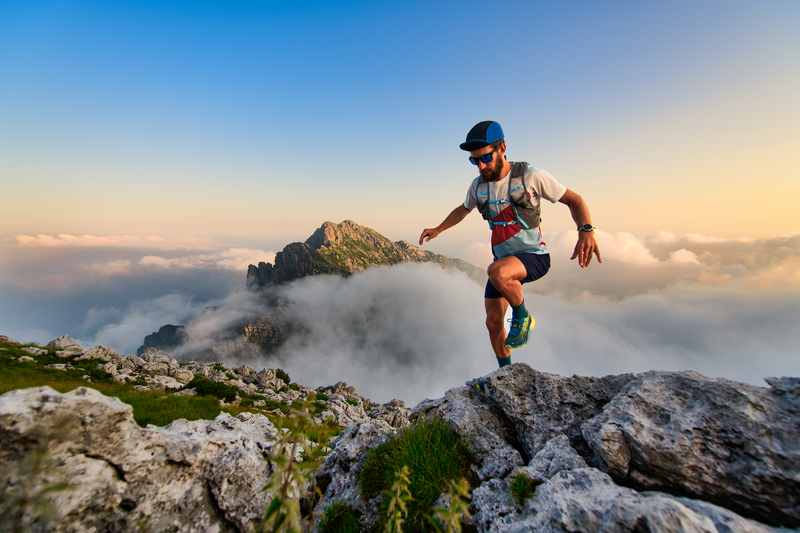
94% of researchers rate our articles as excellent or good
Learn more about the work of our research integrity team to safeguard the quality of each article we publish.
Find out more
ORIGINAL RESEARCH article
Front. Fungal Biol. , 03 January 2022
Sec. Fungal Secondary Metabolites and Mycotoxins
Volume 2 - 2021 | https://doi.org/10.3389/ffunb.2021.777474
The soil microbiome comprises numerous filamentous fungi and bacteria that mutually react and challenge each other by the production of bioactive secondary metabolites. Herein, we show in liquid co-cultures that the presence of filamentous Streptomycetes producing antifungal glycopeptide antibiotics induces the production of the antibacterial and iron-chelating tropolones anhydrosepedonin (1) and antibiotic C (2) in the mold Aspergillus nidulans. Additionally, the biosynthesis of the related polyketide tripyrnidone (5) was induced, whose novel tricyclic scaffold we elucidated by NMR and HRESIMS data. The corresponding biosynthetic polyketide synthase-encoding gene cluster responsible for the production of these compounds was identified. The tropolones as well as tripyrnidone (5) are produced by genes that belong to the broad reservoir of the fungal genome for the synthesis of different secondary metabolites, which are usually silenced under standard laboratory conditions. These molecules might be part of the bacterium-fungus competition in the complex soil environment, with the bacterial glycopeptide antibiotic as specific environmental trigger for fungal induction of this cluster.
Soil is a complex and heterogenous ecosystem highly colonized by microorganisms (Karlovsky, 2008). The dynamic interactions developed by microorganisms in soil to compete for nutrients and space range from symbiotic to antagonistic. Thus, microorganisms have developed a chemical arsenal for signaling, defense and protection in multispecies communities (Linares et al., 2006; Scherlach et al., 2013; Granato et al., 2019; Gerke et al., 2020). These low-molecular-weight organic compounds are called secondary metabolites (SMs, also specialized metabolites or natural products) and are, unlike primary metabolites, not primarily involved in growth, development or reproduction of the producing organism although there are regulatory ties between secondary and primary metabolism (Keller, 2019).
SMs represent a significant source for medical relevant drugs (Watve et al., 2001). Two-thirds of antibiotics with natural origin that are valuable in medicine or agriculture are produced by Streptomyces species (Bentley et al., 2002; Chater, 2006). One of those antibiotics is the glycopeptide bleomycin. It is an antitumor agent and produced by a non-ribosomal peptide synthetase (NRPS) and a polyketide synthase (PKS) (Shen et al., 1999; Galm et al., 2008). Streptomyces are Gram-positive bacteria and form the largest genus of Actinobacteria (Euzéby, 1997). They live in soil as decomposers of plant material in competition with other soil-borne microorganisms such as fungi. Similar to filamentous fungi, Streptomyces exhibit a mycelium with aerial filaments that can bear more than 50 spherical airborne spores (Bentley et al., 2002; Chater, 2006; Chater et al., 2010).
The filamentous fungus Aspergillus nidulans lives in the soil where it forms a hyphal network (Riquelme et al., 2018; Gerke et al., 2020). It can produce a huge arsenal of SMs, the function of which is far from all described. The genes responsible for biosynthesis, transport and regulation of SMs are usually clustered in the fungal genome (Keller, 2019; Rokas et al., 2020). A. nidulans harbors more than 60 different gene clusters (Inglis et al., 2013). Until today and even though A. nidulans is a fungal research model organism since decades, <50% have been linked to SM products (Romsdahl and Wang, 2019). Identification of SMs often proves difficult because most of the biosynthetic gene clusters are silenced under standard laboratory conditions. Over the last years, various methods have been developed to activate these gene clusters (Brakhage and Schroeckh, 2011; Gerke and Braus, 2014; Zhang et al., 2019). Mimicking natural competition in the soil by co-cultivation of A. nidulans with Streptomyces rapamycinicus revealed that the physical interaction induced the expression of silent fungal biosynthetic gene clusters (Schroeckh et al., 2009).
Here, we show that not only the physical interaction, but also the presence of a bacterial metabolite can serve as signal for the induction of a biosynthetic gene cluster producing tropolones in A. nidulans. Co-cultivation of A. nidulans with the glycopeptide antibiotic-producing Streptomyces mobaraensis induced the biosynthesis of the tropolones anhydrosepedonin (1) and antibiotic C (2) which both inhibit bacterial growth. They are formed by the PKS-containing and 2,4-dihydroxy-3-methyl-6-(2-oxopropyl)benzaldehyde (DHMBA)-forming (3) dba (derivative of benzaldehyde) gene cluster in combination with the co-regulated, adjacent gene AN7893 (troA) encoding a non-heme Fe(II) dioxygenase. The addition of pure glycopeptide antibiotics such as bleomycin or phleomycin was sufficient to induce the fungal response. Further, the metabolites azanidulone (4) and tripyrnidone (5) were identified to be produced phleomycin-dependently by the dba cluster. Finally, the biosynthesis of the dba-dependent SMs was identified. These results confirm that SMs can serve as chemical weapons in a competition between A. nidulans and S. mobaraensis.
Escherichia coli DH5α and Morganella morganii SBK3 were cultivated in Lysogeny Broth (LB), containing 1% (w/v) bacto tryptone, 1% (w/v) NaCl and 0.5% (w/v) yeast extract, at 37°C. A. nidulans strains were cultivated in Minimal Medium (MM) [AspA (70 mM NaNO3, 11.2 mM KH2PO4, 7 mM KCl, pH 5.5), 1% (w/v) glucose, 0.1% (v/v) Hutner's trace elements] or in London medium (LM) [1% (w/v) glucose, 7 mM KCl, 2 mM MgSO4, 11.2 mM KH2PO4, 0.1% (v/v) Hutner's trace elements (Hill and Kafer, 2001) without iron sulfate, 10 μM FeCl3, 10 mM NaNO3, pH = 6.5] with 0.0001% (w/v) 4-aminobenzoic acid at 30 or 37°C. Two percentage (w/v) agar was added for cultivation in petri dishes. For induction of the dba gene cluster, 0.1 μg/ml bleomycin (VWR, 203408-10) or 1 μg/ml phleomycin (InvivoGen, ant-ph-5p) were added. All Aspergillus strains were constructed in AGB552 (Bayram et al., 2012) background. Streptomyces strains were cultivated in GYM medium (4 g/l glucose, 4 g/l yeast extract, 10 g/l malt extract, pH = 7.2). For solid GYM medium, 2% (w/v) agar and 2 g/l CaCO3 were added. For co-cultivation of Streptomyces strains with A. nidulans, 93 ml London medium were mixed with 32 ml GYM medium and inoculated with each 1.25*107 spores of Streptomyces spp. and A. nidulans AGB552. The cultures were grown for 2 days at 120 rpm and 30°C. S. mobaraenis (DSM 40847) was provided by Deutsche Sammlung von Mikroorganismen und Zellkulturen GmbH (DSMZ). Streptomyces coelicolor and Streptomyces cellulosae subsp. griseorubiginosus were provided by Axel Zeeck. M. morganii SBK3 was provided by Günther Winkelmann.
For the construction of plasmids used for gene deletions in A. nidulans, pME4319 served as vector, which contains a recyclable phleomycin resistance cassette (Phle resistance gene from Streptoalloteichus hindustanus (ble) (Drocourt et al., 1990; Punt and van den Hondel, 1992; Hartmann et al., 2010; Liu et al., 2021). Sequences of all used primers in this study are listed in Supplementary Table 1. Approximately 1,500 bp of the 5' and 3' flanking regions of the gene of interest were inserted into the SwaI and PmlI sites of pME4319, respectively, by using the GeneArt™ Seamless Cloning and Assembly Enzyme Mix or the GeneArt™ Seamless Cloning and Assembly Kit (Thermo Fisher Scientific). The 5' and 3' flanking regions were amplified from A. nidulans genomic DNA with the primer pairs given in Supplementary Table 2. For transformation of A. nidulans, the deletion cassettes were excised from the corresponding plasmids with PmeI. Supplementary Table 3 includes all plasmids and Supplementary Table 4 all A. nidulans strains used in this study. E. coli and A. nidulans were transformed using the heat-shock method (Inoue et al., 1990) or the polyethylene glycol-mediated protoplast fusion method (Punt and van den Hondel, 1992), respectively. 5 μg/ml phleomycin was added to the medium to select for positive A. nidulans clones. The recyclable marker cassette in A. nidulans was removed by cultivation of the fungus on Minimal Medium containing 0.5% (w/v) glucose and 0.5% (w/v) xylose. Thereby, the xylose inducible promoter of the cassette activates expression of the ß-recombinase, whose gene product recognizes and cleaves off the marker cassette at its two flanking β-six recognition sites, leaving one β-six site (Hartmann et al., 2010; Liu et al., 2021).
Fungal mycelia were harvested, frozen in liquid nitrogen and ground with a table mill. RNA was isolated according to the instruction of the RNeasy® Plant Miniprep Kit (Qiagen). Approximately 0.8 μg of RNA was used for cDNA synthesis according to manufacturer's instructions of the QuantiTect® Reverse Transcription Kit (Qiagen). RT-PCR was performed with the MESA GREEN qPCR MasterMix Plus for SYBR Assay (Eurogentec) in a CFX Connect Real-Time System (BioRad). One microliter of 1:10 diluted cDNA was used per reaction. Two biological replicates with each three technical replicates were used. Primers are listed in Supplementary Table 5. Data were recorded with the CFX Manager software (BioRad). Expression of the housekeeping genes h2A, gpdA, and rps15 were used for normalization.
