- 1Forest Risk Research Centre, Faculty of Forestry and Wood Sciences, Czech University of Life Sciences Prague, Prague, Czechia
- 2Department of Forest Protection and Entomology, Faculty of Forestry and Wood Sciences, Czech University of Life Sciences Prague, Prague, Czechia
- 3Department of Silviculture, Faculty of Forestry and Wood Sciences, Czech University of Life Sciences Prague, Prague, Czechia
- 4Department of Forest Management and Remote Sensing, Faculty of Forestry and Wood Sciences, Czech University of Life Sciences, Prague, Czechia
Bark beetle outbreaks have become increasingly prevalent and intense, causing widespread tree mortality and altering forest ecosystems globally. In this study, we investigate the dynamics between tree root systems of the Norway spruce and ectomycorrhizal fungi in the aftermath of bark beetle-induced tree mortality, focusing on the changes in density of vital and non-vital mycorrhizal tips. The survey was carried out in the Bohemian Switzerland National Park, Czechia. The sampling sites were chosen based on polygon layers delineating individual years of mortality status, obtained by PlanetLab imagery for 2018–2022, classified by Support Vector Machine, a machine learning tool (SVM). Fieldwork involved the collection of soil and root samples. Mycorrhizal tips were examined and counted using a stereomicroscope. Soil pH and root dry weight were determined. Wood decay fungi were identified using a comprehensive approach, combining macroscopic examination with genetic analysis. Due to the favorable conditions, the density of vital mycorrhizal tips (VM) on living trees gradually increased, peaking in the 2nd and 3rd years after the surrounding forest decay. VM on bark beetle snags was significantly lower compared to living trees, with minimal variation over time. The dry biomass of fine roots was significantly greater in living trees. Fine root abundance showed a slight decrease over time in living and dead trees; however, there was a lack of statistical significance. Most of the fine root biomass decomposes within the first half year after tree death. This might be influenced by wood decay fungi identified on dead trees, including genera Armillaria, Fomitopsis, and Pleurotus ostreatus. Overall, the study provides insights into the complex dynamics of mycorrhizal associations, root system biomass, and wood decay fungi in the aftermath of bark beetle-induced tree mortality. Our study demonstrates that mycorrhizal activity increases in trees surviving a bark beetle disturbance, and they probably become another ECM refugia. This research contributes valuable insights into the ecological consequences of bark beetle infestations on below-ground interactions, offering a deeper understanding of forest ecosystem responses to widespread tree mortality. Such knowledge is essential for developing effective strategies to manage and mitigate the ecological impacts of bark beetle outbreaks in forested environments.
Introduction
Dead trees play a key role in natural forest ecosystems (Rondeux and Sanchez, 2010). Since they are associated with a wide range of microorganisms, animals, and fungi, dead trees contribute significantly to forest biodiversity (Stokland et al., 2012). Deadwood also binds carbon and other biogenic elements, allowing them to return gradually to forest soils (Russell et al., 2015). In general, the emergence and presence of dead trees strongly influence the dynamics of forest ecosystems in the temperate zone (Král et al., 2018). Production-oriented silviculture, which has an over 300-year-long tradition in Central Europe, changed the composition of the forest to spruce-dominated stands and has practically ruled out the presence of dead trees (Dieler et al., 2017). The occurrence of dead trees was limited only to small and isolated forest reserves. This situation changed in the 1990s when non-intervention management began to be implemented in large Central European protected areas (Parviainen et al., 2000). This led to an increase in snag deadwood in relatively large areas, such as the Bavarian Forest (Müller et al., 2008), the Bohemian Forest (Svoboda, 2005) or the Tatra Mountains (Potterf et al., 2019), due to the European spruce bark beetle Ips typographus (L.). The spruce bark beetle is a key species that maintains spatial, structural, and age heterogeneity of the spruce forests and provides higher resistance and resilience against most of disturbance agents (Hlásny et al., 2021a). Over the past 20 years, however, there has been a doubling in bark beetle-induced forest disturbances on a Europe-wide level (Patacca et al., 2022), Central Europe’s epicenter. As a result of the ongoing bark beetle outbreak in the Czech Republic, there has been a dramatic increase in dead trees extending beyond protected areas into a large proportion of production forests (Hlásny et al., 2021b; Washaya et al., 2024). Despite the apparent positive impact on biodiversity and carbon storage (Vítková et al., 2018), the increased presence of dead trees raises several questions concerning their stability and, thus also, the safety of the public, forestry personnel, machinery, and infrastructure (Mortimer and Kane, 2004; Schmidlin, 2009).
Degradation processes in the spruce deadwood lead to a gradual change in the physical properties of snags (Löwe et al., 2022; Jelonek et al., 2020), eventually resulting in their collapse. Depending on the tree’s species and size, climatic conditions, and the management of its surroundings, the tree collapses usually within 3 to 20 years after its death (Oettel et al., 2023; Gärtner et al., 2023). Following death by fire, the average time for the spruce to fall is 32 years to collapse (Aakala, 2010). However, trees killed by bark beetles collapse on average after only 13 years (Gärtner et al., 2023). With the Norway spruce, Picea abies (L.) Karst., in the boreal areas of Europe, an average time to fall of 12 to 27 years was determined (Aakala, 2010); however, the cause of death was not considered. In the case of the lodgepole pine, Pinus contorta Douglas ex Loudon, killed by Dendroctonus ponderosae Hopkins, half of the snags fell within 15 to 20 years (Rhoades et al., 2020). According to Rhoades et al. (2020), 53.2% of the dead lodgepole pines fell due to butt rot (i.e., breakage at or below ground level with visible signs of decay), 14.3% due to bole snap (i.e., breakage at 1 m above ground or higher) and 32.5% of trees were tipped up (i.e., trees remained attached to exposed roots and soil mound). Decaying root systems likely cause the decrease in stability of bark beetle snags and their resulting tip-up. However, the long persistence of dead trees, in the case of the spruce, even several decades (Přívětivý et al., 2018), suggests that root degradation takes longer than might be expected.
The spruce’s root system dynamics are mainly determined by soil conditions, such as pH or moisture (Puhe, 2003). Suboptimal soil conditions stimulate the formation of mycorrhizal associations, which facilitate tree nutrition (Cheng et al., 2016). Mycorrhizal fungi form symbiotic connections with most terrestrial plants (Read, 1998). The relationship between a mycorrhizal fungus and its plant symbiont is based on a mutually beneficial exchange of nutrients. The fungus provides minerals (especially nitrogen and phosphorus); in return, the plant provides photosynthetically produced carbon compounds (saccharides). Simultaneously, the hyphal threads of the mycorrhizal fungus provide the plant with a significantly increased surface area where nutrient ions can be absorbed (Maheshwari, 2008). Within a functional mycorrhizal symbiosis, the above-ground biomass and the root network of the host plant increase considerably compared to plants without mycorrhizal associations. This results from an adequate supply of nutrients and water through hyphal threads that are three to five times thinner than root hairs and thus have access to areas of the soil that remain inaccessible to root hairs (Graf et al., 2018). For example, measurements of mycorrhizal hyphae associated with roots of Pinus sylvestris L. have yielded values of 10–80 m of hyphae per 1 cm of root length (Read and Boyd, 1986). This results in a vastly increased surface area and, consequently, a much greater volume of soil that the host plant can exploit (Jansen, 1992). Thus, apart from their significant role in plant nutrition and their influence on species composition of plant communities, mycorrhizal fungi also affect soil aggregation and soil stability by weaving together free soil particles and binding them with sticky substances, such as saccharides and other extracellular metabolites (Rillig and Mummey, 2006; Enkhtuya and Vosátka, 2005). Based on these findings, it can be supposed that the presence of mycorrhizae in a tree’s root system influences its mechanical stability within the soil. The presence of mycorrhizae is, therefore, not only an indicator of a tree’s vitality but also of its mechanical stability.
Ectomycorrhizal fungi (ECM) encompass a great diversity of species. Tens of species can be found even in small forest monocultures (Bruns, 1995). In coniferous forests, they represent roughly one-third of the microbial biomass (Högberg and Högberg, 2002). It is supposed that the activity of an ECM species is directly proportional to the number of mycorrhizal tips (Landeweert et al., 2003). The vitality of mycorrhizae on a given root system can be determined by comparing the numbers of vital and non-vital ECM tips (Pešková, 2007; Corcobado et al., 2014). The number of ECM tips per milligram of fine root biomass is constant. Trees increase nutrient uptake by increasing fine root production, hence the number of ECM tips (Helmisaari et al., 2009). Studies of fine roots and mycorrhizae of the Norway spruce were conducted by Blanck et al. (1995), Feil et al. (1988), Majdi (2001), Palátová (2004), Gaul et al. (2008), Eldhuset et al. (2012), and Konôpka et al., (2013). Morphological properties of ECM roots provide a good indication of a tree’s adaptation to local conditions. These include both absolute markers (e.g., root length, root surface area, root diameter, or number of ECM tips) and relative markers, usually related to dry root biomass (e.g., specific root length, surface area and diameter, and specific number of ECM tips) (Peterson et al., 2004; Ostonen et al., 2013). Specific root length (i.e., length divided by mass) is probably used most commonly. The most significant changes of specific length in reaction to changes in the soil environment can be observed with fine roots. An increase in specific root length gives access to a greater volume of soil and is thus one possible adaptation of plants ensuring sufficient nutrient supply (Ostonen et al., 2007).
The changes that occur over time in a root system following a tree death by bark beetles have not been directly quantified. Bark beetle disturbances are known to lead to wide-ranging changes of forest ecosystems, both on the macroscopic and microscopic levels. I. typographus is the major disturbance agent in Norway spruce dominated forests, causing ecosystem changes in case of eruptive outbreaks. Ips typographus occurs almost the entire Palearctic region: from mountaineous regions in the south to the lowlands in the north. According to the climatic region and current condition, I. typographus can have one to three generations during vegetation season. The tree is colonized by a pheromone-mediated mass attack of beetles. If successful, the infested tree dies after a short period (Lieutier et al., 2004; Wermelinger, 2004; Kausrud et al., 2012; Vega and Hofstetter, 2015, Hlásny et al., 2021a). A gradual discoloration and shedding of needles occur when a tree is infested (Kautz et al., 2022). This results in a gradual decrease in the supply of photosynthetic products to the soil (Štursová et al., 2014). On a macroscopic scale, a fungal community reacts to bark beetle-induced tree dieback by a significant decrease in overall fungal biomass and by changes in its species composition. While mycorrhizal species dominate at first, the relative abundance of saprotrophic species gradually increases. The overall species composition, however, remains constant. This suggests that mycorrhizal species can persist for a long time, even without symbiotic trees. Some species, such as Russulales, can switch from a mycorrhizal to a saprotrophic mode, thus persisting in the community and contributing to wood decomposition (Veselá et al., 2019).
Following the death of the above-ground part of a tree, the root system begins to disintegrate. At first, mycorrhizal community changes, followed by the decomposition of root hairs and fine roots. Later, more massive roots are also decomposed. This work compares the root systems of bark beetle snags at different stages after dying to the root systems of nearby living trees. The work aimed to determine the extent of root system degradation over 5 years from tree death using ECM tip counts as a degradation marker. Concomitantly, the rate at which mycorrhizal parameters change following tree death was investigated. We addressed these main research questions: (i) How long do VM and NVM persist on dead trees? We suppose the immediate decline of the VM after tree death and the successive disappearance of the NVM. (ii) The second research question is connected to the stability of the dead trees through the root system. We assume that roots smaller than 2 mm will degrade quickly following the tree’s dead. (iii) The last question is the species representation of wood fungi in the decomposition process in different years after a tree dead.
Materials and methods
The study was carried out in the Bohemian Switzerland National Park located in northwest Czechia on the eastern bank of the river Elbe, on the borders of Saxony (DE). This is a warm to temperate area with average yearly temperatures between 6 and 8°C and annual precipitation of 800 mm (Tolasz et al., 2007). The 79 km2 area has a high relief and is mostly forested. Relict pinewoods occupy sandstone platforms, while lower areas are populated by various cold-adapted plant communities.
However, most of the national park is covered with non-native pure and even-aged stands of Norway spruce – Picea abies (L.) H. Karst. In 2018, an outbreak of the European spruce bark beetle, Ips typographus (L.), occurred in the area. The infestation began in the warmer western areas and gradually spread across the national park. During the first 2 years of the outbreak, partial sanitation harvests were undertaken. From 2019 onwards, however, all interventions aimed at containing the spread of the bark beetle were abandoned, due to possible massive clear-cuts resulting from a bark beetle intervention, which are unsuitable in a national park. By the end of 2022, 19,953 ha of spruce forest had been destroyed by the bark beetle in the national park and surroundings (Washaya et al., 2024). In 2022, a large wildfire broke out in the western regions of the park (Kudláčková et al., 2023). The area affected by fire was excluded when selecting sites for sample collection.
