- 1Forestry and Forest Products Research Institute, Tsukuba, Japan
- 2Tama Science Forest Garden, Forestry and Forest Products Research Institute, Tokyo, Japan
- 3Faculty of Life and Environmental Sciences, University of Tsukuba, Tsukuba, Japan
- 4Faculty of Science, University of the Ryukyus, Nishihara, Japan
Climate change poses significant threats to forests globally. Understanding the relationship between environmental variables and species distribution is crucial for evaluating the vulnerability of tree species assemblies to anticipated climate change. Here, we address whether projected future changes in climate suitability are related to the structural stability of the old-growth forest community in Japan. We hypothesize that even with the expected changes in climate, the structural stability of the species assembly will remain unchanged until the end of this century. We modeled the influence of climate change on the spatial distribution of major tree species in a temperate deciduous forest reserve using local and regional presence data. We used the Maxent model and QGIS software to project potential habitat changes. Focusing on the period 2081–2,100, we used the MRI-ESM2-O general circulation model under baseline (SSP5–8.5) and mitigation (SSP1–2.6) future climate scenarios. This revealed that winter temperature is the most crucial factor affecting the distribution of tree species in the temperate landscape. Canopy tree species such as Acer pictum and Castanea crenata are projected to remain stable under SSP5–8.5 in 2100. Our results also suggest that the distribution of Quercus serrata, the dominant species in the forest studied, will expand, particularly under extreme climate conditions in 2100. However, there may be potential reductions in the abundance of subcanopy species, indicating a change in the structure of the forest stand. In this sense, the stability of forest ecosystems and local species diversity may be vulnerable under future climate change scenarios. Exploring the future species distribution and stand structure can improve understanding of habitat changes in temperate landscapes and requires more focused research efforts.
1 Introduction
Climate change poses an imminent threat to global biodiversity, prompting urgent investigations into its profound impacts on ecosystems (IPBES, 2019). Moreover, a decline in biodiversity will deepen the climate crisis, with consequences including reduced species abundance, local extinctions, and the rapid degradation or loss of ecosystems such as mangroves, forests, peatlands, meadows, and seagrass (Macreadie et al., 2021). These changes not only affect the capacity of the planet to store carbon but also impede the ability of nature and humanity to adapt to or cope with evolving climate conditions (Pettorelli et al., 2021). Forests, vital agents in climate change mitigation, face unprecedented challenges due to the anticipated pace of environmental shifts (Hansen et al., 2001; Hamann and Wang, 2006; Warren et al., 2018). Despite their critical role, our understanding of how climate influences the spatial distribution (Canham and Murphy, 2017), abundance, and assembly of tree species remains limited (Chu et al., 2019). Future changes in tree assembly will significantly affect forest ecosystems and ecosystem services.
The capability of conservation and management practices to address climate change depends on the ability to forecast climate trends in the coming decades. This includes predicting ecological and biogeographic responses to climate change and anticipating the impacts of future land management practices (Colavito, 2017). A comprehensive understanding of how forest ecosystems react to climate change is crucial for reforestation initiatives, such as selecting species adapted to future climate conditions (Harger, 1993). In many temperate forest ecosystems, a shift from conifer-dominated to broadleaf-dominated ecosystems is anticipated (Hanewinkel et al., 2014; Thom et al., 2017). To forecast such changes, species distribution models (SDMs), also known as Ecological Niche Models, bio-envelope models, or species envelope models, have been widely used to determine the responses of species under different climate change scenarios (e.g., Guisan and Zimmermann, 2000; Elith and Leathwick, 2009; Tanaka et al., 2012; Nakao et al., 2013; Tang Y. et al., 2018; Ohashi et al., 2019).