For SM extraction from liquid cultures, mycelia were removed by filtration. The aqueous filtrates were adjusted to pH 5 and extracted with equivalent volumes of ethyl acetate. The organic solvent was evaporated, and the dried metabolites were stored at −20°C. For LC-MS analysis, the metabolites were reconstituted in acetonitrile/H2O (1:1) and analyzed with a Q Exactive™ Focus orbitrap mass spectrometer coupled to an UltiMate™ 3000 HPLC (Thermo Fisher Scientific) equipped with a DAD-3000 Diode Array Detector (Thermo Fisher Scientific) and a Corona™ Veo™ RS Charged Aerosol Detector (Thermo Fisher Scientific). Five microliter of each sample was injected on a HPLC column [Acclaim™ 120, C18, 5 μm, 120 Å, 4.6 × 100 mm (Thermo Fisher Scientific)] applying a linear acetonitrile/0.1% (v/v) formic acid in H2O/0.1% (v/v) formic acid gradient [from 5 to 95% (v/v) acetonitrile/0.1 formic acid in 20 min, plus additional 10 min with 95% (v/v) acetonitrile/0.1 formic acid] with a flow rate of 0.8 ml/min at 30°C. The measurements were performed in a mass range of 70–1,050 m/z in positive and negative mode. Data acquisition and analysis was performed with Thermo Scientific Xcalibur™ 4.1 (Thermo Fisher Scientific) and with FreeStyle™ 1.6 (Thermo Fisher Scientific) software.
For SM extraction of the fungal biomass, the mycelia were washed with water and dried. The mycelia were covered with 100 ml acetone and placed in a Sonorex™ Digital 10 P ultrasonic bath (Bandelin) for 30 min at 10% performance. The mycelia were removed by filtration and anhydrous magnesium sulfate was added to the organic filtrate to bind remaining water. After another filtering the organic solvent was evaporated as described before. For the HR-ESI-MS analysis, the metabolites were reconstituted in acetonitrile/H2O (1:1) and 2 μl was injected into an Agilent 1200 Infinity Series HPLC (Agilent Technologies, Böblingen, Germany) utilizing a C18 Acquity® UPLC BEH column (2.1 × 50 mm, 1.7 μm; Waters, Milford, MA/USA) connected to a maXis® ESI-TOF-MS (Bruker). HPLC parameters were set as follows: solvent A: H2O + 0.1% formic acid, solvent B: acetonitrile + 0.1% formic acid; gradient: 5% B (0.5 min), 5–100% (19.5 min), 100% (5 min), flowrate 0.6 ml/min, and DAD detection 190–600 nm.
NMR spectra were recorded with an Avance III 700 spectrometer with a 5 mm TCI cryoprobe (1H 700 MHz, 13C 175 MHz) and an Avance III 500 spectrometer (1H 500 MHz, 13C 125 MHz; both Bruker, Billerica, MA/USA). NMR data were referenced to selected chemical shifts of acetone-d6 (1H: 2.05 ppm, 13C: 29.32 ppm), pyridine-d5 (1H: 7.22 ppm, 13C: 123.87 ppm) and DMSO-d6 (1H: 7.27 ppm, 13C: 77.00 ppm), respectively. Optical rotations were taken with a MCP 150 polarimeter (Anton Paar, Graz, Austria); UV spectra were taken with a UV-2450 UV/VIS spectrophotometer (Shimadzu, Kyoto, Japan); ECD spectra were collected with a JD 815 spectrophotometer (Jasco, Pfungstadt, Germany).
ESI-MS spectra were recorded with an UltiMate® 3000 Series uHPLC (Thermo Fisher Scientific, Waltman, MA/USA) utilizing a C18 Acquity® UPLC BEH column (2.1 × 50 mm, 1.7 μm; Waters, Milford, MA/USA) connected to an amaZon® speed ESI Iontrap MS (Bruker). HPLC parameters were set as follows: solvent A: H2O + 0.1% formic acid, solvent B: acetonitrile + 0.1% formic acid; gradient: 5% B (0.5 min), 5–100% (19.5 min), 100% (5 min), flowrate 0.6 mL/min, and DAD detection 190–600 nm. HR-ESI-MS spectra were obtained with an Agilent 1200 Infinity Series HPLC (Agilent Technologies, Böblingen, Germany; conditions same as for ESI MS spectra) connected to a maXis® ESI-TOF-MS (Bruker).
Anhydrosepedonin (1) and antibiotic C (2) were isolated from crude extracts of A. nidulans strains: AGB535 (ΔdbaF) was used for the isolation of the mixture of anhydrosepedonin (1) and antibiotic C (2) as well as the isolation of pure antibiotic C (2), whereas anhydrosepedonin (1) was purified from AGB1430 (ΔdbaB). In total 5 L liquid LM supplemented with 10 μM FeCl3, 0.0001% (w/v) 4-aminobenzoic acid and 1 μg/ml phleomycin (InvivoGen, ant-ph-5p) was inoculated with 1 × 106 spores/ml of either AGB535 or AGB1430 and incubated on a shaker for 2 days at 30°C. Mycelia were removed by filtration, the aqueous filtrates were adjusted to pH 5 and extracted with equivalent volumes of ethyl acetate. The organic solvent was evaporated, and the crude extracts were stored at −20°C.
98 mg crude extract was dissolved in 3 mL MeOH/DMSO (9:1) and subjected to preparative reverse phase HPLC (Pure C-850 FlashPrep, Büchi Labortechnik, Flawil, Switzerland). As stationary phase, Synergi Polar-RP column (250 × 50 mm, 10 μm, Phenomenex, Torrance, CA, USA) was used. The mobile phase consisted of deionized water (solvent A) and acetonitrile (solvent B). Purification of the crude extract was performed by using an isocratic elution of 5% aqueous ACN with 0.05% formic acid at a flow rate of 50 mL/min for 5 min, 5–100% solvent B in 60 min and finally isocratic elution at 100% solvent B for 10 min to afford the mixture of compound 1 and 2 (tR: 37.7–38.1 min, 12.1 mg).
220 mg crude extract of AGB1430 (ΔdbaB) was dissolved in 5 mL MeOH and subjected to preparative reverse phase HPLC (PLC 2250, Gilson, Middleton, WI, USA). As stationary phase, Synergi Polar-RP column (250 × 50 mm, 10 μm, Phenomenex, Torrance, CA, USA) was used. The mobile phase consisted of deionized water (solvent A) and acetonitrile (solvent B). Purification of the crude extract was performed by using an isocratic elution of 15% aqueous ACN with 0.05% formic acid at a flow rate of 50 mL/min for 5 min, 15–50% solvent B in 50 min, 50–100% solvent B in 10 min, and finally isocratic elution at 100% solvent B for 10 min to afford compound 1 (tR: 46.0–46.5 min, 12.7 mg).
148.6 mg crude extract of AGB535 (ΔdbaF) was dissolved in 6 mL MeOH, divided into 4 equal parts and subjected to preparative reverse phase HPLC (Pure C-850 FlashPrep, Büchi Labortechnik, Flawil, Switzerland). As stationary phase, Luna C18(2) column (250 × 21.2 mm, 5 μm, Phenomenex, Torrance, CA, USA) was used. The mobile phase consisted of deionized water (solvent A) and acetonitrile (solvent B). Purification of the crude extract was performed by using an isocratic elution of 15% aqueous ACN with 0.05% formic acid at a flow rate of 20 mL/min for 5 min, 15–45% solvent B in 32 min, 50–100% solvent B in 3 min, and finally isocratic elution at 100% solvent B for 10 min to afford compound 2 (tR: 32.0–32.3 min, 3.6 mg).
Ten liter liquid MM was inoculated with 109 spores of strain AGB527 and grown at 37°C for 36 h. Mycelia were removed by filtering with Miracloth and the pH of the culture filtrate was adjusted to 5. The culture filtrate was extracted twice with an equivalent amount of ethyl acetate and dried to yield the crude extract. The crude extract was separated by preparative HPLC (Instrumentelle Analytik Goebel GmbH: HPLC pump RAININ Dynamax SD-1, HPLC detector RAININ Dynamyx UV-1, column Nucleodur 100-5 C18 ec, 250 × 20 mm, solvent system: A = H2O, B = acetonitrile) under gradient conditions (20% B to 100% B in 20 min). Detection was carried out at 230 nm. The isolation yielded 7.7 mg 7-hydroxy-3,7-dimethyl-6H-2-benzopyran-6,8(7H)-dione that we named azanidulone. 1H-NMR spectra were recorded on a Varian Mercury-Vx 300 (300 MHz) spectrometer. 13C-NMR spectra were recorded on a Varian Inova-500 spectrometer (125.7 MHz). ESI-MS data were acquired using a Finnigan LC-Q mass spectrometer.
Four liter liquid LM with 1 μg/ml phleomycin was inoculated with 109 spores of strain AGB1418 and grown at 30°C for 2 days. Mycelia were removed and the pH of the culture filtrate was adjusted to 5. The culture filtrate was extracted twice with an equivalent amount of ethyl acetate and dried to yield 515.9 mg crude extract. The crude extract was dissolved in 10 mL MeOH and subjected to preparative reverse phase HPLC (PLC 2020, Gilson, Middleton, WI, USA). As stationary phase, VP Nucleodur 100-5 C18 ec column (250 × 20 mm, 7 μm, Macherey-Nagel, Düren, Germany) was used. The mobile phase consisted of deionized water (solvent A) and acetonitrile (solvent B). Purification of the crude extract was performed by using a linear gradient elution of 10–30% aqueous ACN with 0.05% formic acid at a flow rate of 20 mL/min for 35 min, 30–50% solvent B in 10 min, 50–100% solvent B in 5 min, and finally isocratic elution at 100% solvent B for 10 min to afford compound 5 (tR: 29–29.8 min, 3 mg).
C11H10O4, yellow solid, ESI-MS: m/z 206.9 [M + H]+, 204.9 [M – H]−. HRESIMS: m/z 207.0651 [M + H]+ (calcd. for C11H10O4 207.0657). UV (AcCN/H2O/0.1%FA) λmax 221 (sh), 250, 288, 381 nm. 1H-NMR (500 MHz, DMSO-d6): δH 6.76 (s, 1H, 7-H), 6.60 (s, 1H, 3-H), 5.73 (s, 1H, 8-H), 5.00 (s, 1H, 11-H), 1.93 (s, 3H, 10-H). 13C-NMR (125 MHz, DMSO-d6): δC 19.1 (CH3, C-10), 64.5 (CH2, C-11), 105.2 (CH, C-8), 111.2 (CH, C-7), 112.4 (CH, C-3), 112.7 (C, C-5), 140.9 (C, C-4), 161.2 (C, C-9), 161.6 (C, C-6), 165.8 (C, C-2), 169.8 (C, C-1).
C11H10O5, yellow solid, ESI-MS: m/z 222.9 [M + H]+, 220.8 [M – H]−. HRESIMS: m/z 223.0597 [M + H]+ (calcd. For C11H10O5 223.0607). UV (AcCN/H2O/0.1%FA) λmax 195, 223, 271, 305 (sh), 365, 385 nm. 1H-NMR (500 MHz, DMSO-d6): δH6.59 (s, 1H, 3-H), 5.70 (s, 1H, 8-H), 5.14 (s, 1H, 11-H), 1.91 (s, 3H, 10-H). 13C-NMR (125 MHz, DMSO-d6): δC 19.1 (CH3, C-10), 64.6 (CH2, C-11), 104.2 (CH2, C-8), 113.2 (CH, C-3), 114.1 (C, C-5), 132.9 (C, C-4), 146.5 (C, C-2), 154.3 (C, C-1), 155.1 (C, C-6), 157.8 (C, C-9), 160.9 (C, C-7).