Due to the extensive large-scale changes in the national park forests, remote sensing techniques were used to monitor the development of bark beetle disturbance. The chronosequence of dead tree areas was provided from an inventory of the mortality dynamics. The data from PlanetScope satellites were used; they were obtained for years 2018–2022 (Figure 1) at the end of the thermal growing season (mainly September and the first half of October, depending on the cloud coverage), 1 pixel was referred to 3 × 3 meters. The rasters were processed by the Support Vector Machine (SVM) machine learning algorithm in ArcGIS Pro for every period dataset, and the spread and enlargement of attacked trees were delineated based on the color change in the given area. The training and validation samples were supported by visual verification corresponding to available aerial imagery in 2020 and 2021. A complete description of the methods, including the classification details, can be found in Matějčíková et al. (2024).
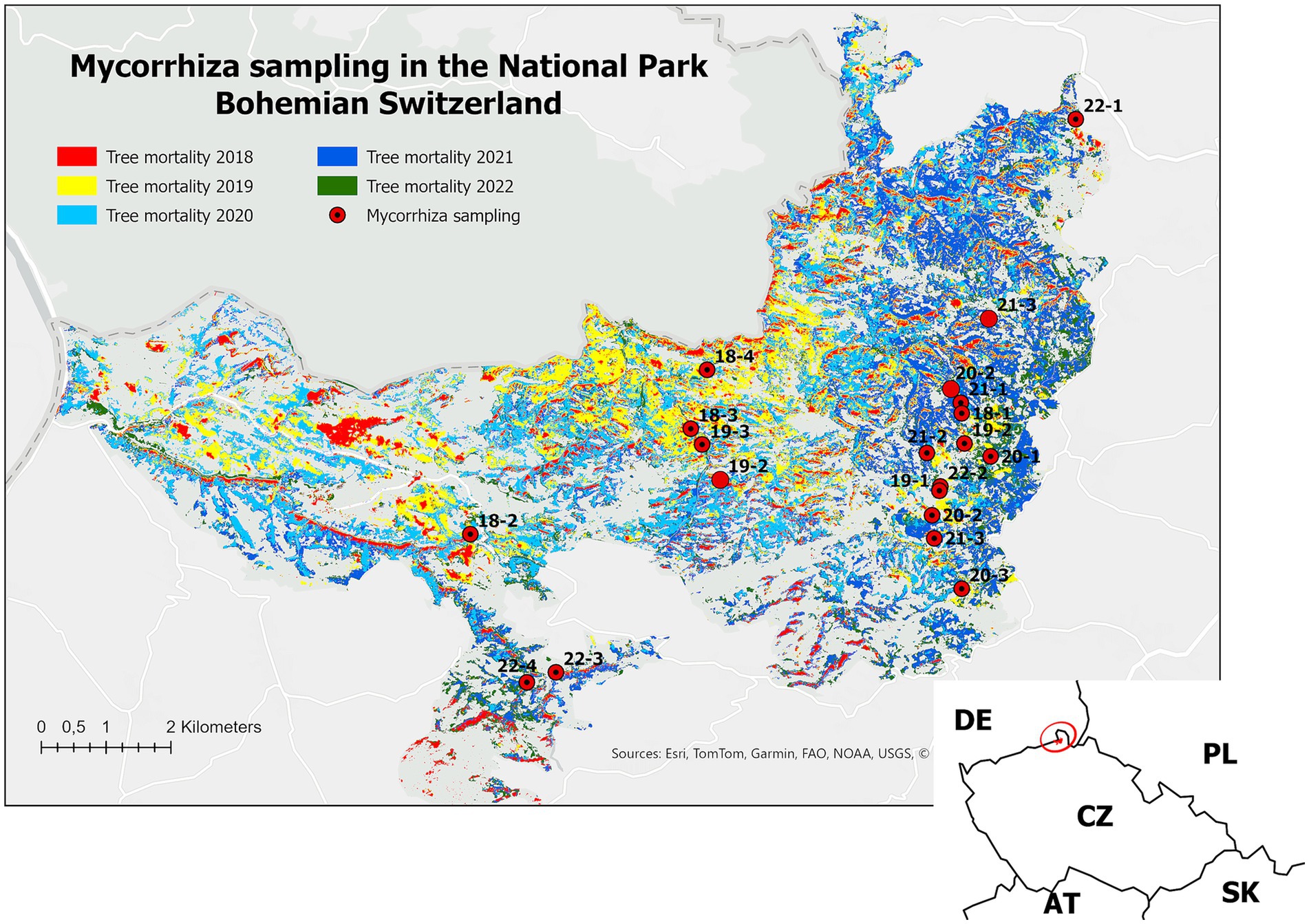
Figure 1. Map of the national park Bohemian Switzerland with indicated mycorrhiza sampling locations and classified layer of the tree mortality in 2018–2022. Mycorrhiza sampling sites are labeled by the year of stand death (18–2018, etc.) and the serial number of the location.
These layers were then matched with data from the forest management plan and, using an attribute query, areas with the following parameters were selected: more than 90% spruce, 80–100 years of age, in Abies – Fagus vegetation zone, acidic, somewhat stony and meagre (5 K resp. 5 N by Viewegh et al., 2003). These parameters correspond to the most common spruce stands in Bohemian Switzerland. The classified stands were randomly visited, and if the surviving spruce was found, a dead tree with a similar dimension was chosen in the nearby surroundings (See Figure 2i). The selection continued until a balanced number of strata for each year of tree death was achieved.
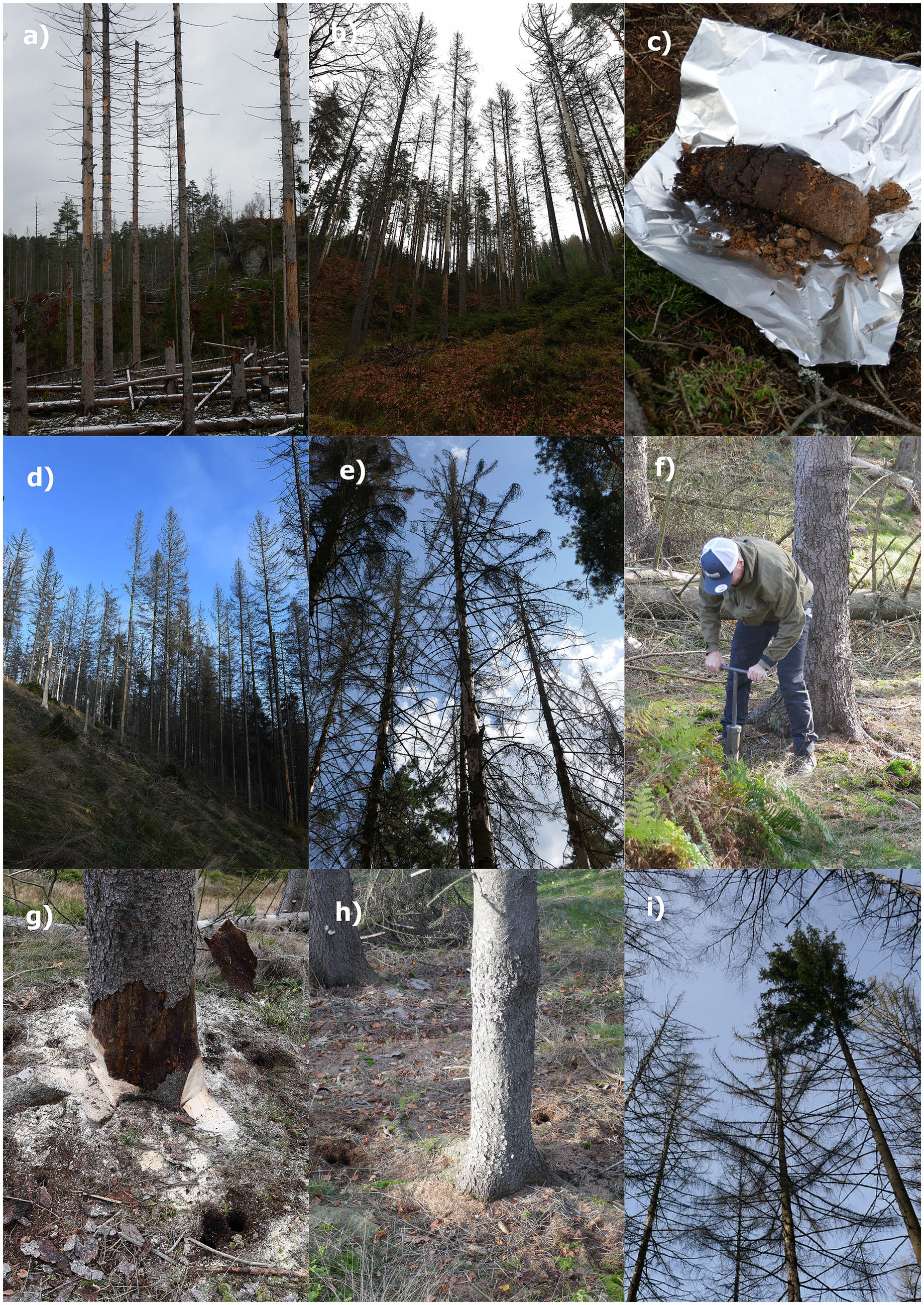
Figure 2. Field sampling: (a) dead standing trees 4 years after disturbance; (b) death standing trees 3 years after disturbance; (c) soil and root sample; (d) death standing trees 2 years after disturbance; (e) death standing trees 1 year after disturbance; (f) collection of soil and root samples using a sampling probe; (g) dead tree sampling; (h) living tree sampling; (i) a surviving tree surrounded by bark-beetle snags.
In total, samples were collected on 17 sites corresponding to different years of tree dieback (Figure 1; for details of layers, see Supplementary material 1). Sample collection was performed using a soil sampling probe with a 6 cm inner diameter and 15 cm sample tube length (Pešková, 2007). On each site, two trees were selected - one snag and a nearby living tree of similar dimensions. Five soil samples were collected randomly up to 1 m from the bole of the living tree and snag (2 × 5 samples, see Figure 2). The bark beetle snag was subsequently felled, and samples were taken in places with visible wood decay. These were used for the macroscopic determination of wood-decaying fungi. Samples were also collected for DNA analysis, they were held in stored in 35% ethanol at −32°C.
In the laboratory, roots were manually extracted from the soil samples and separated according to diameter into <1 mm, 1–2 mm, and 2–5 mm fractions. Roots smaller than 1 mm in diameter were stored in 2.5% glutaraldehyde for further analysis. Roots 1–2 mm and 2–5 mm diameter were dried in a drying cabinet at 105°C for 24 h and weighed with 0.01 g precision. Numbers of mycorrhizal tips were determined on root segments with a diameter of <1 mm using a stereomicroscope with 40× magnification. Smooth, light-colored ECM tips with a well-developed hyphal sheath and high turgor were considered “vital mycorrhizae” (VM). In contrast, flaccid, dark-colored ECM tips without a visible hyphal sheath were categorized as “non-vital mycorrhizae” (NVM) (Corcobado et al., 2014). From each soil sample, 20 root segments (5 cm in length) were evaluated this way. The density of mycorrhizal tips was calculated as the mean number of VM or NVM per 1 cm of root length (Pešková et al., 2015). Soil pH (H2O) was determined in each soil sample by suspending 10 g of soil in 100 mL of 0.1 M aqueous calcium chloride and measuring the pH using a pH 50 VioLab meter (Germany) with a glass electrode until a constant value was displayed. The measurements were carried out in triplicates.
