., 2013). Several fungal pathogens, including Colletotrichum (Glomerellaceae), Alternaria (Pleosporaceae), and Phoma (Didymellaceae), have been tested as bioherbicides for the control of target weeds (Morin, 2020). Currently in Australia, there is only one commercially registered product, Di-Bak® Parkinsonia, which contains three species, Lasiodiplodia pseudotheobromae, Macrophomina phaseolina and Neoscytalidium novaehollandiae in the Botryosphaeriaceae, that is available for the biocontrol of the invasive legume Parkinsonia aculeata (Galea, 2021). As individual plants may host hundreds of fungal species, finding a potential biocontrol agent among them is like searching for a needle in a haystack (Peay et al., 2016; Sutton, 2019).
In this study, we resolved the identities of 79 isolates of microfungi from S. indicus species across the state of Queensland. These microfungi were identified based on multi-locus sequence analysis, which is faster and arguably more accurate than using morphology alone, especially when taxonomic characters are lacking, unavailable or uninformative, and where taxonomic novelty is encountered.
Several fungi, including Microdochium dawsonorium, Pestalotiopsis etonensis, and Neopestalotiopsis nebuloides, have been recently described from Sporobolus spp. in Australia (Crous et al., 2020; Crous et al., 2021; Crous et al., 2022). These three fungal species were shown not to be pathogenic or host-specific against WGS (Lock, 2018; Kukuntod, 2020). Consequently, other isolates of Microdochiaceae and Pestalotiopsidaceae found in this study will likely not be prioritised for pathogenicity and host specificity testing. In contrast, Alternaria, Bipolaris, Colletotrichum, Curvularia, Epicoccum, and Stagonospora isolates identified in this study will be prioritised for pathogenicity studies, as these genera have been identified in biocontrol research or as grass pathogens (Luttrell, 1976; Bowers, 1986; Hetherington and Irwin, 1999; Bewick et al., 2000; Boss et al., 2007b), and are particularly diverse on grasses in Australia (Tan et al., 2016; Hernández-Restrepo et al., 2018; Tan et al., 2018).
Metagenomic approaches reveal a greater diversity of fungal taxa than isolation methods (Peay, 2014; Peršoh, 2015; Dissanayake et al., 2018). In our study, we have captured living fungal isolates from symptomatic tissues of grasses in the S. indicus complex. Many of these fungi are likely endophytes or opportunists. Our approach has identified these 79 living fungal isolates so they can now be prioritised for potential pathogenicity and host-specificity studies on Sporobolus spp. Many of these isolates are fast growing in the laboratory, which is a benefit for both in vitro and in vivo studies, e.g., it allows the mass-production of potential biocontrol agents at low cost thus ensuring the easy and quick production of a mycoherbicide. We acknowledge that our 79 isolates represent a small fraction of the total diversity associated with grasses in the S. indicus complex (Arnold et al., 2007; Higgins et al., 2011; Depetris et al., 2020).
In this study, we have shown that there is a high diversity of potentially pathogenic micro-fungal species associated with grasses in the S. indicus complex in Australia. Our method for finding potential biological control agents was to target infected plant tissue from coexisting and phylogenetically closely related grasses; isolate fungi from diseased leaf samples; and identify the resulting fungal species using multi-locus sequence analysis. This systematic approach may have application in many other agricultural and environmental systems.
Data availability statement
The data presented in the study are deposited in NCBI's GenBank database. The accession numbers can be found in the Supplementary Material.
Author contributions
JV is the project manager. JV and DH visited sites and looked for/collected samples. DH did the isolating, morphological ID, herbarium submission and culture maintenance. TS did the DNA extractions, PCR, Sequencing, data collation, manuscript outline and the phylogenetic analyses. YT provided advice and critique of the phylogenetic analyses, mycology, taxonomy, and manuscript development, as well as mentoring TS throughout. All authors provided feedback and critique of the various iterations of this manuscript.
Funding
This project was supported by AgriFutures Australia (Rural Industries Research and Development Corporation), through funding from the Australian Government Department of Agriculture, Water and the Environment, as part of its Rural Research and Development for Profit program (RnD4Profit-18-04-014; PRJ-012376). Author TS was also supported by funding from the Queensland Government’s Advanced Queensland Women’s Research Assistance Program (WRAP049-2019D1).
