- Department of Plant Pathology and Weed Research, Plant Protection Institute, Agricultural Research Organization, Volcani Institute, Rishon LeZion, Israel
Fungal and bacterial pathogens generate devastating diseases and cause significant tomato crop losses worldwide. Due to chemical pesticides harming the environment and human health, alternative disease control strategies, including microorganismal bio-control agents (BCAs), are increasingly sought-after in agriculture. Bio-control microorganisms such as Trichoderma spp. have been shown to activate induced systemic resistance (ISR) in the host. However, examples of highly active bio-control microorganisms in agricultural settings are still lacking, due primarily to inconsistency in bio-control efficacy, often leading to widespread disease prior to the required ISR induction in the host. As part of its plant colonization strategy, Trichoderma spp. can secrete various compounds and molecules, which can effect host priming/ISR. One of these molecules synthesized and secreted from several species of Trichoderma is the family 11 xylanase enzyme known as ethylene inducing xylanase, EIX. EIX acts as an ISR elicitor in specific plant species and varieties. The response to EIX in tobacco and tomato cultivars is controlled by a single dominant locus, termed LeEIX, which contains two receptors, LeEIX1 and LeEIX2, both belonging to a class of leucine-rich repeat cell-surface glycoproteins. Both receptors are able to bind EIX, however, while LeEIX2 mediates plant defense responses, LeEIX1 acts as a decoy receptor and attenuates EIX induced immune signaling of the LeEIX2 receptor. By mutating LeEIX1 using CRISPR/Cas9, here, we report an enhancement of receptivity to T. harzianum mediated ISR and disease bio-control in tomato.
Introduction
Plant pathogens are the foremost yield limiting factor for many crops in open field and greenhouse cultivation systems (Vitti et al., 2015), and this trend is expected to increase due to climate and regulatory changes. Fungal and bacterial pathogens are the cause of significant tomato crop losses worldwide, generating devastating diseases, in some cases due to wide host range, relatively limited information on pathogen biology and infection strategies, and their ability to remain quiescent for long periods of time and become virulent upon changing conditions (He et al., 2016). Pesticidal strategies can lack effectivity and are often a source of pollution and detrimental effects to consumer health (Yadav and Devi, 2017; Leong et al., 2020), with many pesticides becoming increasingly banned worldwide.
Bio-control of foliar diseases is a potential alternative, non-toxic means of management of foliar pathogens. Induced resistance has been documented as one of the mechanisms responsible for bio-control. Induced resistance is recognized as an important mode of action to achieve bio-control in vegetative tissues (Sequeira, 1983; Kuc, 1987). Induced systemic resistance (ISR) effected by various microorganisms can protect plants against pathogens (Paulitz and Matta, 2000).
One of the most studied commercial bio-control agents (BCAs) is isolate T39 of Trichoderma harzianum, which serves as a model for commercial bio-control and the mechanisms involved. T39 has been shown under commercial greenhouse conditions to control foliar pathogens including Botrytis cinerea, Pseudoperonospora cubensis, Sclerotinia sclerotiorum and Sphaerotheca fusca (syn. S. fuliginea) (Elad, 2000a,b).
ISR is examined by applying a BCA distal to the plant organ subsequently challenged by a pathogen. It was thus demonstrated that T39 causes ISR, inducing plant defense against B. cinerea in several plant hosts. T39 was applied to the soil or the lower leaves, with the pathogen being subsequently applied to the upper canopy of the plants. Given the spatial separation of T39 application from the pathogens, disease protection was attributed to ISR imparted by T. harzianum T39 (De Meyer et al., 1998).
Given the lack of examples of highly successful BCAs in practical disease management, it is possible that current BCAs are unlikely to be able to rapidly achieve satisfactory and stable disease control. The characteristics of BCAs are such that slight changes in the external environment could potentially result in drastic changes in the system dynamics and hence bio-control efficacies (Juroszek and von Tiedemann, 2011). This may explain often observed inconsistencies in bio-control efficacy in practice, as spatio-temporal environmental heterogeneity is a rule rather than an exception, and can lead to widespread disease prior to the required colonization or ISR induction by the bio-control agent. Generating increased receptivity in the host to immunity activation by BCAs could potentially improve BCA effectivity and disease control.
As part of its plant colonization strategy, Trichoderma spp. can secrete various antimicrobial compounds and molecules, which can effect host priming/ISR. One of these molecules synthesized by, and secreted from several species of Trichoderma, including T. viride and T. reseii, is a family 11 xylanase enzyme known as ethylene inducing xylanase, EIX. The Trichoderma fungal protein elicitor EIX, induces ethylene biosynthesis, electrolyte leakage, expression of PR proteins and the hypersensitive response (HR) in specific plant species and/or varieties (Bailey et al., 1990; Sharon et al., 1992; Ron et al., 2000; Elbaz et al., 2002). EIX was shown to specifically bind to the plasma membrane of responsive cultivars of both tomato and tobacco (Hanania and Avni, 1997). The response to EIX in tobacco and tomato cultivars is controlled by a single dominant locus, termed LeEIX (Ron and Avni, 2004). The LeEIX locus contains two receptors, LeEIX1 and LeEIX2, both belonging to a class of leucine-rich repeat cell-surface glycoproteins. Both receptors are able to bind the EIX elicitor, while only the LeEIX2 receptor mediates plant defense responses (Ron and Avni, 2004). LeEIX1 acts as a decoy receptor and attenuates EIX induced signaling of the LeEIX2 receptor (Bar et al., 2010, 2011).
EIX acts to increase plant immunity through binding and downstream signaling of LeEIX2 (Ron and Avni, 2004), while LeEIX1 acts to block this immunity promoting downstream signaling (Bar et al., 2010, 2011). We show here that removing LeEIX1 resulted in stronger immune activation, leading to increased host response to Trichoderma bio-control agents and enhancement of disease resistance conferred by Trichoderma.
Results
Generation of LeEIX1 CRISPR Mutants
Previous research has indicated that LeEIX1 attenuates defense signaling in Solanaceae in a BAK dependent manner (Bar et al., 2010, 2011). To examine this phenomenon, we generated LeEIX1 knockouts using CRISPR/Cas9 as detailed in the Materials and Methods section. CRISPR/Cas9 is a versatile, design-easy, and low-cost tool, which has been used efficiently for precise genome editing in plants. Since its emergence several years ago, CRISPR technology has revolutionized gene editing in molecular biology and agricultural contexts (Molla et al., 2020). Designing specific gRNAs to target only LeEIX1 and not LeEIX2, we were able to obtain two independent homozygous lines, one with a two base deletion (line b5) and the other with a one base deletion (line 14), at a PAM site ~330 nucleotides after the LeEIX1 start codon (Figure 1A, Supplementary Figure 1). Both mutations are predicted to result in a frame shift causing a stop codon, resulting in a truncated 113 amino acid protein from the N-terminus of the 1031 amino acid full LeEIX1, with leeix1-14 additionally having 7 “nonsense” amino acids prior to the premature stop (Figure 1B). The truncated proteins formed in the mutants contain only the signal peptide and N-terminal Leucine zipper, and do not have the LRR domains important for ligand recognition and protein-protein interactions, or the transmembranal domain required for PM insertion (Figure 1C). Thus, these truncated proteins formed would likely not be inserted in the membrane, or be able to bind the xylanase ligand or protein interactors- leading to null of LeEIX1 functionality.