Identification of fungal species from the trees where mycorrhizae were evaluated involved a multi-faceted approach: (i) observation of fruiting bodies on the bole and stump, (ii) search for rhizomorphs and/or mycelial fans beneath the bark, and (iii) assessment of wood rot. Fruiting bodies found on the boles or stumps of the trees were collected for further determination using the identification key for European polypores (Ryvarden and Melo, 2014). If wood rot was present, trees were inspected in more detail. Additionally, samples of rhizomorphs were collected from beneath the bark of stumps or root swellings. Samples of decaying wood were also collected from the felled trees. In the laboratory, wood samples were cut into smaller pieces (3–4 mm) with a sterilized scalpel, superficially sterilized in 70% ethanol, and washed with distilled water. The sterilized pieces were placed onto a malt extract agar (MEA; 33.6 g/L of Malt Extract Agar, Carl Roth), with 4–5 pieces per Petri dish. The samples were incubated at 20°C in the dark, and after the appearance of the first hyphae, they were promptly subcultured onto a fresh MEA medium. Collected rhizomorphs were cut into smaller parts using a sterilized scalpel, superficially sterilized using 70% ethanol and sodium hypochlorite, and subsequently washed with distilled water. These samples were plated onto a MEA amended with 100 mg/L streptomycin (Carl Roth). Additionally, samples of rhizomorphs and mycelium growing beneath the bark were collected and stored in 35% ethanol at −32°C for further molecular analysis, employing the same procedures as the fungal cultures. All obtained isolates were morphologically studied and identified using fungal DNA barcoding. Mycelium taken from developed cultures was lyophilised and used for genetic identification. DNA from these samples was isolated using a modified CTAB-PVP method (Porebski et al., 1997; Schenk et al., 2023). The primer combination ITS1/ITS4 for amplifying the ITS region of the ribosomal RNA gene and LR3/LR0R primers (nuclear large subunit rDNA) were used for PCR. The PCR products were sequenced using the Sanger sequencing method by SEQme s.r.o. (Dobříš, Czech Republic). The nucleotide Basic Local Alignment Search Tool (BLAST) was used to compare the obtained sequences with those in the National Center for Biotechnology Information (NCBI) database (http://www.ncbi.nlm.nih.gov, accessed on 15 October 2023).
The differences between snags and live trees, regarding densities of VM and NVM and dry root biomass, were assessed using independent regression models created according to Zuur et al. (2010). The independent variables were (i) Tree, a categorical variable with two levels – bark beetle snag and live tree; (ii) Year, an ordinal variable representing the time elapsed between tree death and sample collection with five levels – 0.5 years; 1 year; 2 years; 3 years; 4 years. Since the live and dead trees originated from the same site and multiple samples were taken from the same tree, a generalized mixed effect model (GLMM) with the two-time nested factor was applied. A suitable family of distributions was chosen according to the Akaike information criterion (AIC). The fit quality was evaluated visually by plotting the model’s residuals against the quantiles of a normal distribution. In all cases, the negative binomial family was most suitable. The significance of factors was subsequently determined by χ2 tests using the command drop1. Differences between the levels of Tree and Year were tested in the selected model using a contrast matrix (Pekár and Brabec, 2016). The model formulation was performed in R version 4.3.1 (R Core Team, 2023) in the package glmmTMB following the procedures described by Brooks et al., (2017). The connections between Tree mycorrhizal parameters (Roots12 – mean dry weight mass of roots with 1–2 mm; Roots25 – mean dry weight mass of roots with 2–5 mm; VM – mean density of vital mycorrhizae; NVM – mean density of non-vital mycorrhizae) and environmental, respectively, specific factors (pH – mean pH of soil samples, decay – sum of trees with wood decaying, tree – categorical variable: Dead standing tree / Living tree) were determined using a principal component analysis (PCA) according to Meloun and Militký (2011) and visualized using the factoextra package (Kassambara and Mundt, 2020).
Results
Mycorrhizal density and dynamics
The density of vital mycorrhizae on bark beetle snags was significantly lower compared to living trees (χ2: df = 1; p < 0.001). The number of VM tips changed with different dynamics between living and dead trees (χ2: df = 4; p < 0.01). On living trees, a gradual increase of VM, peaking in the 2nd and 3rd year after the bark beetle disturbance of the surrounding forest stand, was observed (Figure 3). The difference between VM density on live trees in the 1st, 2nd, and 3rd year after the bark beetle disturbance and VM density 0.5 years after the disturbance was statistically significant (Table 1). In the 4th year, however, a pronounced decrease in VM was observed (Figure 3). The density of VM on the root systems of dead trees was very low from the beginning and randomly fluctuated in later years (Figure 3, Table 1, and Supplementary Table S1). A statistically significant difference was observed in all periods when comparing VM density between live and dead trees (Table 1).
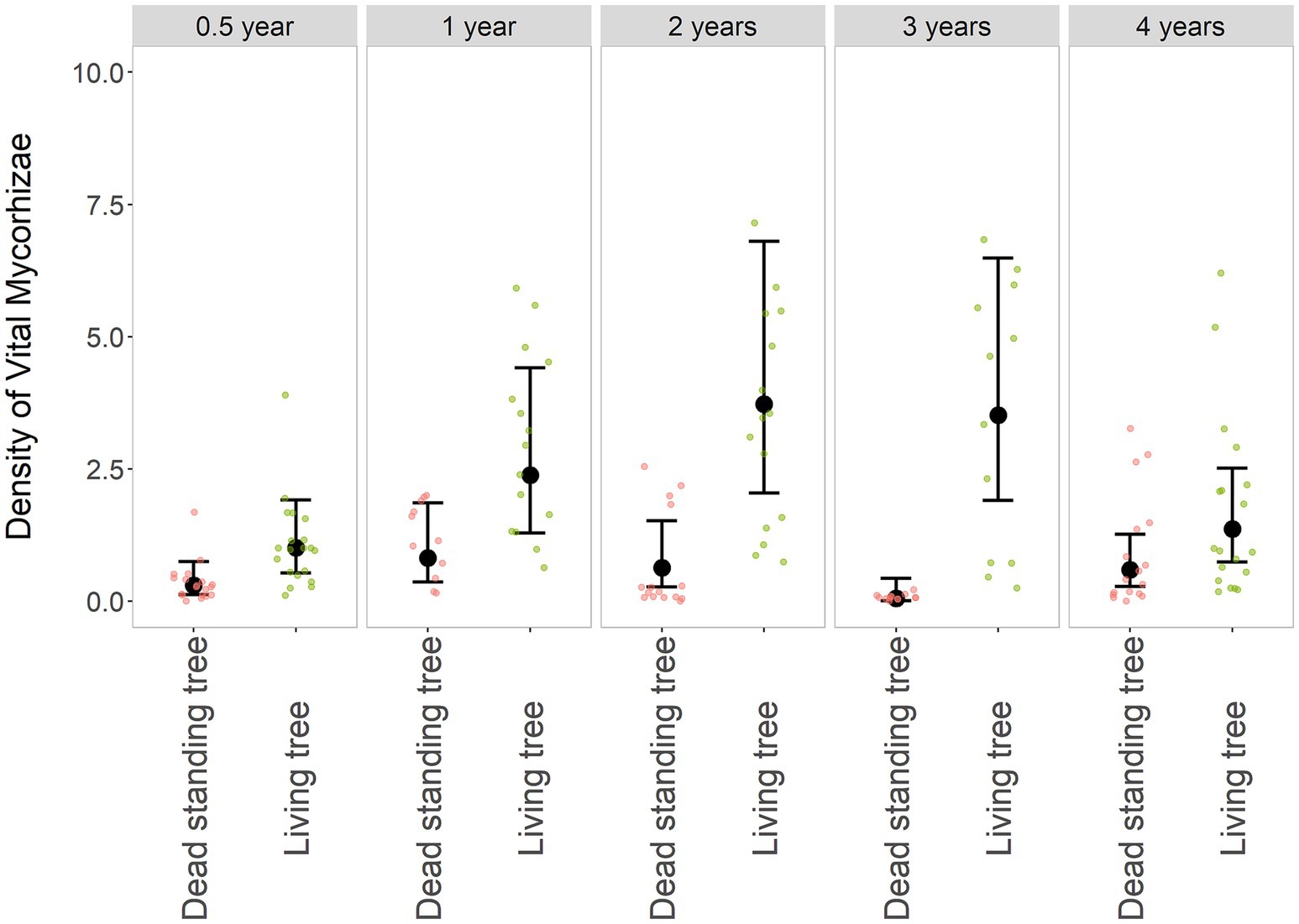
Figure 3. Density of vital mycorrhizae (VM) in dead and living trees over time. The black dot represents the fitted value and whiskers 95% confidence intervals of the GLMM model VM ~ Tree * Year + (1 | Locality | Individual), family = negative binomial. The smaller dots in the background are original values, red-dead standing trees, and green-living trees. A comparison of the dead and living trees is grouped along the years from the disturbance of the surrounding forest stand, resp—the tree death.
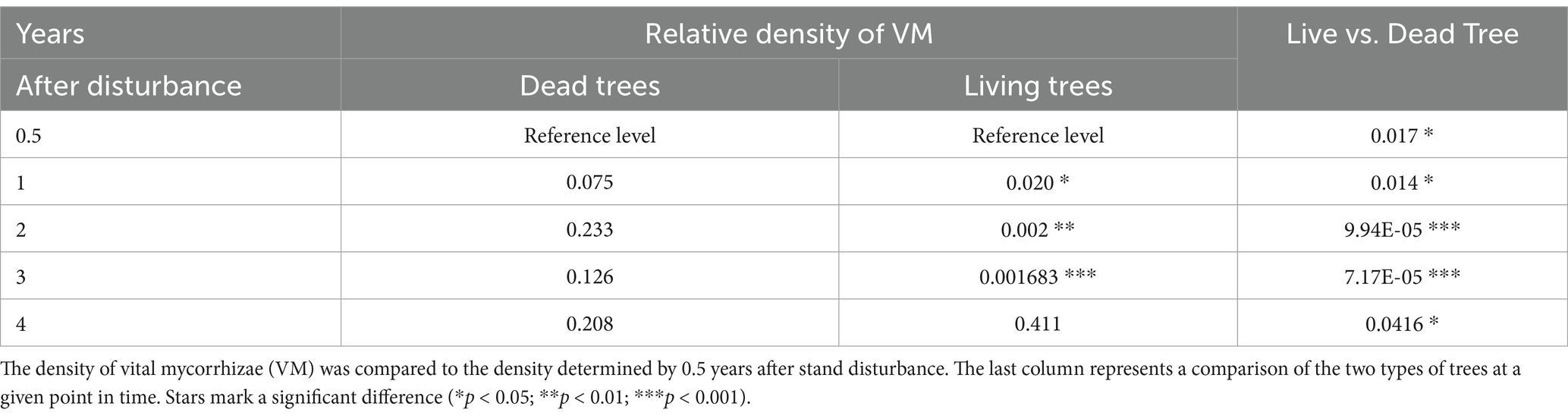
Table 1. Result of the contrast comparison (p-values) for GLMM model VM ~ Tree * Year + (1 | Locality/TreeIndividual), family = negative binomial.
The density of non-vital mycorrhizal tips was significantly higher on snags compared to living trees (χ2: df = 1; p < 0.001) and did not change as markedly over time as the density of VM. Nevertheless, it was unstable (χ2: df = 4; p < 0.01). The dynamics of change in the density of NVM was also significantly different between live and dead trees (χ2: df = 4; p < 0.01). With dead trees, a significant increase in NVM was observed within the 1st year of tree death (Figure 4, Table 2, and Supplementary Table S2). This is likely due to a change of vital mycorrhizal tips to non-vital ones. After the 2nd and 3rd years, living trees had a low density of NVM but did not differ owing to the situation 0.5 years after the disturbance (Table 2). A significant difference between dead and surviving trees was observed in the 1st and 3rd year (contrast t-test; p < 0.01 and p < 0.05, respectively), where bark beetle snags had a higher density of NVM tips.