Acknowledgments
We acknowledge the Traditional Custodians of the land on which we work and sample in Australia, and their Elders past, present and emerging. Thanks to Drew Rapley and Nontaporn Kukuntod for technical support; and to Gavin Hunter and Roger Shivas for comments which greatly improved this manuscript.
Conflict of interest
The authors declare that the research was conducted in the absence of any commercial or financial relationships that could be construed as a potential conflict of interest.
Publisher’s note
All claims expressed in this article are solely those of the authors and do not necessarily represent those of their affiliated organizations, or those of the publisher, the editors and the reviewers. Any product that may be evaluated in this article, or claim that may be made by its manufacturer, is not guaranteed or endorsed by the publisher.
Supplementary material
The Supplementary Material for this article can be found online at: https://www.frontiersin.org/articles/10.3389/ffunb.2022.956837/full#supplementary-material
References
Alaraimi S., Al-Hatmi A., Elshafie A., Al-Bahry S., Al-Wahaibi Y., Al-Bimani A., et al. (2019). New record of Aureobasidium mangrovei from plant debris in the sultanate of Oman. Czech Mycology 71, 219–229. doi: 10.33585/cmy.71207
Ansong M., Pickering C., Arthur J. M. (2015). Modelling seed retention curves for eight weed species on clothing. Austral Ecol. 40 (7), 765–774. doi: 10.1111/aec.12251
Arnold A. E., Henk D. A., Eells R. L., Lutzoni F., Vilgalys R. (2007). Diversity and phylogenetic affinities of foliar fungal endophytes in loblolly pine inferred by culturing and environmental PCR. Mycologia 99 (2), 185–206. doi: 10.1080/15572536.2007.11832578
Auld B. A. (2000). “Success in biological control of weeds by pathogens, including bioherbicides,” in Biological control: Measures of success. Eds. Gurr G., Wratten S. (Dordrecht: Springer Netherlands), 323–340.
Bewick T., Porter J., Ostrowski R. (2000). “Smolder™: A bioherbicide for suppression of dodder (Cuscuta spp.),” in Proceedings of the Annual Meeting - Southern Weed Science Society.
Bills G. F. (1996). “Isolation and analysis of endophytic fungal communities from woody plants,” in Endophytic fungi in grasses and woody plants: Systematics, ecology, and evolution, eds. Eds. Redlin S. C., Carris L. M. (St. Paul, Minnesota: American Phytopathological Society), 31–65.
Biosecurity Queensland (2018). "Rat's tail grasses" (Brisbane Australia: The State of Queensland, Department of Agriculture and Fisheries).
Boss D., Maurhofer M., Schläpfer E., Défago G. (2007a). Elsinochrome a production by the bindweed biocontrol fungus Stagonospora convolvuli LA39 does not pose a risk to the environment or the consumer of treated crops. FEMS Microbiol. Ecol. 59 (1), 194–205. doi: 10.1111/j.1574-6941.2006.00207.x
Boss D., Schläpfer E., Fuchs J., Défago G., Maurhofer M. (2007b). Improvement and application of the biocontrol fungus Stagonospora convolvuli LA39 formulation for efficient control of Calystegia sepium and Convolvulus arvensis. J. Plant Dis. Prot. 114 (5), 232–238. doi: 10.1007/BF03356223
Bowers R. C. (1986). Commercialization of collego™ - an industrialist's view. Weed Sci. 34 (S1), 24–25. doi: 10.1017/S0043174500068326
Business Queensland (2020) Giant rat's tail grass (Brisbane QLD: Queensland Government). Available at: https://www.business.qld.gov.au/industries/farms-fishing-forestry/agriculture/land-management/health-pests-weeds-diseases/weeds-diseases/invasive-plants/restricted/giant-rats-tail-grass (Accessed 23rd October 2020).
Charudattan R. (1988). “Inundative control of weeds with indigenous fungal pathogens,” in Fungi in biological control systems. Ed. Burge M. N. (Manchester: Manchester University Press), 86–110.