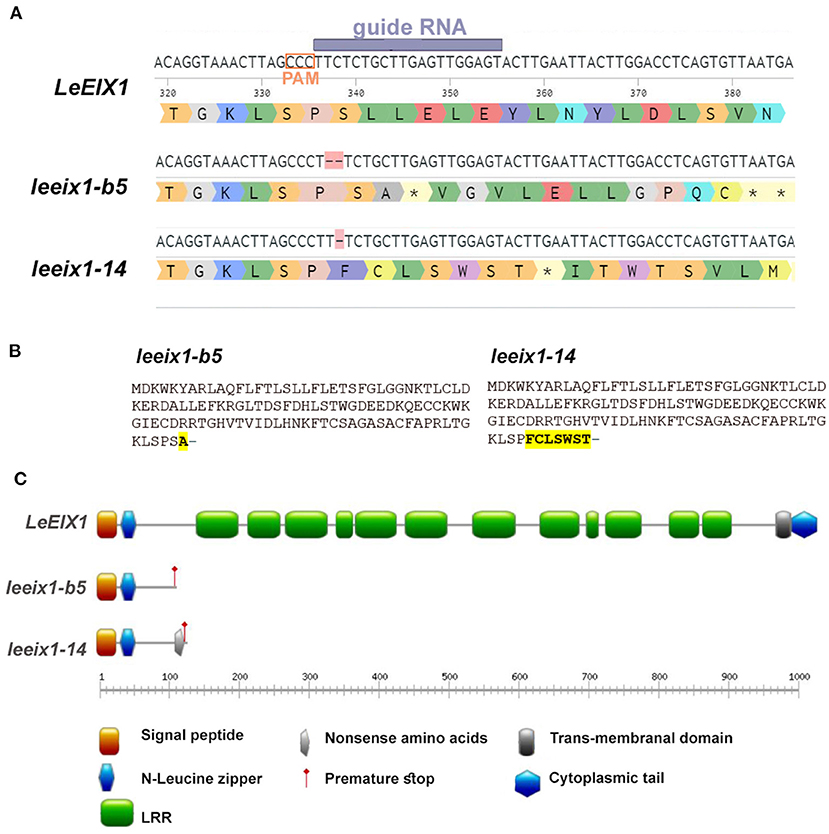
Figure 1. Description of generated leeix1 lines. (A) Representation of gRNA/ PAM site and resultant mutations in the LeEIX1 sequence. (B) Predicted protein sequence of the mutants. (C) Protein domain analysis of LeEIX1 alongside the mutant truncated proteins. (A) Generated with Benchling (Biology Software) (2021). Retrieved from https://benchling.com. (C) Generated with Prosite MyDomain (Hulo et al., 2008).
Verification of Agricultural Quality of Generated Mutants
LeEIX1 and LeEIX2 were originally identified using a screen of the Solanum pennellii M82 introgression populations (Eshed et al., 1992; Ron and Avni, 2004). Based on our previous knowledge that the introgression line IL-7-5 has ~350 genes on the short arm of chromosome 7 originating from S. pennellii, including both LeEIX1 and LeEIX2 which contain various mutations, has ostensibly normal development, we surmised that the mutated LeEIX1 lines would likely not have developmental defects. We analyzed their growth and developmental quality, finding that the leeix1 plants have similar developmental progression, agricultural quality, yield, and tomato quality, as their wild type M82 background line (Figure 2, Supplementary Figures 2, 3). Figure 2 shows results obtained from both homozygous lines, used simultaneously in experiments, while supplemental Supplementary Figure 2 provides data for each line individually.
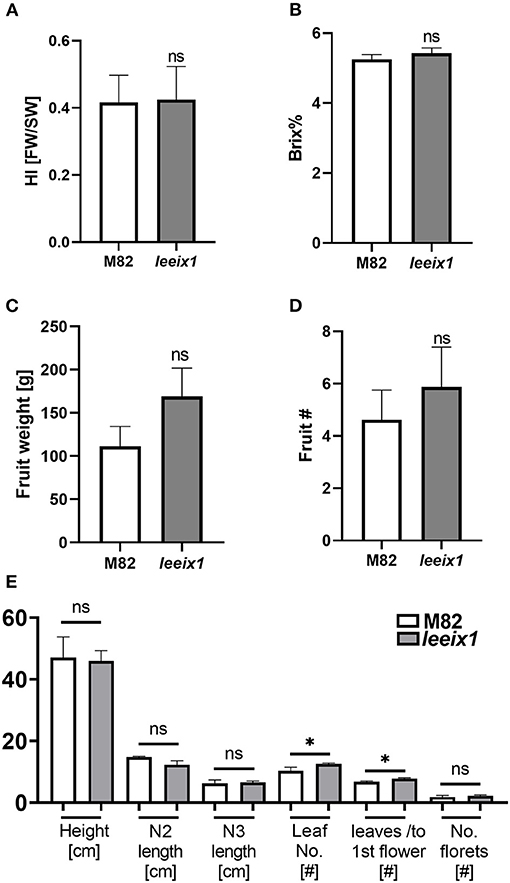
Figure 2. leeix1 mutants have similar agricultural quality as the background M82 line. Agricultural and developmental parameters were measured in M82 and leeix1 plants. Both mutant lines, #b5 and #14, were used in the experiments. (A) Harvest index (HI) of plants was calculated as the ratio between the total mass of fruit yield and the total biomass. (B) Total soluble sugars were measured using a refractometer and are expressed as °Brix. (C) Total fruit weight per plant. (D) The average total number of tomato fruits produced per plant. (E) Analysis of growth and development parameters: Height, length of nodes 2 and 3, Number of leaves, Number of leaves produced in the vegetative stage (before the first flower), and Number of florets. Average ± SEM of at least four independent replicates is shown, N = 18. Except in the number of leaves, no statistically significant differences were observed among WT and leeix1 (t-test, Welch's correction). *p < 0.05.
T. harzianum Treatment Results in Stronger Disease Reduction in leeix1 Mutants
To examine whether T. harzianum mediated bio-control was improved in leeix1 mutants, we treated WT and leeix1 plants with T. harzianum T39, and infected the plants with the necrotrophic fungi B. cinerea or S. sclerotiorum, or the biotrophic fungus Oidium neolycopersici, 3 days after the first treatment. leeix1 plants displayed greater reduction in disease levels following T39 treatment than WT plants (Figure 3, Supplementary Figure 4).