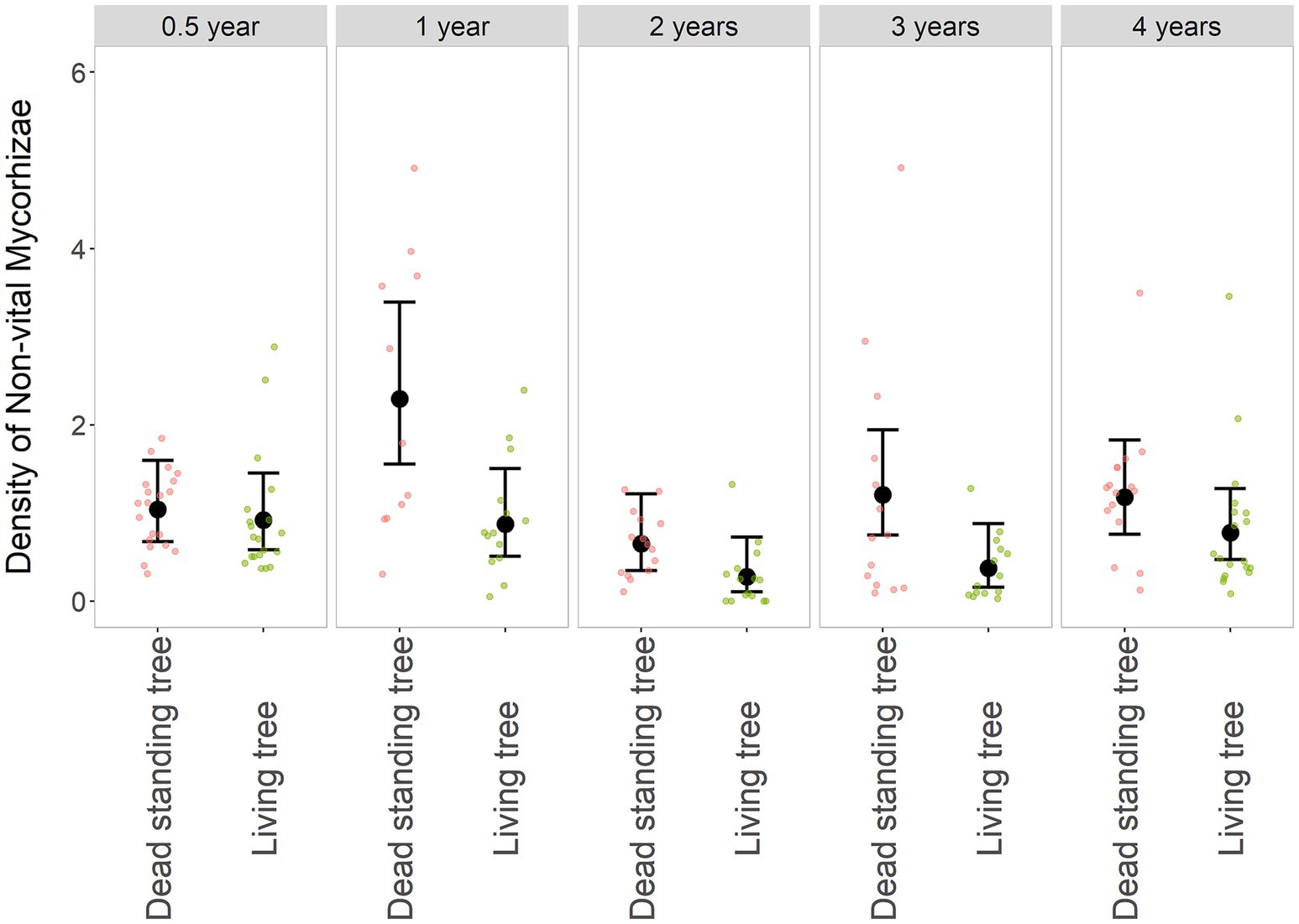
Figure 4. Relative density of non-vital mycorrhizae (NVM) in dead and living trees over time. The black dot represents the fitted value and whiskers 95% confidence intervals of the GLMM model NVM ~ Tree * Year + (1 | Locality | Individual), family = negative binomial. The smaller dots in the background are original values, red-dead standing trees, and green-living trees. A comparison of the dead and living trees is grouped along the years from the disturbance of the surrounding forest stand, resp—the tree death.
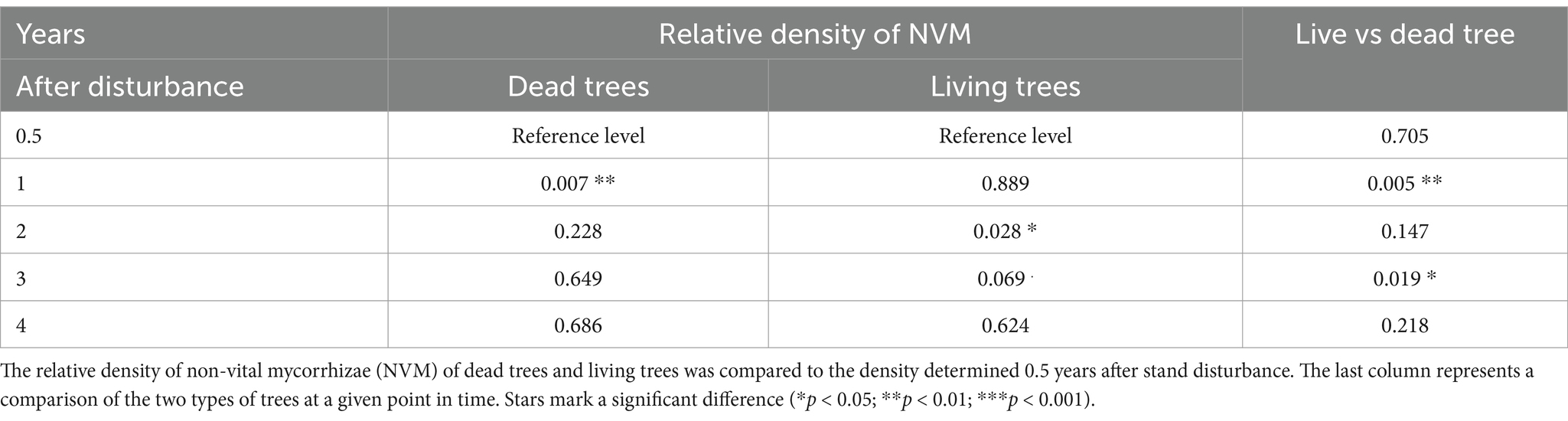
Table 2. Result of the contrast comparison (p-value) for GLMM model NVM ~ Tree * Year + (1 | Locality/TreeIndividual), family = negative binomial.
Root system biomass
The volume of the root system, as represented by the dry biomass of roots smaller than 2 mm in diameter, was significantly greater in living trees (χ2: df = 1; p < 0.01). The abundance of fine roots gradually decreased as the surrounding tree cover died (Supplementary Table S3). However, the change between individual periods was statistically insignificant (χ2: df = 4; p = 0.12). A higher ratio of non-vital mycorrhizae was associated with dead trees. The gradual decrease in root biomass in living and dead trees also occurred at a similar rate. The interaction between factors was statistically insignificant (χ2: df = 4; p = 0.92; Figure 5). The dry weight of roots <2 mm in size was negatively associated with an increasing pH value (Figure 6). The higher the soil pH, the fewer fine roots are present. The connection between the mass of larger roots and soil pH was rather indifferent (only a weak negative association was found).
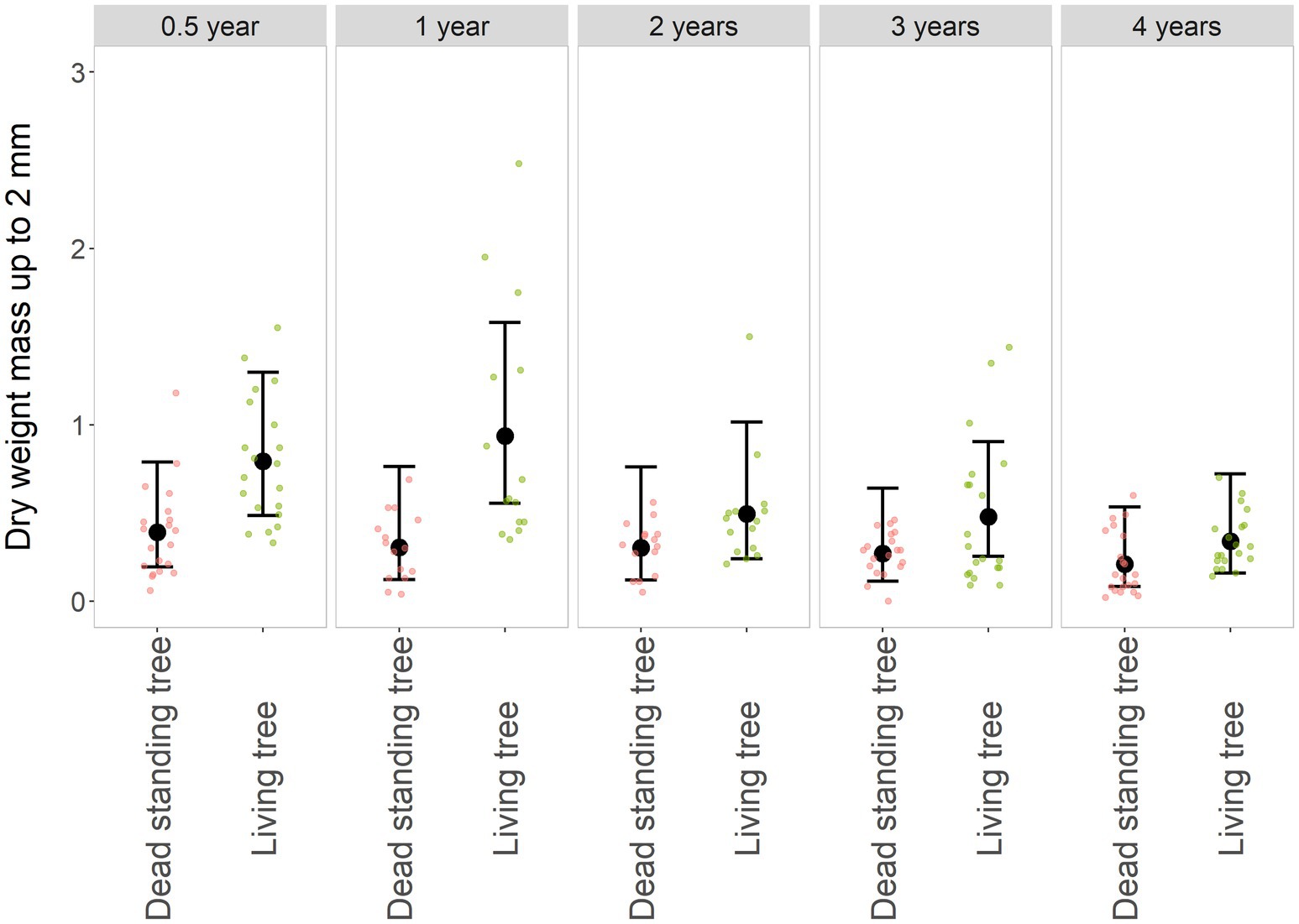
Figure 5. Dry biomass of roots up to 2 mm in diameter. The black dot represents the fitted value and whiskers 95% confidence intervals of the GLMM model Roots < 2 mm ~ Tree * Year + (1 | Locality | Individual), family = negative binomial. The smaller dots in the background are original values, red-dead standing trees, and green-living trees. A comparison of the dead and living trees is grouped along the years from the disturbance of the surrounding forest stand, resp—the tree death.
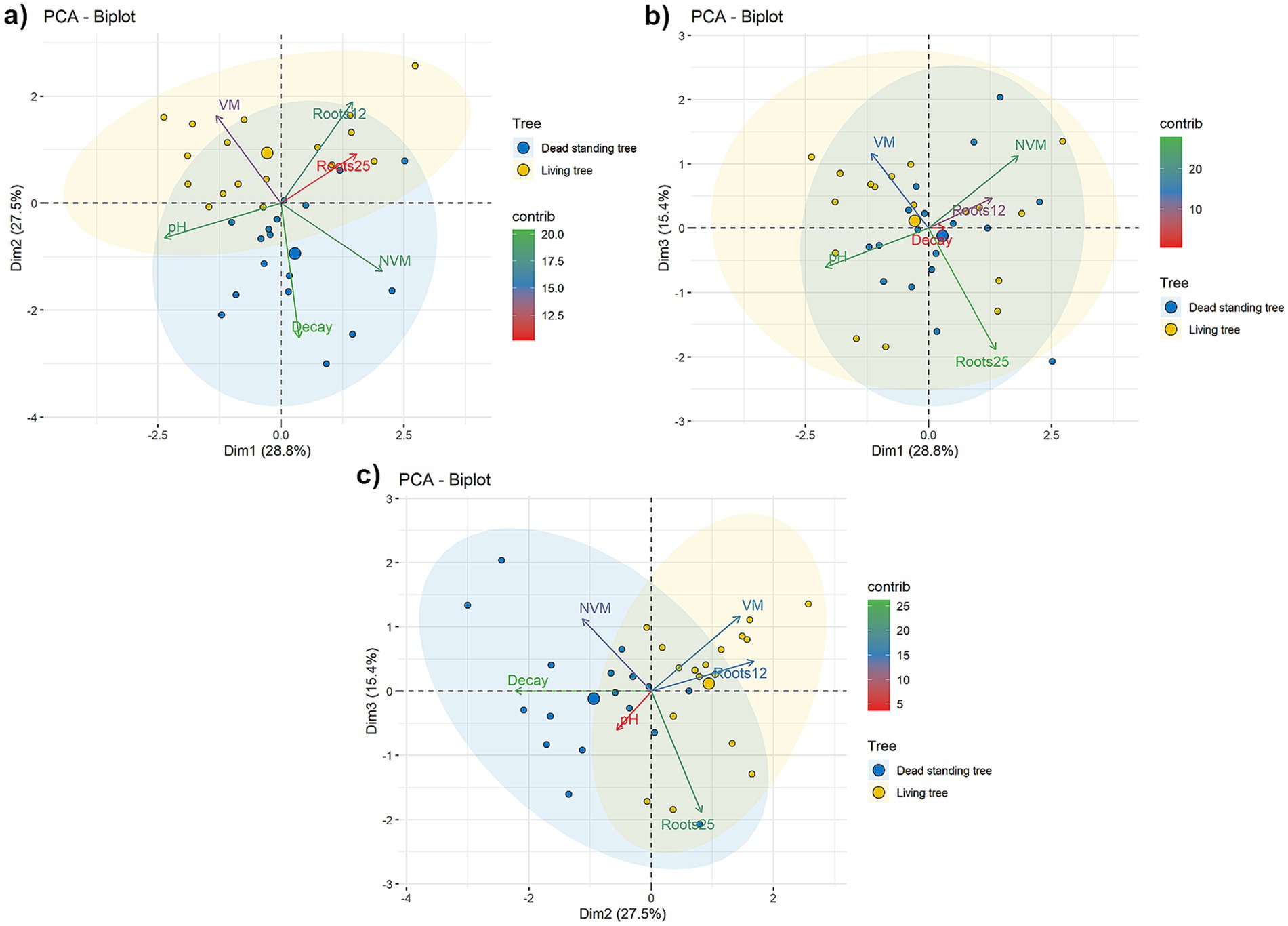
Figure 6. BiPlots for PCA analysis, displayed are the first three principal components (first – Dim 1, second – Dim2, and third – Dim3 components); (a) Dim1 and Dim2; (b) Dim1 and Dim3; (c) Dim2 and Dim3. Dead and living trees are represented by blue and yellow dots, respectively. The size of the dots represents the importance of the observation. Loadings are color-coded according to their contribution to explaining the variability of the plotted principal components.