C11H10O4, yellow solid, ESI-MS: m/z (%) 229.1 [M + Na]+, 207.1 [M + H]+. 1H-NMR (300 MHz, CD3OD): δH 1.46 (s, 3H, 10-H), 2.19 (s, 3H, 9-H), 5.44 (s, 1H, 5-H), 6.34 (s, 1H, 4-H), 8.01 (s, 1H, 1-H). 13C-NMR (500 MHz, CD3OD): δC 19.2 (CH3, C-11), 28.0 (CH3, C-12), 84.5 (C, C-7), 106.1 (CH, C-5), 109.8 (CH, C-4), 117.0 (C, C-9), 146.2 (C, C-10), 154.9 (CH, C-1), 161.5 (C, C-3), 198.6 (C, C-8), 198.9 (C, C-6). UV λmax (acetonitrile/H2O/formic acid)/nm: 228, 338. 1H-NMR data are in agreement with reported data (Marsini et al., 2006), 13C-NMR data are in agreement with reported data (Zhang et al., 2016).
C17H16O7: HRESIMS m/z 355.0777 [M + Na]+ (calcd. for C17H16O7Na, 355.0794), m/z 333.0961 [M + H]+ (calcd for C17H17O7, 333.0974), m/z 315.0857 [M − H2O + H]+ (calcd. for C17H15O6, 315.0869), m/z 331.0811 [M − H]− (calcd. for C17H15O7, 331.0818). UV (AcCN/H2O/0.1%FA) λmax 196, 217, 250 (sh), 292, 338 nm. 1H NMR (500 MHz, DMSO-d6): see Table 1; 13C NMR (125 MHz, acetone-d6): see Table 1; Rt 7.47 min.
Data for all identified compounds are shown in Supplementary Figures 2–11 and Supplementary Tables 6–8.
The bioassay for detection of siderophores with keto-hydroxy bidentate ligands was performed as described previously (Thieken and Winkelmann, 1993). Plates with solid LB medium (containing agar agar, SERVA, 9002-18-0) with or without 600 μM 2,2'-dipyridyl were inoculated with fresh M. morganii SBK3 over-night cultures. SMs were extracted from 125 ml liquid cultures of A. nidulans AGB552 and ΔdbaI as described above and dissolved in 100 μl methanol. Filter discs were coated with 10 μl metabolite extracts diluted 1:1 with methanol and placed on the agar. Ten microliter pure methanol served as control. Plates were cultivated for 3 days at 37°C in darkness. Growth and inhibition zones were detected and measured.
Bioactivity assays were performed to analyze the direct growth inhibiting effect of the SM extracts from A. nidulans on Streptomyces. 2 × 106 spores of S. mobaraensis, S. coelicolor, and S. cellulosae were distributed on whole agar plates containing 40 ml GYM medium. The SM extract of fungal reference strain AGB552 and ΔdbaI was dissolved in 80 μl methanol and 10 μl were spotted on a filter disk which was incubated with the inoculated plates for 2 days at 30°C. To test the direct effect of anhydrosepedonin and antibiotic C these metabolites were isolated from the fungal extracts by preparative HPLC. The anhydrosepedonin/antibiotic C mixture as well as separately isolated anhydrosepedonin (1) and antibiotic C (2) were dissolved in methanol to a final concentration of 2 mg/ml. 12.5 μl of the extract was spotted on filter disks, which were incubated with the Streptomyces inoculated GYM plates for 2 days at 30°C. Methanol served as control.
Minimum inhibitory concentrations (MIC) were determined as described previously (Becker et al., 2021). The following fungal and bacterial organisms were tested: Bacteria: Bacillus subtilis (DSM10), Staphylococcus aureus (DSM346), Acinetobacter baumanii (DSM30008), Chromobacterium violaceum (DSM30191), E. coli (DSM1116), Pseudomonas aeruginosa (PA14); mycobacteria: Mycolicibacterium smegmatis (ATCC700084); fungi: Candida albicans (DSM1665), Schizosaccharomyces pombe (DSM70572), Mucor hiemalis (DSM2656), Pichia anomala (DSM6766), Rhodotorula glutinis (DSM10134). The cytotoxicity assay against mouse fibroblast cell line L929, human cervical cancer cell line KB 3.1, human epidermoid carcinoma cell line A431, human lung carcinoma cell line A549, human prostate carcinoma cell line PC-3, human ovarian adenocarcinoma cell line SKOV-3 as well as human breast adenocarcinoma cell line MCF-7 was performed as described by (Becker et al., 2021).
To unravel the chemical response of A. nidulans toward different Streptomyces species, A. nidulans AGB552 was co-cultivated with S. mobaraensis, S. coelicolor or S. cellulosae on solid and in liquid medium at 30°C. The three Streptomyces strains are producers of different kinds of antibiotics (Höfs et al., 2000; Bentley et al., 2002; Komaki and Harayama, 2006).
First, solid GYM medium was inoculated with A. nidulans and one of the Streptomyces strains. After 6 days of growth an inhibition zone was visible between the colonies of S. mobaraensis and A. nidulans (Figure 1). No inhibition zone was observed when S. coelicolor or S. cellulosae were co-cultivated with A. nidulans. S. mobaraensis is a known producer of the glycopeptide antibiotic bleomycin, which is active against Aspergilli (Austin et al., 1990; Moore et al., 2003).
Figure 1. A growth inhibition zone is formed between colonies of S. mobaraensis and A. nidulans during co-cultivation. Confrontation assay of A. nidulans strain AGB552 with different Streptomycete strains (S. mobaraensis, S. coelicolor and S. cellulosae). 104 spores of A. nidulans (left colony) were point inoculated with 3 cm distance to 104 spores of Streptomycete strains (right colony) on GYM medium and incubated at 30°C for 6 days.
Next, SMs from liquid co-cultivation experiments were extracted to analyze whether A. nidulans produces specific molecules as a chemical response to S. mobaraensis, which might induce bacterial inhibition. After 2 days of cultivation, mycelia were removed and the metabolites in the filtrate were extracted with ethyl acetate and analyzed in LC-MS/MS equipped with a diode array detector (DAD). The HPLC chromatogram at 366 nm revealed two peaks in the sample extracted from the S. mobaraensis co-culture that were not present in the other co-cultures (Figure 2).
Figure 2. Co-cultivation of A. nidulans with S. mobaraensis induces the production of anhydrosepedonin (1), antibiotic C (2) and 2,4-dihydroxy-3-methyl-6-(2-oxopropyl)benzaldehyde (DHMBA, 3). (A) A. nidulans reference strain AGB552 (rf) was co-cultivated with S. mobaraensis, S. coelicolor and S. cellulosae in liquid medium for 2 days. SMs were extracted from medium and analyzed with LC-MS/MS equipped with a DAD. The relative absorbance at 366 nm is shown. As control, only A. nidulans was cultivated. (B) Extracted ion chromatograms (EIC) for DHMBA (3).
S. mobaraensis is a producer of the glycopeptide antibiotic bleomycin, which shows antifungal activity against Aspergilli and other ascomycetes (Austin et al., 1990; Moore et al., 2003). A growth test of A. nidulans AGB552 on medium supplemented with different concentrations of bleomycin revealed that A. nidulans grows on low concentrations of 0.1 μg/ml bleomycin (Supplementary Figure 1). To unravel the bacterial signal for fungal production of the tropolones, A. nidulans AGB552 was cultivated in liquid medium supplemented with 0.1 μg/ml bleomycin. After metabolite extraction, the chromatogram revealed the production of several metabolites that were not present in extracts from medium without bleomycin (Figure 3A). Phleomycin is another glycopeptide antibiotic produced by Streptomyces and closely related to bleomycin. According to the literature, A. nidulans cannot grow at phleomycin concentrations of c ≥ 10 μg/ml (Punt and van den Hondel, 1992). A growth test of A. nidulans AGB552 on medium supplemented with phleomycin revealed fungal growth at 1 μg/ml phleomycin (Supplementary Figure 1A). Cultivation of A. nidulans AGB552 in liquid medium supplemented with 1 μg/ml phleomycin and subsequent metabolite analysis showed the production of metabolites 1 and 2 as well as the two new peaks 4 and 5 (Figure 3B) that were also detected in cultures induced by bleomycin (Figure 3A; Supplementary Table 6).
Figure 3. The presence of the glycopeptide antibiotics bleomycin and phleomycin induces the production of the dbaI-dependent metabolites 1, 2, 4, and 5. A. nidulans reference strain AGB552 (rf) was cultivated in liquid medium with or without the presence of 0.1 μg/ml bleomycin (A) or 1 μg/ml phleomycin (B) for 2 days. SMs were extracted from medium and analyzed with LC-MS/MS equipped with a DAD. The relative absorbance at 366 nm is shown.
Metabolites 1 and 2 were isolated by preparative HPLC and elucidated as the tropolones anhydrosepedonin (1) and antibiotic C (2) (Figure 4A) by NMR, HRESIMS and UV/VIS data. In addition, DHMBA (3) was identified by HRESIMS and UV/VIS spectra in small amounts from the S. mobaraensis co-culture. DHMBA (3) was identified earlier as PKS-derived metabolite in A. nidulans (Ahuja et al., 2012; Gerke et al., 2012). Anhydrosepedonin (1) has been isolated earlier from the fungus Sepedonium chrysospermum (Wright et al., 1970) and antibiotic C (2) from A. nidulans strain IMI238850 (McDonald et al., 1983), but no full set of NMR data has been published. The corresponding biosynthetic gene clusters for both compounds have not been identified, either. We conclude that the three identified compounds are produced by A. nidulans in co-culture with S. mobaraensis.
Figure 4. Overview of identified metabolites and their structures. (A) Chemical structures of anhydrosepedonin (1), antibiotic C (2), DHMBA (3), azanidulone (4), tripyrnidone (5), sepedonin (6) and TAL, (7). The stereochemistry of 6 is not known. (B) Selected 1,1 ADEQUATE (red arrows) and 1H,13C HMBC (black arrows) correlations of compound 5.
Compound 4 was isolated by preparative HPLC and identified as the azaphilone 7-hydroxy-3,7-dimethyl-6H-2-benzopyran-6,8(7H)-dione that we named azanidulone (Figure 4). It has been isolated before from the ascomycete Daldinia concentrica (Buchanan et al., 1995) and the ascomycete Nigrospora sp. YE3033 (Zhang et al., 2016). A 7S configuration was assigned due to a negative CE observed at ca. 355 nm in the CD spectrum of 4 (Supplementary Figure 7) (Clark et al., 2008).
Taken together, the glycopeptide antibiotics bleomycin and phleomycin, which are naturally produced by Streptomyces, induce the production of the tropolones anhydrosepedonin (1) and antibiotic C (2) as well as of DHMBA (3), azanidulone (4) and 5 in A. nidulans.
