- 1School of Plant Sciences, University of Arizona, Tucson, AZ, United States
- 2International Institute of Tropical Agriculture (IITA), Lilongwe, Malawi
- 3International Institute of Tropical Agriculture (IITA), Ibadan, Nigeria
- 4United States Department of Agriculture - Agriculture Research Service, Tucson, AZ, United States
- 5College of Food Science and Engineering, Ocean University of China, Qingdao, China
Fungal species within Aspergillus section Flavi contaminate food and feed with aflatoxins. These toxic fungal metabolites compromise human and animal health and disrupt trade. Genotypically and phenotypically diverse species co-infect crops, but temporal and spatial variation in frequencies of different lineages suggests that environmental factors such as temperature may influence structure of aflatoxin-producing fungal communities. Furthermore, though most species within Aspergillus section Flavi produce sclerotia, divergent sclerotial morphologies (small or S-type sclerotia vs. large or L-type sclerotia) and differences in types and quantities of aflatoxins produced suggest lineages are adapted to different life strategies. Temperature is a key parameter influencing pre- and post-harvest aflatoxin contamination of crops. We tested the hypothesis that species of aflatoxin-producing fungi that differ in sclerotial morphology will vary in competitive ability and that outcomes of competition and aflatoxin production will be modulated by temperature. Paired competition experiments between highly aflatoxigenic S-type species (A. aflatoxiformans and Lethal Aflatoxicosis Fungus) and L-type species (A. flavus L morphotype and A. parasiticus) were conducted on maize kernels at 25 and 30°C. Proportions of each isolate growing within and sporulating on kernels were measured using quantitative pyrosequencing. At 30°C, S-type fungi were more effective at host colonization compared to L-type isolates. Total aflatoxins and the proportion of B vs. G aflatoxins were greater at 30°C compared to 25°C. Sporulation by L-type isolates was reduced during competition with S-type fungi at 30°C, while relative quantities of conidia produced by S-type species either increased or did not change during competition. Results indicate that both species interactions and temperature can shape population structure of Aspergillus section Flavi, with warmer temperatures favoring growth and dispersal of highly toxigenic species with S-type sclerotia.
Introduction
Aflatoxins are carcinogenic secondary metabolites produced by several species within Aspergillus section Flavi. Aflatoxins contaminate food and feed worldwide and are a health concern, causing liver cancer, stunting of growth, and immune suppression at lower concentrations and rapid death after very high exposure (Gong et al., 2004; Williams et al., 2004; Liu and Wu, 2010). Aflatoxins are also an economic burden to farmers, traders, and nations due to loss of revenue as a result of strict regulations that limit sale of contaminated crops (Mitchell et al., 2016). It is estimated that strict regulations of aflatoxins by the European Union cost African maize exporters over $670 million annually (Wu, 2015). Though aflatoxin contamination is a perennial problem in some regions, the frequency and severity of aflatoxin contamination events is highly variable and dependent on factors including compositions of fungal communities associated with crops and environmental conditions such as temperature (Cotty et al., 2008; Probst et al., 2010).
Aflatoxin-producing fungi within Aspergillus section Flavi are phenotypically and genotypically diverse with large inter- and intra-specific variation in quantities of asexual spores (conidia) produced, size and quantities of sclerotia produced, and types and quantities of aflatoxins produced in crops (Cotty et al., 1994; Mehl and Cotty, 2010; Frisvad et al., 2019). Aspergillus section Flavi, species produce sclerotia that are either large (L-type > 400 μm) or small (S-type <400 μm). Most lineages within Aspergillus section Flavi demonstrate a single sclerotial type, while A. flavus, the most common causal agent of aflatoxin contamination, includes both S and L sclerotial types (Cotty, 1989). There are several phylogenetically distinct species within Aspergillus section Flavi, some of which were previously misidentified as A. flavus S-type, that have the S-type morphology. These species include A. texensis (Singh et al., 2018), A. toxicus (Singh et al., 2020), A. agricola (Singh et al., 2020), A. aflatoxiformans (Cotty and Cardwell, 1999; Frisvad et al., 2019), and the unnamed Lethal Aflatoxicosis Fungus (LAF), a species closely related to A. minisclerotigenes and associated with multiple deaths in Kenya in 2004 (Probst et al., 2007, 2012; Frisvad et al., 2019). Aspergillus parasiticus is another primary causal agent of aflatoxin contamination (Horn, 2003; Klich, 2007), and though it can be distinguished morphologically from A. flavus based on colony color and conidial ornamentation, it produces L-type sclerotia (Frisvad et al., 2019). On certain culture media, S-type species tend to produce fewer conidia and abundant sclerotia whereas L-type species produce copious amounts of conidia and few sclerotia (Cotty, 1989).
In addition to variation in morphological characteristics, species within Aspergillus section Flavi also vary in the types and quantities of aflatoxins produced. Aspergillus parasiticus and A. aflatoxiformans produce both B and G aflatoxins, while A. flavus and LAF produce only B aflatoxins. Among the four types of aflatoxins, aflatoxin B1 is the most potent carcinogen followed by aflatoxins G1, B2 and G2 respectively (Hernandez-Martinez and Navarro-Blasco, 2010; Liu and Wu, 2010). Furthermore, the S-type fungi and A. parasiticus consistently produce high levels of aflatoxins, while aflatoxin production by A. flavus L-type is highly variable (Cotty, 1989; Probst et al., 2007). Interactions among these species that differ in morphology and aflatoxin production have important implications for the etiology of crop aflatoxin contamination in regions where these species co-occur (Nesci and Etcheverry, 2002; Barros et al., 2005; Giorni et al., 2007; Atehnkeng et al., 2008a; Donner et al., 2009; Probst et al., 2010; Diedhiou et al., 2011; Kachapulula et al., 2017; Agbetiameh et al., 2018; Singh and Cotty, 2019; Sserumaga et al., 2020). In some parts of sub-Saharan Africa, A. flavus L-type, A. parasiticus, A. aflatoxiformans and LAF are the most frequently co-occurring species. Temporal and spatial co-occurrence of Aspergillus species can lead to interspecific competition for nutrients, space, and other limiting resources, with potential impacts on the quantity and type of aflatoxins produced in crops.
Some studies suggest differential niche adaptation within lineages of Aspergillus section Flavi. For example, based on morphological and genomic features it can be inferred that S-type and L-type species vary in their life strategies in the environment and during crop colonization (Cotty et al., 1994; Ohkura et al., 2018). Abundant sporulation by L-type fungi may provide a dispersal advantage in the phyllosphere, allowing for exploitation of new nutrient environments or hosts, while allocating more resources to production of mycelia may confer an advantage in invading host tissues and acquiring nutrients (Cotty et al., 1994; Mehl and Cotty, 2010; Mehl et al., 2012). Abundant production of sclerotia may be advantageous for survival in the soil environment (Garber and Cotty, 1997; Mehl et al., 2012; Ohkura et al., 2018) where microbial competition is high. This contrasts with the phyllosphere where microbial species diversity is lower (Lindow and Brandl, 2003; Delmotte et al., 2009) and interacting fungi may use different strategies to compete. Some genotypes of A. flavus are highly competitive during host colonization, while others are less competitive in terms of invasion and nutrient acquisition but outcompete other fungi through greater dispersal of conidia (Mehl and Cotty, 2010; Sweany et al., 2011). While intraspecific interactions among A. flavus genotypes in different nutrient environments and hosts has been studied (Mehl and Cotty, 2013a,b), little is known about interspecific interactions between different Aspergillus section Flavi species.
In addition to adaptation to hosts or nutrient environments, there is also evidence that species in Aspergillus section Flavi may be adapted to different abiotic conditions such as temperature. For example, A. parasiticus colonizes crops at lower temperatures than A. flavus and grows faster than A. flavus in culture media at temperatures between 22 and 25°C; however, it grows slower than A. flavus at temperatures above 30°C (Pitt and Miscamble, 1995; Horn, 2005). The S-type fungi are more frequently reported (up to 80%), in the tropics, subtropics and desert environments where average temperatures are high (>25oC), suggesting that the S-type fungi are adapted to these environments (Cardwell and Cotty, 2002; Pildain et al., 2004, 2008; Donner et al., 2009; Perrone et al., 2014). Temperature also plays a crucial role in sporulation and aflatoxin production. Higher temperatures favor growth, sporulation, and dispersal of A. flavus (Payne et al., 1985; Diener et al., 1987; Scheidegger and Payne, 2003; Jaime-Garcia and Cotty, 2010). At temperatures below 20°C, Aspergillus section Flavi species occur in low frequencies while at temperatures >25°C, aflatoxin-producing fungi are common throughout the soil, the air, and on crop surfaces (Manabe et al., 1978; Shearer et al., 1992; Cotty and Jaime-Garcia, 2007). High levels of aflatoxin production have been observed between 25 and 35°C (Schmidt-Heydt et al., 2009), with aflatoxin production by A. flavus often being maximal at 30°C (Bhatnagar et al., 2006; O'Brian et al., 2007).