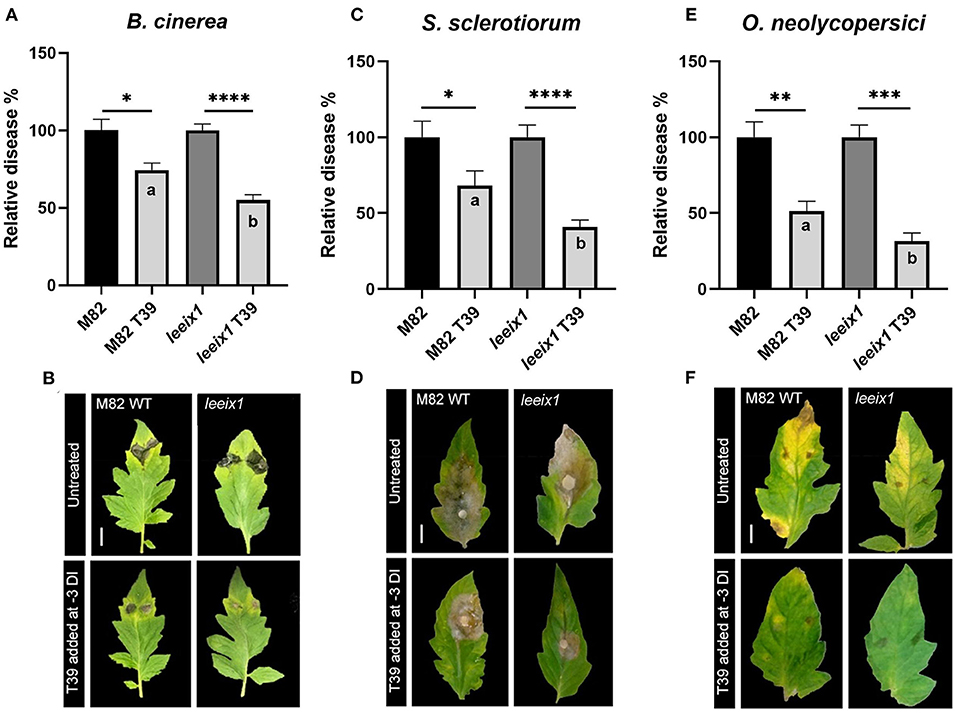
Figure 3. leeix1 mutants have improved disease resistance in response to T. harzianum T39. WT M82 and leeix1 plants were challenged with B. cinerea (106 spores /mL) (A,B), S. sclerotiorum (mycelial plugs from 5 day old plates) (C,D), and O. neolycopersici (104 conidia /mL) (E,F). Relative disease area was calculated as the lesion area measured 5 days after inoculation in each genotype treated with T39 as compared to mock-treated controls for B. cinerea and S. sclerotiorum, and similarly, 10 days after inoculation, for O. neolycopersici. (B,D,F) representative images taken on day of measurement. (A) Average ± SEM of 6 independent replicates is shown, N = 85. (C) Average ± SEM of 3 independent replicates is shown, N = 20. (E) Average ± SEM of 3 independent replicates is shown, N = 12. In all cases, asterisks represent statistical significance of T39 treatment over control in a one way analysis of variance with a Bonferroni post-hoc test, p < 0.0165, and letters represent statistical significance between the WT and leeix1 T39 treated samples in a one way analysis of variance with a Bonferroni post-hoc test, p < 0.03.
T. harzianum Treatment Elicits Stronger Defense Response Activation in leeix1 Mutants
As T. harzianum primed enhanced disease resistance in leeix1 mutants, we examined whether leeix1 mutants had stronger immune responses than control plants when treated with Trichoderma. We measured defense responses following treatment with the Trichoderma derived elicitor EIX, in leeix1 mutants and control plants. leeix1 plants displayed greater responses to EIX, generating significantly higher levels of ethylene (Figure 4A) and ion leakage (Figure 4B), as well as reactive oxygen species (ROS, Figures 4C,D) in response to EIX treatment.
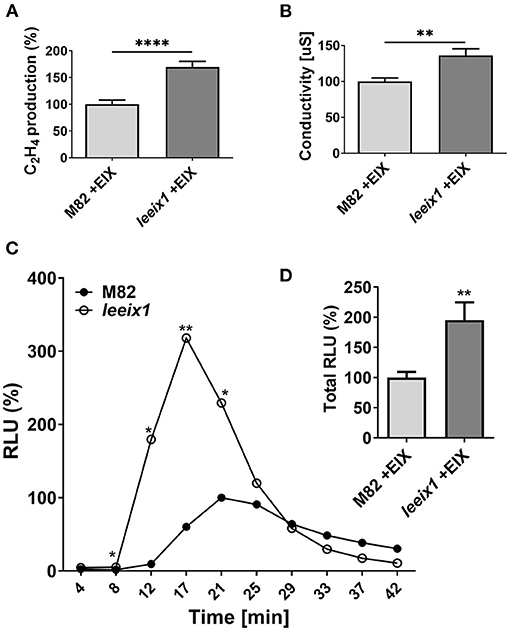
Figure 4. leeix1 mutants have improved defense responses to the Trichoderma elicitor Xyn11/EIX. (A) Ethylene induction after EIX elicitation in M82 and leeix1 was measured using gas-chromatography. M82 average ethylene production was defined as 100%. Average ± SEM of 3 independent experiments is presented, N = 10. Asterisks represent statistical significance in a t-test with Welch's correction (****p < 0.0001). (B) Conductivity of M82 and leeix1 samples immersed in water supplemented with EIX for 24 h was measured. Average ± SEM of 3 independent replicates is shown. Asterisks represent statistical significance in t-test with Welch's correction (**p < 0.01). (C) ROS production of WT M82 and leeix1 was measured immediately after EIX application every 4 min, using the HRP-luminol method. Average ± SEM of 3 independent experiments is shown, N = 26 per time point. Asterisks represent statistical significance in multiple t-tests (one per time point), *p < 0.05, **p < 0.01. (D) Shows total ROS production, **p < 0.01 in a t-test with Welch's correction, N = 26.
leeix1 Possesses Higher Receptivity to Additional Trichoderma Strains
To further characterize the improved receptivity to bio-control we observed in leeix1 mutants, we examined two more Trichoderma isolates in their ability to induce resistance. Another isolate of T. harzianum, NCIM1185 (hereinafter: NCIM), was selected, as well as an isolate of a different species of Trichoderma, T. longibrachiatum, known as T166. Both isolates have proven bio-control activity, though neither was previously tested in tomato (Kapat et al., 1998; Elad and Kapat, 1999; Maymon et al., 2004). Similarly to the T. harzianum T39 strain, the two other Trichoderma isolates showed improved bio-control activity against B. cinerea in leeix1 as compared with the WT M82 background (Figure 5). These results indicate a common mode of action for the Trichoderma isolates.
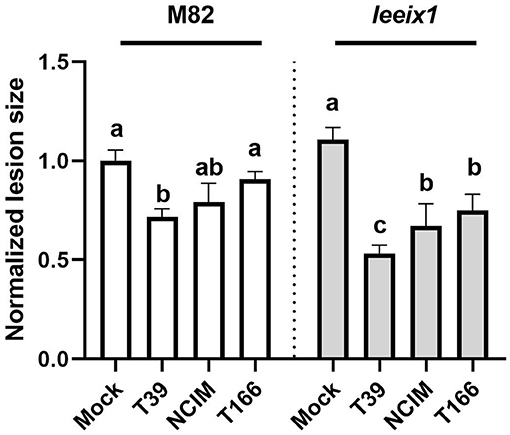
Figure 5. leeix1 mutants have improved disease resistance in response to additional Trichoderma strains. WT M82 and leeix1 plants were challenged with B. cinerea (106 conidia /mL). Relative disease area was calculated as the lesion area measured 5 days after inoculation in each genotype treated with indicated Trichoderma strains as compared to mock-treated controls. Average ± SEM of 4 independent replicates is shown, N = 16, p < 0.0077 in a two-tailed t-test.