Identification of fungal species
The most frequently identified fungal species belonged to the genus Armillaria (Armillaria ostoyae (Romagn.) Herink, Armillaria cepistipes Velen.) and Fomitopsis pinicola (Sw.) P. Karst. (Table 3 and Figure 7). Fungi from the genus Armillaria were detected and isolated on four snags between 2018 and 2021. The genus Fomitopsis was detected between 2018 and 2021 on seven snags. The white-rot fungus Pleurotus ostreatus (Jacq.) P. Kumm. was observed on one snag from 2021. Results of PCA analysis (Figure 6) show the more complex view of the relationships between mycorrhizal parameters, root biomass, decaying processes and selected habitat characteristics. The first component (Dim1) is associated with habitat richness (characterized by pH), which is positively connected to the density of VM and negatively connected to NVM. The second component (Dim2) directly separated living and dead trees according to the presence and number of wood-decaying fungi. The third component (Dim3) is probably led by the wetness of the habitat, where sites more enriched with water had more VM and NVM, and the opposite, relatively dryer sites contained more roots with a 2–5 mm diameter. Projections of the three principal components explained 72% of the variability. The general trend across the dimensions was the following–density of VM was positively associated with living trees. Conversely, decaying processes and the presence of saprotrophic fungi were associated with dead trees.
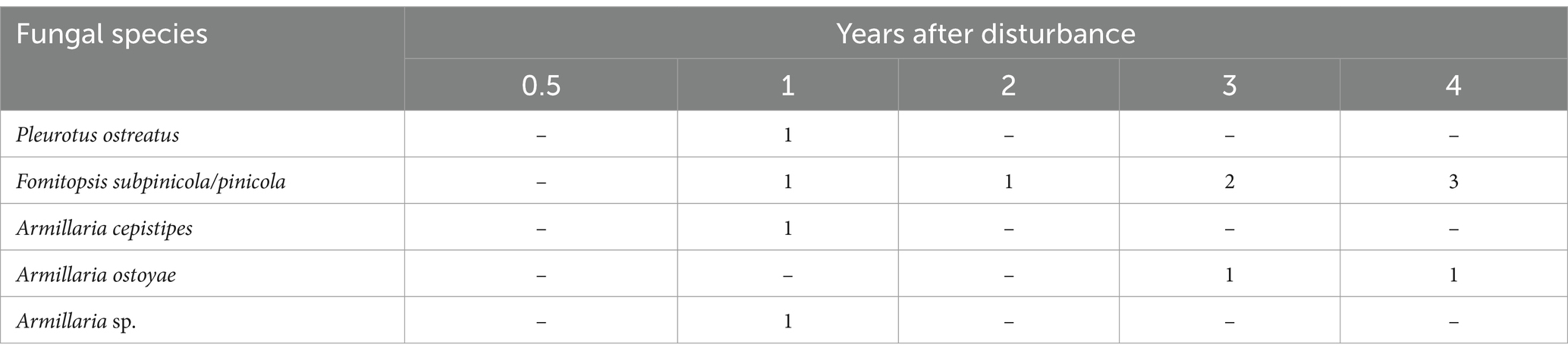
Table 3. Wood decay fungal species identified in bark beetle snags of different ages (number of identifications).
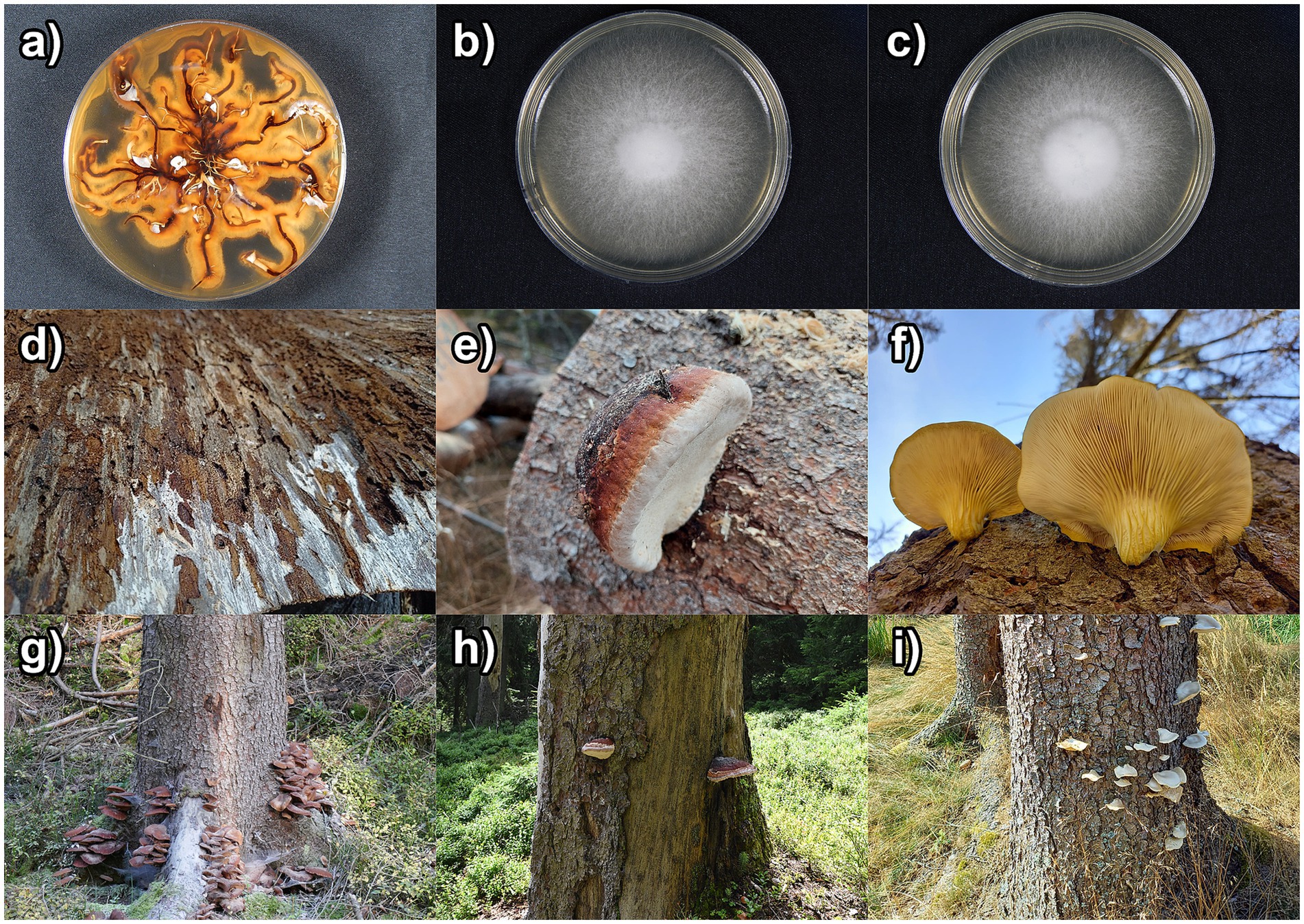
Figure 7. Selected wood decay fungi found on bark beetle snags: (a) culture of Armillaria cepistipes after 60 days on PDA agar medium; (b) Fomitopsis pinicola after 8 days on PDA agar medium; (c) Pleurotus ostreatus after 8 days on PDA agar medium; (d) syrrocium of Armillaria sp.; (e) fruiting body Fomitopsis pinicola; (f) fruiting bodies Pleurotus ostreatus; (g) massive fructification of Armillaria sp. in the autumn; (h) bole with fruiting bodies Fomitopsis pinicola; (i) bole with amounts of fruiting bodies P. ostreatus.
Discussion
The bark beetle outbreak of the I. typographus in the Bohemian Switzerland National Park has been intense since 2018, with vast areas being infested (Hlásny et al., 2021c; Washaya et al., 2024). It can be assumed that all standing snags in the area result from the bark beetle attack. The few surviving spruces were mostly of lower canopy, an example of classification by Pommerening et al. (2021), and thus somewhat smaller than the surrounding trees. Contrary to bark beetle-surviving trees in the Šumava National Park (cf. Korolyova et al., 2022), the surviving trees in Bohemian Switzerland did not show any regular spatial distribution pattern. The trees in Šumava, however, belonged to the natural spruce zone, often in waterlogged locations with a high abundance of natural forests. In contrast, the studied locations in Bohemian Switzerland consisted mostly of azonal, artificially planted growths.
During the first years after the disturbance, the surviving trees gradually increased VM tips. The mean values of VM density on live trees in the 2nd and 3rd years after the disturbance were more than double the values reported in previous studies (Pešková, 2007; Pešková et al., 2011; Lorenc et al., 2018). An increased access to sunlight, water, and nutrients will likely cause an increase in VM density in the surviving trees. Most of the current Central European spruce forests are overly dense. Thinning can improve tree vitality manifested, for example, by increases in transpiration rate (Özçelik et al., 2022) and photosynthetic activity. A higher allocation of photosynthetic products to the soil can stimulate ECM species’ development (Štursová et al., 2014; Corcobado et al., 2014). In addition to improved tree conditions, fungal growth conditions are directly affected after stand decay. For example, an increase in soil temperature leads to the development of ECM fungi (Mohan et al., 2014), which has also been observed in areas where trees were removed following a windstorm (Veselá et al., 2019). Temperature can directly affect mycorrhizae both positively and negatively. The higher temperatures above 35–40°C damage hyphae of ECM fungi (Smith and Read, 2008), but an increase in soil temperature usually leads to a rise in ECM fungi density (Mohan et al., 2014). However, the temperature optimum varies among species (Domisch et al., 2002). Low temperatures can have a lethal effect, especially on fungi colonizing the upper soil horizons (Gryndler et al., 2004). Many species of ECM fungi can survive exposure to low temperatures, but the time required to resume active growth and their subsequent growth rates vary inter- and intraspecifically (France et al., 1979). It can be assumed that mycorrhizal tips formed gradually, in an immature form at first, and subsequent years transitioned to a mature vital form, as was the case during drought stress in spruce stands (Pešková et al., 2015).
The decline in vital mycorrhizae in the fourth year in living trees may be related to the overall decline of ECM fungal species in sites disturbed by bark beetle outbreaks (Štursová et al., 2014; Mayer et al., 2022; Choma et al., 2023) or to increased drought stress due to stronger exposition to sunlight. Similar results were reached by Sterkenburg et al. (2019), who investigated the effect of logging in pine stands on ECM’s abundance and species diversity. After 3 years, they observed a 95% decrease in ECM fungi in logged or partially logged forests compared to the unlogged area. This decline was due to the increased stress and stronger soil exposure to sunlight. Preserved were only a few common species of ECM fungi, while rarer species gradually disappeared. Thus, it can be agreed that trees surviving bark beetle disturbance are crucial for maintaining the community of ECM fungi (Mayer et al., 2022; Choma et al., 2023).