Since genetically and phenotypically diverse species of Aspergillus section Flavi coexist in agricultural environments, interspecies competition is likely to impact the composition and aflatoxin-producing potential of crop-associated fungal communities. Abiotic factors including temperature will likely influence both competition among species (Bock et al., 2004; Hiscox et al., 2016) and the extent to which aflatoxin biosynthesis occurs (Singh et al., 2020). We test the hypothesis that temperature will impact both competition between L-type and S-type species of Aspergillus section Flavi and the dynamics of aflatoxin production. The objectives of the current study were to: (1) quantify outcomes of competition between Aspergillus species differing in sclerotial type and aflatoxin production (A. flavus, A. parasiticus, A. aflatoxiformans, and LAF) and (2) evaluate the impacts of temperature on interspecific interactions and aflatoxin production during colonization of maize kernels.
Materials and Methods
Fungal Isolates
Four highly aflatoxigenic isolates were used in this study: two L-type, A. flavus (AF13) and A. parasiticus (AP2999), and two S-type, A. aflatoxiformans (BN008-R) and LAF (K0550K). Information for these isolates including the types of aflatoxins they produce are listed in Table 1. Cultures started from single conidia were cultivated on 5/2 agar (5% V8 juice, 2% agar, pH 6.0) (Cotty, 1989), and plugs from growing colonies were transferred into 4 ml of sterile water for storage. Conidial suspensions were prepared from 7-day-old cultures grown on 5/2 agar as described previously (Mehl and Cotty, 2013a). Concentrations of conidia were quantified using a turbidity meter (Turbidimeter TB 300IR; Orbeco Analytical Systems, Farmingdale, NY), and conidia per ml were calculated with a nephelometric turbidity unit (NTU) vs. CFU standard curve (conidia per ml = 49,937 x NTU) (Bock and Cotty, 1999; Probst et al., 2010).
Competition Experiments
Competition experiments were conducted on mature maize kernels (Pioneer hybrid N82VGT) that were sterilized by autoclaving (121°C, 20 min) (Probst and Cotty, 2012). Maize kernel inoculations were conducted as described previously (Mehl and Cotty, 2013a) with some modifications. Maize kernel moisture content was measured using a moisture analyzer (HB43 Halogen Moisture Analyzer, Mettler-Toledo), and the final moisture content of the maize was adjusted to 30% with either sterile water or sterile water plus the inoculum. The four isolates were inoculated singly or paired in all possible combinations, resulting in four single isolate treatments and six co-inoculation treatments. In the treatments, 5 g sterile maize in 250-ml Erlenmeyer flasks sealed with gas-permeable BugStopper plugs were inoculated with either 5 × 104 conidia of one isolate or with suspensions that contained 5 × 104 conidia of each isolate (105 conidia total) for co-inoculation treatments.
A total of eight flasks per treatment were prepared: four for aflatoxin analyses and four for conidia quantification and DNA analyses. Eight flasks of uninoculated maize were included as controls. The treatment and control flasks were incubated for seven days in the dark at either 25 or 30°C, temperatures that are common in agricultural environments where maize is grown and where Aspergillus species and aflatoxin contamination are common. At the end of the incubation period, maize kernels were washed with 20 ml 0.01% Tween-80 followed by 20 ml of distilled water to recover conidia. Washings were sieved using Miracloth (EMD Millipore, Billerica, MA) to separate sclerotia from the conidial suspension, transferred into 50 ml conical tubes, and measured for turbidity. The quantities of conidia were calculated from turbidity as described above.
Following centrifugation of the conidial suspensions (4400 g, 5 min), DNA was extracted from the conidial pellet using a previously described protocol (Callicott and Cotty, 2015). Washed kernels were immediately dried at 60oC for 48 h and then ground for 15 s in an analytical mill (IKA Works, Wilmington, NC) for DNA extraction representing the colonizing mycelial DNA. Colonizing mycelial DNA was extracted from 200 mg of ground maize kernels by modifying the method described for conidial DNA extraction. In short, 800 μl lysis buffer was added to 200 mg of ground kernels as starting material, and all subsequent steps were followed as described previously (Callicott and Cotty, 2015).
Pyrosequencing Assays
Sequences were obtained from GenBank for the nitrate reductase (niaD), aflatoxin transcription factor (aflR) and calmodulin (cmdA) genes of A. flavus (AF13 GenBank accessions MH760530, MH752568, and MK119698), A. parasiticus (AP2999 GenBank accessions MH76053, KT829482, and MK119703) and A. aflatoxiformans (BN008-R GenBank accessions MK119681, AF441441, and MK119715). The sequences for aflR, cmdA, and niaD of the LAF isolate (K0550K GenBank accessions MZ673642, MZ673640 and MZ673641) were determined using PCR amplification and bidirectional sequencing using the primers and conditions described previously (Probst et al., 2012; Singh and Cotty, 2019). Sequences were aligned using MUSCLE (Edgar, 2004) within Geneious Pro Version 7.1.9 (Biomatters Ltd, Auckland, New Zealand). Putative single nucleotide polymorphisms (SNPs) were identified visually from the alignment and were confirmed by pyrosequencing. Species-specific pyrosequencing assays based on these SNPs were designed using PyroMark Assay Design software v2.0.1.15 (Qiagen, Germantown, MD). PCR primer pairs 5′-Biotin-GGTTTTGGATCTGACCAGTGTAG-3′ / 5′-GGCAGATAGTACCCGGCTTG-3′ and a sequencing primer 5′-TAGTACCCGGCTTGC-3′ targeted an A. flavus-specific SNP in the aflR gene. PCR primer pairs 5′-Biotin-CGGGCTGGCCATTTATTATGAT-3′ / 5′-GGGAAGACGGGCGTTGTTTA-3′ and sequencing primer 5′-GGAACCGACCCGACT-3′ targeted an A. aflatoxiformans-specific SNP in the cmdA gene region. PCR primer pairs 5′-Biotin-GGCTGAAGAGGCTGATCTTGAC-3′ / 5′-CGCGGTTGTCATTGATATGGTA-3′ and sequencing primer 5′-GTCATTGATATGGTACCAG-3' targeted an A. parasiticus-specific SNP in the niaD gene region. These three sets of primers distinguished between LAF and the species being targeted by the pyrosequencing assay. PCR reactions were performed in Bioneer AccuPower Hotstart PCR PreMix tubes (Bioneer, Inc., Alameda, CA). For each primer pair, one primer was 5′ biotinylated and HPLC-purified. The reactions were performed in 20 μl and included 0.25 μM each primer and 10 ng genomic DNA. PCR conditions for all the assays were: DNA denaturation at 94°C for 5 min, followed by 38 cycles of denaturation at 94°C for 20 s, primer annealing at 62°C for 30 s, extension at 72°C for 30 s, and a final extension step at 72°C for 5 min. Amplicons were visualized with GelRed on 1.0% agarose gels before pyrosequencing to confirm amplification and correct amplicon size for each assay. Proportions of species-specific SNPs in pools of amplicons were quantified by pyrosequencing using a PyroMark Q48 Autoprep Pyrosequencer (Qiagen, Germantown MD), following the manufacturer's instructions. Percentages of DNA from each isolate were deduced from the proportions of species-specific SNPs calculated using PyroMark Q48 Autoprep software v.2.4.2. No amplicons were detected from uninoculated control maize.
Aflatoxin Extraction and Quantification
Aflatoxins were extracted from maize kernels following inoculation and incubation by adding 50 ml of 70% methanol to each flask and homogenizing the kernels/methanol using a laboratory grade blender (Waring Laboratory, Torrington, CT, USA) at full speed for 30 s. Extracts were separated by thin layer chromatography (TLC) (Silica gel 60 plates, EMD, Darmstadt, Germany) in ethyl ether-methanol-water (96:3:1) along with an aflatoxin standard (Aflatoxin Mix Kit-M, Supelco, Bellefonte, PA, USA). Aflatoxins on TLC plates were visualized using 365-nm UV light and quantified directly with a scanning densitometer (TLC Scanner 3; Camag Scientific Inc, Wilmington, NC, USA) (Pons et al., 1969). Peaks generated by a scanning densitometer were used to estimate aflatoxin concentrations by comparing the area under the peak generated by sample to the area under the peak generated by a standard. There were no detectable aflatoxins in uninoculated control flasks. The limit of quantification was 10 μg/Kg.