Trichoderma Leaf Colonization Is Similar in WT and leeix1 Mutants
Micro-organismal bio-control of plant diseases can be a result of induced systemic resistance, as was reported previously in several cases including for T39 (De Meyer et al., 1998; Elad, 2000a; Perazzolli et al., 2008; Meller-Harel et al., 2014), or, alternatively, can also be the result of microorganism colonization of the plant and direct or chemical effects of said colonizing microorganisms on the attacking pathogens (De Meyer et al., 1998; Elad, 2000a; Perazzolli et al., 2008; Meller-Harel et al., 2014). Additionally, BCA colonization of the plant may be a requirement for the induction of ISR. We examined colonization of different Trichoderma strains in tomato leaves, finding no difference in colonization between WT and leeix1 (Figure 6). As Trichoderma colonization is not favored in leeix1, we conclude that ISR enhancement in leeix1 is likely to be due to another mechanism.
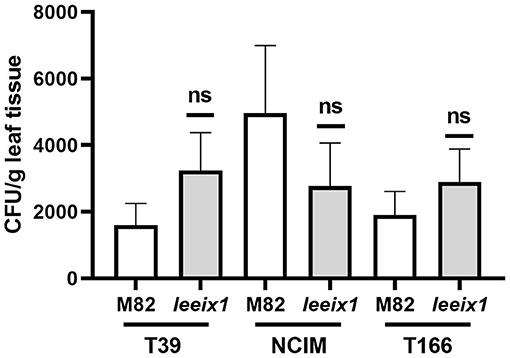
Figure 6. Trichoderma colonizes WT and Leeix1 leaves to similar levels. CFU amount of indicated Trichoderma strains in leaf tissue. Trichoderma spp. were cultured on potato dextrose agar (PDA) (Difco Lab) plates and incubated at 25°C for 7 days. Conidia concentration was adjusted to 106 cells mL−1, and sprayed onto plants. Two weeks after treatment, leaves were harvested and washed in water with thorough shaking. Leaves were then removed and sterilized in bleach for 2 min, then ground with a mortar and pestle. One gram of the ground tissue was re-suspended in 1 mL water. Serial dilutions were plated on PDA plates containing 0.02% of rose bengal. Resultant colonies were counted to determine CFU count in the leaves. Graph represents average CFU per leaf ± SEM of three independent experiments, N = 8. No significant differences among samples were found (two-tailed t-test).
Discussion
BCAs have promising prospects for use as an alternative to fertilizers and pesticides, allowing for the development of environmentally friendly agricultural practices. To this end, microbial BCAs have been intensely investigated in recent years (Koch et al., 2018; Tian et al., 2020). Generally, the activities of microbial BCAs are varied and can serve to promote plant vigor, or assist the plant in combating pathogens, either directly or indirectly. Exemplary BCA activities include nitrogen fixation (Li et al., 2017), phosphate solubilization (Mitra et al., 2020), secretion of metabolite-like compounds which can directly control phytopathogens (Kenawy et al., 2019), and induction of host systemic resistance that allows the plant to better combat invading pathogens (Nguvo and Gao, 2019). Various BCAs, including Trichoderma spp., are known to be systemic inducers of plant immunity (Gupta and Bar, 2020).
Trichoderma spp. are opportunistic plant colonizers that trigger host ISR and elicit rapid plant defense responses (De Meyer et al., 1998; Nawrocka and Małolepsza, 2013). Root and leaf colonization by Trichoderma can prime plant defenses, resulting in robust plant responses to subsequent pathogen attack (Elad and Kirshner, 1993; Gupta and Bar, 2020). Trichoderma spp. are potent inducers of plant ISR (Alfiky and Weisskopf, 2021).
In past years, the use of Trichoderma spp. in both research and agriculture has seen significant increases. Trichoderma spp. are interesting models to investigate when probing the interaction between plant hosts and their symbionts, with proven and anticipated successes as BCAs for the promotion of sustainable agriculture (López-Bucio et al., 2015; Guzmán-Guzmán et al., 2017). Trichoderma interacts with many plant hosts, and can thus play significant roles in both plant growth and plant resistance to pathogens in varied ecosystems (Kashyap et al., 2017). Not surprisingly, efforts are underway to understand the molecular mechanisms that influence the ability of Trichoderma to effect ISR and promote plant disease resistance.
Although BCAs have become extremely attractive agriculturally, many of them have not become agricultural market products, because their activity in promoting plant growth and/or pathogen resistance is insufficient or irreproducible under changing environments, or affected by unknown factors (Mathre et al., 1999; Harman et al., 2012). To address this issue, this work utilized genome editing to genetically manipulate the tomato host to be more receptive to Trichoderma activity.
Several MAMPs from Trichoderma have been reported to be involved in ISR induction (Hoitink et al., 2006; Vinale et al., 2008; Hermosa et al., 2012). Trichoderma spp. produced enzymes including cellulase and xylanase, are known to stimulate resistance in plants, independent of their enzymatic activity (Martinez et al., 2001). The first discovered and perhaps most extensively studied Trichoderma MAMP is EIX, which elicits plant defenses in responsive tomato and tobacco cultivars, independent of its enzymatic activity (Furman-Matarasso et al., 1999; Hanania et al., 1999; Rotblat et al., 2002). EIX induces 1-aminocyclopropane-1-carboxylic acid (ACC) synthase expression, ethylene biosynthesis, and ISR (Matarasso et al., 2005). The xylanase EIX is recognized by the transmembranal LRR pattern recognition receptors (PRRs) LeEIX1 and LeEIX2 (Ron and Avni, 2004; Bar and Avni, 2009).
Previous work has demonstrated that LeEIX1 serves as a decoy receptor, attenuating the signals propagated by LeEIX2, toward immune response activation (Bar et al., 2010, 2011). Here, we report that CRISPR/Cas9 knockout plants of LeEIX1 showed improved disease reduction (Figures 3, 5, Supplementary Figure 4) and immune response activation (Figure 4) following Trichoderma treatment. LeEIX1 knockout, as expected, did not affect agricultural quality in greenhouse settings (Figure 2, Supplementary Figures 2, 3).