Compound 5 was isolated by preparative HPLC as a red oil. Its UV/VIS spectrum with maxima at 292 and 389 nm indicated an extensive π-system. The molecular formula C17H16O7 was obtained from the peak at m/z 333.0972 in the HRESIMS spectrum, specifying 10 units of unsaturation. The 1H and 13C NMR spectra of 5 in DMSO-d6 contained two sets of resonances with a ratio of about 4:3, signifying spontaneous interconversion. Structure elucidation is described with data of the main isomer as follows: The proton and HSQC spectra of 5 indicated the presence of three methyls (δC/δH 29.5/2.19, 23.1/1.25, 19.8/2.28), one methylene (δC/δH 46.3/3.83 and 3.76) and three olefinic methines (δC/δH 124.0/5.92, 121.2/6.89, 100.1/6.34). Additionally, the carbon NMR spectrum showed signals for two ketones (δC 204.4, 198.5) and eight additional (δC 165.1, 163.2, 160.8, 147.8, 126.3, 100.0, 98.4, 78.9) carbon atoms devoid bound protons. Since 5 has a ratio of H/C atoms of <1, the crowding of HMBC correlations from just a few protons complicated the structure elucidation process. Consequently, an ADEQUTE NMR spectrum was measured for the distinction between 2JC, H and 3JC, H correlations. Foremost, the assignment of two-bond correlations from 3-H to C−4, from 10–H to C−9 and C−11, from 13–H to C−12 and C−14, from 15–H3 to C−14 and from 17–H3 to C−7 facilitated the assignment. Subsequently, observed 3J correlations from the HMBC NMR spectrum assembled the planar structure on 5 (Figure 4B). The largest difference in chemical shift between the isomers was observed for methyl group C−17 (δC 14.2 vs. 23.1), suggesting that the isomers differ in this region of the molecule. Since all HMBC correlations are analogous for both isomers, a stereo chemical difference was expected. The stereochemistry of C−7 is immutable and can be deduced from the absolute configuration of the biosynthetically related azanidulone (4) as 7S, but the neighboring hemiketal C−8 is metabolically unstable and prone to epimerize. Since a ROESY correlation between 8–OH and 17–H3 was observed for the minor isomer, 7S, 8S and 7S,8R absolute configurations were assigned for the minor and for the major epimer, respectively.
Compound 5 is a novel substance with a novel tricyclic scaffold that we named tripyrnidone. It showed no bioactivity in our test assays against bacteria or fungi and no cytotoxicity against the tested cell lines.
DHMBA (3) was shown to be the direct product of the non-reducing PKS DbaI in A. nidulans (Ahuja et al., 2012; Gerke et al., 2012). We deleted dbaI in AGB552 background and analyzed the SM profile with LC-MS/MS with and without the addition of bleomycin and phleomycin (Figure 3). The deletion of dbaI abolished the production of DHMBA (3) as well as of the SMs 1, 2, 4, and 5. It can be concluded that the tropolones as well as the compounds 4 and 5 are produced by the PKS DbaI and are most likely biosynthesized from DHMBA by other enzymes.
In fungi, biosynthetic genes for a specific SM are usually clustered in the genome (Keller, 2019; Rokas et al., 2020). The gene encoding the DHMBA-producing PKS DbaI is embedded within the dba gene cluster on chromosome II of A. nidulans (Gerke et al., 2012) (Figure 5A). The cluster spans 9 genes, dbaA-dbaI, and encodes two putative transcription factors DbaA and DbaG. Overexpression of dbaA upregulates all genes of the cluster, which is usually silenced under standard laboratory conditions (Gerke et al., 2012). To understand the influence of glycopeptide antibiotics on dba gene cluster expression, the relative gene expressions of the dba cluster genes were analyzed with quantitative real-time polymerase chain reaction (qPCR). The neighbored genes AN7890-AN7895 and AN7904 were included in the analysis. The genes AN7893-7895 were shown earlier to be co-regulated with the dba cluster under certain conditions (Nahlik et al., 2010; Gerke et al., 2012; Bohnert et al., 2014; Oiartzabal-Arano et al., 2015). The A. nidulans reference strain AGB552 (rf) and the two transcription factor deletion strains ΔdbaA and ΔdbaG were cultivated with and without phleomycin. The relative gene expression pattern showed that all 9 dba genes as well as AN7893-AN7895 were upregulated in the reference strain when phleomycin was added (Figure 5B). In contrast, expression of AN7893-dbaI was not increased in phleomycin-containing medium when dbaA was deleted, proving that DbaA is the specific transcriptional activator for the gene cluster. The putative transcription factor DbaG is not involved in dba gene regulation but showed a repressive function on expression of the uncharacterized AN7891 gene, which encodes a β-1,4-endoglucanase, in medium without phleomycin. In summary, the presence of 1 μg/ml phleomycin induces the expression of the dba genes plus AN7893-AN7895. To test what is the lowest phleomycin concentration for the gene cluster induction, we performed qPCR with RNA extracted from cultures containing 0.01 to 1 μg/ml phleomycin. The analysis revealed expression of dbaI at 0.5 and 1 μg/ml phleomycin, but lower concentrations are rather insufficient (Supplementary Figure 1B).
Figure 5. Expression of genes from AN7893 (troA) to AN7903 (dbaI) is induced in presence of phleomycin. (A) Scheme of dba gene cluster (orange) and neighbored genes (blue) on chromosome II of A. nidulans. (B) Relative gene expression levels of AN7890-AN7904 in reference strain AGB552 (rf), ΔdbaA and ΔdbaG after cultivation with (+) and without (–) the addition of 1 μg/ml phleomycin. Expression of the housekeeping genes h2A, gpdA and rps15 were used for normalization. Error bars represent the standard error of two biological replicates.
Compounds 1–5 are polyketides. The structures as well as the abolished production in the PKS deletion strain ΔdbaI suggest that the compounds 1, 2, 4, and 5 are synthesized from the DbaI-product DHMBA (3) (Gerke et al., 2012) by other co-regulated enzymes. Therefore, all genes from AN7893-dbaI were deleted in A. nidulans AGB552 background. A SM analysis with LC-MS/MS after cultivation in medium with phleomycin revealed that without the transcriptional activator DbaA and without the PKS DbaI the compounds 1–5 were not produced (Figure 6A; Supplementary Figure 12). Deletion of the transcription factor gene dbaG as well as AN7895, dbaE and dbaF had no impact on compound production. In the strain without the transporter gene dbaD, the compounds 2 and 4 were not detected in the medium, but 5, which is released to the medium independently of DbaD. 1 was only detected in very low amounts (Supplementary Figure 12A). An additional intracellular extraction of SMs from mycelia of ΔdbaD did not reveal the presence of any of the metabolites (Supplementary Figure 13). For the synthesis of anhydrosepedonin (1) the non-heme Fe(II) dioxygenase AN7893 as well as the FAD-dependent monooxygenase DbaH are needed in addition to the PKS DbaI. Interestingly, the production of 1 increased when dbaB was deleted, indicating that DbaB converts 1 into another compound. Antibiotic C (2) was not detected from strains lacking AN7893, AN7894, DbaB, DbaC, DbaH, and DbaI. To produce azanidulone (4) only the PKS DbaI and the FAD-dependent monooxygenase DbaH are necessary. Tripyrnidone (5) is not detected from strains without DbaB, DbaH and DbaI. Double deletion of dbaB and AN7893 abolished 1 and 2, but 3–5 were still produced (Figure 6B).
Figure 6. The dba/troA cluster produces the tropolones anhydrosepedonin and antibiotic C. (A) The indicated strains were cultivated in liquid medium supplemented with 1 μg/ml phleomycin for 2 days. Ethyl acetate extracts of culture filtrates were analyzed with LC-MS/MS equipped with a DAD. The relative absorbance at 366 nm is shown. (1) anhydrosepedonin, (2) antibiotic C, (3) DHMBA, (4) azanidulone, (5) tripyrnidone. (B) Extracted ion chromatograms of 1–5 in positive ionization mode. Extracted ion chromatograms of all compounds are shown in Supplementary Figure 12.
Starting from DHMBA as precursor, we propose the following biosynthesis for compounds 1, 2, and 4 (Figure 7). DHMBA is oxidized by the FAD-dependent monooxygenase DbaH at position C-3. A ring closure follows to form the azaphilone skeleton and a subsequent spontaneous release of a water molecule leads to the formation of azanidulone (4). For the biosynthesis of the tropolones 1 and 2, the carbon atom of the methyl group at position C-3 is incorporated by the non-heme Fe(II) dioxygenase AN7893 yielding sepedonin (6) with the 7-membered tropolone ring. A spontaneous water molecule release forms the tropolone anhydrosepedonin (1), which is further oxidized by the FAD-dependent monooxygenase DbaB to the tropolone antibiotic C (2). In summary, the PKS DbaI, DbaH and AN7893 are responsible for the formation of tropolones. Due to its key role in tropolone ring formation, AN7893 was designated TroA (tropolone-forming A).
Figure 7. Proposed biosynthesis of compounds 1–6. Compounds shown with names have been detected, compounds without names are hypothetical. The stereochemistry of 6 is not known.
Since the putative intermediate sepedonin (6) was not identified from the HPLC chromatogram at 366 nm, we searched for the presence of its mass in the LC-MS/MS data of the reference and the deletion strains. The extracted ion chromatogram at m/z = 225.0757 [M + H]+ revealed a peak with the UV/VIS spectrum of sepedonin (Supplementary Figure 2) (Supka, 1981). As expected, sepedonin (6) is not produced in the deletion strains of troA, dbaA, dbaH, and dbaI. In contrast, the production of sepedonin (6) is enhanced in ΔdbaB as well as in the two YCII-domain encoding deletion strains ΔAN7894 and ΔdbaC. The function of YCII domains was not identified yet, but they seem to have a regulatory role on SM production.
For the biosynthesis of tripyrnidone (5), the oxidized DHMBA precursor presumably reacts with triacetic acid lactone (TAL). To date, TAL has not been identified from A. nidulans. We checked our LC-MS/MS data whether the reference strain AGB552 cultivated in bleomycin- or phleomycin-containing medium produces TAL. The extracted ion chromatogram at m/z = 127.0390 [M + H]+ revealed a peak at a retention time of 5.4 min (Figure 8). The peak was identified as TAL (7) by comparison with commercial TAL (Sigma-Aldrich). The TAL-producing PKS has not yet been identified in A. nidulans.
Figure 8. A. nidulans produces triacetic acid lactone (TAL, 7). The production is increased in the presence of glycopeptide antibiotics bleomycin and phleomycin but is independent from the PKS DbaI. Reference strain AGB552 (rf) and ΔdbaI were cultivated in liquid medium with or without the presence of 0.1 μg/ml bleomycin or 1 μg/ml phleomycin. SMs were extracted from medium and analyzed with LC-MS/MS. Extracted ion chromatograms (EIC) for m/z = 127.0390 in positive ionization mode are shown. TAL was identified by comparison with commercial TAL (Sigma-Aldrich, lower panel).
Theoretically, three tropolone molecules can form a complex with one Fe3+ ion. The iron binding activity of anhydrosepedonin (1) was shown earlier (Supka, 1981). To test whether the dba cluster produced tropolones can function as alternative siderophores, a bioassay for detection of siderophores with keto-hydroxy bidentate ligands was used (Thieken and Winkelmann, 1993). M. morganii SBK3 is a bacterium which uses siderophores with keto-hydroxy bidentate ligands for growth. Filter discs with A. nidulans SM extracts from reference strain AGB552 and ΔdbaI were put on LB agar plates supplemented with the iron chelator 2,2'-dipyridyl and inoculated with M. morganii. LB plates without 2,2'-dipyridyl were used as control. If the extract contains siderophores with keto-hydroxy bidentate ligands, a Morganella growth zone can be detected. The bioassay shows that M. morganii grows on the whole LB plate without 2,2'-dipyridyl but an inhibition zone of 1.6 ± 0.1 cm (p ≤ 0.01, n = 4) is visible for the extract of the reference strain, which is not present for the ΔdbaI extract (Figure 9). On plates where all free iron is bound by 2,2'-dipyridyl, M. morganii is able to grow in 3.9 ± 0.5 cm (p ≤ 0.001, n = 4) distance around the reference strain extract, whereas no growth is detected around the ΔdbaI extract. However, an inhibition zone is detected 2.6 ± 0.4 cm (p ≤ 0.001, n = 4) around the reference strain extract, surrounded by the growth zone. No bacterial growth can be observed close to the extract from the PKS deletion strain ΔdbaI neither to the methanol control. These results show that the tropolones produced by the A. nidulans AGB552 upon induction of the dba/troA gene cluster by phleomycin serve as siderophores and provide the bacteria with iron at low SM concentrations. At high concentrations, the SMs produced by the dba/troA cluster have an inhibitory effect on growth of the Gram-negative M. morganii.