Experimental Design and Data Analysis
The experiment was a randomized factorial design (ten inoculation treatments by two temperatures) with four replicates (one flask per replicate). Data were analyzed in JMP 11.1.1 (SAS Institute, Cary, NC, USA, 2013). Conidial quantities and aflatoxin data were log-transformed, and isolate percentages were arcsine transformed before analysis. Percentages of coinfecting isolates were compared using Student's t-test. The influence of temperature and inoculation treatment on sporulation and aflatoxin production was analyzed using a factorial analysis of variance. Mean separation was done using Tukey's HSD. Expected percentages of conidia for each isolate were calculated based on quantities of conidia produced from individual inoculations using the formula:
where Xe= expected percent isolate, Xc = quantities of conidia produced by isolate “X” grown individually, and Yc = quantities of conidia produced by isolate “Y” grown individually.
Conidia produced by each species during competition were calculated by multiplying the total number of conidia per gram host substrate by the proportion of each isolate in the conidia DNA using the formula:
where M = calculated isolate M conidia, C = total conidia, K = proportion of isolate M from conidia DNA.
Proportion of aflatoxins comprised of B aflatoxins was calculated using the formula:
where %B = proportion of aflatoxins comprised of B aflatoxins, TB = total B aflatoxins, TG = total G aflatoxins. Expected total aflatoxin production by co-inoculated isolates was calculated using the formula:
where Te = expected total aflatoxin, Tm = aflatoxin produced by isolate “M” grown individually, Tn = aflatoxin produced by isolate “N” grown individually, %Mm = % isolate “M” in mycelia, %Nm = % isolate “N” in mycelia.
The expected and observed aflatoxins or expected and measured conidial percentages were compared using paired t-test. Significant differences are reported at α = 0.05. Non-transformed means are reported for clarity.
Results
Influence of Temperature on Interactions Among Species During Maize Kernel Colonization
When four different Aspergillus species were co-inoculated on maize kernels, relative colonization of the species was temperature and isolate dependent, as measured by the proportion of isolate-specific mycelial DNA in the kernels (Figure 1). The proportion of A. flavus colonizing kernels was not influenced by temperature (P = 0.5299) or the identity of the co-infecting isolate (P = 0.3901), but there was a significant temperature by co-inoculated isolate interaction (P = 0.0246). For example, proportions of A. flavus and A. parasiticus were equal at 25°C, but the proportion of A. flavus was significantly greater than A. parasiticus at 30°C (Figure 1). In contrast, the proportion of A. flavus was greater than A. aflatoxiformans at 25°C, but the two isolates were equal at 30°C. When co-inoculated with other isolates, the proportion of A. parasiticus was significantly greater at 25°C compared to 30°C (P < 0.0001), but proportions of A. parasiticus DNA were not influenced by the co-infecting isolate (P = 0.4015) or the interaction between temperature and co-infecting isolate (P = 0.1917). When co-inoculated with other isolates, the proportion of LAF was influenced by the main factors of co-inoculated isolate (P = 0.0409) and temperature (P < 0.0001) but there were no interactions among factors (P = 0.0772). Proportions of LAF DNA in kernels, indicating colonization, were high at 30°C compared to 25°C, and overall, the proportion of LAF was greater than the two L-type fungi (A. flavus and A. parasiticus) but was equal to the other S-type isolate (A. aflatoxiformans). Overall, the proportions of A. aflatoxiformans DNA in kernels did not differ between 25 and 30°C, but proportions were influenced by the co-inoculated isolate (P = 0.0013) and the interaction between co-inoculated isolate and temperature (P = 0.0005). When co-inoculated with A. parasiticus or A. flavus, proportions of A. aflatoxiformans were greater at 30°C compared to 25°C. However, when co-inoculated with LAF, proportions of A. aflatoxiformans DNA in kernels were lower at 30°C compared to 25°C (Figure 1).
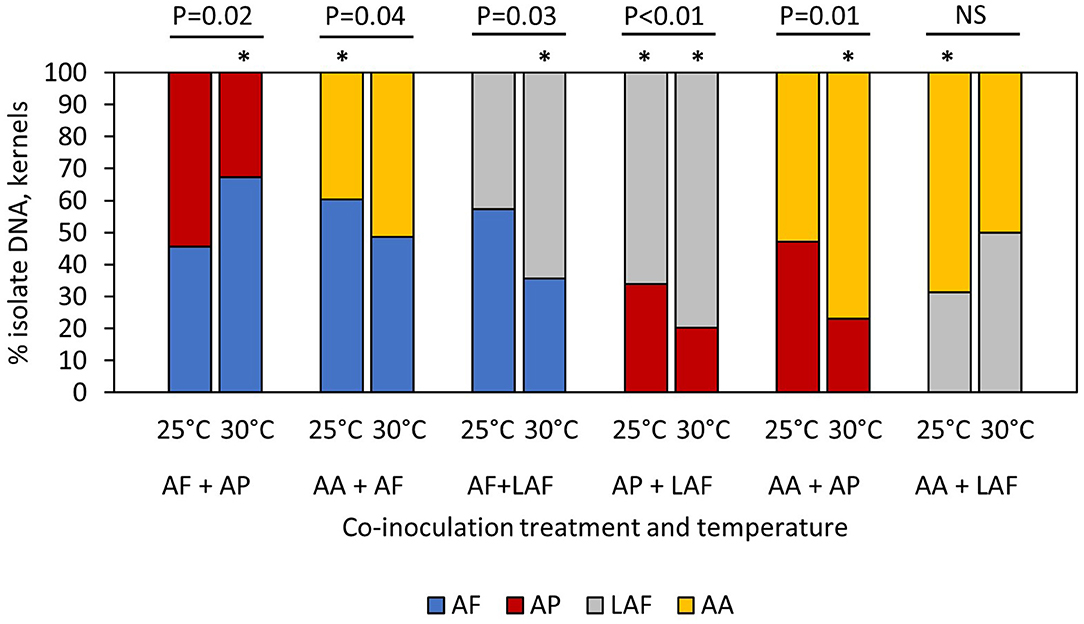
Figure 1. Influence of temperature on competition between co-inoculated Aspergillus isolates during maize kernel colonization. AF, A. flavus L strain (AF13) (L-type); AP, A. parasiticus (AP2999) (L-type); AA, A. aflatoxiformans (BN008-R) (S-type); LAF, Lethal Aflatoxicosis Fungus (K0550K) (S-type). Asterisks above individual bars indicate treatments with percentages that are significantly different than 50% (t-test, P < 0.05). P values above the groups of paired bars indicate outcomes of competition were significantly different at 25 and 30°C (t-test, P < 0.05). NS indicate not significant.
Influence of Temperature and Co-inoculation on Aflatoxin Production
When aflatoxin production was measured following co-inoculation of the four Aspergillus species on maize, total aflatoxin production varied by both inoculation treatment (P < 0.0001) and temperature (P < 0.0001), and there was an interaction between the two factors (P < 0.0001). Individual and paired isolates produced similar quantities of total aflatoxins at 25°C. Except for A. flavus alone or when it was co-inoculated with LAF, greater concentrations of total aflatoxins were produced at 30°C compared to 25°C (Table 2). Among the individually inoculated isolates, A. parasiticus produced the greatest concentrations of aflatoxins and A. flavus produced the least at 30°C. For maize co-inoculated with either A. flavus and A. parasiticus or A. aflatoxiformans and LAF, aflatoxin concentrations at 30°C were similar to what would be expected based on the aflatoxin-producing potential of individual isolates and the proportions of each isolate colonizing the kernels. However, measured aflatoxin concentrations were greater than expected in kernels co-inoculated with A. aflatoxiformans and either A. flavus (354 vs. 184 μg/g; P = 0.0007) or A. parasiticus (455 vs. 311 μg/g; P = 0.0394). In contrast, measured aflatoxin concentrations were less than expected for kernels co-inoculated with LAF and either A. flavus (60 vs. 133 μg/g; P < 0.0001) or A. parasiticus (175 vs. 235 μg/g; P = 0.0262).
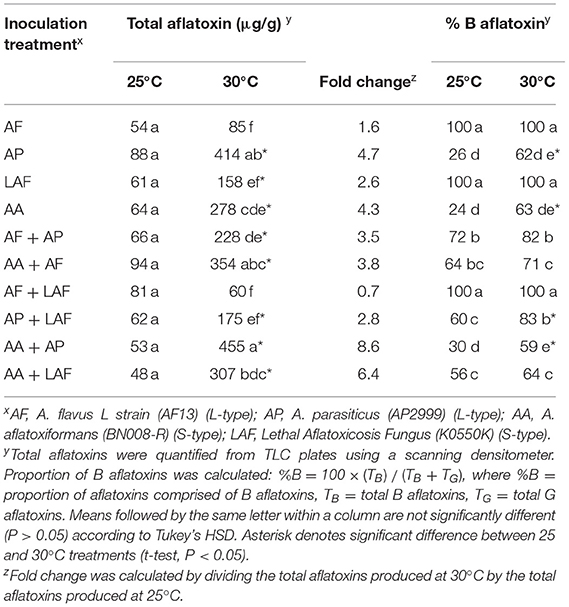
Table 2. Effect of temperature and co-inoculation of Aspergillus isolates on aflatoxin production on maize kernels at 25 and 30°C.