Crous P. W., Boers J., Holdom D. G., Osieck E. R., Steinrucken T. V., Tan Y. P., et al. (2022). Fungal planet description sheets: 1383-1435. Persoonia - Mol. Phylogeny Evol. Fungi 48 (1), 261–371. doi: 10.3767/persoonia.2022.48.08
Crous P. W., Cowan D. A., Maggs-Kölling G., Yilmaz N., Thangavel R., Wingfield M. J., et al. (2021). Fungal planet description sheets: 1182-1283. Persoonia - Mol. Phylogeny Evol. Fungi 46 (1), 313–528. doi: 10.3767/persoonia.2021.46.11
Crous P. W., Wingfield M. J., Chooi Y. H., Gilchrist C. L. M., Lacey E., Pitt J. I., et al. (2020). Fungal planet description sheets: 1042 - 1111. Persoonia - Mol. Phylogeny Evol. Fungi 44 (1), 301–459. doi: 10.3767/persoonia.2020.44.11
Depetris M. B., Acuña C. A., Gutierrez S., Marcón F., Felitti S. A. (2020). Fungal endophyte diversity in Paspalum and effects on plant growth. Grass Forage Sci. 75 (3), 316–325. doi: 10.1111/gfs.12494
Dissanayake A. J., Purahong W., Wubet T., Hyde K. D., Zhang W., Xu H., et al. (2018). Direct comparison of culture-dependent and culture-independent molecular approaches reveal the diversity of fungal endophytic communities in stems of grapevine (Vitis vinifera). Fungal Diversity. 90, 85–10. doi: 10.1007/s13225-018-0399-3
Fletcher G. J., Leemon D. (2015). "Biological control of giant rat’s tail grass utilising nigrospora oryzae - final report" (Sydney Australia: Meat & Livestock Australia).
Galea V. J. (2021). Use of stem implanted bioherbicide capsules to manage an infestation of Parkinsonia aculeata in northern Australia. Plants 10 (9), 1909. doi: 10.3390/plants10091909
Gao S., Meng W., Zhang L., Yue Q., Zheng X., Xu L. (2021). Parametarhizium (Clavicipitaceae) gen. nov. with two new species as a potential biocontrol agent isolated from forest litters in northeast China. Front. Microbiol. 12. doi: 10.3389/fmicb.2021.627744
Hao Y., Aluthmuhandiram J. V. S., Chethana K. W. T., Manawasinghe I. S., Li X., Liu M., et al. (2020). Nigrospora species associated with various hosts from Shandong peninsula, China. Mycobiology 48 (3), 169–183. doi: 10.1080/12298093.2020.1761747
Hernández-Restrepo M., Madrid H., Tan Y. P., da Cunha K. C., Gené J., Guarro J., et al. (2018). Multi-locus phylogeny and taxonomy of Exserohilum. Persoonia 41, 71–108. doi: 10.3767/persoonia.2018.41.05
Hetherington S. D. (1997). Evaluation of endemic fungal pathogens as biological control agents of Sporobolus species. doctoral thesis (Brisbane: The University of Queensland). doi: 10.14264/uql.2019.332
Hetherington S. D., Irwin J. A. G. (1999). Pathological and molecular genetic variation in the interaction between Sporobolus spp. and Bipolaris spp. Aust. J. Agric. Res. 50 (4), 583–588. doi: 10.1071/a98126
Higgins K. L., Coley P. D., Kursar T. A., Arnold A. E. (2011). Culturing and direct PCR suggest prevalent host generalism among diverse fungal endophytes of tropical forest grasses. Mycologia 103 (2), 247–260. doi: 10.3852/09-158
Huelsenbeck J. P., Ronquist F. (2001). MRBAYES: Bayesian inference of phylogenetic trees. Bioinformatics 17 (8), 754–755. doi: 10.1093/bioinformatics/17.8.754
Katoh K., Misawa K., Kuma K. I., Miyata T. (2002). MAFFT: a novel method for rapid multiple sequence alignment based on fast Fourier transform. Nucleic Acids Res. 30 (14), 3059–3066. doi: 10.1093/nar/gkf436
Kukuntod N. (2020). “The study of fungal pathogens as biological controls of weedy sporobolus grasses (WSG),” in Bachelor of Environmental Management (Honours) Thesis. (Brisbane, QLD, Australia: University of Queensland).