Figure 9. SMs of dba/troA cluster of A. nidulans are siderophores. Bioassay for the identification of siderophores with monoprotic keto-hydroxy bidentate ligands (KHBL). A. nidulans reference strain AGB552 (rf) and ΔdbaI were cultivated in liquid medium with phleomycin to induce the tropolone production. The metabolites were extracted, dissolved in methanol and spotted on filter disks. Pure methanol served as negative control. Solid LB medium without and with the iron-chelator 2,2'-dipyridyl (600 μM) were inoculated with M. morganii SBK3. The filter disks containing the metabolite extracts were placed on the agar plates, which were cultivated for 3 days at 37°C. The inhibition zone on LB without 2,2'-dipyridyl was 1.6 ± 0.1 cm (p ≤ 0.01, n = 4) for the reference strain AGB552 (rf) in comparison to 0.2 ± 0.4 cm for ΔdbaI. On LB with 2,2'-dipyridyl the rf extract induces an inhibition zone of 2.6 ± 0.4 cm (***p ≤ 0.001, n = 4) (blue bar) surrounded by a growth zone of 3.9 ± 0.5 cm (p ≤ 0.001, n = 4) (gray bar). No inhibition neither a growth zone was detected around the extract from ΔdbaI in presence of 2,2'-dipyridyl [n = 4, for significance testing a T-test (ri-rj) was used].
Confrontation experiments of A. nidulans and Streptomyces species on solid medium showed an inhibition zone (Figure 1). SM extraction revealed that A. nidulans produces the tropolones anhydrosepedonin and antibiotic C from the dba/troA cluster in response to either co-cultivation with S. mobaraensis or by media supplementation with the glycopeptides bleomycin and phleomycin. Hence, it was tested whether the SM extract from an A. nidulans culture supplemented with phleomycin would inhibit growth of Streptomyces species. Filter disks containing the extract of the reference strain AGB552 and the PKS deletion strain ΔdbaI were incubated with spores of different Streptomyces strains. The tested Streptomyces strains show a zone of inhibition around the filter disk containing the SM extract of the Aspergillus reference strain, whereas no inhibition zone was detected around the control filter disk containing methanol (Figure 10A). For the extract of the PKS deletion strain, only a minor or no inhibition zone was detected. These results revealed that the SM extract of the reference strain has a dba-cluster dependent antibacterial activity on Streptomyces strains.
Figure 10. Anhydrosepedonin/antibiotic C inhibit the growth of Streptomyces spp. Bioactivity tests with 2 × 106 spores of S. mobaraensis, S. coelicolor, and S. cellulosae distributed on whole GYM medium plates. (A) The SM extract of A. nidulans reference strain AGB552 (rf) and ΔdbaI was dissolved in methanol and 10 μl were spotted in a filter disk which was incubated with the inoculated plates for 2 days at 30°C. The extract of the reference strain induces a growth inhibition zone for all tested Streptomyces strains. Methanol served as control. (B) Anhydrosepedonin/antibiotic C were isolated from fungal extracts and 12.5 μl of the extract (stock concentration 2 mg/ml) resuspended in methanol was spotted on a filter disk and methanol served as control. The inhibition zone induced by anhydrosepedonin/antibiotic C is 1.9 cm (S. mobaraensis), 1.7 cm (S. coelicolor) and 1.5 cm (S. cellulosae) after 2 days of incubation at 30°C. (C) Anhydrosepedonin (1) and antibiotic C (2) were purified and tested separately. The compounds were dissolved in methanol to a final concentration of 2 mg/ml. Filter disc were coated with 12.5 μl extract and incubated with the Streptomyces inoculated GYM plates for 2 days at 30°C. Both metabolites induce an inhibition zone with a diameter of 1.4 cm (S. mobaraensis), 1 cm (S. coelicolor and S. cellulosae). Methanol served as control.
To test whether the antibacterial activity is based on anhydrosepedonin (1) and/or antibiotic C (2), which are components of the reference strain extract, these metabolites were isolated from A. nidulans (Supplementary Figure 14). A filter disk was coated with either the pure compounds or a mixture of both (Figures 10B,C). Both purified compounds as well as the mixture inhibited bacterial growth.
Furthermore, anhydrosepedonin (1) and antibiotic C (2) were subjected to a broad range bioactivity test pipeline. The bacteria B. subtilis (DSM10), S. aureus (DSM346), A. baumanii (DSM30008), C. violaceum (DSM30191), E. coli (DSM1116) and P. aeruginosa (PA14), the mycobacterium M. smegmatis (ATCC700084) and the fungi C. albicans (DSM1665), S. pombe (DSM70572), M. hiemalis (DSM2656), P. anomala (DSM6766) and R. glutinis (DSM10134) were used. Additionally, the mouse fibroblast cell line L929, human cervical cancer cell line KB 3.1, human epidermoid carcinoma cell line A431, human lung carcinoma cell line A549, human prostate carcinoma cell line PC-3, human ovarian adenocarcinoma cell line SKOV-3 and human breast adenocarcinoma cell line MCF-7 were used. Antibiotic C (2) showed a broad, weak activity against all the tested organisms, except for P. aeruginosa, and showed strong cytotoxic activity against the tested cell lines with the highest activity against the cell line from human breast adenocarcinoma with an IC50 of 0.041 μg ml−1. Comparison showed lower activities for the tested anhydrosepedonin (1) (Table 2).
Taken together, the soil fungus A. nidulans produces the SMs anhydrosepedonin (1) and antibiotic C (2) from the dba/troA gene cluster, which have an antibiotic activity against Streptomycetes and a range of different other microorganisms.
Secondary metabolites are used for defense, communication and competition of soil communities including microorganisms as well as their predators (Linares et al., 2006; Granato et al., 2019; Gerke et al., 2020; Liu et al., 2021). Fungi and bacteria are in a continuous rivalry in consumption of substrates in soil (Boer et al., 2005). This leads to a selection pressure on bacteria to compete for easily degradable nutrients. The result is a broad repertoire of antifungal strategies like antibiotic or nutrient sequestering factors such as iron-chelating siderophores (Boer et al., 2005), which were both in focus of this study. The enormous arsenal of secondary metabolites produced by fungi and bacteria contributes to their ecological success in colonizing almost all habitats (Bills and Gloer, 2016).
We uncovered an inter-species competition between the fungus A. nidulans and the bacterium S. mobaraensis. The latter produces the antifungal glycopeptide bleomycin, which was identified as chemical signal for the activation of the tropolone-producing gene cluster dba/troA in A. nidulans. Bleomycin and the related phleomycin show antifungal activity against Aspergilli (Austin et al., 1990; Moore et al., 2003). Both glycopeptides cause DNA double strand breaks at unmethylated sites and single strand breaks at inverted repeat sequences in a yet unknown mechanism (Kross et al., 1982; Hertzberg et al., 1985; Moore, 1989; Ueda et al., 1992). The mechanism of the bacterium-derived glycopeptide-mediated activation of the fungal dba/troA cluster and therefore tropolone synthesis are yet unknown. Several fungal SM gene clusters like ors (orsellenic acid), dba, cic (cichorine), mic (microperfuranone) and eas (emericellamide), which are controlled by the transcription factor BasR, were induced by bacterial signals upon co-cultivation of A. nidulans with S. rapamycinicus (Fischer et al., 2018). It will be interesting to examine whether bleomycin or phleomycin induce the expression for tropolone synthesis in a basR dependent manner. Vice versa, it will be interesting to analyze, whether fungal tropolones are capable of inducing the glycopeptide production of S. mobaraensis.
Whereas, in A. nidulans cultures supplemented with bleomycin only anhydrosepedonin and antibiotic C were detected, the supplementation with phleomycin additionally led to the identification of azanidulone (4), tripyrnidone (5), sepedonin (6) and TAL (7). Compound 4 was isolated before from the ascomycete D. concentrica (Buchanan et al., 1995) and the ascomycete Nigrospora sp. YE3033 (Zhang et al., 2016). An in vitro study showed that DHMBA (3) can be converted to azanidulone (4) by the addition of the enzyme AzaH of A. niger, which corresponds in A. nidulans to AN7902 coding for DbaH with 40% protein sequence identity (62% positives, 93% query coverage) (Altschul et al., 1990; Pyser et al., 2019). This suggests that no second enzyme is necessary to form the bicyclic ring of azanidulone (4) in A. nidulans. The novel scaffold of 5 was determined by NMR and high-resolution MS data and the compound was named tripyrnidone. Most likely, tripyrnidone is a PKS product of an oxidized DHMBA precursor that reacts with TAL. This study is the first to report about TAL (7) in A. nidulans. However, the non-reducing PKS predictively catalyzing this step is not yet identified. It has been shown that orsellinic aldehyde, which is structurally similar to DHMBA, can react with TAL to form precursors of melanin in Ustilago maydis (Reyes-Fernández et al., 2021).
This is the first report of the biosynthetic pathway of the tropolones anhydrosepedonin (1) and antibiotic C (2) in A. nidulans. This was elucidated by metabolite analysis of dba/troA single deletion strains (Figures 6A, 7). These mutants did not show alteration of their phenotype on medium without glycopeptides supporting that the dba cluster is silent under laboratory conditions (Gerke et al., 2012). We showed that the non-heme Fe(II) dioxygenase AN7893 is a key enzyme in the production of the tropolones anhydrosepedonin and antibiotic C in A. nidulans and therefore denoted AN7893 as TroA. Deletion of troA abolished the production of both compounds but not the production of the other dba-dependent metabolites 3–5 (Figure 6). It was recently discovered that non-heme Fe(II) dioxygenases are responsible for the incorporation of a C-atom to form the typical seven-membered ring of tropolones (Davison et al., 2012; Cox and Al-Fahad, 2013). The ascomycete Talaromyces stipitatus produces the tropolone stipitatic acid. The biosynthetic enzymes were identified as the non-reducing PKS TropA, the FAD-dependent monooxygenase TropB, the non-heme Fe(II) dioxygenase TropC as well as the cytochrome P450 monooxygenase TropD (Davison et al., 2012). A protein homology search revealed that the dba/troA cluster is fully conserved in T. stipitatus with protein sequence identities of 58–92% (Table 3; Supplementary Figure 15). By querying the JGI fungal genome database MycoCosm (Grigoriev et al., 2014) it was found that about 7.5% of Aspergillus species contain the dba/troA gene cluster. However, outside the Aspergillus, the dba/troA gene cluster was only detected in T. stipitatus ATCC 10500, in Coccidioides immitis and C. posadasii (Supplementary Table 9) (Sharpton et al., 2009; Davison et al., 2012). The dba cluster of Coccidioides spp. is rearranged and lacks an AN7901 (dbaG) orthologous gene (Supplementary Figure 15).
Table 3. Biosynthetic proteins encoded in dba/troA cluster of A. nidulans responsible for production of 1–5.
Besides biosynthetic genes, the dba cluster encodes genes for transcription control and transport. Deletion of the putative MFS transporter gene dbaD abolished the extracellular detection of 2 and 4, but these compounds were also not detected in intracellular extracts (Supplementary Figure 13). This indicates that instead of being a plasma membrane transporter, DbaD might be an organelle membrane transporter. For several fungal SMs it was shown that biosynthetic enzymes are co-compartmentalized and the biosynthetic intermediates are shuttled between different organelles in order to channel precursors and to promote pathway efficiency (Kistler and Broz, 2015).