Two of the isolates, A. parasiticus and A. aflatoxiformans, produced both B and G aflatoxins, and the proportion of total aflatoxins comprised of B aflatoxin varied by inoculation treatment (P < 0.0001), temperature (P < 0.0001), and the interaction of the two factors (P < 0.0001). At 25°C, B aflatoxins comprised 30% or less of the total aflatoxins produced by A. parasiticus and A. aflatoxiformans grown individually or together, but at 30°C, over 50% of aflatoxins produced were B aflatoxins (Table 2).
Influence of Temperature on Interactions Among Species Sporulating on Maize Kernels
Similar to the results for colonization, the interaction between temperature and co-inoculated isolate influenced the proportions of different species sporulating on maize kernels (P < 0.0001, Table 3). In general, proportions of isolates colonizing and sporulating on kernels were similar. However, the proportion of A. flavus was greater during sporulation compared to colonization when co-inoculated with either A. parasiticus at 25°C (P = 0.0034) or A. aflatoxiformans at 30°C (P = 0.0010). Comparisons between expected proportions of conidia produced by each isolate during co-infection (calculated based on sporulation of individually inoculated isolates) and measured proportions are summarized in Table 3. The proportion of A. flavus was greater than expected when co-inoculated with A. parasiticus at both temperatures (25°C: P = 0.0493; 30°C; P = 0.0023). However, the proportion of A. flavus was less than expected when co-inoculated with A. aflatoxiformans at 25°C (P = 0.0009) and 30°C (P = 0.0213) and with LAF at 30°C (P = 0.0082). Proportions of A. parasiticus were less than expected when co-inoculated with A. flavus (P = 0.0071) and A. aflatoxiformans (P = 0.0026) at 25°C and when co-inoculated with any other species at 30°C (Table 3). In contrast, the proportion of A. aflatoxiformans was greater than expected (P < 0.0001) at 25°C when co-inoculated with LAF. However, the proportions of the two species were equal (P = 0.0529) at 30°C (Table 3).
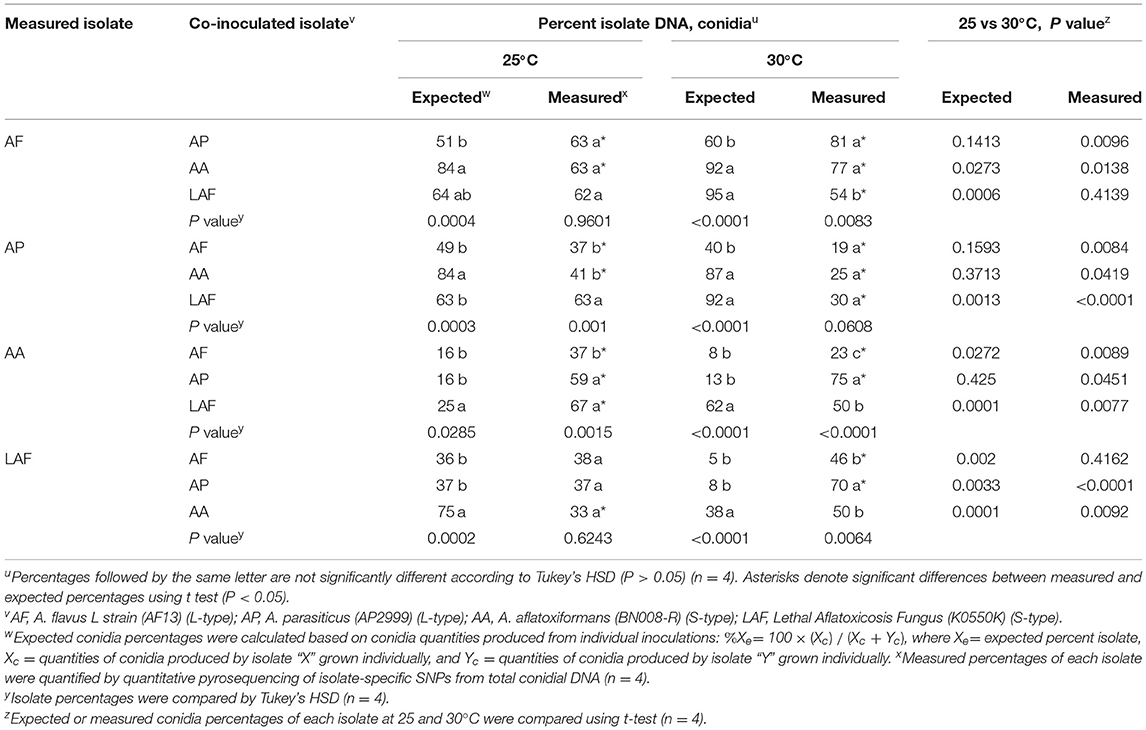
Table 3. Influence of temperature on predicted and measured conidia percentages from paired co-inoculation of Aspergillus isolates at 25 and 30°C.
Quantities of conidia produced by each isolate growing individually or in competition with another isolate on maize kernels are summarized in Figure 2. Except for LAF, sporulation by the individually inoculated isolates was greater at 30°C than 25°C. The impact of co-inoculation on sporulation varied by isolate and temperature. Sporulation by A. flavus, A. parasiticus, and LAF was influenced by co-inoculated isolate (P < 0.0001, P < 0.0001, and P < 0.0001 respectively), temperature (P < 0.0001, P < 0.0001, and P < 0.0001, respectively) and the interaction of the two factors (P = 0.0372, P < 0.0001, and P < 0.0001, respectively). In contrast, sporulation by A. aflatoxiformans was dependent on temperature (P < 0.0001) and the interaction between temperature and co-inoculated isolate (P < 0.0001) but not by the identity of the co-inoculated isolate (P = 0.0735). Co-inoculation suppressed sporulation (P < 0.05) by A. flavus (Figure 2A) and A. parasiticus (Figure 2B) at both 25°C and 30°C. However, the effects of co-inoculation on sporulation by the S morphology species, A. aflatoxiformans and LAF, was more variable. Co-inoculation reduced sporulation by LAF at 25°C, but at 30°C LAF produced more conidia during co-inoculation with A. flavus and A. parasiticus compared to when it grew alone (Figure 2C). Sporulation by A. aflatoxiformans was the least affected during co-inoculation at both temperatures, with reductions only occurring at 30°C during co-inoculation with LAF (Figure 2D).
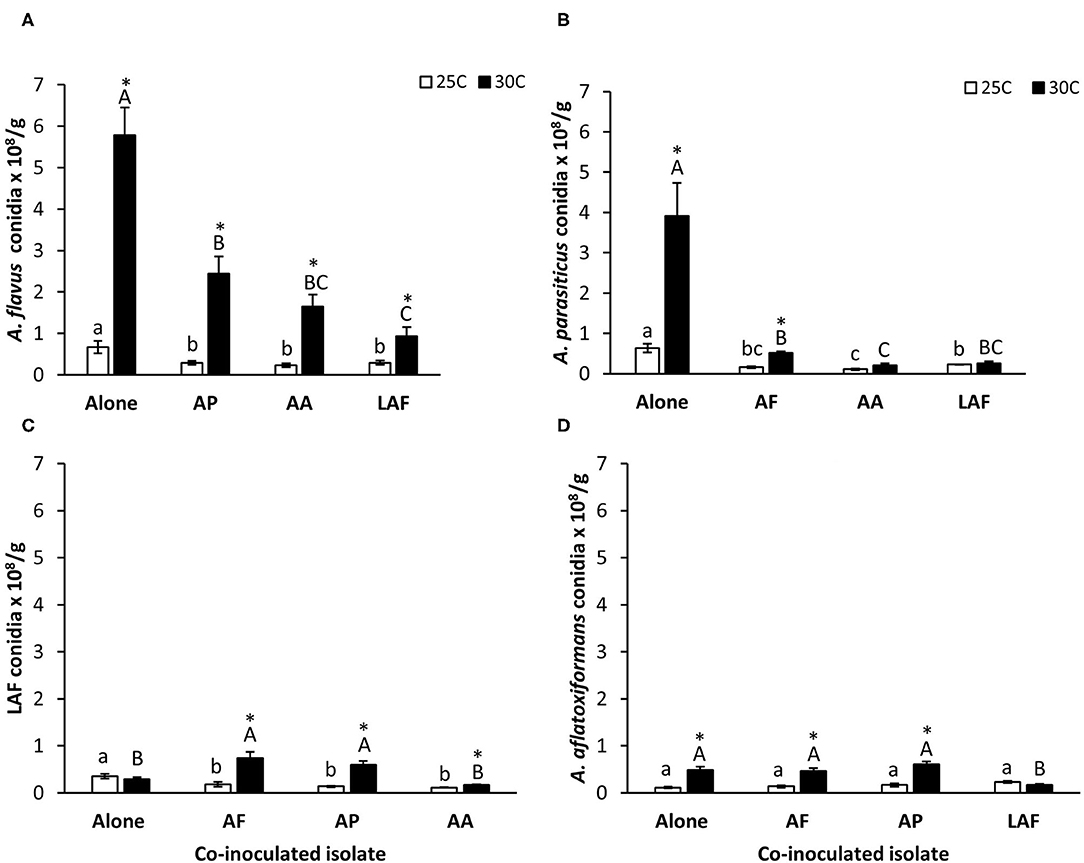
Figure 2. Effect of temperature and co-inoculation of Aspergillus isolates on sporulation during growth on maize kernels at 25 and 30°C, (A) A. flavus conidia alone and in competition with other isolates, (B) A. parasiticus conidia alone and in competition with other isolates, (C) LAF conidia alone and in competition with other isolates and (D) A. aflatoxiformans conidia alone and in competition with other isolates. AF, A. flavus L strain (AF13) (L-type); AP, A. parasiticus (AP2999) (L-type); AA, A. aflatoxiformans (BN008-R) (S-type); LAF; Lethal Aflatoxicosis Fungus (K0550K) (S-type). Quantities of conidia produced by each species during competition were determined by multiplying the proportion of conidial DNA of each isolate measured using quantitative pyrosequencing by the total number of conidia produced by the co-inoculated isolates. Bars followed by similar letters (lower case letters 25°C treatment and uppercase letters 30°C treatment) are not significantly different (P >0.05) Tukey's HSD. Asterisks denote significant difference between 25 and 30°C treatments (t-test, P < 0.05).