Increasingly, research is examining long-term changes in vegetation in response to climate change (Anderson and Song, 2020) and the associated changes in the carbon balance (Mo et al., 2023). It seems unlikely that forests will be able to maintain their current stand structure and total carbon sequestration and storage potential with the changing climate (Thompson et al., 2009). Considering the likelihood that some species will decline under climate change, then which other tree species will be more resilient to the anticipated climate and may therefore be considered suitable alternatives? Instead of relying on models centered on individual species, it might be more beneficial to predict forthcoming changes in spatial distribution as a collective assembly (Zurell et al., 2020). It is important to predict potential habitat distributions at national, regional, and landscape scales under current and future climate conditions (Tanaka et al., 2005).
Temperate deciduous forests in the Northern Hemisphere, situated between evergreen and subarctic coniferous forests, experience distinct seasonal cycles and diverse compositions (Gilliam, 2016). These characteristics make them especially vulnerable to compositional changes driven by climate shifts. Despite their ecological significance, research on future species assemblages in East Asia, particularly in Japan, remains limited. Here the southern (lower) limit of distribution lies adjacent to the upper limit of evergreen broad-leaved forests, and if these forests are invaded, the light environment of the forest floor is likely to change significantly, leading to shifts in species composition and a potential decline in species diversity. The unique position of temperate deciduous forests makes them ideal systems for studying the impacts of climate change on forest ecosystems (Ohsawa, 1993). Moreover, temperate forests are home to numerous tertiary relict plants and endangered species, emphasizing their critical importance for biodiversity conservation and ecological research (Tang C. Q. et al., 2018).
Within this context, the Ogawa Forest Reserve (OFR) emerges as a unique example of an old-growth forest, situated in the southern Abukuma Mountains of Japan. Despite detailed studies on its forest dynamics and structure (Masaki et al., 1992; Nakashizuka et al., 1992; Shibata et al., 2010; Masaki et al., 2021; Wijenayake et al., 2023) there is little in-depth information on the future distribution of species in the vicinity of the Forest Reserve.
This study seeks to bridge this knowledge gap using species distribution models to predict the current and future geographical distribution of major tree species found in vicinity of the OFR under different climate change scenarios. More specifically, we address whether the projected changes in climate suitability are related to the structural stability of a plant community in terms of vertical and horizontal spatial organization and verify the generality of the findings based on the previous related studies on temperate forests. We hypothesized that even with the expected changes in climate, the structural stability of the species assembly will remain unchanged until the end of this century. This hypothesis is based on the observation that populations are already responding to climate trends in the same direction as expected in the future (Jump et al., 2009).
Our results provide detailed insights into the possible trends of the most abundant tree species and the impact of changes in the local tree species abundance and composition on the tree species assembly under future climate scenarios in the study area. They also provide information on potential forest ecosystem stability, which should be given special attention in the context of local species diversity. Overall, this work offers insights into the intricate relationships among species distribution, climate change, and the landscape surrounding OFR, offering valuable information for optimizing conservation and management strategies.
2 Materials and methods
2.1 Study area and target species
We studied the major tree species found in the OFR (100 ha) in northern Ibaraki Prefecture, Japan (36.56°N, 140.35°E) (Figure 1) at elevations of 610–660 m. The climate is variable, with average temperatures ranging from −0.9°C in February to 22.6°C in August and a maximum snow depth of around 50 cm (Mizoguchi et al., 2002). The topography is gently undulating (Yoshinaga et al., 2002). This old-growth forest is characterized by the dominance of Quercus and Fagus (Masaki et al., 1992). The distributions of 17 major tree species were projected under both the present and future climate scenarios. The species included five subcanopy and 12 canopy species (life forms and life history traits of the species from Table 1 in Wijenayake et al., 2023). We defined a 20 × 20 km2 region located centrally in the OFR to determine potential habitat changes surrounding the reserve.