Trichoderma colonization leads to transcriptional and translational changes in plant host tissues (Shoresh et al., 2010; Hermosa et al., 2012). It has been suggested that the stimulation of plant growth and activation of ISR requires Trichoderma to colonize the host plant (Nawrocka and Małolepsza, 2013). Trichoderma species are able to colonize different plant organs, altering plant metabolism by producing growth-stimulating compounds (Elad, 2000a; Harman et al., 2004). Some Trichoderma strains promote plant fitness by enhancing nutrient availability as well as resistance against various pathogens (Howell, 2003; Harman, 2006). However, it has been reported that bio-control potential might not be dependent on the level of Trichoderma colonization, but rather, on the activation of systemic resistance as a result of the initial colonization signals (Ruano-Rosa et al., 2016), as well as host immunity inducing molecules (MAMPs) secreted by Trichoderma (Ramírez-Valdespino et al., 2019). Indeed, we observed that T. harzianum T39 and NCIM, and T. longibrachiatum T166 were able to colonize the leaves of both M82 and leeix1 mutant plants to a similar extent. There were no significant differences in the amount of Trichoderma found in these plants (Figure 6), supporting the notion that although colonization may be required for ISR activation and Trichoderma based priming, differential colonization is not required for achieving differential ISR levels and disease resistance outputs.
In summary, we generated LeEIX1 CRISPR/Cas9 mutants, which retained agricultural quality in greenhouse settings, and investigated their response to Trichoderma based bio-control, finding increased disease resistance and defense responses in leeix1 mutants when compared with WT M82 plants, with no observed differences in Trichoderma plant colonization. It will be interesting to examine the performance of these plants and their response to BCAs in the future in agricultural settings.
Materials and Methods
Generation of CAS9 leeix1 Mutants
Tomato CRISPR-Cas9 editing was executed according to Xie et al. (2015). A sgRNA targeting the first exon of LeEIX1 (nucleotides 355-335 from the atg) was designed using CRISPR-P 2.0 (Liu et al., 2017), taking care not to target LeEIX2, which does not contain the PAM present in LeEIX1 is its sequence. The sgRNA was divided in two, with both oligos being amplified using an sgRNA spacer primer (sgRNA-F 5′-taggtctccCTCAAGCAGAGAAttttagagctagaaat-3′, sgRNA-R 5′-taggtctccTGAGTTGGAGTtgcaccagccgggaa-3′) and terminal specific primers containing a FokI site (L5AD5/L3AD5; see Supplementary Table 1). The products were digested with FokI, and the fragment inserted into a BsaI digested modified pUC57-cloning vector, containing a U6 promoter. The cassette was then subcloned into the binary vector pMR286 (Mily Ron plasmid collection, unpublished). Agrobacterium tumefaciens strain GV3101 harboring pMR286 containing the LeEIX1 sgRNA was used to transform Solanum lycopersicum cv M82, according to standard practice (McCormick, 1991). Genomic DNA was extracted from obtained transformant plants, and used as a template for LeEIX1 sgRNA flanking-fragment amplification. PCR fragments were verified to originate from edited individuals by sequencing (see genotyping primers in Supplementary Table 1). Relevant transgenic lines were selfed, and the resulting T2 plants were re-analyzed. Leaves 4–5 of 6-week-old plants were used for assays.
Plant Materials and Growth Conditions
S. lycopersicum cv M82 and homozygous T3 leeix1 independent CRISPR lines leeix1-14 and leeix1-b5 were grown from seeds in soil (Green Mix; EvenAri, Ashdod, Israel) in a growth chamber, under long-day conditions (16 h:8 h, light:dark) at 24°C. Plants from both independent CRISPR lines were used in assays.
Fungal Infection
B. cinerea isolate BcI16 and S. sclerotiorum isolate Scl5 were cultured on potato dextrose agar (PDA) (Difco Lab) plates and incubated at 22 ± 4°C for 5–7 days. B. cinerea conidia were harvested in 1 mg ml−1 glucose and 1 mg ml−1 K2HPO4 and filtered through cheesecloth. Conidia concentration was adjusted to 106 cells ml−1 using a haemocytometer. Leaves 4–6 from 5 to 6-week old tomato plants were excised and immediately placed in humid chambers. Each tomato leaflet was inoculated with two droplets of 10 μL suspension. For S. sclerotiorum, uniform mycelial plugs (5 mm diameter) were taken using a cork-borer from colony margins and placed mycelial side down on the adaxial surface of each leaf. Inoculated leaves were kept in a humid growth chamber at 21°C.
O. neolycopersici was isolated from young leaves of 4–6 week old tomato plants grown in a greenhouse during the winter. Conidia were collected by rinsing infected leaves with sterile water. The concentrations of conidial suspensions were determined under a light microscope using a hemocytometer. Suspensions were adjusted to 104 ml−1, and sprayed onto 5–6-week old tomato plants (5 ml per plant). Suspensions were sprayed within 10–15 min of initial conidia collection, with a hand-held spray bottle, and plants were left to dry in an open greenhouse for up to 30 min. Inoculated plants were kept in a humid growth chamber at 21°C. In all cases, controls consisted of leaves treated with water/buffer without the inoculation of pathogen. The diameter of the necrotic lesions or % of infected leaf tissue was measured 3–10 days post inoculation, as indicated, using ImageJ.
Oxidative Burst (ROS) Measurement
ROS measurement was performed as previously described (Leibman-Markus et al., 2017). 0.5 cm diameter leaf disks were harvested from leaves 4 to 6 of 5–6 week old M82 and leeix1 plants. Disks were floated in a white 96-well plate (SPL Life Sciences, Korea) containing 250 μl distilled water for 4–6 h at room temperature. The water was then removed, and a ROS measurement reaction containing either 1 μg /mL EIX or water (mock) was added. Light emission was immediately measured using a luminometer (GloMax® Discover, Promega, USA). EIX was purified as previously described (Anand et al., 2021).
Ethylene Measurement
Ethylene production was measured as previously described (Leibman-Markus et al., 2017). 0.9 cm diameter leaf disks were harvested from leaves 4 to 6 of 5–6 week old M82 and leeix1 tomato plants. Disks were washed in water for 1–2 h. Every six disks were sealed in a 10 mL flask containing 1 ml assay medium with 1 μg /mL EIX for 5 h at room temperature. Ethylene production was measured by gas chromatography (Varian 3350, Varian, California, USA).
Electrolyte Leakage Measurement
0.9 cm diameter leaf disks were harvested from leaves 4 to 6 of 5–6 week old M82 and leeix1 plants. Disks were washed in 50 mL water for 3 h. For each sample, five disks were floated in a 12-well plate containing 1 mL of water with 1 μg /mL EIX, adaxial surface down, at room temperature, with agitation (75 rpm). Conductivity was measured in the water solution after 24 h incubation using a conductivity meter (EUTECH instrument con510).
Development and Growth Measurements
Growth measurements were performed as previously described by Gur et al. (2010). Plant vegetative weight was determined by excising the plant at the base, after harvesting the fruits. Total fruit yield per plant included both the red and the green fruits. Concentration of total soluble sugars (BRIX percentage) was measured using a digital refractometer with a range of BRIX 0–85 ± 0.2%, from a random sample of 5 red fruits per plant. Harvest index (HI) was calculated as the ratio between the total yield and total biomass.