The number of VM tips on the roots of dead trees was very low in our study compared to live or drought-stressed trees (Pešková et al., 2015; Lorenc et al., 2018). Trees infested by the spruce bark beetle gradually change their physiological functions, i.e., they must invest available resources into various defense responses, thus stopping assimilate deposition and slowing down photosynthesis. Subsequently, the transpiration stream is interrupted, and the tree dies within about a month (Krokene, 2015). The lower rate of photosynthesis (or its gradual cessation) leads to a decrease in C deposition in the soil. Carbon balance and its allocation are closely linked to the vitality of the tree and its ability to respond to stress factors such as drought, nutrient deficiency or pest incidence. In healthy trees, carbon obtained through photosynthesis is primarily used for growth, with some of this carbon being transported to the roots, which also supports symbiosis with mycorrhizal fungi (Finzi et al., 2015; Brunner et al., 2015). However, environmental stress or pest infestation can significantly disrupt this process. After tree death, the ectomycorrhizal symbiosis can be temporarily maintained due to previously stored carbon stocks, as studies from boreal forests suggest (Lindahl et al., 2007). These changes could lead to the subsequent alteration in the composition of the soil fungal community with the dominance of saprotrophic species and the gradual disappearance of ECM fungi (Yarwood et al., 2009, Štursová et al., 2014, Mayer et al., 2022, Choma et al., 2023). Despite that, vital mycorrhizal tips were found even 4 years after tree death. It has been suggested that ECM fungi could temporarily switch to a saprotrophic mode of nutrition (Štursová et al., 2014). The presence of ECM fungi on dead roots was demonstrated 1 year after the tree was felled (Veselá et al., 2019). The number of NVM tips was statistically significantly higher in bark beetle snags compared to live trees in all surveyed years. Declines in mycorrhizal diversity due to insect damage to trees, pathogens, nutritional deficiency in the soil, drought, and other disturbances have been described in several studies (Jones et al., 2008; Blom et al., 2009; Corcobado et al., 2014). In the case of dead trees, there was a significant increase in the number of NVM tips in the first year after tree death. This significant increase in the proportion of NVM tips probably occurred due to a change from the vital to the non-vital form and their gradual death in the following years (Scatollin et al., 2008).
Root volume, represented by the dry weight of roots <2 mm in diameter, was significantly larger in live trees than in bark beetle snags. The fact that the fine root biomass of bark beetle snags was so low compared to live trees and did not decline significantly over time suggests that most of the fine roots had already decomposed before sampling was initiated. This is in accordance with our initial supposition, based on Rhoades et al. (2020), that fine root decay will occur soon after tree mortality. In live trees, root dry weight was relatively high during the first year after stand dieback and gradually decreased in fine roots. The difference between periods, however, was not statistically significant. The development of fine roots in the first year after disturbance followed an improved supply of solar energy and water, as in the case of vital mycorrhizae. However, the increase in root volume can also be associated with the surviving tree’s need to improve its stability against the action of wind. While the surrounding dead trees may temporarily slow wind speeds, their eventual fall exposes the surviving trees to conditions they may not be adapted to Oberle et al. (2018). This situation is also well known in commercial forests, where individual trees left in clearings after clear-cutting are often subject to the forces of winds, as is also the case with newly exposed stand edges (Modlinger and Novotný, 2015). A higher risk of storm damage is also well-known after the thinning. However, the number of fine roots was relatively lower from the second year after the bark beetle disturbance. This may be partly due to the same unfavorable climatic conditions of 2018 and 2019, which caused the large outbreak of bark beetles in the first place (Netherer et al., 2019; Hlásny et al., 2021c).
The extreme dry season of 2018 may have influenced biological processes for multiple years ahead (Bose et al., 2022). Drought-stressed trees typically experience a decline in mycorrhizae (Gehring et al., 1997; Kuikka et al., 2003; Ostonen et al., 2013) and an increase in the relative abundance of roots compared to above-ground parts. However, the total amount of root biomass, especially in fine roots, decreases during drought stress (Brunner et al., 2015). A gradual decrease in root mass also occurred in dead trees. This corresponds with the gradual decrease in the dry weight of fine roots we observed. When tree mortality is high, there is an increase in wood-decaying fungi, at least in the short term. This may also have implications for ECM fungi diversity. Extremes in environmental conditions (e.g., drought, high temperatures, CO2, insect infestation) predispose stressed trees to subsequent infestation by wood decay fungi (Kim et al., 2021). These fungi represent a specific physiological-ecological group of heterotrophic organisms that initiate successional processes, leading to humification and, in some cases, mineralization of deadwood (Murray and Leslie, 2021).
Genetic analyses have identified several pathogens in our research, the most prominent of which belonged to the genus Armillaria. Armillaria causes root rot and a decline in dry root biomass in infected trees (Kubiak et al., 2017; Murray and Leslie, 2021). Armillaria species are primarily saprotrophic, with occasional opportunistic pathogenicity, causing primary infections of the root system (Coetzee et al., 2018). Another identified fungal species was Fomitopsis, a pathogen typically infecting fresh bark-beetle snags (Vogel et al., 2017). As saprotrophs, Fomitopsis species can rapidly decompose their hosts, leading to a collapse within several years. Fungi of both genera, Armillaria and Fomitopsis, can significantly influence local conditions through the decomposition of newly emergent deadwood and influence nutrient cycles in the soil. Therefore, wood decay fungi can contribute to changes in vegetation composition that generally impact mycorrhizae, likely due to their obligately symbiotic relationships (Anderson et al., 2010).
Multivariate analysis of correlations between mycorrhizal abundance, root dry weight, and environmental factors, as well as detected fungal species, confirmed an association between VM density, live trees, and root abundance. In contrast, NVM tips’ density and wood-decaying fungi’s presence were associated with dead trees. Soil pH was not directly positively associated with any of the observed factors, but the analysis showed a relatively strong negative association with the number of fine roots. The range of measured pH values was not very large at the individual sites, ranging from 2.78 to 3.65, i.e., these were relatively acidic soils, which corresponds to the soil classification assigned by foresters (Viewegh et al., 2003). Mycorrhizal root formation is significantly affected by soil acidity in mature stands of Norway spruce (Nowotny et al., 1998), and even small changes in pH are sufficient to induce long-term changes in the composition of ECM communities, as demonstrated by Kjøller and Clemmensen, (2009). However, these differences in our study were only small because of the relatedness of soil and habitat originating from variability within the same classification.
Our study demonstrates that mycorrhizal activity increases in trees surviving a bark beetle disturbance, and they probably become another ECM refugia. In active management of the spruce bark beetle, in addition to felling infested trees, living trees are usually also removed for easier mechanized restoration. With the current extensive deforestation that has occurred in Czechia as a result of the spruce bark beetle (see Washaya et al., 2024), the chances of survival of symbiotic root organisms are considerably reduced (Sterkenburg et al., 2019). The success of subsequent restoration of clearings is thus made even more complicated. The goal of forest management should, therefore, be to preserve trees that have survived bark beetle disturbance and, previously, increase their chances of survival when the collapse of the surrounding forest stand comes. However, this can only be achieved through targeted stand tending at a young age (up to approximately 40 years), supporting the tree’s stability and resistance to bark beetle attacks. In the context of the increasing frequency and intensity of disturbances in forests, enhancing tree resistance also brings other benefits that will strengthen forest resilience due to higher ECM persistence.
Conclusion
The findings of this study underscore the complex relationships between tree mortality, mycorrhizal dynamics, and forest ecosystem processes in the aftermath of bark beetle-induced dieback. We confirmed that the density and dynamics of vital mycorrhizae (VM) differ markedly between living trees and bark beetle snags, with living trees exhibiting a temporal increase in VM density. In contrast, snags showed a higher prevalence of non-vital mycorrhizal (NVM) tips, reflecting a pronounced shift from vital to non-vital mycorrhizae within the first year following tree death. This transition highlights the immediate below-ground consequences of tree mortality.
The study further reveals that the biomass of fine roots, represented by the dry weight of roots smaller than 2 mm, is substantially greater in living trees compared to dead ones. The rapid decomposition of fine root biomass—primarily within 0.5 years of tree death—emphasizes the transient nature of below-ground structures. However, the differences in fine root biomass between living and dead trees 2, 3, and 4 years after mortality aren’t surprisingly so markable.
From an ecological perspective, the role of wood decay fungi is significant. Identifying fungi such as Armillaria, Fomitopsis, and Pleurotus ostreatus through macroscopic analysis and DNA barcoding underscores their contribution to the decomposition process. Additionally, environmental factors, particularly soil pH, influenced these below-ground dynamics, as evidenced by the negative correlation between fine root abundance and pH levels.
In conclusion, our findings highlight the intricate interplay between tree mortality, mycorrhizal dynamics, and forest ecosystems in the wake of bark beetle infestations. This research contributes valuable insights into the broader ecological consequences of such disturbances, offering another insight into below-ground processes and providing useful information for future strategies in managing and mitigating the impacts of bark beetle outbreaks on forest ecosystems.
Data availability statement
The raw data supporting the conclusions of this article will be made available by the authors, without undue reservation.
Author contributions
RM: Conceptualization, Data curation, Formal analysis, Investigation, Methodology, Project administration, Supervision, Validation, Visualization, Writing – original draft, Writing – review & editing. VP: Investigation, Methodology, Supervision, Writing – original draft, Writing – review & editing. JZ: Investigation, Writing – original draft. MM: Investigation, Methodology, Writing – original draft. ML: Investigation, Writing – original draft. DT: Investigation, Visualization, Writing – original draft, Writing – review & editing. ON: Writing – original draft. VZ: Writing – original draft. PS: Data curation, Methodology, Project administration, Resources, Writing – original draft, Writing – review & editing.
Funding
The author(s) declare that financial support was received for the research and/or publication of this article. This research was funded by the Ministry of Agriculture of the Czech Republic, grant number QK21010435.
Acknowledgments
We thank Tomáš Tonka (University of South Bohemia, Czech Republic) for conducting genetic analyses. We also thank Adéla Juříková and Johana Šindelářová for their help in soil probes separation and Elisha Njomaba for linguistic support.
Conflict of interest
The authors declare that the research was conducted in the absence of any commercial or financial relationships that could be construed as a potential conflict of interest.
Publisher’s note
All claims expressed in this article are solely those of the authors and do not necessarily represent those of their affiliated organizations, or those of the publisher, the editors and the reviewers. Any product that may be evaluated in this article, or claim that may be made by its manufacturer, is not guaranteed or endorsed by the publisher.
Supplementary material
The Supplementary material for this article can be found online at: https://www.frontiersin.org/articles/10.3389/ffgc.2025.1492622/full#supplementary-material
References
Aakala, T. (2010). Coarse woody debris in late-successional Picea abies forests in northern Europe: variability in quantities and models of decay class dynamics. For. Ecol. Manag. 260, 770–779. doi: 10.1016/j.foreco.2010.05.035
Anderson, P., Brundrett, M., Grierson, P., and Robinson, R. (2010). Impact of severe forest dieback caused by Phytophthora cinnamomi on macrofungal diversity in the northern jarrah forest of Western Australia. For. Ecol. Manag. 259, 1033–1040. doi: 10.1016/j.foreco.2009.12.015
Blanck, K., Lamersdorf, N., Dohrenbusch, A., and Murach, D. (1995). Response of a Norway spruce forest ecosystem to drought/rewetting experiments at Solling, Germany. Water Air Soil Pollut. 85, 1251–1256. doi: 10.1007/BF00477153
Blom, J. M., Vannini, A., Vettraino, A. M., Hale, M. D., and Godbold, D. L. (2009). Ectomycorrhizal community structure in a healthy and a Phytophthora-infected chestnut (Castanea sativa mill.) stand in Central Italy. Mycorrhiza 20, 25–38. doi: 10.1007/s00572-009-0256-z
Bose, A. K., Rohner, B., Bottero, A., Ferretti, M., and Forrester, D. I. (2022). Did the 2018 megadrought change the partitioning of growth between tree sizes and species? A Swiss case-study. Plant Biol. 24, 1146–1156. doi: 10.1111/plb.13380
Brooks, M. E., Kristensen, K., Van Benthem, K. J., Magnusson, A., Berg, C. W., Nielsen, A., et al. (2017). glmmTMB balances speed and flexibility among packages for zero-inflated generalized linear mixed modeling. R J. 9, 378–400. doi: 10.32614/RJ-2017-066
Brunner, I., Herzog, C., Dawes, M. A., Arend, M., and Sperisen, C. (2015). How tree roots respond to drought. Front. Plant Sci. 6:547. doi: 10.3389/fpls.2015.00547
Bruns, T. D. (1995). Thoughts on the processes that maintain local species diversity of ectomycorrhizal fungi. Plant Soil 170, 63–73. doi: 10.1007/BF02183055
Cheng, L., Chen, W., Adams, T. S., Wei, X., Li, L., McCormack, M. L., et al. (2016). Mycorrhizal fungi and roots are complementary in foraging within nutrient patches. Ecology 97, 2815–2823. doi: 10.1002/ecy.1514
Choma, M., Bače, R., Čapek, P., Kaňa, J., Kaštovská, E., Tahovská, K., et al. (2023). Surviving trees are key elements in the fate of ectomycorrhizal community after severe bark-beetle forest disturbance. FEMS Microbiol. Ecol. 99, 1–10. doi: 10.1093/femsec/fiad082
Coetzee, M. P. A., Wingfield, B. D., and Wingfield, M. J. (2018). Armillaria root-rot pathogens: species boundaries and global distribution. Pathogens 7:83. doi: 10.3390/pathogens7040083
Corcobado, T., Vivas, M., Moreno, G., and Solla, A. (2014). Ectomycorrhizal symbiosis in declining and non-declining Quercus ilex trees infected with or free of Phytophthora cinnamomi. For. Ecol. Manag. 324, 72–80. doi: 10.1016/j.foreco.2014.03.040
Dieler, J., Uhl, E., Biber, P., Müller, J., Rötzer, T., and Pretzsch, H. (2017). Effect of forest stand management on species composition, structural diversity, and productivity in the temperate zone of Europe. Eur. J. For. Res. 136, 739–766. doi: 10.1007/s10342-017-1056-1
Domisch, T., Finér, L., and Lehto, T. (2002). Growth, carbohydrate and nutrient allocation of scots pine seedlings after exposure to simulated low soil temperature in spring. Plant Soil 246, 75–86. doi: 10.1023/A:1021527716616
Eldhuset, T., Nagy, N. E., Volařík, D., Børja, I., Gebauer, R., Yakovlev, I., et al. (2012). Drought affects tracheid structure, dehydrin expression, and above- and below-ground growth in 5-year-old Norway spruce. Plant Soil 366, 305–320. doi: 10.1007/s11104-012-1432-z
Enkhtuya, B., and Vosátka, M. (2005). Interaction between grass and trees mediated by extraradical mycelium of symbiotic arbuscular mycorrhizal fungi. Symbiosis 38, 261–276.