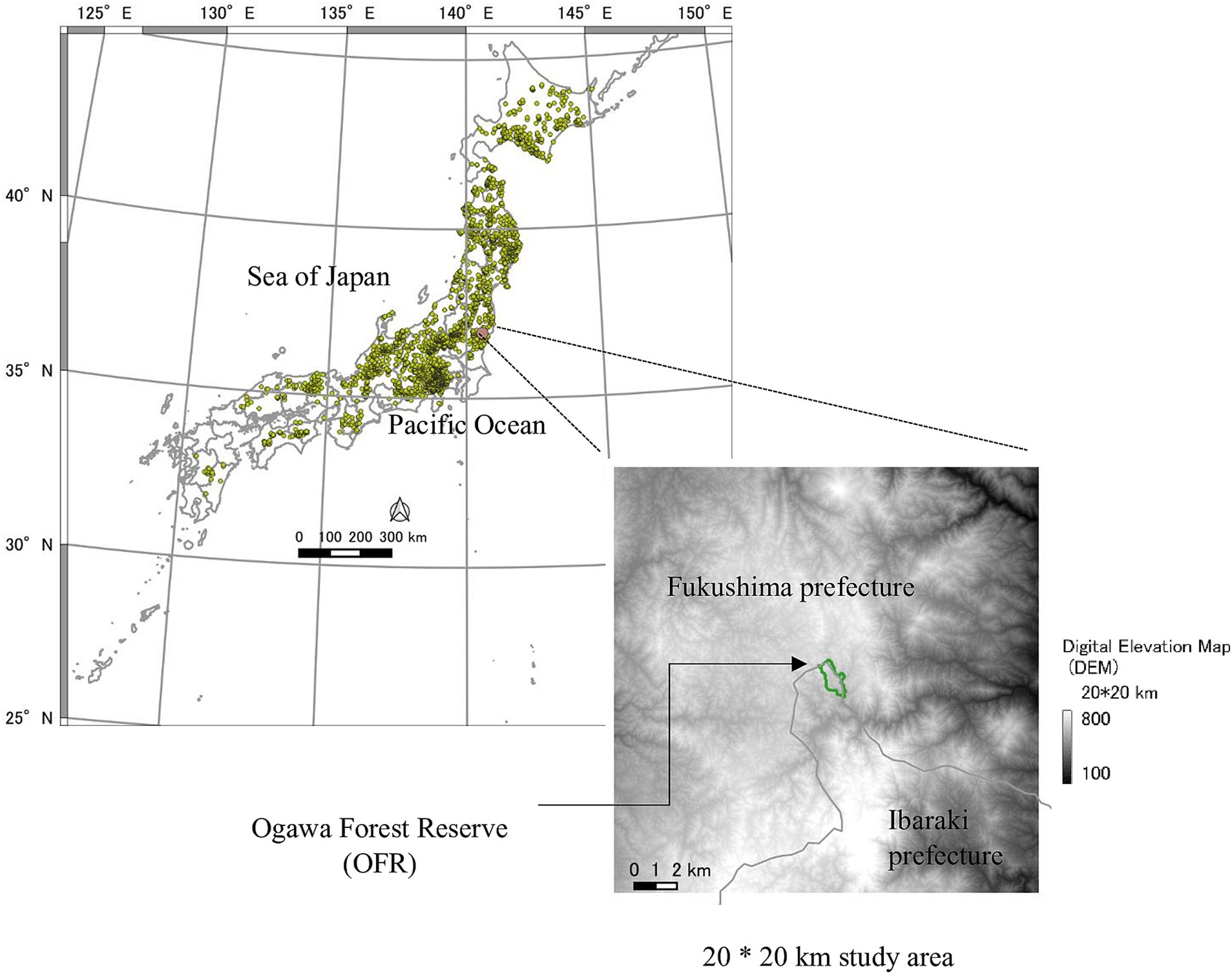
Figure 1. Occurrence records of Carpinus cordata throughout the Japanese Archipelago and the location of Ogawa Forest Reserve (OFR) (36.560°N, 140.350°E). The gray line is the prefecture border. The study area includes the OFR and surrounding area (20 × 20 km) in Ibaraki and Fukushima Prefectures.