Discussion
This study empirically demonstrates the complex dynamics that occur during co-colonization of a crop substrate by different aflatoxin-producing fungal species and the role of temperature on outcomes of competition. As hypothesized, temperature had a significant influence on the competition between Aspergillus spp., with higher temperature favoring colonization of maize kernels by the S-type species, A. aflatoxiformans and LAF. In contrast, the relative colonizing ability of A. parasiticus was greater at the lower temperature (25°C). In most cases, aflatoxin production by both individual and paired isolates increased at the higher temperature as did the relative proportion of B vs. G aflatoxins. Competition suppressed sporulation by L-type isolates of A. flavus and A. parasiticus, but the response of the S-type fungi was variable. With a few exceptions, the results of competition during colonization was predictive of the relative sporulation of co-inoculated species. Overall, results indicate that temperature will influence both the structure of fungal communities associated with a crop and the quantity and types of aflatoxins produced.
The ability of a pathogen to colonize and effectively exploit a host substrate is crucial for its growth and reproduction. In addition, colonization is of special concern when it comes to aflatoxin contamination events. For example, it has been previously demonstrated that A. flavus individuals that are highly competitive during colonization have the greatest influence on aflatoxin content within infected host tissues (Cotty, 1989; Mehl and Cotty, 2010). In this study, differences in relative kernel colonization of Aspergillus spp. were observed with a shift in temperature from 25 to 30°C. At 30°C the S-type species were more competitive than L-types A. flavus and A. parasiticus, suggesting that at higher temperatures, S-type species dominate somatic growth and potentially have greater access to nutrient resources essential for growth and reproduction. Furthermore, by dominating mycelial growth within the substrate, the S-type fungi could be the major contributors of aflatoxins in the crop when both species are present in the same field. It has been repeatedly observed that S-type fungi are the major contributors of aflatoxin contamination even if they comprise a relatively small proportion of the overall fungal community associated with the crop (Cotty, 1997; Cardwell and Cotty, 2002; Atehnkeng et al., 2008a). Thus, not only do these species have high aflatoxin-producing potential, their increased competitive ability during colonization at higher temperatures may favor dominance of S-type species in the crop-infecting fungal community.
The reduction in sporulation by the L-type fungi A. flavus and A. parasiticus and the increase or no change in sporulation by the S-type fungi during competition suggests that S-type species have the ability to modulate the behavior of other species. The S-type species may be enhancing their reproductive success over those species that produce copious quantities of conidia in the absence of competition by maintaining or increasing their sporulation while suppressing sporulation by L-type fungi. This ability to produce more conidia during competition thus enhances ability to colonize new substrates and to increase one's frequency in the environment (Jaime-Garcia and Cotty, 2004; Mehl and Cotty, 2010; Mehl et al., 2012). By maintaining or increasing sporulation while suppressing sporulation by L-type fungi, the S-type species may gain a dispersal advantage over L-type species that produce copious quantities of conidia in the absence of competition. Sporulation behavior of the S-type fungi during competition with the L-type fungi may reflect differences in growth strategies between the two sclerotial types, with each type shifting resources from somatic growth to production of conidia under different conditions resulting in increased dispersal and of each sclerotial type under different conditions (Mehl and Cotty, 2010). Although we did not quantify sclerotia in this study, Garber and Cotty (1997) reported a reduction in sclerotial formation when A. flavus L-type and S-type competed. In addition, Garber and Cotty (1997) assumed total spores produced during competition between A. flavus L-type and S-type belonged primarily to the A. flavus L-type. However, in the current study we discovered that S-type fungi produce greater quantities of conidia than expected during competition with an L-type A. flavus isolate based on sporulation in the absence of competition. In addition, since the S-type fungi were better colonizers than the L-type fungi at the higher temperature, competitive exclusion and greater access to host resources would provide S-type species with nutrients necessary for growth and dispersal (Cotty and Bayman, 1993; Lee and Magan, 2000; Mehl and Cotty, 2010; Hruska et al., 2014). It is also possible that the reduction in sporulation by the L-type species during competition resulted from production of secondary metabolites by S-type species that inhibited sporulation by the competitors during competition for resources (Losada et al., 2009; Mehl and Cotty, 2013b). This is supported by co-cultivation competition experiments that found novel secondary metabolites may be produced through activation of silent gene clusters in Aspergillus species (Brakhage and Schroeckh, 2011).
The apparent difference in growth strategy observed for the S-type species compared to the L-type suggests that niche partitioning exists between these fungi, allowing for their coexistence in the environment (Bayman and Cotty, 1991; Mehl and Cotty, 2010; Ohkura et al., 2018). The change in strategy manifested by the interacting species at different temperatures suggests that temperature may also influence niche partitioning (Fitt et al., 2006), with warmer temperatures driving the selection of highly toxigenic S-type fungi (Bock et al., 2004; Jaime-Garcia and Cotty, 2010). Thus, over time, consistently warmer temperatures could shift the Aspergillus community structure making S-type fungi more prevalent in the environment. Consistent with this observation, other field studies (Nesci and Etcheverry, 2002; Barros et al., 2005; Giorni et al., 2007; Atehnkeng et al., 2008a; Donner et al., 2009; Probst et al., 2010; Diedhiou et al., 2011; Ortega-Beltran et al., 2015; Kachapulula et al., 2017; Agbetiameh et al., 2018; Sserumaga et al., 2020) have reported high frequencies of S-type species across warm regions. In regions where Aspergillus species co-occur, changes in weather patterns such as shifts to prolonged hot and dry seasons as a result of climate change might favor increases in the frequency of highly aflatoxigenic S-type fungi associated with crops, thus increasing the frequency and severity of aflatoxin contamination.
Outcomes of competition and the quantity and types of aflatoxins produced by different Aspergillus species greatly impact the extent to which crops are contaminated with aflatoxins and thus have the potential to affect both animal and human health. Although all the isolates used in this study produce high concentrations of aflatoxins, aflatoxin concentrations in co-inoculation treatments were sometimes greater or less than expected based on outcomes of competition and aflatoxin production of individual isolates. For example, although only a single isolate was evaluated for each species in this study, A. aflatoxiformans in competition with the L-type species A. flavus and A. parasiticus resulted in greater than expected aflatoxin while LAF in competition with these species resulted in less aflatoxin than expected. Thus, interactions between Aspergillus species can be synergistic or antagonistic in terms of aflatoxin production, with consequences on overall aflatoxin production potential of the fungal community dependent on which species are present. Total aflatoxins produced by both the paired and individually cultivated isolates were high at both temperatures evaluated; this was expected since other studies have found that temperatures ranging from 25 to 35°C are favorable for aflatoxin production (Ogundero, 1987; Schmidt-Heydt et al., 2009; Singh et al., 2020). Except for A. flavus alone and A. flavus with LAF, paired and individual species produced more than twice as much aflatoxin with an increase in temperature from 25 to 30°C. In addition, the proportion of total aflatoxins comprised of B aflatoxins increased with increasing temperature in most of the treatments that included species that produce both B and G aflatoxins. The change in proportion of B aflatoxins can be explained by differences in expression ratios of aflR and aflS genes. Lower temperatures have been shown to favor expression of the aflS gene, which results in increased G aflatoxin production, while higher temperatures are associated with an increase in aflR gene expression that is associated with B aflatoxin production (Schmidt-Heydt et al., 2010). Thus, higher temperatures may increase the severity and incidence of aflatoxin contamination (Probst et al., 2010) as well as an increasing concentration of the more carcinogenic B aflatoxins in crops compared to G aflatoxins.