Trichoderma Treatment and Colonization Assay
T. harzianum isolates were cultured on potato dextrose agar (PDA) (Difco Lab) plates and incubated at 25°C for 5–7 days. Spores were harvested in water and filtered through cheesecloth. Conidia concentration was adjusted to 106 cells ml−1 using a hemocytometer. Conidia suspensions were sprayed onto plants of desired genotypes. For fungal infections, plants were infected as described above, 3 days after Trichoderma treatment. For colonization assays, 2 weeks after Trichoderma treatment leaves were harvested and washed in water with thorough shaking. Leaves were then removed and sterilized in bleach for 2 min, and ground with a mortar and pestle. One gram of the ground tissue was re-suspended in 1 mL water. Serial dilutions were plated on PDA plates containing 0.02% of rose bengal dye as a selective agent (Elad et al., 1981). Resultant colonies were counted to determine CFU count in the leaves.
Statistics and Reproducibility
All data are presented as average ± SEM. Differences between two groups were analyzed for statistical significance using a two tailed t-test with Welch's correction for unequal variances, or Holm-Sidak correction for multiple t-tests, where applicable. Differences among three groups or more were analyzed for statistical significance using one-way ANOVA. Regular ANOVA was used for groups with equal variances, and Welch's ANOVA for groups with unequal variances. When a significant result for a group in an ANOVA was returned, significance in differences between the means of different samples in the group were assessed using a post-hoc test. The Tukey test was employed for samples with equal variances when the mean of each sample was compared to the mean of every other sample. The Bonferroni test was employed for samples with equal variances when the mean of each sample was compared to the mean of a control sample. The Dunnett test was employed for samples with unequal variances. All statistical analyses were conducted using Prism8™. All experiments were conducted in at least three biologically independent repeats. The number of replicates is indicated for each experiment in each figure legend.
Data Availability Statement
The datasets presented in this study can be found in online repositories. The names of the repository/repositories and accession number(s) can be found in the article/Supplementary Material.
Author Contributions
YE and MB: conceptualization and funding. ML-M, RG, LP, OG, and DR-D: experimentation. ML-M, RG, YE, and MB: analysis. ML-M, RG, LP, YE, and MB: manuscript. All authors contributed to the article and approved the submitted version.
Funding
This work was supported by the Chief Scientist of the Israeli Ministry of Agriculture and rural development, MOAG, Grant No. 20-02-0075.
Conflict of Interest
The authors declare that the research was conducted in the absence of any commercial or financial relationships that could be construed as a potential conflict of interest.
Acknowledgments
The IL-7-5 35S::LeEIX2 line was kindly provided by Prof. Adi Avni, Tel-Aviv University. We thank the Bar group members for continuous discussion and support.
Supplementary Material
The Supplementary Material for this article can be found online at: https://www.frontiersin.org/articles/10.3389/ffunb.2021.678840/full#supplementary-material
References
Alfiky, A., and Weisskopf, L. (2021). Deciphering Trichoderma–plant–pathogen interactions for better development of biocontrol applications. J. Fungi 7:61. doi: 10.3390/jof7010061
Anand, G., Leibman-Markus, M., Elkabetz, D., and Bar, M. (2021). Method for the production and purification of plant immuno-active xylanase from Trichoderma. Int. J. Mol. Sci. 22:4214. doi: 10.3390/ijms22084214
Bailey, B. A., Dean, J. F. D., and Anderson, J. D. (1990). An ethylene biosynthesis-inducing endoxylanase elicits electrolyte leakage and necrosis in Nicotiana tabacum cv Xanthi leaves. Plant Physiol. 94, 1849–1854. doi: 10.1104/pp.94.4.1849
Bar, M., and Avni, A. (2009). EHD2 inhibits ligand-induced endocytosis and signaling of the leucine-rich repeat receptor-like protein LeEIX2. Plant J. 59, 600–611. doi: 10.1111/j.1365-313X.2009.03897.x
Bar, M., Sharfman, M., and Avni, A. (2011). LeEIX1 functions as a decoy receptor to attenuate LeEIX2 signaling. Plant Signal. Behav. 6, 455–457. doi: 10.4161/psb.6.3.14714
Bar, M., Sharfman, M., Ron, M., and Avni, A. (2010). BAK1 is required for the attenuation of ethylene-inducing xylanase (Eix)-induced defense responses by the decoy receptor LeEIX1. Plant J. 63, 791–800. doi: 10.1111/j.1365-313X.2010.04282.x
De Meyer, G., Bigirimana, J., Elad, Y., and Hofte, M. (1998). Induced systemic resistance in Trichoderma harzianum T39 biocontrol ofBotrytis cinerea. Eur. J. Plant Pathol. 104, 279–286. doi: 10.1023/A:1008628806616
Elad, Y. (2000a). Biological control of foliar pathogens by means of Trichoderma harzianum and potential modes of action. Crop Protect. 19, 709–714. doi: 10.1016/S0261-2194(00)00094-6
Elad, Y. (2000b). Trichoderma harzianum T39 preparation for biocontrol of plant diseases-control of Botrytis cinerea, Sclerotinia sclerotiorum and Cladosporium fulvum. Biocontrol Sci. Technol. 10, 499–507. doi: 10.1080/09583150050115089
Elad, Y., Chet, I., and Henis, Y. (1981). A selective medium for improving quantitative isolation of Trichoderma spp. from soil. Phytoparasitica 9, 59–67. doi: 10.1007/BF03158330
Elad, Y., and Kapat, A. (1999). The role of Trichoderma harzianum protease in the biocontrol of Botrytis cinerea. Eur. J. Plant Pathol. 105, 177–189. doi: 10.1023/A:1008753629207
Elad, Y., and Kirshner, B. (1993). Survival in the phylloplane of an introduced biocontrol agent (Trichoderma harzianum) and populations of the plant pathogen Botrytis cinerea as modified by abiotic conditions. Phytoparasitica 21, 303–313. doi: 10.1007/BF02981048
Elbaz, M., Avni, A., and Weil, M. (2002). Constitutive caspase-like machinery executes programmed cell death in plant cells. Cell Death Differ. 9, 726–733. doi: 10.1038/sj.cdd.4401030
Eshed, Y., Abu-Abied, M., Saranga, Y., and Zamir, D. (1992). Lycopersicon esculentum lines containing small overlapping introgressions from L. pennellii. Theor. Appl. Genetics 83, 1027–1034. doi: 10.1007/BF00232968
Furman-Matarasso, N., Cohen, E., Du, Q., Chejanovsky, N., Hanania, U., and Avni, A. (1999). A point mutation in the ethylene-inducing xylanase elicitor inhibits the beta-1-4-Endoxylanase activity but not the elicitation activity. Plant Physiol. 121, 345–352. doi: 10.1104/pp.121.2.345
Gupta, R., and Bar, M. (2020). Plant Immunity, Priming, and Systemic Resistance as Mechanisms for Trichoderma spp. Biocontrol. Singapore: Springer, 81–110.