Feil, W., Kottke, I., and Oberwinkler, F. (1988). The effect of drought on mycorrhizal production and very fine root system development of Norway spruce under natural and experimental conditions. Plant Soil 108, 221–231. doi: 10.1007/BF02375652
Finzi, A. C., Abramoff, R. Z., Spiller, K. S., Brzostek, E. R., Darby, B. A., Kramer, M. A., et al. (2015). Rhizosphere processes are quantitatively important components of terrestrial carbon and nutrient cycles. Glob. Chang. Biol. 21, 2082–2094. doi: 10.1111/gcb.12816
France, R. C., Cline, M. L., and Reid, C. P. (1979). Recovery of ectomycorrhizal fungi after exposure to subfreezing temperatures. Botany 57, 1845–1848. doi: 10.1139/b79-231
Gärtner, A., Jönsson, A. M., Metcalfe, D. B., Pugh, T. A. M., Tagesson, T., and Ahlström, A. (2023). Temperature and tree size explain the mean time to fall of dead standing trees across large scales. Forests 14:1017. doi: 10.3390/f14051017
Gaul, D., Hertel, D., Borken, W., Matzner, E., and Leuschner, C. (2008). Effects of experimental drought on the fine root system of mature Norway spruce. For. Ecol. Manag. 256, 1151–1159. doi: 10.1016/j.foreco.2008.06.016
Gehring, C. A., Cobb, N. S., and Whitham, T. G. (1997). Three-way interactions among ectomycorrhizal mutualists, scale insects, and resistant and susceptible pinyon pines. Am. Nat. 149, 824–841. doi: 10.1086/286026
Graf, F., Bast, A., Gärtner, H., and Yildiz, A. (2018). “Effects of mycorrhizal fungi on slope stabilisation functions of plants” in Recent advances in geotechnical research. ed. W. Wu (Cham, Switzerland: Springer Series in Geomechanics and Geoengineering), 55–77.
Gryndler, M., Baláž, M., Hršelová, H., Jansa, J., and Vosátka, M. (2004). Mykorhizní symbióza. O soužití hub s kořeny rostlin. Praha: Academia.
Helmisaari, H. J., Ostonen, I., Lõhmus, K., Derome, J., Lindroos, A. J., Merilä, P., et al. (2009). Ectomycorrhizal root tips in relation to site and stand characteristics in Norway spruce and scots pine stands in boreal forests. Tree Physiol. 29, 445–456. doi: 10.1093/treephys/tpn042
Hlásny, T., König, L., Krokene, P., Lindner, M., Montagné-Huck, C., Müller, J., et al. (2021a). Bark beetle outbreaks in Europe: state of knowledge and ways forward for management. Curr. Forestry Rep. 7, 138–165. doi: 10.1007/s40725-021-00142-x
Hlásny, T., Merganičová, K., Modlinger, R., Marušák, R., Löwe, R., and Turčáni, M. (2021c). Prognosis of bark beetle outbreak and a new platform for the dissemination of information about the forests in the Czech Republic. Zprávy Lesnického Výzkumu 66, 197–205.
Hlásny, T., Zimová, S., Merganičová, K., Štěpánek, P., Modlinger, R., and Turčáni, M. (2021b). Devastating outbreak of bark beetles in the Czech Republic: drivers, impacts and management implications. For. Ecol. Manag. 490:119075. doi: 10.1016/j.foreco.2021.119075
Högberg, M. N., and Högberg, P. (2002). Extramatrical ectomycorrhizal mycelium contributes one-third of microbial biomass and produces, together with associated roots, half the dissolved organic carbon in a forest soil. New Phytol. 154, 791–795. doi: 10.1046/j.1469-8137.2002.00417.x
Jansen, A.E., (1992). “Importance of ectomycorrhiza for forest ecosystems“, in Responses of forest ecosystems to environmental changes, ed. Teller, A, Mathy, P, and Jeffers, J.N.R (Springer: Dordrecht), 456–461.
Jelonek, T., Klimek, K., Kopaczyk, J., Wieruszewski, M., Arasimowicz-Jelonek, M., Tomczak, A., et al. (2020). Influence of the tree decay duration on mechanical stability of Norway spruce wood (Picea abies (L.) Karst.). Forests 11, 1–13. doi: 10.3390/f11090980
Jones, M. D., Twieg, B. D., Durall, D. M., and Berch, S. M. (2008). Location relative to a retention patch affects the ectomycorrhizal fungal community more than patch size in the first season after timber harvesting on Vancouver Island, British Columbia. For. Ecol. Manag. 255, 1342–1352. doi: 10.1016/j.foreco.2007.10.042
Kassambara, A., and Mundt, F. (2020) Factoextra: extract and visualize the results of multivariate data analyses. R Package Version 1.0.7.
Kausrud, K., Økland, B., Skarpaas, O., Grégoire, J. C., Erbilgin, N., and Stenseth, N. C. (2012). Population dynamics in changing environments: the case of an eruptive forest pest species. Biol. Rev. Camb. Philos. Soc. 87, 34–51. doi: 10.1111/j.1469-185X.2011.00183.x
Kautz, M., Peter, F. J., Harms, L., Kammen, S., and Delb, H. (2022). Patterns, drivers and detectability of infestation symptoms following attacks by the European spruce bark beetle. J. Pest. Sci. 96, 403–414. doi: 10.1007/s10340-022-01490-8
Kim, M. S., Hanna, J. W., Stewart, J. E., Warwell, M. V., McDonald, G. I., and Klopfenstein, N. B. (2021). Predicting present and future suitable climate spaces (potential distributions) for an Armillaria root disease pathogen (Armillaria solidipes) and its host, Douglas-fir (Pseudotsuga menziesii), under changing climates. Front. For. Glob. Change 4:740994. doi: 10.3389/ffgc.2021.740994
Kjøller, R., and Clemmensen, K. E. (2009). Below-ground ectomycorrhizal fungal communities respond to liming in three southern Swedish coniferous forest stands. For. Ecol. Manag. 257, 2217–2225. doi: 10.1016/j.foreco.2009.02.038
Konôpka, B., Pajtík, J., and Maľová, M. (2013). Fine root standing stock and production in young beech and spruce stands. For. J. 59, 161–171. doi: 10.2478/v10114-011-0023-x
Korolyova, N., Buechling, A., Ďuračiová, R., Zabihi, K., Turčáni, M., Svoboda, M., et al. (2022). The last trees standing: climate modulates tree survival factors during a prolonged bark beetle outbreak in Europe. Agric. For. Meteorol. 322:109025. doi: 10.1016/j.agrformet.2022.109025
Král, K., Daněk, P., Janík, D., Krůček, M., and Vrška, T. (2018). How cyclical and predictable are central European temperate forest dynamics in terms of development phases? J. Veg. Sci. 29, 84–97. doi: 10.1111/jvs.12590
Krokene, P. (2015). “Conifer defense and resistance to bark beetles” in Bark beetles: Biology and ecology of native and invasive species. eds. F. E. Vega and R. W. Hofstetter (London-San Diego-Waltham-Oxford: Elsevier Academic Press).
Kubiak, K., Zółciak, A., Damszel, M., Lech, P., and Sierota, Z. (2017). Armillaria pathogenesis under climate changes. Forests 8:100. doi: 10.3390/f8040100
Kudláčková, L., Poděbradská, M., Bláhová, M., Cienciala, E., Beranová, J., McHugh, C., et al. (2023). Using FlamMap to assess wildfire behavior in bohemian Switzerland National Park. Nat. Hazards 120, 3943–3977. doi: 10.1007/s11069-023-06361-8
Kuikka, K., Härmä, E., Markkola, A., Rautio, P., Roitto, M., Saikkonen, K., et al. (2003). Severe defoliation of scots pine reduces reproductive investment by ectomycorrhizal symbionts. Ecology 84, 2051–2061. doi: 10.1890/02-0359
Landeweert, R., Leeflang, P., Kuyper, T. W., Hoffland, E., Rosling, A., Wernars, K., et al. (2003). Molecular identification of ectomycorrhizal mycelium in soil horizons. App. Environ. Microbiol. 69, 327–333. doi: 10.1128/AEM.69.1.327-333.2003
Lieutier, F., Keith, D. R., Battisti, A., Grégorie, J. C., and Evans, H. F. (2004). Bark and wood boring insects in living trees in Europe, a synthesis. Dodrecht-Boston-London: Kluwer Academic Publishers.
Lindahl, B. D., Ihrmark, K., Bobeberg, J., Trumbore, S. E., Högberg, P., Stenlid, J., et al. (2007). Spatial separation of litter decomposition and mycorrhizal nitrogen uptake in a boreal forest. New Phytol. 173, 611–620. doi: 10.1111/j.1469-8137.2006.01936.x
Lorenc, F., Pešková, V., Modlinger, R., Mrnka, L., Tomášková, I., Šenfeld, P., et al. (2018). Fine roots of Picea abies compensate for drought stress in the rainfall reduction experiment. Dendrobiology 80, 91–100. doi: 10.12657/denbio.080.009
Löwe, R., Sedlecký, M., Sikora, A., Prokůpková, A., Modlinger, R., Novotný, K., et al. (2022). How bark beetle attack changes the tensile and compressive strength of spruce wood (Picea abies (L.) H. Karst.). Forests 13:87. doi: 10.3390/f13010087
Maheshwari, R. (2008). A botanist, mycorrhiza and a knighthood. Resonance 13, 541–547. doi: 10.1007/s12045-008-0060-7
Majdi, H. (2001). Changes in fine root production and longevity in relation to water and nutrient availability in a Norway spruce stand in northern Sweden. Tree Physiol. 21, 1057–1061. doi: 10.1093/treephys/21.14.1057
Matějčíková, J., Vébrová, D., and Surový, P. (2024). Comparative analysis of machine learning techniques and data sources for dead tree detection: what is the best way to go? Remote Sens. 16:3086. doi: 10.3390/rs16163086
Mayer, M., Rosinger, C., Gorfer, M., Berger, H., Deltedesco, E., Bässler, C., et al. (2022). Surviving trees and deadwood moderate changes in soil fungal communities and associated functioning after natural forest disturbance and salvage logging. Soil Biol. Biochem. 166:108558. doi: 10.1016/j.soilbio.2022.108558
Meloun, M., and Militký, J. (2011). Statistical data analysis: A practical guide. Cambridge: Woodhead Publishing.