First, we examined the occurrence of the selected tree species throughout Japan. These species, recognized as pivotal components of old-growth forests, particularly within the OFR, formed the basis for a comprehensive understanding of their distribution nationwide. Subsequently, climate projections were developed to cover Japan, shedding light on the prevailing climate. Building on this broader perspective, we refined our analysis by narrowing the focus to the specific geographic area. This involved selectively cropping the designated region, centering it around the OFR. This facilitated a more detailed examination of climate scenarios and potential habitat changes within the immediate vicinity of the OFR. The transition from nationwide considerations to localized assessments ensured a thorough reliable understanding of the distributions of the targeted tree species in response to varying climate conditions.
2.2 Distribution data compilation
We used the Japan Biodiversity Mapping Project (J-BMP) database, which contains species occurrence information for vascular plants accumulated through research activities and environmental assessments conducted independently by researchers, local governments, environmental assessment companies, and citizen scientists (Shiono et al., 2021). The J-BMP database includes comprehensive information on species occurrence, functional traits, and phylogeny, which are described in detail on the J-BMP website.1 The data used in the current study included only presence records with defined geographic coordinates following the WGS84 geographic coordinate system. Geographic locations were verified using latitude and longitude information, and any records with discrepancies were discarded. After this data selection process, the final dataset included 8,510 species. Species distribution models (SDMs) were then constructed for each of these species using MaxEnt, with presence data serving as the response variable. Inaccurate data were filtered using QGIS 3.22.7,2 resulting in 66,424 occurrence points. These points were used to develop an initial model predicting the current species distributions. The nomenclature followed that of Yonekura and Kajita (2003).
2.3 Environmental data
We considered two future climate scenarios: the baseline and mitigation scenarios. In the baseline scenario (SSP5–8.5), in which no reductions in greenhouse gas emissions are assumed, the global mean surface temperature is projected to increase by 2.6–4.8°C. By contrast, the mitigation scenario (SSP1–2.6) assumes ambitious climate change mitigation efforts aligned with the goal of limiting global warming to less than 2°C by 2,100.
For species distribution models, the selection of environmental variables is crucial. In this study, we selected variables from WorldClim, which includes 19 global climatic layers from 1970 to 2000 at 1 km2 spatial resolution. In addition, WorldClim CMIP Phase 6 (CIMP6) provided 95 global climate layers for future suitability models, focusing on the period 2081–2,100, using the MRI-ESM2–0 General Circulation Model (GCM) and two shared socioeconomic pathways (SSPs): SSP1–2.6 and SSP5–8.5 (see also Hijmans et al., 2005).3
2.4 Variable selection
Regions with low topographical complexity may be at particular risk of critical transitions under climate change, as evidenced in intermediate and uniform topography scenarios (Scheffer et al., 2012). Given the gentle topography of the study area, we used the first 19 bioclimatic variables for our SDMs, excluding nonclimate variables such as topography, soil, surface geology, slope, and aspect. Furthermore, the relative importance of these variables was minimal in previous studies (Nakao et al., 2013; Matsui et al., 2018; Shitara et al., 2021).
2.5 Species distribution model
As the modeling statistical method, MaxEnt (ver. 3.4.3) 4was chosen due to its higher performance and accuracy compared to other SDM tools. MaxEnt is successful because its regularization process avoids overfitting, particularly when using small sample sizes (Elith et al., 2011). From among 19 variables, we initially selected 12 climate variables that could be interpreted physiologically or ecologically as candidate variables for modeling. Then the bioclimatic variables that best explained the species distribution were determined using the ‘varSel’ function in the ‘SDMtune’ R package. The correlation threshold for removing highly correlated variables was set at a Spearman’s correlation coefficient of 0.7. Next, the models were optimized using the regularization multiplier and feature combination. The regularization multiplier values were set from 0.5 to 5 at 0.5 increments. For feature combinations, we tested the pattern, which combines linear (l), quadratic (q), product (p), threshold (t), and hinge (h). The best models were selected using the Akaike Information Criterion adjusted for small samples (AICc) and assessed using the area under the curve (AUC) of the receiver operating characteristic (ROC) curve. The mean of the MaxEnt predictions was mapped using QGIS. Model performance was evaluated using the continuous Boyce index (CBI), a metric designed to assess the quality of predictions based solely on presence data. The index ranges from −1 to 1, where negative values indicate a poor model, values near 0 represent random predictions, and positive values reflect predictions aligned with the presence distribution in the test data. In this study, models with a CBI > 0 within the 95% confidence interval were selected for subsequent analyses.