Gur, A., Semel, Y., Osorio, S., Friedmann, M., Seekh, S., Ghareeb, B., et al. (2010). Yield quantitative trait loci from wild tomato are predominately expressed by the shoot. Theor. Appl. Genet. 122, 405–420. doi: 10.1007/s00122-010-1456-9
Guzmán-Guzmán, P., Alemán-Duarte, M. I., Delaye, L., Herrera-Estrella, A., and Olmedo-Monfil, V. (2017). Identification of effector-like proteins in Trichoderma spp. and role of a hydrophobin in the plant-fungus interaction and mycoparasitism. BMC Genetics 18:16. doi: 10.1186/s12863-017-0481-y
Hanania, U., and Avni, A. (1997). High affinity binding site for ethylene inducing xylanase elicitor on Nicotiana tabacum membranes. Plant J. 12, 113–120. doi: 10.1046/j.1365-313X.1997.12010113.x
Hanania, U., Furman-Matarasso, N., Ron, M., and Avni, A. (1999). Isolation of a novel SUMO protein from tomato that suppresses EIX-induced cell death. Plant J. 19, 533–541. doi: 10.1046/j.1365-313X.1999.00547.x
Harman, G. E. (2006). Overview of mechanisms and uses of Trichoderma spp. Phytopathology 96, 190–194. doi: 10.1094/PHYTO-96-0190
Harman, G. E., Herrera-Estrella, A. H., Horwitz, B. A., and Lorito, M. (2012). Special issue: Trichoderma–from basic biology to biotechnology. Microbiology 158, 1–2. doi: 10.1099/mic.0.056424-0
Harman, G. E., Howell, C. R., Viterbo, A., Chet, I., and Lorito, M. (2004). Trichoderma species - opportunistic, avirulent plant symbionts. Nat. Rev. Microbiol. 2, 43–56. doi: 10.1038/nrmicro797
He, D. C., Zhan, J. S., and Xie, L. H. (2016). Problems, challenges and future of plant disease management: from an ecological point of view. J. Integr. Agric. 15, 705–715. doi: 10.1016/S2095-3119(15)61300-4
Hermosa, R., Viterbo, A., Chet, I., and Monte, E. (2012). Plant-beneficial effects of Trichoderma and of its genes. Microbiology 158, 17–25. doi: 10.1099/mic.0.052274-0
Hoitink, H. A. J., Madden, L. V., and Dorrance, A. E. (2006). Systemic resistance induced by Trichoderma spp.: interactions between the host, the pathogen, the biocontrol agent, and soil organic matter quality. Phytopathology 96, 186–189. doi: 10.1094/PHYTO-96-0186
Howell, C. R. (2003). Mechanisms employed by Trichoderma species in the biological control of plant diseases: the history and evolution of current concepts. Plant Dis. 87, 4–10.
Hulo, N., Bairoch, A., Bulliard, V., Cerutti, L., Cuche, A., De Castro, E., et al. (2008). The 20 years of PROSITE. Nucleic Acids Res. 36, 245–249. doi: 10.1093/nar/gkm977
Juroszek, P., and von Tiedemann, A. (2011). Potential strategies and future requirements for plant disease management under a changing climate. Plant Pathol. 60, 100–112. doi: 10.1111/j.1365-3059.2010.02410.x
Kapat, A., Zimand, G., and Elad, Y. (1998). Effect of two isolates of Trichoderma harzianum on the activity of hydrolytic enzymes produced by Botrytis cinerea. Physiol. Mol. Plant Pathol. 52, 127–137. doi: 10.1006/pmpp.1997.0140
Kashyap, P. L., Rai, P., Srivastava, A. K., and Kumar, S. (2017). Trichoderma for climate resilient agriculture. World J. Microbiol. Biotechnol. 33:155. doi: 10.1007/s11274-017-2319-1
Kenawy, A., Dailin, D. J., Abo-Zaid, G. A., Malek, R. A., Ambehabati, K. K., Zakaria, K. H. N., et al. (2019). Biosynthesis of Antibiotics by PGPR and Their Roles in Biocontrol of Plant Diseases. Singapore: Springer.
Koch, E., Ole Becker, J., Berg, G., Hauschild, R., Jehle, J., Köhl, J., et al. (2018). Biocontrol of plant diseases is not an unsafe technology! J. Plant Dis. Protect. 125, 121–125. doi: 10.1007/s41348-018-0158-4
Kuc, J. (1987). “Plant immunization and its applicability for disease control,” in Innovative Approaches to Plant Disease Control, ed. I. Chet (New York, NY: Wiley), 255–274.
Leibman-Markus, M., Schuster, S., and Avni, A. (2017). “LeEIX2 Interactors' analysis and EIX-mediated responses measurement,” in Plant Pattern Recognition Receptors: Methods and Protocols, eds. L. Shan and P. He (New York, NY: Springer New York), 167–172.
Leong, W. H., Teh, S. Y., Hossain, M. M., Nadarajaw, T., Zabidi-Hussin, Z., Chin, S. Y., et al. (2020). Application, monitoring and adverse effects in pesticide use: the importance of reinforcement of good agricultural practices (GAPs). J. Environ. Manage. 260:109987. doi: 10.1016/j.jenvman.2019.109987
Li, H. B., Singh, R. K., Singh, P., Song, Q. Q., Xing, Y. X., Yang, L. T., et al. (2017). Genetic diversity of nitrogen-fixing and plant growth promoting Pseudomonas species isolated from sugarcane rhizosphere. Front. Microbiol. 8:1268. doi: 10.3389/fmicb.2017.01268
Liu, H., Ding, Y., Zhou, Y., Jin, W., Xie, K., and Chen, L.-L. (2017). CRISPR-P 2.0: an improved CRISPR/Cas9 tool for genome editing in plants. Mol. Plant 10, 530–532. doi: 10.1016/j.molp.2017.01.003
López-Bucio, J., Pelagio-Flores, R., and Herrera-Estrella, A. (2015). Trichoderma as biostimulant: exploiting the multilevel properties of a plant beneficial fungus. Sci. Hortic. 196, 109–123. doi: 10.1016/j.scienta.2015.08.043
Martinez, C., Blanc, F., Le Claire, E., Besnard, O., Nicole, M., and Baccou, J. C. (2001). Salicylic acid and ethylene pathways are differentially activated in melon cotyledons by active or heat-denatured cellulase from Trichoderma longibrachiatum. Plant Physiol. 127, 334–344. doi: 10.1104/pp.127.1.334
Matarasso, N., Schuster, S., and Avni, A. (2005). A novel plant cysteine protease has a dual function as a regulator of 1-aminocyclopropane-1-carboxylic acid synthase gene expression. Plant Cell 17, 1205–1216. doi: 10.1105/tpc.105.030775
Mathre, D. E., Cook, R. J., and Callan, N. W. (1999). From discovery to use: traversing the world of commercializing biocontrol agents for plant disease control. Plant Dis. 83, 972–983. doi: 10.1094/PDIS.1999.83.11.972
Maymon, M., Minz, D., Barbul, O., Zveibil, A., Elad, Y., and Freeman, S. (2004). Note: identification of Trichoderma biocontrol isolates to clades according to ap-PCR and ITS sequence analyses. Phytoparasitica 32, 370–375. doi: 10.1007/BF02979848
McCormick, S. (1991). “Transformation of tomato with Agrobacteriumtumifaciens,” in Plant Tissue Culture Manual, ed. K. Lindsey (Dordrecht, The Netherlands: Kluwer Academic Publishers), 1–9.