Tropolones are rare natural products comprising a seven-membered aromatic ring, also called the tropolonoid motif, and are found in fungi, bacteria, and plants (Bentley, 2008; Davison et al., 2012; Cox and Al-Fahad, 2013; Guo et al., 2019). They show a wide range of bioactivity including antiviral, antitumor, bactericidal and bacteriostatic properties against a wide range of bacteria (Trust, 1975; Nagao et al., 2006; Ononye et al., 2013; Tavis and Lomonosova, 2015). Inhibited proliferation of some (pathogenic) fungi and an insecticidal effect are described against Tyrophagus putrescentiae, Dermatophagoides farina, and Captotermes formosanus (Morita et al., 2003, 2004; Quang et al., 2010; Azadbakht et al., 2020). In this study, the produced tropolones anhydrosepedonin (1) and antibiotic C (2) show antibacterial activity against S. mobaraensis. Further MIC tests revealed bioactivity against a broad range of bacteria and fungi as well as cytotoxicity against all tested cell lines (Table 2). The discovery of the biosynthetic pathways and novel compounds that are bioactive against different microorganisms is an interesting starting point for future research.
Streptomyces are rarely pathogenic to humans with only a few pathogenic species, wherefore the tropolone antibiotic effect against Streptomyces is rather irrelevant for pharmaceutical use. The reported broad spectrum of bioactivity of tropolones (Trust, 1975; Morita et al., 2003; Azadbakht et al., 2020; Duan et al., 2020) and our MIC tests suggest an promising medical potential of these identified compounds against other microorganisms and cancer cell lines. Biotechnological applications of 1 and 2 might be facilitated by our discovery of the biosynthetic pathway and the physiological condition under which the dba/troA cluster is active. The production of these tropolones under laboratory conditions using phleomycin is an advantage for further studies on their bioactive potential.
Tropolones not only show anti-microbial, anti-insecticidal and anti-cancer potential but have the ability of metalloprotease inhibition. Therefore, the bioactivity of different tropolones is often referred to their metal-chelating and redox potential (Morita et al., 2003; Chen et al., 2019). Our experiments show that the extracted tropolones anhydrosepedonin (1) and antibiotic C (2) can inhibit growth of S. mobaraensis, S. coelicolor, and S. cellulosae. Three tropolone molecules can bind one Fe3+ and therefore serve as siderophores (Supka, 1981). Siderophores are chelators produced by microorganisms for the uptake and storage of iron (Kraemer, 2004). Iron is an essential trace element for many vital pathways and cellular functions where it serves as cofactor for numerous enzymes (Coffey and Ganz, 2017; Misslinger et al., 2021). According to their structure, tropolones belong to the rare group of keto-hydroxy bidentate ligand (KHBL) siderophores. A bioassay for the detection of KHBL siderophores (Thieken and Winkelmann, 1993) showed that dba-dependent metabolites such as the tropolones anhydrosepedonin (1) and antibiotic C (2) are KHBL siderophores at least for M. morganii (Figure 9). Whether A. nidulans utilizes the tropolones as alternative siderophores is still speculative. Previously, it was shown that the biosynthetic precursor sepedonin (6) can function as KHBL siderophore as well (Thieken and Winkelmann, 1993). Interestingly, the KHBL siderophore bioassay further revealed that the dba/troA SMs have an antibiotic effect on the Gram-negative M. morganii. Around the SM-coated filter disc a SM concentration gradient exists due to diffusion. In short distance to the filter disc high metabolite concentrations are present inhibiting the growth of M. morganii, whereas in long distance to the filter disc with low SM concentrations a growth zone is present, showing that the dba/troA SMs can provide M. morganii with iron (Figure 9). A myriad of applications are described for siderophores (Ahmed and Holmström, 2014; Albelda-Berenguer et al., 2019). Siderophores are used in bioremediation of metal pollution and growth enhancement of plants. Siderophores have medical applications in reducing the iron overload during blood transfusion, or as sideromycins in which a siderophore is covalently bound to an antibiotic (Braun et al., 2009). In A. nidulans the siderophore ferricrocin is known for its iron storage properties and is involved in oxidative stress control (Eisendle et al., 2006; Albelda-Berenguer et al., 2019). It is interesting to further investigate the advantage of the newly identified siderophores of A. nidulans.
Even in well-studied organisms, the identification of the complete arsenal of SMs proves difficult, because the expression of the responsible biosynthetic gene clusters is often silenced under laboratory conditions. SMs are specialized chemicals, which are only active under certain environmental stimuli, developmental stages or due to genetic manipulation (Calvo et al., 2002; Gerke and Braus, 2014). Activation of certain biosynthetic gene clusters that produce metabolites used for signaling and defense in nature can be achieved by co-cultivation of different species (Schroeckh et al., 2009). The co-cultivation of this study helped to understand the physiological conditions needed to activate the dba/troA gene cluster. Thereby, we found otherwise overlooked bioactive compounds with potential relevant pharmacological use. The plethora of possible microbial interactions in the complex soil ecosystem was also used in previous co-cultivation studies to identify several new compounds. For example, A. niger in co-cultures with S. coelicolor resulted in the production of ten new compounds that were not present in monocultures (Wu et al., 2015). When co-cultivated with Streptomyces sp., Aspergillus flavipes produces cytochalasans, which are cytotoxic to the bacterium and thus provide an advantage for the fungus (Yu et al., 2016). S. rapamycinicus activated the cryptic meroterpenoid pathway synthesizing the beforehand unknown prenylated polyketide fumicycline in A. fumigatus (König et al., 2013). Another study showed that the physical interaction of A. nidulans with S. rapamycinicus induced the expression of three neighbored biosynthetic gene clusters, the PKS-containing ors gene cluster, producing orsellinic acid and F-9775A/B, the NRPS-containing atn gene cluster, producing aspercryptin, as well as the PKS-containing dba gene cluster producing DHMBA (Schroeckh et al., 2009; Gerke et al., 2012; Chiang et al., 2016). Interestingly, AN7893 (troA), which is indispensable for converting the dba cluster SMs to tropolones, was not upregulated during co-cultivation of A. nidulans with S. rapamycinicus.
Whereas, the before mentioned study proved that a physical interaction of the two microorganisms was necessary to induce SM production (Schroeckh et al., 2009), we showed that not the physical contact but the secretion of chemicals is used as signal. The advantage for A. nidulans is that chemicals can be detected over distances, diffusion being the limiting factor, which allows the fungus to counteract early with the production of antibacterial substances. Furthermore, the SM can have concentration-dependent functions, where it acts as a signal and toxin (Keller, 2019). This study shows that bleomycin and phleomycin have an antifungal effect as well as a signaling function by inducing the dba gene cluster. Furthermore, the tropolone-containing SM extract showed a concentration-dependent growth inhibition and iron-supplying effect for M. morganii (Figure 9). This information is important for understanding the physiological conditions of SM synthesis and their biological effect.
The discovery of new pharmaceutically or agriculturally relevant natural products is a challenging task since most of these metabolites are hidden by silent biosynthetic gene clusters. The here described co-cultivation of bleomycin-producing Streptomyces with A. nidulans uncovered the novel metabolite tripyrnidone (5) as well as the azaphilone azanidulone (4) and the antibacterial tropolones anhydrosepedonin (1) and antibiotic C (2). We described the biosynthesis pathway with the responsible involved enzymes and showed that the products have an iron-chelating as well as a growth inhibitory function on Streptomyces species. These findings revealed an inter-species communication or competition via chemical signals in form of the SMs bleomycin and tropolones, latter with potential for next-generation drug development.
The original contributions presented in the study are included in the article/Supplementary Material, further inquiries can be directed to the corresponding author/s.
JG, HB, FS, and GB conceived the study and designed the experiments. JG, AK, J-PW, VG, LS, and AH performed the experiments. JG, AK, J-PW, AH, WC, and FS analyzed the data. JG, AK, J-PW, FS, and GB wrote the manuscript. All authors contributed to the study and approved the final version of the manuscript.
Funding was provided by the German Research Council to GB (DFG grant BR 1502/19-1). We acknowledge support by the Open Access Publication Funds of the University of Göttingen.
The authors declare that the research was conducted in the absence of any commercial or financial relationships that could be construed as a potential conflict of interest.
All claims expressed in this article are solely those of the authors and do not necessarily represent those of their affiliated organizations, or those of the publisher, the editors and the reviewers. Any product that may be evaluated in this article, or claim that may be made by its manufacturer, is not guaranteed or endorsed by the publisher.
We thank Günther Winkelmann and Axel Zeeck for kindly providing Morganella and Streptomyces strains. We thank Marc Stadler for generous support, Christel Kakoschke for performing the NMR measurements and Wera Collisi for conducting bioassays.
The Supplementary Material for this article can be found online at: https://www.frontiersin.org/articles/10.3389/ffunb.2021.777474/full#supplementary-material
Ahmed, E., and Holmström, S. J. M. (2014). Siderophores in environmental research: roles and applications. Microb. Biotechnol. 7, 196–208. doi: 10.1111/1751-7915.12117
Ahuja, M., Chiang, Y. M., Chang, S. L., Praseuth, M. B., Entwistle, R., Sanchez, J. F., et al. (2012). Illuminating the diversity of aromatic polyketide synthases in Aspergillus nidulans. J. Am. Chem. Soc. 134, 8212–8221. doi: 10.1021/ja3016395
Albelda-Berenguer, M., Monachon, M., and Joseph, E. (2019). Siderophores: from natural roles to potential applications. Adv. Appl. Microbiol. 106, 193–225. doi: 10.1016/bs.aambs.2018.12.001
Altschul, S. F., Gish, W., Miller, W., Myers, E. W., and Lipman, D. J. (1990). Basic local alignment search tool. J. Mol. Biol. 215, 403–410. doi: 10.1016/S0022-2836(05)80360-2
Austin, B., Hall, R. M., and Tyler, B. M. (1990). Optimized vectors and selection for transformation of Neurospora crassa and Aspergillus nidulans to bleomycin and phleomycin resistance. Gene 93, 157–162. doi: 10.1016/0378-1119(90)90152-H
Azadbakht, M., Davoodi, A., Hosseinimehr, S. J., Keighobadi, M., Fakhar, M., Valadan, R., et al. (2020). Tropolone alkaloids from Colchicum kurdicum (Bornm.) Stef. (Colchicaceae) as the potent novel antileishmanial compounds; purification, structure elucidation, antileishmanial activities and molecular docking studies. Exp. Parasitol. 213:107902. doi: 10.1016/j.exppara.2020.107902
Basenko, E., Pulman, J., Shanmugasundram, A., Harb, O., Crouch, K., Starns, D., et al. (2018). FungiDB: an integrated bioinformatic resource for fungi and oomycetes. J. Fungi 4:39. doi: 10.3390/jof4010039
Bayram, Ö., Bayram, Ö. S., Ahmed, Y. L., Maruyama, J., Valerius, O., Rizzoli, S. O., et al. (2012). The Aspergillus nidulans MAPK Module AnSte11-Ste50-Ste7-Fus3 controls development and secondary metabolism. PLoS Genet. 8:e1002816. doi: 10.1371/journal.pgen.1002816
Becker, K., Pfütze, S., Kuhnert, E., Cox, R. J., Stadler, M., and Surup, F. (2021). Hybridorubrins A–D: azaphilone heterodimers from stromata of Hypoxylon fragiforme and insights into the biosynthetic machinery for azaphilone diversification. Chem. A Eur. J. 27, 1438–1450. doi: 10.1002/chem.202003215
Bentley, R.. (2008). A fresh look at natural tropolonoids. Nat. Prod. Rep. 25, 118–138. doi: 10.1039/B711474E
Bentley, S. D., Chater, K. F., Cerdeño-Tárraga, A.-M., Challis, G. L., Thomson, N. R., James, K. D., et al. (2002). Complete genome sequence of the model actinomycete Streptomyces coelicolor A3(2). Nature 417, 141–147. doi: 10.1038/417141a
Bills, G. F., and Gloer, J. B. (2016). Biologically active secondary metabolites from the fungi. Microbiol. Spectr. 4, 1087–1119. doi: 10.1128/microbiolspec.FUNK-0009-2016
Boer, W., Folman, L. B., Summerbell, R. C., and Boddy, L. (2005). Living in a fungal world: impact of fungi on soil bacterial niche development. FEMS Microbiol. Rev. 29, 795–811. doi: 10.1016/j.femsre.2004.11.005
Bohnert, M., Nützmann, H. W., Schroeckh, V., Horn, F., Dahse, H. M., Brakhage, A. A., et al. (2014). Cytotoxic and antifungal activities of melleolide antibiotics follow dissimilar structure-activity relationships. Phytochemistry 105, 101–108. doi: 10.1016/j.phytochem.2014.05.009
Brakhage, A. A., and Schroeckh, V. (2011). Fungal secondary metabolites - strategies to activate silent gene clusters. Fungal Genet. Biol. 48, 15–22. doi: 10.1016/j.fgb.2010.04.004
Braun, V., Pramanik, A., Gwinner, T., Köberle, M., and Bohn, E. (2009). Sideromycins: tools and antibiotics. BioMetals 22:3. doi: 10.1007/s10534-008-9199-7
Buchanan, M. S., Hashimoto, T., Yasuda, A., Takaoka, S., and Kan, Y. (1995). The structures of novel azaphilones, and aromatic compounds isolated from five ascomycetous fungi. Tennen Yuki Kagobutsu Toronkai Koen Yoshishu 37, 409–414.