When examining future-scenario models, it can be challenging to determine the areas that are either more or less suitable for each species. In this study, the distribution maps for each species were assessed using QGIS 3.22.7. Habitat suitability was calculated for each current scenario and 2,100 climate-model scenarios across all species. Then we combined each future scenario model projection with the present-time data to generate a categorical map showing stable, shrinking, and expanding areas.
3 Results
3.1 Model performance and variable importance
The predictive accuracies of all SDM algorithms were generally good across most species. The mean AUC varied between 0.613 and 0.829 and the mean CBI varied between 0.775 and 1.000 (Table 1). This indicates that the habitat suitability suggested by the models resembles the real probability of species occurrence. These metrics also indicate the strength of climate controls on the distribution of each species. In terms of AUC, the predictive accuracies were smaller for Cornus controversa and Kalopanax septemlobus (AUC ≈ 0.62).
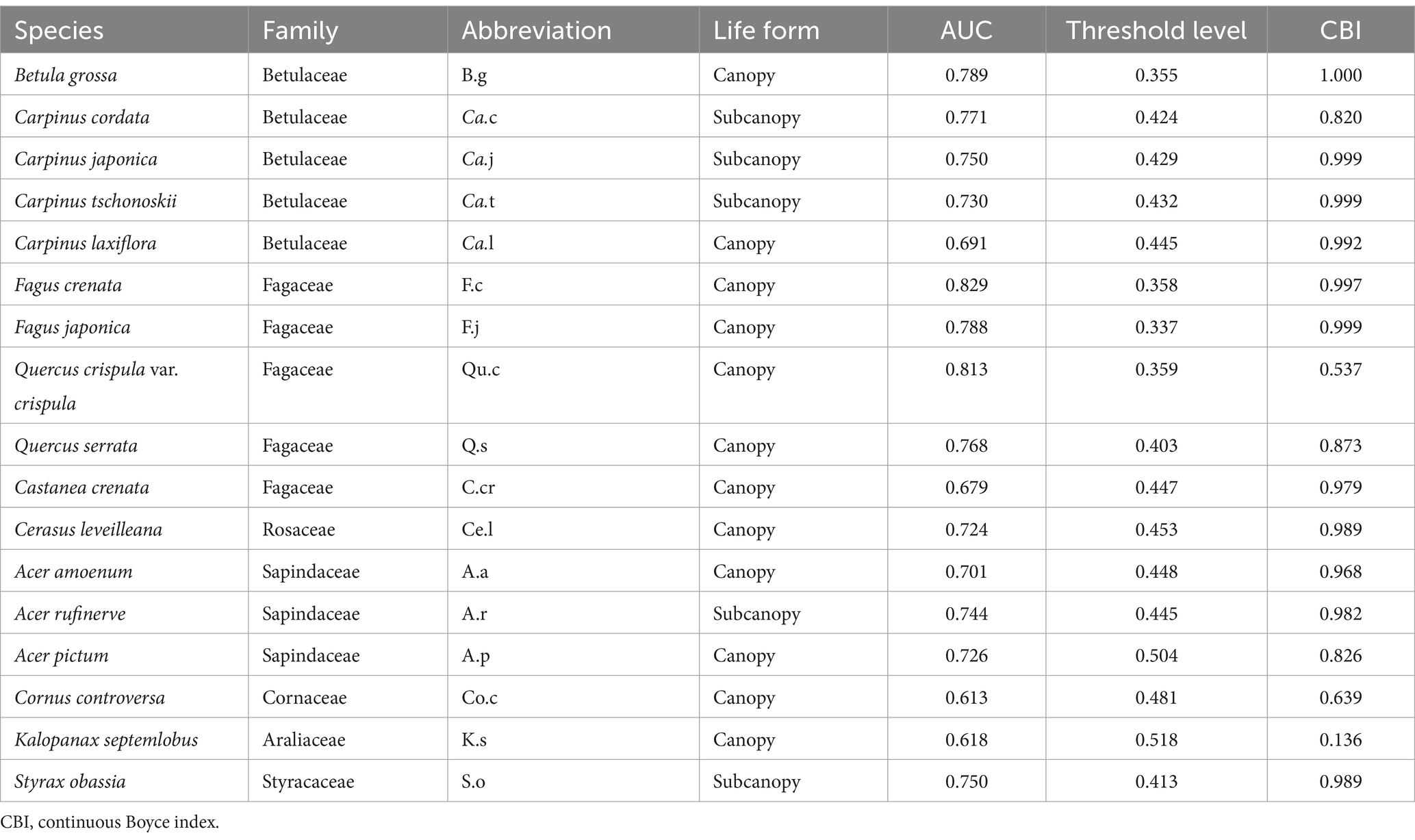
Table 1. Evaluation of algorithm performance based on the mean area under the curve (AUC) for the species distribution models (SDMs) of each tree species.