Meller-Harel, Y. M., Mehari, Z. H., Rav-David, D., and Elad, Y. (2014). Systemic resistance to gray mold induced in tomato by benzothiadiazole and Trichoderma harzianum T39. Phytopathology 104, 150–157. doi: 10.1094/PHYTO-02-13-0043-R
Mitra, D., Andelkovi,ć, S., Panneerselvam, P., Senapati, A., Vasi,ć, T., Ganeshamurthy, A. N., et al. (2020). Phosphate-solubilizing microbes and biocontrol agent for plant nutrition and protection: current perspective. Commun. Soil Sci. Plant Anal. 51, 645–657. doi: 10.1080/00103624.2020.1729379
Molla, K. A., Karmakar, S., and Islam, M. T. (2020). Wide Horizons of CRISPR-Cas-Derived Technologies for Basic Biology, Agriculture, and Medicine. New York, NY: Humana, 1–23.
Nawrocka, J., and Małolepsza, U. (2013). Diversity in plant systemic resistance induced by Trichoderma. Biol. Control 67, 149–156. doi: 10.1016/j.biocontrol.2013.07.005
Nguvo, K. J., and Gao, X. (2019). Weapons hidden underneath: bio-control agents and their potentials to activate plant induced systemic resistance in controlling crop Fusarium diseases. J. Plant Dis. Protect. 126, 177–190. doi: 10.1007/s41348-019-00222-y
Paulitz, T. C., and Matta, A. (2000). “The role of the host in biological control of diseases,” in Integrated Pest and Disease Management in Greenhouse Crops, eds R. Albajes, M. L. Gullino, J. C. van Lenteren, and Y. Elad (Wageningen: Kluwer Academic Publisher).
Perazzolli, M., Dagostin, S., Ferrari, A., Elad, Y., and Pertot, I. (2008). Induction of systemic resistance against Plasmopara viticola in grapevine by Trichoderma harzianum T39 and benzothiadiazole. Biol. Control 47, 228–234. doi: 10.1016/j.biocontrol.2008.08.008
Ramírez-Valdespino, C. A., Casas-Flores, S., and Olmedo-Monfil, V. (2019). Trichoderma as a model to study effector-like molecules. Front. Microbiol. 10:1030. doi: 10.3389/fmicb.2019.01030
Ron, M., and Avni, A. (2004). The receptor for the fungal elicitor ethylene-inducing xylanase is a member of a resistance-like gene family in tomato. Plant Cell 16, 1604–1615. doi: 10.1105/tpc.022475
Ron, M., Kantety, R., Martin, G. B., Avidan, N., Eshed, Y., Zamir, D., et al. (2000). High-resolution linkage analysis and physical characterization of the EIX-responding locus in tomato. Theor. Appl. Genet. 100, 184–189. doi: 10.1007/s001220050025
Rotblat, B., Enshel-Seijffers, D., Gershoni, M. J., Schuster, S., and Avni, A. (2002). Identification of an essential component of the elicitation active site of the EIX protein elicitor. Plant J. 32, 1–7. doi: 10.1046/j.1365-313X.2002.01490.x
Ruano-Rosa, D., Prieto, P., Rincón, A. M., Gómez-Rodríguez, M. V., Valderrama, R., Barroso, J. B., et al. (2016). Fate of Trichoderma harzianum in the olive rhizosphere: time course of the root colonization process and interaction with the fungal pathogen Verticillium dahliae. BioControl 61, 269–282. doi: 10.1007/s10526-015-9706-z
Sequeira, L. (1983). Mechanisms of induced resistance in plants. Annu. Rev. Microbiol. 37, 51–79. doi: 10.1146/annurev.mi.37.100183.000411
Sharon, A., Bailey, B. A., McMurtry, J. P., Taylor, R., and Anderson, J. D. (1992). Characteristics of ethylene biosynthesis-inducing xylanase movement in tobacco leaves. Plant Physiol. 100, 2059–2065. doi: 10.1104/pp.100.4.2059
Shoresh, M., Harman, G. E., and Mastouri, F. (2010). Induced systemic resistance and plant responses to fungal biocontrol agents. Annu. Rev. Phytopathol. 48, 21–43. doi: 10.1146/annurev-phyto-073009-114450
Tian, L., Lin, X., Tian, J., Ji, L., Chen, Y., Tran, L.-S. P., et al. (2020). Research advances of beneficial microbiota associated with crop plants. Int. J. Mol. Sci. 21:1792. doi: 10.3390/ijms21051792
Vinale, F., Sivasithamparam, K., Ghisalberti, E. L., Marra, R., Woo, S. L., and Lorito, M. (2008). Trichoderma-plant-pathogen interactions. Soil Biol. Biochem. 40, 1–10. doi: 10.1016/j.soilbio.2007.07.002
Vitti, A., Sofo, A., Scopa, A., and Nuzzaci, M. (2015). “Sustainable agricultural practices in disease defence of traditional crops in Southern Italy: the case study of tomato cherry protected by Trichoderma harzianum T-22 against cucumber mosaic virus (CMV),” in The Sustainability of Agro-Food and Natural Resource Systems in the Mediterranean Basin, ed. A. Vastola (Cham: Springer International Publishing), 133–143.
Xie, K., Minkenberg, B., and Yang, Y. (2015). Boosting CRISPR/Cas9 multiplex editing capability with the endogenous tRNA-processing system. Proc. Natl. Acad. Sci. U. S. A. 112, 3570–3575. doi: 10.1073/pnas.1420294112
Keywords: xylanase, LeEIX1, Trichoderma, bio-control, tomato, gene-editing
Citation: Leibman-Markus M, Gupta R, Pizarro L, Gershony O, Rav-David D, Elad Y and Bar M (2021) Gene Editing of the Decoy Receptor LeEIX1 Increases Host Receptivity to Trichoderma Bio-Control. Front. Fungal Biol. 2:678840. doi: 10.3389/ffunb.2021.678840
Received: 10 March 2021; Accepted: 20 May 2021;
Published: 21 June 2021.
Edited by:
Prasun K. Mukherjee, Bhabha Atomic Research Centre (BARC), IndiaReviewed by:
Praveen Kumar Verma, National Institute of Plant Genome Research (NIPGR), IndiaKutubuddin A. Molla, National Rice Research Institute (ICAR), India
Copyright © 2021 Leibman-Markus, Gupta, Pizarro, Gershony, Rav-David, Elad and Bar. This is an open-access article distributed under the terms of the Creative Commons Attribution License (CC BY). The use, distribution or reproduction in other forums is permitted, provided the original author(s) and the copyright owner(s) are credited and that the original publication in this journal is cited, in accordance with accepted academic practice. No use, distribution or reproduction is permitted which does not comply with these terms.
*Correspondence: Maya Bar, bWF5YWJhciYjeDAwMDQwO3ZvbGNhbmkuYWdyaS5nb3YuaWw=
†Present address: Lorena Pizarro, Institute of Agri-Food, Animal and Environmental Sciences, Universidad de O'Higgins, Rancagua, Chile