As demonstrated in the current study, the complex interplay between competing Aspergillus species and temperature has important implications for both incidence and severity of aflatoxin contamination in crops. Despite this, little attention has been paid when assessing aflatoxin mitigation methods in specific regions that vary in both temperature during the growing season and composition of aflatoxigenic species associated with crops. Thus, there is a need for future studies to address the impacts of other abiotic and biotic factors (e.g., host, other competing microorganisms, moisture, pH) on the degree of aflatoxin contamination on crops. In addition, since this study only examined the behavior of a single isolate of each species, future studies should compare multiple isolates within a species to ascertain if their behavior during competition is species or isolate specific (or both). Results from this study may also have implications on aflatoxin management strategies that aim at modulating aflatoxigenic fungal communities, such as the use of non-aflatoxigenic (atoxigenic) A. flavus as a biocontrol agent (Cotty, 1994). During the initial stages of selection of atoxigenic A. flavus for development of aflatoxin biocontrol products, candidate atoxigenic isolates are typically co-inoculated with an aflatoxigenic A. flavus isolate in a laboratory competition assay at 31°C (Atehnkeng et al., 2008b; Mauro et al., 2015; Agbetiameh et al., 2019). However, this study suggests that competition experiments for biocontrol strain selection should be conducted against multiple aflatoxigenic species at multiple temperatures in order to determine which atoxigenic A. flavus will be the most effective at displacing and reducing aflatoxin production by a variety of aflatoxin-producing species across a range of environments. In addition, the implementation of biocontrol strategies should take into consideration seasonal changes in temperature across regions and the effects of climate change, both of which are capable of shifting Aspergillus population structure, especially when high temperature events favor highly toxigenic S-type fungi. With increasing global temperatures, the performance of biological control strains should be periodically assessed with respect to their effectiveness against a shifting Aspergillus community structure.
Data Availability Statement
The datasets generated for this study are available upon request to the corresponding author.
Author Contributions
CC and PC contributed to conception and design of the study. CC performed the experiments, HM helped with data analysis CC wrote first draft of the manuscript. HM, KC, RB, JA, MO, and PC provided supervision. All authors contributed to manuscript revision and read and approved the submitted version.
Funding
This research was supported by the Agricultural Research Service, The United States Department of Agriculture CRIS project 2020-42000-022-00D, USAID Feed the Future Malawi Improved Seed System and Technologies (Aflasafe Component) project and the Bill and Melinda Gates Foundation (OPP OPP1007117).
Author Disclaimer
Mention of trade names or commercial products in this publication is solely to provide specific information and does not imply recommendation or endorsement by the U.S. Departure of Agriculture. The U.S. Department of Agriculture is an Equal Opportunity Employer.
Conflict of Interest
The authors declare that the research was conducted in the absence of any commercial or financial relationships that could be construed as a potential conflict of interest.
Publisher's Note
All claims expressed in this article are solely those of the authors and do not necessarily represent those of their affiliated organizations, or those of the publisher, the editors and the reviewers. Any product that may be evaluated in this article, or claim that may be made by its manufacturer, is not guaranteed or endorsed by the publisher.
References
Agbetiameh, D., Ortega-Beltran, A., Awuah, R. T., Atehnkeng, J., Cotty, P. J., and Bandyopadhyay, R. (2018). Prevalence of aflatoxin contamination in maize and groundnut in Ghana: population structure, distribution, and toxigenicity of the causal agents. Plant Dis. 102, 764–772. doi: 10.1094/PDIS-05-17-0749-RE
Agbetiameh, D., Ortega-Beltran, A., Awuah, R. T., Atehnkeng, J., Islam, M. S., Callicott, K. A., et al. (2019). Potential of atoxigenic Aspergillus flavus vegetative compatibility groups associated with maize and groundnut in Ghana as biocontrol agents for aflatoxin management. Front. Microbiol. 10:2069. doi: 10.3389/fmicb.2019.02069
Atehnkeng, J., Ojambo, P. S., Ikotun, T., Sikora, R. A., Cotty, P. J., and Bandyopadhyay, R. (2008b). Evaluation of atoxigenic isolates of Aspergillus flavus as potential biocontrol agents for aflatoxin in maize. Food Addit. Contam. 25, 1264–1271. doi: 10.1080/02652030802112635
Atehnkeng, J., Ojiambo, P. S., Donner, M., Ikotun, T., Sikora, R. A., Cotty, P. J., et al. (2008a). Distribution and toxigenicity of Aspergillus species isolated from maize kernels from three agro-ecological zones in Nigeria. Int. J. Food Microbiol. 122, 74–84. doi: 10.1016/j.ijfoodmicro.2007.11.062
Barros, G., Torres, A., and Chulze, S. (2005). Aspergillus flavus population isolated from soil of Argentina's peanut-growing region: sclerotia production and toxigenic profile. J. Sci. Food Agric. 85, 2349–2353. doi: 10.1002/jsfa.2257
Bayman, P., and Cotty, P. J. (1991). Vegetative compatibility and genetic diversity in the Aspergillus flavus population of a single field. Can. J. Bot. 69, 1707–1711. doi: 10.1139/b91-216
Bhatnagar, D., Cary, J. W., Ehrlich, K., Yu, J., and Cleveland, T. E. (2006). Understanding the genetics of regulation of aflatoxin production and Aspergillus flavus development. Mycopathologia 162:155. doi: 10.1007/s11046-006-0050-9
Bock, C. H., and Cotty, P. J. (1999). Wheat seed colonized with atoxigenic Aspergillus flavus: characterization and production of a biopesticide for aflatoxin control. Biocontrol Sci. Tech. 9, 529–543. doi: 10.1080/09583159929497
Bock, C. H., Mackey, B., and Cotty, P. J. (2004). Population dynamics of Aspergillus flavus in the air of an intensively cultivated region of south-west Arizona. Plant Pathol. 53, 422–433. doi: 10.1111/j.0032-0862.2004.01015.x
Brakhage, A. A., and Schroeckh, V. (2011). Fungal secondary metabolites–strategies to activate silent gene clusters. Fungal Genet. Biol. 48, 15–22. doi: 10.1016/j.fgb.2010.04.004
Callicott, K. A., and Cotty, P. J. (2015). Method for monitoring deletions in the aflatoxin biosynthesis gene cluster of Aspergillus flavus with multiplex PCR. Lett. Appl. Microbiol. 60, 60–65. doi: 10.1111/lam.12337
Cardwell, K. F., and Cotty, P. J. (2002). Distribution of Aspergillus section Flavi among field soils from the four agroecological zones of the Republic of Benin, West Africa. Plant Dis. 86, 434–439. doi: 10.1094/PDIS.2002.86.4.434
Cotty, P. J. (1989). Virulence and cultural characteristics of two Aspergillus flavus strains pathogenic on cotton. Phytopathology 79, 808–814. doi: 10.1094/Phyto-79-808
Cotty, P. J. (1994). Influence of field application of an atoxigenic strain of Aspergillus flavus on the populations of A. flavus infecting cotton bolls and on the aflatoxin content of cottonseed. Phytopathology 84, 1270–1277. doi: 10.1094/Phyto-84-1270