Variable selection using Maxent reduced the environmental suitability components to 11 variables (Table 2). The importance of each variable differed across species; however, temperature in winter, represented by BIO06 and BIO11, was the most important predictor in more than half of the species. BIO11 (the mean temperature of the coldest quarter) suggests that Carpinus tschonoskii, Fagus crenata, C. crenata, Cerasus leveilleana, and Cornus controversa thrive in locations where the temperature remains at about 0° during winter. BIO06 is related to the minimum temperature of the coldest month and suggests that Carpinus cordata, Carpinus laxiflora, Styrax obassia, and Acer amoenum can survive at temperatures in the −10°C to −5°C range while lower temperatures are much less suitable. The variable BIO05 is related to the maximum temperature of the warmest month and suggests that Q. serrata prefers a range of 20–25°C while Acer rufinerve has a slightly wider range. The majority of species are associated with the mean temperature of the wettest quarter (BIO08). The distributions of F. crenata and Carpinus japonica are mostly influenced by the precipitation in the warmest (BIO18) and coldest (BIO19) quarters. Figure 2 shows the response curve of Cerasus leveilleana while the other response curves are in Appendix 1.
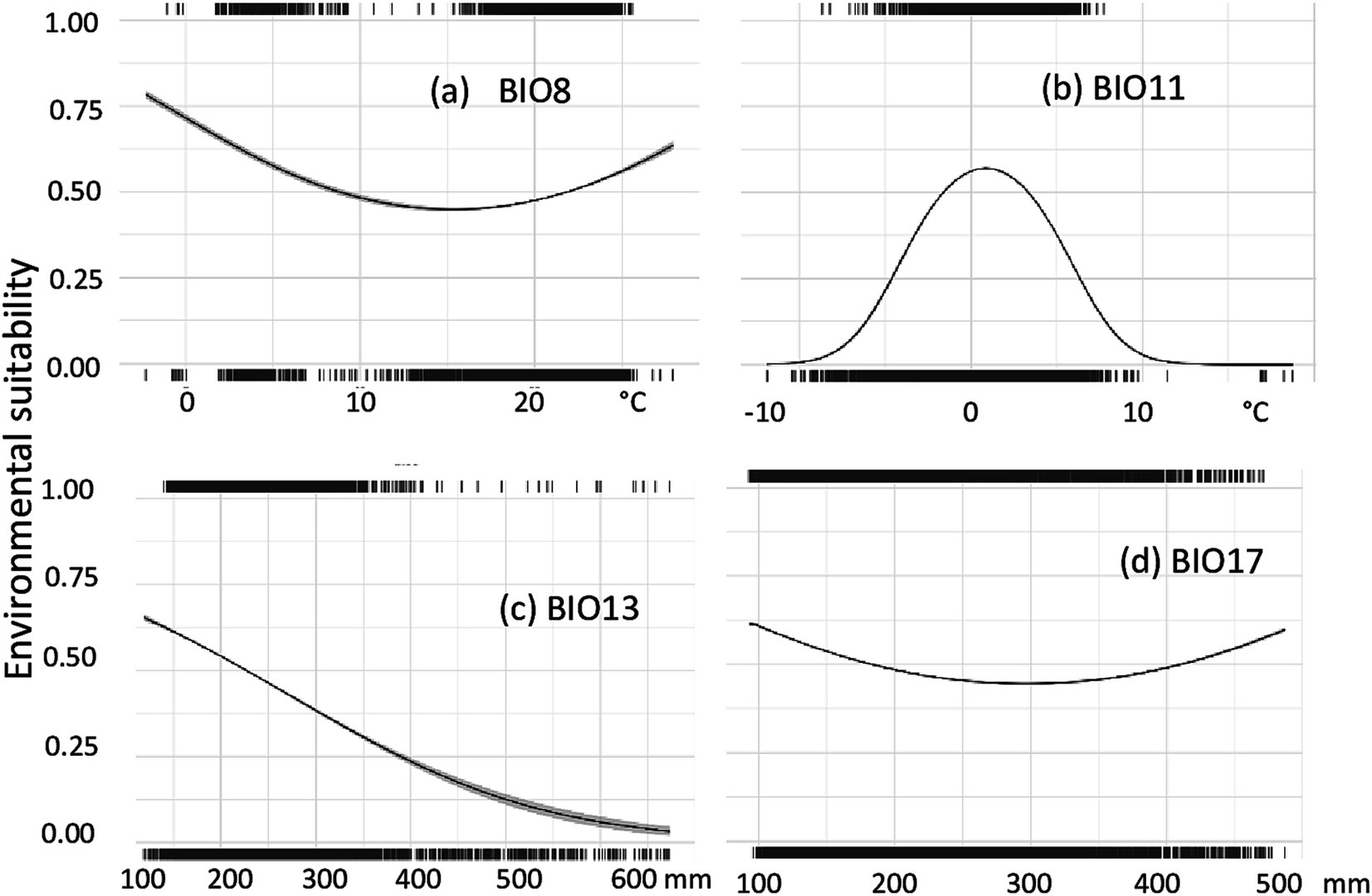
Figure 2. Response curves in the Maxent model of habitat probabilities for Cerasus leveilleana with respect to different bioclimatic variables. The response curves are presented as the means of 100 replicates with standard deviations shown in gray. (A) BIO8, mean temperature of the wettest quarter; (B) BIO11, mean temperature of the coldest quarter; (C) BIO13, precipitation of the wettest month; and (D) BIO17, precipitation of the driest quarter.
3.2 Current and future geographical distribution of major tree species
Figure 3 compares the environmental suitability at present and in 2100. This depicts a future where the species seem to have limited spread. This extreme CO2 emissions scenario (SSP5–8.5) negatively affected all of the major tree species except for Castanea.crenata, A. pictum, and Q. serrata. The distributions of the subcanopy species Carpinus cordata, Carpinus japonica, C. tschonoskii, A. rufinerve, and S. obassia all shrink (Table 1 of Wijenayake et al., 2023).