Lawrie A. C. (2011). "Project no AWRC 08-08 using the fungus nigrospora oryzae for the biological control of giant parramatta grass" (Canberra: Canberra, ACT).
Lock C. (2018). Investigation of fungal pathogens for the biological control of giant rat’s tail grass (Sporobolus natalensis) in Australia. Bachelor of Environmental Management (Honours) Thesis. (Brisbane, QLD, Australia: University of Queensland).
Luo J., Vines P. L., Grimshaw A., Hoffman L., Walsh E., Bonos S. A., et al. (2017). Magnaporthiopsis meyeri-festucae, sp. nov., associated with a summer patch-like disease of fine fescue turfgrasses. Mycologia 109 (5), 780–789. doi: 10.1080/00275514.2017.1400306
Luttrell E. S. (1976). Ovarian infection of Sporobolus poiretii by Bipolaris ravenelii. Phytopathology 66, 260–268. doi: 10.1094/Phyto-66-260
Mascarin G. M., Jaronski S. T. (2016). The production and uses of Beauveria bassiana as a microbial insecticide. World J. Microbiol. Biotechnol. 32 (11), 177. doi: 10.1007/s11274-016-2131-3
McRae C. F., Auld B. A. (2000). “Inundative biological control of weeds - the bioherbicide tactic,” in Australian Weed management systems. Ed. Sindel B. M. (Maryborough: Australian Print Group), 193–208.
Morin L. (2020). Progress in biological control of weeds with plant pathogens. Annu. Rev. Phytopathol. 58 (1), 201–223. doi: 10.1146/annurev-phyto-010820-012823
Palmer B. (2012). “Sporobolus spp. - weedy Sporobolus grasses,” in Biological control of weeds in Australia. Eds. Julien M., McFayden R., Cullen J. (Collingwood: CSIRO Publishing), 569–575.
Peay K. G. (2014). Back to the future: natural history and the way forward in modern fungal ecology. Fungal Ecol. 12, 4–9. doi: 10.1016/j.funeco.2014.06.001
Peay K. G., Kennedy P. G., Talbot J. M. (2016). Dimensions of biodiversity in the earth mycobiome. Nat. Rev. Microbiol. 14 (7), 434–447. doi: 10.1038/nrmicro.2016.59
Peršoh D. (2015). Plant-associated fungal communities in the light of meta'omics. Fungal Diversity 75 (1), 1–25. doi: 10.1007/s13225-015-0334-9
Peterson P. M., Romaschenko K., Arrieta Y. H., Saarela J. M. (2014). A molecular phylogeny and new subgeneric classification of Sporobolus (Poaceae: Chloridoideae: Sporobolinae). Taxon 63 (6), 1212–1243. doi: 10.12705/636.19
Peterson P. M., Romaschenko K., Arrieta Y. H., Saarela J. M. (2017). A molecular phylogeny of the subtribe sporobolinae and a classification of the subfamily chloridoideae (Poaceae). Memoirs New York Botanical Garden 118, 127–151. doi: 10.21135/893275341.003
Phukhamsakda C., McKenzie E. H. C., Phillips A. J. L., Gareth Jones E. B., Jayarama Bhat D., Stadler M., et al. (2020). Microfungi associated with Clematis (Ranunculaceae) with an integrated approach to delimiting species boundaries. Fungal Diversity 102 (1), 1–203. doi: 10.1007/s13225-020-00448-4
Rapley D. R. B. (2020). The biology of Ustilago sporoboli-indici and its potential role in integrated management of weedy sporobolus grasses in Australia. Bachelor of Environmental Management (Honours) Thesis. (Brisbane, QLD, Australia: University of Queensland).