Cotty, P. J. (1997). Aflatoxin-producing potential of communities of Aspergillus section Flavi from cotton producing areas in the United States. Mycol. Res. 101, 698–704. doi: 10.1017/S0953756296003139
Cotty, P. J., and Bayman, P. (1993). Competitive exclusion of a toxigenic strain of Aspergillus flavus by an atoxigenic strain. Phytopathology 83, 1283–1287. doi: 10.1094/Phyto-83-1283
Cotty, P. J., Bayman, P., Egel, D. S., and Elias, K. S. (1994). “Agriculture, aflatoxins and Aspergillus,” in The Genus Aspergillus (Boston, MA: Springer), 1–27. doi: 10.1007/978-1-4899-0981-7_1
Cotty, P. J., and Cardwell, K. F. (1999). Divergence of West African and North American Communities of Aspergillus Section Flavi. Appl. Environ. Microbiol. 65, 2264–2266. doi: 10.1128/AEM.65.5.2264-2266.1999
Cotty, P. J., and Jaime-Garcia, R. (2007). Influences of climate on aflatoxin producing fungi and aflatoxin contamination. Int. J. Food Microbiol. 119, 109–115. doi: 10.1016/j.ijfoodmicro.2007.07.060
Cotty, P. J., Probst, C., and Jaime-Garcia, R. (2008). “Etiology and management of aflatoxin contamination,” in Mycotoxins: Detection Methods, Management, Public Health and Agricultural Trade, eds J. F. Leslie, R. Bandyopadhyay, and A. Visconti (Wallingworth: CABI), 287–299. doi: 10.1079/9781845930820.0287
Delmotte, N., Knief, C., Chaffron, S., Innerebner, G., Roschitzki, B., Schlapbach, R., et al. (2009). Community proteogenomics reveals insights into the physiology of phyllosphere bacteria. Proc. Natl. Acad. Sci. U. S. A. 106, 16428–16433. doi: 10.1073/pnas.0905240106
Diedhiou, P. M., Bandyopadhyay, R., Atehnkeng, J., and Ojiambo, P. S. (2011). Aspergillus colonization and aflatoxin contamination of maize and sesame kernels in two agro-ecological zones in Senegal. J. Phytopathol. 159, 268–275. doi: 10.1111/j.1439-0434.2010.01761.x
Diener, U. L., Cole, R. J., Sanders, T. H., Payne, G. A., Lee, L. S., and Klich, M. A. (1987). Epidemiology of aflatoxin formation by Aspergillus flavus. Annu. Rev. Phytopathol. 25, 249–270. doi: 10.1146/annurev.py.25.090187.001341
Donner, M., Atehnkeng, J., Sikora, R. A., Bandyopadhyay, R., and Cotty, P. J. (2009). Distribution of Aspergillus section Flavi in soils of maize fields in three agroecological zones of Nigeria. Soil Biol. Biochem. 41, 37–44. doi: 10.1016/j.soilbio.2008.09.013
Edgar, R. C. (2004). MUSCLE: multiple sequence alignment with high accuracy and high throughput. Nucleic Acids Res. 32, 1792–1797. doi: 10.1093/nar/gkh340
Fitt, B. D., Huang, Y. J., van den Bosch, F., and West, J. S. (2006). Coexistence of related pathogen species on arable crops in space and time. Annu. Rev. Phytopathol. 44, 163–182. doi: 10.1146/annurev.phyto.44.070505.143417
Frisvad, J. C., Hubka, V., Ezekiel, C. N., Hong, S. B., Novákov,á, A., Chen, A. J., et al. (2019). Taxonomy of Aspergillus section Flavi and their production of aflatoxins, ochratoxins and other mycotoxins. Stud. Mycol. 93, 1–63. doi: 10.1016/j.simyco.2018.06.001
Garber, R. K., and Cotty, P. J. (1997). Formation of sclerotia and aflatoxins in developing cotton bolls infected by the S strain of Aspergillus flavus and potential for biocontrol with an atoxigenic strain. Phytopathology 87, 940–945. doi: 10.1094/PHYTO.1997.87.9.940
Giorni, P., Magan, N., Pietri, A., Bertuzzi, T., and Battilani, P. (2007). Studies on Aspergillus section Flavi isolated from maize in northern Italy. Int. J. Food Microbiol. 113, 330–338. doi: 10.1016/j.ijfoodmicro.2006.09.007
Gong, Y., Hounsa, A., Egal, S., Turner, P. C., Sutcliffe, A. E., Hall, A. J., et al. (2004). Postweaning exposure to aflatoxin results in impaired child growth: a longitudinal study in Benin, West Africa. Environ. Health Perspect. 112, 1334–1338. doi: 10.1289/ehp.6954
Hernandez-Martinez, R., and Navarro-Blasco, I. (2010). Aflatoxin levels and exposure assessment of Spanish infant cereals. Food Addit. Contam. 3, 275–288. doi: 10.1080/19393210.2010.531402
Hiscox, J., Clarkson, G., Savoury, M., Powell, G., Savva, I., Lloyd, M., et al. (2016). Effects of pre-colonisation and temperature on interspecific fungal interactions in wood. Fungal Ecol. 21, 32–42. doi: 10.1016/j.funeco.2016.01.011
Horn, B. W. (2003). Ecology and population biology of aflatoxigenic fungi in soil. J. Toxicol. Toxin. Rev. 22, 351–379. doi: 10.1081/TXR-120024098
Horn, B. W. (2005). Colonization of wounded peanut seeds by soil fungi: selectivity for species from Aspergillus section Flavi. Mycologia 97, 202–217. doi: 10.3852/mycologia.97.1.202
Hruska, Z., Rajasekaran, K., Yao, H., Kinkaid, R., Darlington, D., Brown, R. L., et al. (2014). Co-inoculation of aflatoxigenic and non-aflatoxigenic strains of Aspergillus flavus to study fungal invasion, colonization, and competition in maize kernels. Front. Microbiol. 5, 122. doi: 10.3389/fmicb.2014.00122
Jaime-Garcia, R., and Cotty, P. J. (2004). Aspergillus flavus in soils and corncobs in south Texas: implications for management of aflatoxins in corn-cotton rotations. Plant Dis. 88, 1366–1371. doi: 10.1094/PDIS.2004.88.12.1366
Jaime-Garcia, R., and Cotty, P. J. (2010). Crop rotation and soil temperature influence the community structure of Aspergillus flavus in soil. Soil Biol. Biochem. 42, 1842–1847. doi: 10.1016/j.soilbio.2010.06.025
Kachapulula, P. W., Akello, J., Bandyopadhyay, R., and Cotty, P. J. (2017). Aspergillus section Flavi community structure in Zambia influences aflatoxin contamination of maize and groundnut. Int. J. Food Microbiol. 261, 49–56. doi: 10.1016/j.ijfoodmicro.2017.08.014
Klich, M. A. (2007). Aspergillus flavus: the major producer of aflatoxin. Mol. Plant Pathol. 8, 713–722. doi: 10.1111/j.1364-3703.2007.00436.x
Lee, H. B., and Magan, N. (2000). Impact of environment and interspecific interactions between spoilage fungi and Aspergillus ochraceus on growth and ochratoxin production in maize grain. Int. J. Food Microbiol. 61, 11–16. doi: 10.1016/S0168-1605(00)00385-8
Lindow, S. E., and Brandl, M. T. (2003). Microbiology of the phyllosphere. Appl. Environ. Microbiol. 69, 1875–1883. doi: 10.1128/AEM.69.4.1875-1883.2003
Liu, Y., and Wu, F. (2010). Global burden of aflatoxin-induced hepatocellular carcinoma: a risk assessment. Environ. Health Perspect. 118, 818–824. doi: 10.1289/ehp.0901388
Losada, L., Ajayi, O., Frisvad, J. C., Yu, J., and Nierman, W. C. (2009). Effect of competition on the production and activity of secondary metabolites in Aspergillus species. Med. Mycol. 47, 88–96. doi: 10.1080/13693780802409542
Manabe, M., Tsuruta, O., Goto, T., and Matsuura, S. (1978). Study on distribution of mycotoxin-producing fungi, 4: Mycotoxin-producing ability of Aspergillus strains inhabited in Southeast Asia. Rep. Natl. Food. Res. Inst. 33, 49–56.
Mauro, A., Battilani, P., and Cotty, P. J. (2015). Atoxigenic Aspergillus flavus endemic to Italy for biocontrol of aflatoxins in maize. BioControl 60, 125–134. doi: 10.1007/s10526-014-9624-5
Mehl, H. L., and Cotty, P. J. (2010). Variation in competitive ability among isolates of Aspergillus flavus from different vegetative compatibility groups during maize infection. Phytopathology 100, 150–159. doi: 10.1094/PHYTO-100-2-0150
Mehl H. L. Cotty P. J. (2013a) Influence of plant host species on intraspecific competition during infection by Aspergillus flavus. Plant Pathol. 62, 1310–1318.