Modlinger, R., and Novotný, P. (2015). Quantification of time delay between damages caused by windstorms and by Ips typographus. For. J. 61, 221–231. doi: 10.1515/forj-2015-0030
Mohan, J. E., Cowden, C. C., Baas, P., Dawadi, A., Frankson, P. T., Helmick, K., et al. (2014). Mycorrhizal fungi mediation of terrestrial ecosystem responses to global change: Mini-review. Fungal Ecol. 10, 3–19. doi: 10.1016/j.funeco.2014.01.005
Mortimer, M. J., and Kane, B. (2004). Hazard tree liability in the United States: uncertain risks for owners and professionals. Urban For. Urban Green. 2, 159–165. doi: 10.1078/1618-8667-00032
Müller, J., Bussler, H., Gossner, M., Rettelbach, T., and Duelli, P. (2008). The European spruce bark beetle Ips typographus in a national park: from pest to keystone species. Biodivers. Conserv. 17, 2979–3001. doi: 10.1007/s10531-008-9409-1
Murray, M. P., and Leslie, A. (2021). Climate, radial growth, and mortality associated with conifer regeneration infected by root disease (Armillaria ostoyae). For. Chron. 97, 43–51. doi: 10.5558/tfc2021-006
Netherer, S., Panassiti, B., Pennerstorfer, J., and Matthews, B. (2019). Acute drought is an important driver of bark beetle infestation in Austrian Norway spruce stands. Front. For. Glob. Change 2:39. doi: 10.3389/ffgc.2019.00039
Nowotny, I., Schwanz, J., and Rothe, G. M. (1998). Influence of soil acidification and liming on selected enzymes of the carbohydrate metabolism and the contents of two major organic acids of mycorrhizal roots of Norway spruce (Picea abies (L.) Karst.). Plant Soil 199, 41–51. doi: 10.1023/A:1004221910199
Oberle, B., Ogle, K., Zanne, A. E., and Woodall, C. W. (2018). When a tree falls: controls on wood decay predict standing dead tree fall and new risks in changing forests. PLoS One 13:e0196712. doi: 10.1371/journal.pone.0196712
Oettel, J., Zolles, A., Gschwantner, T., Lapin, K., Kindermann, G., Schweinzer, K. M., et al. (2023). Dynamics of standing deadwood in Austrian forests under varying forest management and climatic conditions. J. Appl. Ecol. 60, 696–713. doi: 10.1111/1365-2664.14359
Ostonen, I., Püttsepp, Ü., Biel, C., Alberton, O., Bakker, M. R., Lõhmus, K., et al. (2007). Specific root length as an indicator of environmental change. Plant Biosyst. 141, 426–442. doi: 10.1080/11263500701626069
Ostonen, I., Rosenvald, K., Helmisaari, H. S., Godbold, D., Parts, K., Uri, V., et al. (2013). Morphological plasticity of ectomycorrhizal short roots in Betula sp and Picea abies forests across climate and forest succession gradients: its role in changing environments. Plant Sci. 4:335. doi: 10.3389/fpls.2013.00335
Özçelik, M. S., Tomášková, I., Surový, P., and Modlinger, R. (2022). Effect of forest edge cutting on transpiration rate in Picea abies (L.) H. Karst. Forests 13:1238. doi: 10.3390/f13081238
Palátová, E. (2004). Effect of increased nitrogen depositions and drought stress on the development of young Norway spruce Picea abies (L.) Karst. Stands. Dendrobiology 51, 41–45.
Parviainen, J., Bücking, W., Vandekerkhove, K., Schuck, A., and Päivinen, R. (2000). Strict forest reserves in Europe: efforts to enhance biodiversity and research on forests left for free development in Europe (EU-COST-action E4). Forestry 73, 107–118. doi: 10.1093/forestry/73.2.107
Patacca, M., Lindner, M., Lucas-Borja, M. E., Cordonnier, T., Fidej, G., Gardiner, B., et al. (2022). Significant increase in natural disturbance impacts on European forests since 1950. Glob. Chang. Biol. 29, 1359–1376. doi: 10.1111/gcb.16531
Pešková, V. (2007). Changes in the mycorrhizal status of some mountain spruce forests. J. For. Sci. 53, 82–88. doi: 10.17221/2160-JFS
Pešková, V., Landa, J., and Soukup, F. (2011). Findings regarding ectotrophic stability of Norway spruce forest of the Krkonoše and Orlické Mountains based on mycorrhiza studies. J. For. Sci. 57, 500–513. doi: 10.17221/139/2010-JFS
Pešková, V., Lorenc, F., Modlinger, R., and Pokorná, V. (2015). Impact of drought and stand edge on mycorrhizal density on the fine roots of Norway spruce. Ann. For. Res. 58, 1–257. doi: 10.15287/afr.2015.364
Peterson, R. L., Massicotte, H. B., and Melville, H. (2004). Mycorrhizas: Anatomy and cell biology. Ottawa: NRC Research Press, 1–173.
Pommerening, A., Maleki, K., and Haufe, J. (2021). Tamm review: individual-based forest management or seeing the tree for the forest. For. Ecol. Manag. 501:119677. doi: 10.1016/j.foreco.2021.119677
Porebski, S., Bailey, L. G., and Baum, B. R. (1997). Modification of a CTAB DNA extraction protocol for plants containing high polysaccharide and polyphenol components. Plant Mol. Biol. Report. 15, 8–15. doi: 10.1007/BF02772108
Potterf, M., Nikolov, C., Kocická, E., Ferenčík, J., Mezei, P., and Jakuš, R. (2019). Landscape-level spread of beetle infestations from windthrown-and beetle-killed trees in the non-intervention zone of the Tatra National Park, Slovakia (Central Europe). For. Ecol. Manag. 432, 489–500. doi: 10.1016/j.foreco.2018.09.050
Přívětivý, T., Adam, D., and Vrška, T. (2018). Decay dynamics of Abies alba and Picea abies deadwood in relation to environmental conditions. For. Ecol. Manag. 427, 250–259. doi: 10.1016/j.foreco.2018.06.008
Puhe, J. (2003). Growth and development of the root system of Norway spruce (Picea abies) in forest stands-a review. For. Ecol. Manag. 175, 253–273. doi: 10.1016/S0378-1127(02)00134-2
R Core Team (2023). R: A language and environment for statistical computing. Vienna: R Foundation for Statistical Computing.
Read, D. J., and Boyd, R. (1986). “Water relations of mycorrhizal fungi and their host plants” in Water, fungi and plants. eds. P. Ayres and L. Boddy (Cambridge: Cambridge University Press), 287–303.
Rhoades, C. C., Hubbard, R. M., Hood, P. R., Starr, B. J., Tinker, D. B., and Elder, K. (2020). Snagfall the first decade after severe bark beetle infestation of high-elevation forests in Colorado, USA. Ecol. Appl. 30:e02059. doi: 10.1002/eap.2059
Rillig, M. C., and Mummey, D. L. (2006). Mycorrhizas and soil structure. New Phytol. 171, 41–53. doi: 10.1111/j.1469-8137.2006.01750.x
Rondeux, J., and Sanchez, C. (2010). Review of indicators and field methods for monitoring biodiversity within national forest inventories. Core variable: deadwood. Environ. Monit. Assess. 164, 617–630. doi: 10.1007/s10661-009-0917-6
Russell, M. B., Fraver, S., Aakala, T., Gove, J. H., Woodall, C. W., D’Amato, A. W., et al. (2015). Quantifying de and decomposition in dead wood: A review. For. Ecol. Manag. 350, 107–128. doi: 10.1016/j.foreco.2015.04.033
Ryvarden, L., and Melo, I. (2014). Poroid fungi of Europe. Oslo: Fungiflora. Fungiflora, Oslo. Synopsis fungorum 31, 1–455.
Scatollin, L., Montecchio, L., Mosca, E., and Agerer, R. (2008). Vertical distribution of the ectomycorrhizal community in the top soil of Norway spruce stands. Eur. J. For. Res. 127, 347–357. doi: 10.1007/s10342-008-0209-7
Schenk, J. J., Becklund, L. E., Carey, S. J., and Fabre, P. P. (2023). What is the “modified” CTAB protocol? Characterizing modifications to the CTAB DNA extraction protocol. Appl. Plant Sci. 11:e11517. doi: 10.1002/aps3.11517
Schmidlin, T. W. (2009). Human fatalities from wind-related tree failures in the United States, 1995-2007. Nat. Hazards 50, 13–25. doi: 10.1007/s11069-008-9314-7
Smith, S. E., and Read, D. J. (2008). Mycorrhizal Symbiosis. 3rd Edn. New York: Academic Press, 605p.
Sterkenburg, E., Clemmensen, K. E., Lindahl, B. D., and Dahlberg, A. (2019). The significance of retention trees for survival of ectomycorrhizal fungi in clear-cut scots pine forests. J. Appl. Ecol. 56, 1367–1378. doi: 10.1111/1365-2664.13363
Stokland, J. N., Siitonen, J., and Jonsson, B. G. (2012). Biodiversity in dead wood. New York: Cambridge University Press.
Štursová, M., Šnajdr, J., Cajthaml, T., Bárta, J., Šantrůčková, H., and Baldrian, P. (2014). When the forest dies: the response of forest soil fungi to a bark beetle-induced tree dieback. ISME 8, 1920–1931. doi: 10.1038/ismej.2014.37
Svoboda, M. (2005). Deadwood amount and structure and its importance for forest regeneration in spruce mountain forest in area Trojmezi reserve. Zprávy Lesnického Výzkumu 50, 33–45.
Tolasz, R., Míková, T., Valeriánová, A., and Voženílek, V. (2007). Climate atlas of Czechia. Prague, Olomouc: Czech Hydrometeorological Institute, Palackého University.
Vega, F. E., and Hofstetter, R. W. (2015). Bark beetles – Biology and ecology of native and invasive species. London-San Diego-Waltham-Oxford: Elsevier.
Veselá, P., Vašutová, M., Edwards-Jonášová, M., and Cudlín, P. (2019). Soil fungal community in Norway spruce forests under bark beetle attack. Forests 10:109. doi: 10.3390/f10020109
Viewegh, J., Kusbach, A., and Mikeska, M. (2003). Czech forest ecosystem classification. J. For. Sci. 49, 74–82. doi: 10.17221/4682-JFS
Vítková, L., Bače, R., Kjučukov, P., and Svoboda, M. (2018). Deadwood mnagement in central European forests: key consideration for practical implementation. For. Ecol. Manag. 429, 394–405. doi: 10.1016/j.foreco.2018.07.034
Vogel, S., Alvarez, B., Bässler, C., Müller, J., and Thorn, S. (2017). The red-belted bracket (Fomitopsis pinicola) colonizes spruce trees early after bark beetle attack and persists. Fungal Ecol. 27, 182–188. doi: 10.1016/j.funeco.2016.12.007
Washaya, P., Modlinger, R., Tyšer, D., and Hlásny, T. (2024). Patterns and impacts of an unprecedented outbreak of bark beetles in Central Europe: A glimpse into the future? For. Ecosyst. 11:100243. doi: 10.1016/j.fecs.2024.100243
Wermelinger, B. (2004). Ecology and management of the spruce bark beetle Ips typographus – a review of recent research. For. Ecol. Manag. 202, 67–82. doi: 10.1016/j.foreco.2004.07.018
Yarwood, S. A., Myrold, D. D., and Högberg, M. N. (2009). Termination of below-ground C allocation by trees alters soil fungal and bacterial communities in a boreal forest. FEMS Microbiol. Ecol. 70, 151–162. doi: 10.1111/j.1574-6941.2009.00733.x
Keywords: Norway spruce, Ips typographus , root, deadwood, disturbance, forest health, stability, conservation forests
Citation: Modlinger R, Pešková V, Zelený J, Macháčová M, Leiner M, Tyšer D, Nakládal O, Zumr V and Surový P (2025) Ectomycorrhizal response to bark beetle attack: a comparison of dead and surviving trees. Front. For. Glob. Change. 8:1492622. doi: 10.3389/ffgc.2025.1492622
Edited by:
Allan L. Carroll, University of British Columbia, CanadaReviewed by:
Vijay Sheri, East Carolina University, United StatesPäivi Lyytikäinen-Saarenmaa, University of Eastern Finland, Finland
Copyright © 2025 Modlinger, Pešková, Zelený, Macháčová, Leiner, Tyšer, Nakládal, Zumr and Surový. This is an open-access article distributed under the terms of the Creative Commons Attribution License (CC BY). The use, distribution or reproduction in other forums is permitted, provided the original author(s) and the copyright owner(s) are credited and that the original publication in this journal is cited, in accordance with accepted academic practice. No use, distribution or reproduction is permitted which does not comply with these terms.
*Correspondence: Roman Modlinger, bW9kbGluZ2VyQGZsZC5jenUuY3o=