Saikkonen K., Lehtonen P., Helander M., Koricheva J., Faeth S. H. (2006). Model systems in ecology: dissecting the endophyte-grass literature. Trends Plant Sci. 11 (9), 428–433. doi: 10.1016/j.tplants.2006.07.001
Saikkonen K., Ruokolainen K., Huitu O., Gundel P. E., Piltti T., Hamilton C. E., et al. (2013). Fungal endophytes help prevent weed invasions. Agric. Ecosyst. Environ. 165, 1–5. doi: 10.1016/j.agee.2012.12.002
Simon B. K., Jacobs S. W. L. (1999). Revision of the genus Sporobolus (Poaceae, chloridoideae) in Australia. Aust. Systematic Bot. 12 (3), 375–448. doi: 10.1071/SB97048
Stamatakis A. (2014). RAxML version 8: a tool for phylogenetic analysis and post-analysis of large phylogenies. Bioinformatics 30 (9), 1312–1313. doi: 10.1093/bioinformatics/btu033
Sutton G. F. (2019). Searching for a needle in a haystack: Where to survey for climatically-matched biological control agents for two grasses (Sporobolus spp.) invading Australia. Biol. Control 129, 37–44. doi: 10.1016/j.biocontrol.2018.11.012
Tan Y. P., Crous P. W., Shivas R. G. (2016). Eight novel Bipolaris species identified from John l. alcorn’s collections at the Queensland plant pathology herbarium (BRIP). Mycological Prog. 15 (10-11), 1203–1214. doi: 10.1007/s11557-016-1240-6
Tan Y. P., Crous P. W., Shivas R. G. (2018). Cryptic species of Curvularia in the culture collection of the Queensland plant pathology herbarium. MycoKeys 35, 1–25. doi: 10.3897/mycokeys.35.25665
Templeton G. E., Greaves M. P. (1984). Biological control of weeds with fungal pathogens. Trop. Pest Manage. 30 (4), 333–338. doi: 10.1080/09670878409370907
Vega F. E., Posada F., Aime M. C., Pava-Ripoll M., Infant F., Rehner S. A. (2008). Entomopathogenic fungal endophytes. Biol. Control 46(1), 72–82. doi: 10.1016/j.biocontrol.2008.01.008
Vitelli J. S., Tan Y. P., Riding N., Holdom D. G., Chamberlain A., Shivas R. G. (2017). First record of Ustilago sporoboli-indici in Australia. Australas. Plant Dis. Notes 12 (1), 3. doi: 10.1007/s13314-017-0277-y
Wang M., Liu F., Crous P. W., Cai L. (2017). Phylogenetic reassessment of Nigrospora: ubiquitous endophytes, plant and human pathogens. Persoonia - Mol. Phylogeny Evol. Fungi 39 (1), 118–142. doi: 10.3767/persoonia.2017.39.06
Wong P. T. W., Tan Y. P., Weese T. L., Shivas R. G. (2022). Magnaporthiopsis species associated with patch diseases of turfgrasses in Australia. Mycosphere 13 (1), 602–611. doi: 10.5943/mycosphere/13/1/5
Yobo K. S., Laing M. D., Palmer W. A., Shivas R. G. (2009). Evaluation of Ustilago sporoboli-indici as a classical biological control agent for invasive Sporobolus grasses in Australia. Biol. Control 50 (1), 7–12. doi: 10.1016/j.biocontrol.2009.01.006
Keywords: systematics, pathogen diversity, biological control, new taxa, grass endophytes, poaceae
Citation: Steinrucken TV, Vitelli JS, Holdom DG and Tan YP (2022) The diversity of microfungi associated with grasses in the Sporobolus indicus complex in Queensland, Australia. Front. Fungal Biol. 3:956837. doi: 10.3389/ffunb.2022.956837
Received: 30 May 2022; Accepted: 26 July 2022;
Published: 19 August 2022.
Edited by:
Claudia Riccioni, Institute of Bioscience and Bioresources (CNR), ItalyReviewed by:
Divjot Kour, Eternal University, IndiaEverlon Cid Rigobelo, São Paulo State University, Brazil
Copyright © 2022 Steinrucken, Vitelli, Holdom and Tan. This is an open-access article distributed under the terms of the Creative Commons Attribution License (CC BY). The use, distribution or reproduction in other forums is permitted, provided the original author(s) and the copyright owner(s) are credited and that the original publication in this journal is cited, in accordance with accepted academic practice. No use, distribution or reproduction is permitted which does not comply with these terms.
*Correspondence: Tracey V. Steinrucken, TVSteinrucken@gmail.com