Mehl, H. L., and Cotty, P. J. (2013b). Nutrient environments influence competition among Aspergillus flavus genotypes. Appl. Environ. Microbiol. 79, 1473–1480. doi: 10.1128/AEM.02970-12
Mehl, H. L., Jaime, R., Callicott, K. A., Probst, C., Garber, N. P., Ortega-Beltran, A., et al. (2012). Aspergillus flavus diversity on crops and in the environment can be exploited to reduce aflatoxin exposure and improve health. Ann. N. Y. Acad. Sci. 1273, 7–17. doi: 10.1111/j.1749-6632.2012.06800.x
Mitchell, N. J., Bowers, E., Hurburgh, C., and Wu, F. (2016). Potential economic losses to the US corn industry from aflatoxin contamination. Food Addit. Contam. 33, 540–550. doi: 10.1080/19440049.2016.1138545
Nesci, A., and Etcheverry, M. (2002). Aspergillus section Flavi populations from field maize in Argentina. Lett. Appl. Microbiol. 34, 343–348. doi: 10.1046/j.1472-765X.2002.01094.x
O'Brian, G. R., Georgianna, D. R., Wilkinson, J. R., Yu, J., Abbas, H. K., Bhatnagar, D., et al. (2007). The effect of elevated temperature on gene transcription and aflatoxin biosynthesis. Mycologia 99, 232–239. doi: 10.1080/15572536.2007.11832583
Ogundero, V. W. (1987). Temperature and aflatoxin production by Aspergillus flavus and A. parasiticus strains from Nigerian groundnuts. J. Basic Microbiol. 27, 511–514. doi: 10.1002/jobm.3620270910
Ohkura, M., Cotty, P. J., and Orbach, M. J. (2018). Comparative genomics of Aspergillus flavus S and L morphotypes yield insights into niche adaptation. G3 Genes Genom. Genet. 8, 3915–3930. doi: 10.1534/g3.118.200553
Ortega-Beltran, A., Jaime, R., and Cotty, P. J. (2015). Aflatoxin-producing fungi in maize field soils from sea level to over 2000 masl: a three year study in Sonora, Mexico. Fungal Biol. 119, 191–200. doi: 10.1016/j.funbio.2014.12.006
Payne, G. A., Cassel, D. K., and Adkins, C. R. (1985). Reduction of aflatoxin levels in maize due to irrigation and tillage. Phytopathology 75, 1283–1283.
Perrone, G., Gallo, A., and Logrieco, A. F. (2014). Biodiversity of Aspergillus section Flavi in Europe in relation to the management of aflatoxin risk. Front. Microbiol. 5:377. doi: 10.3389/fmicb.2014.00377
Pildain, M. B., Frisvad, J. C., Vaamonde, G., Cabral, D., Varga, J., and Samson, R. A. (2008). Two novel aflatoxin-producing Aspergillus species from Argentinean peanuts. Int. J. Syst. Evol. Microbiol. 58, 725–735. doi: 10.1099/ijs.0.65123-0
Pildain, M. B., Vaamonde, G., and Cabral, D. (2004). Analysis of population structure of Aspergillus flavus from peanut based on vegetative compatibility, geographic origin, mycotoxin and sclerotia production. Int. J. Food Microbiol. 93, 31–40. doi: 10.1016/j.ijfoodmicro.2003.10.007
Pitt, J. I., and Miscamble, B. F. (1995). Water relations of Aspergillus flavus and closely related species. J. Food Protect. 58, 86–90. doi: 10.4315/0362-028X-58.1.86
Pons, W. A. Jr., Robertson, J. A., and Goldblatt, L. A. (1969). Collaborative study on the determination of aflatoxins in cottonseed products. J. Assoc. Off. Anal. Chem. 51, 61–72. doi: 10.1093/jaoac/52.1.61
Probst, C., Callicott, K. A., and Cotty, P. J. (2012). Deadly strains of Kenyan Aspergillus are distinct from other aflatoxin producers. Eur. J. Plant Pathol. 132, 419–429. doi: 10.1007/s10658-011-9887-y
Probst, C., and Cotty, P. J. (2012). Relationships between in vivo and in vitro aflatoxin production: reliable prediction of fungal ability to contaminate maize with aflatoxins. Fungal Biol. 116, 503–510. doi: 10.1016/j.funbio.2012.02.001
Probst, C., Njapau, H., and Cotty, P. J. (2007). Outbreak of an acute aflatoxicosis in Kenya in 2004: identification of the causal agent. Appl. Environ. Microbiol. 73, 2762–2764. doi: 10.1128/AEM.02370-06
Probst, C., Schulthess, F., and Cotty, P. J. (2010). Impact of Aspergillus section Flavi community structure on the development of lethal levels of aflatoxins in Kenyan maize (Zea mays). J. Appl. Microbiol. 108, 600–610. doi: 10.1111/j.1365-2672.2009.04458.x
Rambo, G. W., Tuite, J., and Crane, P. (1974). Preharvest inoculation and infection of dent corn ears with Aspergillus flavus and Aspergillus parasiticus. Phytopathology 64, 797–800. doi: 10.1094/Phyto-64-797
Scheidegger, K. A., and Payne, G. A. (2003). Unlocking the secrets behind secondary metabolism: a review of Aspergillus flavus from pathogenicity to functional genomics. J. Toxicol. Toxin. Rev. 22, 423–459. doi: 10.1081/TXR-120024100
Schmidt-Heydt, M., Abdel-Hadi, A., Magan, N., and Geisen, R. (2009). Complex regulation of the aflatoxin biosynthesis gene cluster of Aspergillus flavus in relation to various combinations of water activity and temperature. Int. J. Food Microbiol. 135, 231–237. doi: 10.1016/j.ijfoodmicro.2009.07.026
Schmidt-Heydt, M., Rufer, C. E., Abdel-Hadi, A., Magan, N., and Geisen, R. (2010). The production of aflatoxin B1 or G1 by Aspergillus parasiticus at various combinations of temperature and water activity is related to the ratio of aflS to aflR expression. Mycotoxin Res. 26, 241–246. doi: 10.1007/s12550-010-0062-7
Shearer, J. F., Sweets, L. E., Baker, N. K., and Tiffany, L. H. (1992). A study of Aspergillus flavus/parasiticus in Iowa crop fields: 1988-1990. Plant Dis. 76, 19–22. doi: 10.1094/PD-76-0019
Singh, P., Callicott, K. A., Orbach, M. J., and Cotty, P. J. (2020). Molecular analysis of S-morphology aflatoxin producers from the United States reveals previously unknown diversity and two new taxa. Front. Microbiol. 11:1236. doi: 10.3389/fmicb.2020.01236
Singh, P., and Cotty, P. J. (2019). Characterization of Aspergilli from dried red chilies (Capsicum spp.): Insights into the etiology of aflatoxin contamination. Int. J. Food Microbiol. 289, 145–153. doi: 10.1016/j.ijfoodmicro.2018.08.025
Singh, P., Orbach, M. J., and Cotty, P. J. (2018). Aspergillus texensis: a novel aflatoxin producer with S morphology from the United States. Toxins 10:513. doi: 10.3390/toxins10120513
Sserumaga, J. P., Ortega-Beltran, A., Wagacha, J. M., Mutegi, C. K., and Bandyopadhyay, R. (2020). Aflatoxin-producing fungi associated with pre-harvest maize contamination in Uganda. Int. J. Food Microbiol. 313:108376. doi: 10.1016/j.ijfoodmicro.2019.108376
Sweany, R. R., Damann, K. E. Jr, and Kaller, M. D. (2011). Comparison of soil and corn kernel Aspergillus flavus populations: evidence for niche specialization. Phytopathology 101,952–959. doi: 10.1094/PHYTO-09-10-0243
Williams, J. H., Phillips, T. D., Jolly, P. E., Stiles, J. K., Jolly, C. M., and Aggarwal, D. (2004). Human aflatoxicosis in developing countries: a review of toxicology, exposure, potential health consequences, and interventions. Am. J. Clin. Nutr. 80, 1106–1122. doi: 10.1093/ajcn/80.5.1106
Keywords: Aspergillus flavus, Aspergillus aflatoxiformans, Aspergillus parasiticus, Lethal Aflatoxicosis Fungus, interspecific competition, aflatoxins
Citation: Ching'anda C, Atehnkeng J, Bandyopadhyay R, Callicott KA, Orbach MJ, Mehl HL and Cotty PJ (2021) Temperature Influences on Interactions Among Aflatoxigenic Species of Aspergillus Section Flavi During Maize Colonization. Front. Fungal Biol. 2:720276. doi: 10.3389/ffunb.2021.720276
Received: 04 June 2021; Accepted: 26 July 2021;
Published: 26 August 2021.
Edited by:
Isabelle P. Oswald, INRA UMR1331 Toxicologie Alimentaire, FranceReviewed by:
Carol Verheecke-Vaessen, Cranfield University, United KingdomSelma Pascale Snini, UMR5503 Laboratoire de Génie Chimique (LGC), France
Copyright © 2021 Ching'anda, Atehnkeng, Bandyopadhyay, Callicott, Orbach, Mehl and Cotty. This is an open-access article distributed under the terms of the Creative Commons Attribution License (CC BY). The use, distribution or reproduction in other forums is permitted, provided the original author(s) and the copyright owner(s) are credited and that the original publication in this journal is cited, in accordance with accepted academic practice. No use, distribution or reproduction is permitted which does not comply with these terms.
*Correspondence: Hillary L. Mehl, hillary.mehl@usda.gov; Connel Ching'anda, connelchinganda@email.arizona.edu
†These authors share senior authorship
‡These authors share last authorship