Calvo, A. M., Wilson, R. A., Bok, J. W., and Keller, N. P. (2002). Relationship between secondary metabolism and fungal development. Microbiol. Mol. Biol. Rev. 66, 447–459. doi: 10.1128/MMBR.66.3.447-459.2002
Chater, K. F.. (2006). Streptomyces inside-out: a new perspective on the bacteria that provide us with antibiotics. Philos. Trans. R. Soc. B Biol. Sci. 361, 761–768. doi: 10.1098/rstb.2005.1758
Chater, K. F., Biró, S., Lee, K. J., Palmer, T., and Schrempf, H. (2010). The complex extracellular biology of Streptomyces. FEMS Microbiol. Rev. 34, 171–198. doi: 10.1111/j.1574-6976.2009.00206.x
Chen, A. Y., Adamek, R. N., Dick, B. L., Credille, C. V., Morrison, C. N., and Cohen, S. M. (2019). Targeting metalloenzymes for therapeutic intervention. Chem. Rev. 119, 1323–1455. doi: 10.1021/acs.chemrev.8b00201
Chiang, Y. M., Ahuja, M., Oakley, C. E., Entwistle, R., Asokan, A., Zutz, C., et al. (2016). Development of genetic dereplication strains in Aspergillus nidulans results in the discovery of aspercryptin. Angew. Chemie Int. Ed. 55, 1662–1665. doi: 10.1002/anie.201507097
Clark, R. C., Yeul Lee, S., and Boger, D. L. (2008). Total synthesis of chlorofusin, its seven chromophore diastereomers, and key partial structures. J. Am. Chem. Soc. 130, 12355–12369. doi: 10.1021/ja8012819
Coffey, R., and Ganz, T. (2017). Iron homeostasis: an anthropocentric perspective. J. Biol. Chem. 292, 12727–12734. doi: 10.1074/jbc.R117.781823
Cox, R. J., and Al-Fahad, A. (2013). Chemical mechanisms involved during the biosynthesis of tropolones. Curr. Opin. Chem. Biol. 17, 532–536. doi: 10.1016/j.cbpa.2013.06.029
Davison, J., Al Fahad, A., Cai, M., Song, Z., Yehia, S. Y., Lazarus, C. M., et al. (2012). Genetic, molecular, and biochemical basis of fungal tropolone biosynthesis. Proc. Natl. Acad. Sci. U. S. A. 109, 7642–7647. doi: 10.1073/pnas.1201469109
Drocourt, D., Calmels, T., Reynes, J. P., Baron, M., and Tiraby, G. (1990). Cassettes of the Streptoalloteichus hindustanus ble gene for transformation of lower and higher eukaryotes to phleomycin resistance. Nucleic Acids Res. 18:4009. doi: 10.1093/nar/18.13.4009
Duan, Y., Petzold, M., Saleem-Batcha, R., and Teufel, R. (2020). Bacterial tropone natural products and derivatives: overview of their biosynthesis, bioactivities, ecological role and biotechnological potential. ChemBioChem. 21, 2384–2407. doi: 10.1002/cbic.201900786
Eisendle, M., Schrettl, M., Kragl, C., Müller, D., Illmer, P., and Haas, H. (2006). The intracellular siderophore ferricrocin is involved in iron storage, oxidative-stress resistance, germination, and sexual development in Aspergillus nidulans. Eukaryot. Cell 5, 1596–1603. doi: 10.1128/EC.00057-06
Euzéby, J. P.. (1997). List of bacterial names with standing in nomenclature: a folder available on the internet. Int. J. Syst. Bacteriol. 47, 590–592. doi: 10.1099/00207713-47-2-590
Fischer, J., Müller, S. Y., Netzker, T., Jäger, N., Gacek-Matthews, A., Scherlach, K., et al. (2018). Chromatin mapping identifies BasR, a key regulator of bacteria-triggered production of fungal secondary metabolites. Elife 7:e40969. doi: 10.7554/eLife.40969
Galm, U., Wang, L., Wendt-Pienkowski, E., Yang, R., Liu, W., Tao, M., et al. (2008). In Vivo manipulation of the bleomycin biosynthetic gene cluster in Streptomyces verticillus ATCC15003 revealing new insights into its biosynthetic pathway. J. Biol. Chem. 283, 28236–28245. doi: 10.1074/jbc.M804971200
Gerke, J., Bayram, Ö., Feussner, K., Landesfeind, M., Shelest, E., Feussner, I., et al. (2012). Breaking the silence: protein stabilization uncovers silenced biosynthetic gene clusters in the fungus Aspergillus nidulans. Appl. Environ. Microbiol. 78, 8234–8244. doi: 10.1128/AEM.01808-12
Gerke, J., and Braus, G. H. (2014). Manipulation of fungal development as source of novel secondary metabolites for biotechnology. Appl. Microbiol. Biotechnol. 98, 8443–55. doi: 10.1007/s00253-014-5997-8
Gerke, J., Köhler, A. M., Meister, C., Thieme, K. G., Amoedo, H., and Braus, G. H. (2020). “Coordination of fungal secondary metabolism and development,” in Genetics and Biotechnology. The Mycota (A Comprehensive Treatise on Fungi as Experimental Systems for Basic and Applied Research), Vol. 2. eds J. P. Benz and K. Schipper (Cham: Springer), 173–205.
Granato, E. T., Meiller-Legrand, T. A., and Foster, K. R. (2019). The evolution and ecology of bacterial warfare. Curr. Biol. 29, R521–R537. doi: 10.1016/j.cub.2019.04.024
Grigoriev, I. V., Nikitin, R., Haridas, S., Kuo, A., Ohm, R., Otillar, R., et al. (2014). MycoCosm portal: gearing up for 1000 fungal genomes. Nucleic Acids Res. 42, D699–704. doi: 10.1093/nar/gkt1183
Guo, H., Roman, D., and Beemelmanns, C. (2019). Tropolone natural products. Nat. Prod. Rep. 36, 1137–1155. doi: 10.1039/C8NP00078F
Hartmann, T., Dümig, M., Jaber, B. M., Szewczyk, E., Olbermann, P., Morschhäuser, J., et al. (2010). Validation of a self-excising marker in the human pathogen Aspergillus fumigatus by employing the beta-rec/six site-specific recombination system. Appl. Environ. Microbiol. 76, 6313–6317. doi: 10.1128/AEM.00882-10
Hertzberg, R. P., Caranfa, M. J., and Hecht, S. M. (1985). DNA methylation diminishes bleomycin-mediated strand scission. Biochemistry 24, 5285–5289. doi: 10.1021/bi00341a001
Hill, T. W., and Kafer, E. (2001). Improved protocols for Aspergillus minimal medium: trace element and minimal medium salt stock solutions. Fungal Genet. Rep. 48, 20–21. doi: 10.4148/1941-4765.1173
Höfs, R., Walker, M., and Zeeck, A. (2000). Hexacyclinic acid, a polyketide from streptomyces with a novel carbon skeleton. Angew. Chem. 39, 3258–3261. doi: 10.1002/1521-3773(20000915)39:18<3258::AID-ANIE3258>3.0.CO;2-Q
Inglis, D. O., Binkley, J., Skrzypek, M. S., Arnaud, M. B., Cerqueira, G. C., Shah, P., et al. (2013). Comprehensive annotation of secondary metabolite biosynthetic genes and gene clusters of Aspergillus nidulans, A. fumigatus, A. niger and A. oryzae. BMC Microbiol. 13:91. doi: 10.1186/1471-2180-13-91
Inoue, H., Nojima, H., and Okayama, H. (1990). High efficiency transformation of Escherichia coli with plasmids. Gene 96, 23–28. doi: 10.1016/0378-1119(90)90336-P
Karlovsky, P.. (2008). “Secondary metabolites in soil ecology,” in Secondary Metabolites in Soil Ecology. Soil Biology, ed P. Karlovsky (Berlin, Heidelberg: Springer), 1–19.
Keller, N. P.. (2019). Fungal secondary metabolism: regulation, function and drug discovery. Nat. Rev. Microbiol. 17, 167–180. doi: 10.1038/s41579-018-0121-1
Kistler, H. C., and Broz, K. (2015). Cellular compartmentalization of secondary metabolism. Front. Microbiol. 6:68. doi: 10.3389/fmicb.2015.00068
Komaki, H., and Harayama, S. (2006). Sequence diversity of type-II polyketide synthase genes in streptomyces. Actinomycetologica 20, 42–48. doi: 10.3209/saj.20.42
König, C. C., Scherlach, K., Schroeckh, V., Horn, F., Nietzsche, S., Brakhage, A. A., et al. (2013). Bacterium induces cryptic meroterpenoid pathway in the pathogenic fungus Aspergillus fumigatus. ChemBioChem 14, 938–942. doi: 10.1002/cbic.201300070
Kraemer, S. M.. (2004). Iron oxide dissolution and solubility in the presence of siderophores. Aquat. Sci. Res. Across Boundaries 66, 3–18. doi: 10.1007/s00027-003-0690-5
Kross, J., Henner, W. D., Hecht, S. M., and Haseltine, W. A. (1982). Specificity of deoxyribonucleic acid cleavage by bleomycin, phleomycin, and tallysomycin. Biochemistry 21, 4310–4318. doi: 10.1021/bi00261a021
Linares, J. F., Gustafsson, I., Baquero, F., and Martinez, J. L. (2006). Antibiotics as intermicrobial signaling agents instead of weapons. Proc. Natl. Acad. Sci. 103, 19484–19489. doi: 10.1073/pnas.0608949103
Liu, L., Sasse, C., Dirnberger, B., Valerius, O., Fekete-Szücs, E., Harting, R., et al. (2021). Secondary metabolites of Hülle cells mediate protection of fungal reproductive and overwintering structures against fungivorous animals. Elife 10:e68058. doi: 10.7554/eLife.68058
Marsini, M. A., Gowin, K. M., and Pettus, T. R. R. (2006). Total synthesis of (±)-mitorubrinic acid. Org. Lett. 8, 3481–3483. doi: 10.1021/ol0610993
McDonald, K. D., Middleton, A. J., and Cole, D. S. (1983). Antibiotic. UK Patent Application GB 2 113 672 A. London: The Patent Office.
Misslinger, M., Hortschansky, P., Brakhage, A. A., and Haas, H. (2021). Fungal iron homeostasis with a focus on Aspergillus fumigatus. Biochim. Biophys. Acta Mol. Cell Res. 1868:118885. doi: 10.1016/j.bbamcr.2020.118885
Moore, C. W.. (1989). Cleavage of cellular and extracellular Saccharomyces cerevisiae DNA by bleomycin and phleomycin. Cancer Res. 49, 6935–6940.
Moore, C. W., McKoy, J., Del Valle, R., Armstrong, D., Bernard, E. M., Katz, N., et al. (2003). Fungal cell wall septation and cytokinesis are inhibited by bleomycins. Antimicrob. Agents Chemother. 47, 3281–3289. doi: 10.1128/AAC.47.10.3281-3289.2003
Morita, Y., Matsumura, E., Okabe, T., Fukui, T., Ohe, T., Ishida, N., et al. (2004). Biological activity of beta-dolabrin, gamma-thujaplicin, and 4-acetyltropolone, hinokitiol-related compounds. Biol. Pharm. Bull. 27, 1666–1669. doi: 10.1248/bpb.27.1666
Morita, Y., Matsumura, E., Okabe, T., Shibata, M., Sugiura, M., Ohe, T., et al. (2003). Biological activity of tropolone. Biol. Pharm. Bull. 26, 1487–1490. doi: 10.1248/bpb.26.1487
Nagao, K., Yoshida, N., Iwai, K., Sakai, T., Tanaka, M., and Miyahara, T. (2006). Production of sepedonin by Sepedonium chrysospermum NT-1 in submerged culture. Environ. Sci. 13, 251–6. doi: 10.1007/s00248-011-9923-7
Nahlik, K., Dumkow, M., Bayram, Ö., Helmstaedt, K., Busch, S., Valerius, O., et al. (2010). The COP9 signalosome mediates transcriptional and metabolic response to hormones, oxidative stress protection and cell wall rearrangement during fungal development. Mol. Microbiol. 78, 964–979. doi: 10.1111/j.1365-2958.2010.07384.x
Oiartzabal-Arano, E., Garzia, A., Gorostidi, A., Ugalde, U., Espeso, E. A., and Etxebeste, O. (2015). Beyond asexual development: modifications in the gene expression profile caused by the absence of the Aspergillus nidulans transcription factor FlbB. Genetics 199, 1127–1142. doi: 10.1534/genetics.115.174342
Ononye, S. N., VanHeyst, M. D., Oblak, E. Z., Zhou, W., Ammar, M., Anderson, A. C., et al. (2013). Tropolones as lead-like natural products: the development of potent and selective histone deacetylase inhibitors. ACS Med. Chem. Lett. 4, 757–761. doi: 10.1021/ml400158k
Punt, P. J., and van den Hondel, C. A. M. J. J. (1992). “[39] Transformation of filamentous fungi based on hygromycin b and phleomycin resistance markers,” in Methods in Enzymology, ed R. Wu (Cambridge, Massachusetts: Academic Press), 447–457.
Pyser, J. B., Baker Dockrey, S. A., Benítez, A. R., Joyce, L. A., Wiscons, R. A., Smith, J. L., et al. (2019). Stereodivergent, chemoenzymatic synthesis of azaphilone natural products. J. Am. Chem. Soc. 141, 18551–18559. doi: 10.1021/jacs.9b09385
Quang, D. N., Schmidt, J., Porzel, A., Wessjohann, L., Haid, M., and Arnold, N. (2010). Ampullosine, a new isoquinoline alkaloid from Sepedonium ampullosporum (Ascomycetes). Nat. Prod. Commun. 5, 869–872. doi: 10.1177/1934578X1000500609
Reyes-Fernández, E. Z., Shi, Y.-M., Grün, P., Bode, H. B., and Bölker, M. (2021). An unconventional melanin biosynthesis pathway in Ustilago maydis. Appl. Environ. Microbiol. 87, e01510–20. doi: 10.1128/AEM.01510-20
Riquelme, M., Aguirre, J., Bartnicki-García, S., Braus, G. H., Feldbrügge, M., Fleig, U., et al. (2018). Fungal morphogenesis, from the polarized growth of hyphae to complex reproduction and infection structures. Microbiol. Mol. Biol. Rev. 82, e00068–17. doi: 10.1128/MMBR.00068-17
Rokas, A., Mead, M. E., Steenwyk, J. L., Raja, H. A., and Oberlies, N. H. (2020). Biosynthetic gene clusters and the evolution of fungal chemodiversity. Nat. Prod. Rep. 37, 868–878. doi: 10.1039/C9NP00045C
Romsdahl, J., and Wang, C. C. C. (2019). Recent advances in the genome mining of Aspergillus secondary metabolites (covering 2012–2018). Medchemcomm 10, 840–866. doi: 10.1039/C9MD00054B
Scherlach, K., Graupner, K., and Hertweck, C. (2013). Molecular bacteria-fungi interactions: effects on environment, food, and medicine. Annu. Rev. Microbiol. 67, 375–397. doi: 10.1146/annurev-micro-092412-155702
Schroeckh, V., Scherlach, K., Nutzmann, H.-W., Shelest, E., Schmidt-Heck, W., Schuemann, J., et al. (2009). Intimate bacterial-fungal interaction triggers biosynthesis of archetypal polyketides in Aspergillus nidulans. Proc. Natl. Acad. Sci. 106, 14558–14563. doi: 10.1073/pnas.0901870106
Sharpton, T. J., Stajich, J. E., Rounsley, S. D., Gardner, M. J., Wortman, J. R., Jordar, V. S., et al. (2009). Comparative genomic analyses of the human fungal pathogens Coccidioides and their relatives. Genome Res. 19, 1722–1731. doi: 10.1101/gr.087551.108
Shen, B., Du, L., Sanchez, C., Chen, M., and Edwards, D. J. (1999). “Bleomycin biosynthesis in Streptomyces verticillus ATCC15003: a model of hybrid peptide and polyketide biosynthesis,” in Bioorganic Chemistry, 27:155–171. doi: 10.1006/bioo.1998.1131
Sievers, F., Wilm, A., Dineen, D., Gibson, T. J., Karplus, K., Li, W., et al. (2011). Fast, scalable generation of high-quality protein multiple sequence alignments using Clustal Omega. Mol. Syst. Biol. 7:539. doi: 10.1038/msb.2011.75
Supka, R.. (1981). Sepedonin und Anhydrospedonin - Zwei Tropolon-Antibiotika aus Sepedonium chrysospermum Bulliard Ex Fries. (PhD thesis). Tübingen, Germany, Eberhard-Karls-Universität Tübingen.
Tavis, J. E., and Lomonosova, E. (2015). The hepatitis B virus ribonuclease H as a drug target. Antiviral Res. 118, 132–138. doi: 10.1016/j.antiviral.2015.04.002
Thieken, A., and Winkelmann, G. (1993). A novel bioassay for the detection of siderophores containing keto-hydroxy bidentate ligands. FEMS Microbiol. Lett. 111, 281–285. doi: 10.1111/j.1574-6968.1993.tb06399.x
Trust, T. J.. (1975). Antibacterial activity of tropolone. Antimicrob. Agents Chemother. 7, 500–506. doi: 10.1128/AAC.7.5.500
Ueda, K., Hiasa, H., Takebe, S., Sakai, H., and Komano, T. (1992). Alternative secondary structures in the phage G4 origin of the complementary DNA strand synthesis: effects of NaCl concentration on the bleomycin-DNA interaction. Biosci. Biotechnol. Biochem. 56, 394–398. doi: 10.1271/bbb.56.394
Watve, M. G., Tickoo, R., Jog, M. M., and Bhole, B. D. (2001). How many antibiotics are produced by the genus Streptomyces? Arch. Microbiol. 176, 386–390. doi: 10.1007/s002030100345
Wright, J. L. C., McInnes, A. G., Smith, D. G., and Vining, L. C. (1970). Structure of sepedonin, a tropolone metabolite of Sepedonium chrysospermum Fries. Can. J. Chem. 48, 2702–2708. doi: 10.1139/v70-456
Wu, C., Zacchetti, B., Ram, A. F. J., van Wezel, G. P., Claessen, D., and Hae Choi, Y. (2015). Expanding the chemical space for natural products by Aspergillus-Streptomyces co-cultivation and biotransformation. Sci. Rep. 5:10868. doi: 10.1038/srep10868
Yu, L., Ding, W., and Ma, Z. (2016). Induced production of cytochalasans in co-culture of marine fungus Aspergillus flavipes and actinomycete Streptomyces sp. Nat. Prod. Res. 30, 1718–1723. doi: 10.1080/14786419.2015.1136910
Zhang, S. P., Huang, R., Li, F. F., Wei, H. X., Fang, X. W., Xie, X. S., et al. (2016). Antiviral anthraquinones and azaphilones produced by an endophytic fungus Nigrospora sp. from Aconitum carmichaeli. Fitoterapia 112, 85–89. doi: 10.1016/j.fitote.2016.05.013
Keywords: Aspergillus nidulans, Streptomyces, glycopeptide antibiotics, structure elucidation, tropolones, fungal-bacterial co-cultivation
Citation: Gerke J, Köhler AM, Wennrich J-P, Große V, Shao L, Heinrich AK, Bode HB, Chen W, Surup F and Braus GH (2022) Biosynthesis of Antibacterial Iron-Chelating Tropolones in Aspergillus nidulans as Response to Glycopeptide-Producing Streptomycetes. Front. Fungal Biol. 2:777474. doi: 10.3389/ffunb.2021.777474
Received: 15 September 2021; Accepted: 06 December 2021;
Published: 03 January 2022.
Edited by:
Philipp Wiemann, Solugen, Inc., United StatesReviewed by:
Vito Valiante, Leibniz Institute for Natural Product Research and Infection Biology, GermanyCopyright © 2022 Gerke, Köhler, Wennrich, Große, Shao, Heinrich, Bode, Chen, Surup and Braus. This is an open-access article distributed under the terms of the Creative Commons Attribution License (CC BY). The use, distribution or reproduction in other forums is permitted, provided the original author(s) and the copyright owner(s) are credited and that the original publication in this journal is cited, in accordance with accepted academic practice. No use, distribution or reproduction is permitted which does not comply with these terms.
*Correspondence: Gerhard H. Braus, Z2JyYXVzQGd3ZGcuZGU=; Frank Surup, ZnJhbmsuc3VydXBAaGVsbWhvbHR6LWh6aS5kZQ==
Disclaimer: All claims expressed in this article are solely those of the authors and do not necessarily represent those of their affiliated organizations, or those of the publisher, the editors and the reviewers. Any product that may be evaluated in this article or claim that may be made by its manufacturer is not guaranteed or endorsed by the publisher.
Research integrity at Frontiers
Learn more about the work of our research integrity team to safeguard the quality of each article we publish.