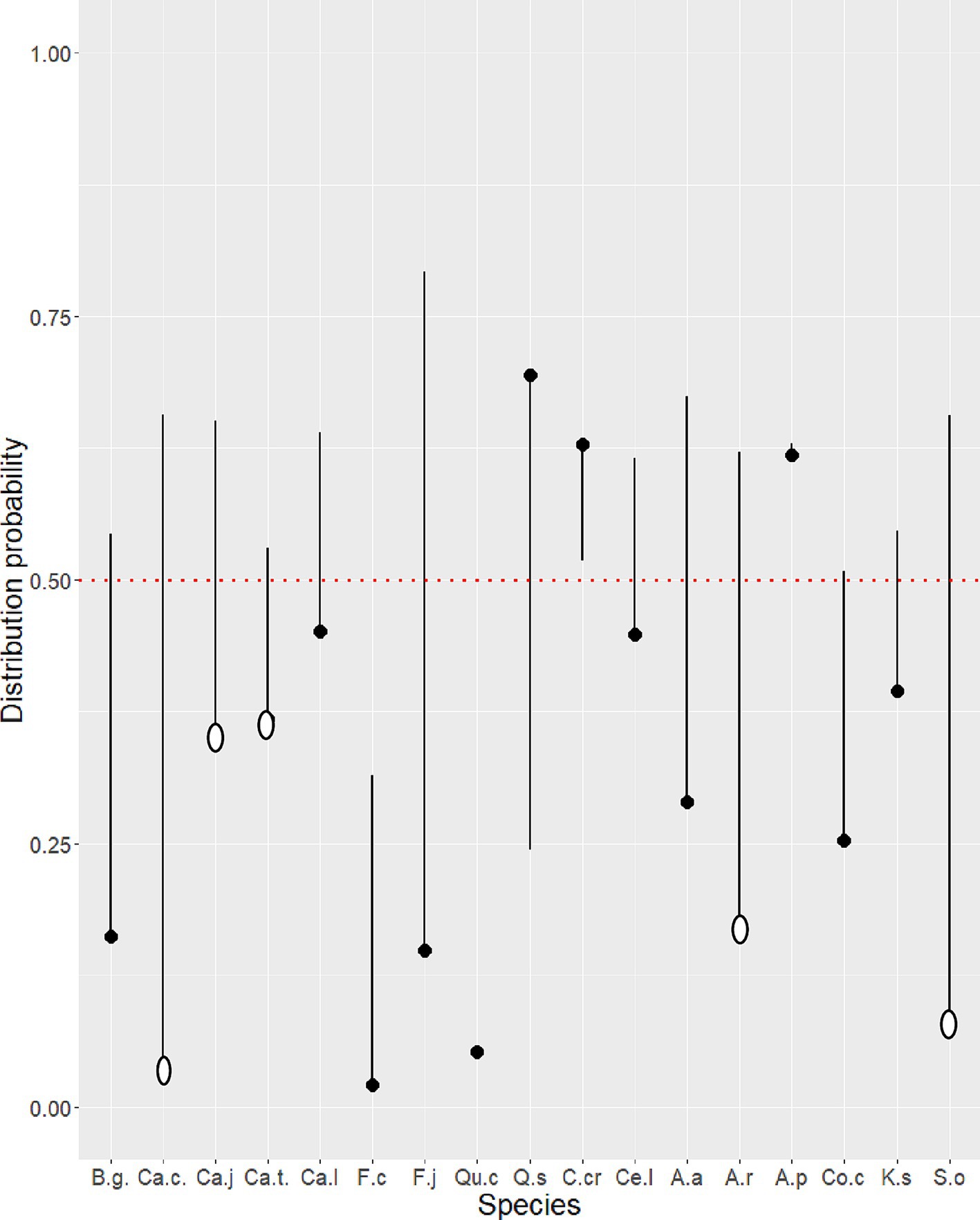
Figure 3. Expected values of distribution probabilities of 16 major species near OFR from the present to 2,100 are shown by the black dots (canopy) and white ovals (subcanopy). The ends of the lines extending from the dots indicate the present values. The red dotted line is the threshold level. Here, only the SSP5–85 scenario is considered.
Figure 4 presents the habitat suitability and difference in (future–present) suitability for two species: C. tschonoskii and Q. serrata. The areas suitable for C. tschonoskii shift inland away from the Pacific Coast, while the habitat suitability of Q. serrata expands under a changing climate. Habitat suitability maps for the other species are in Appendix 2.
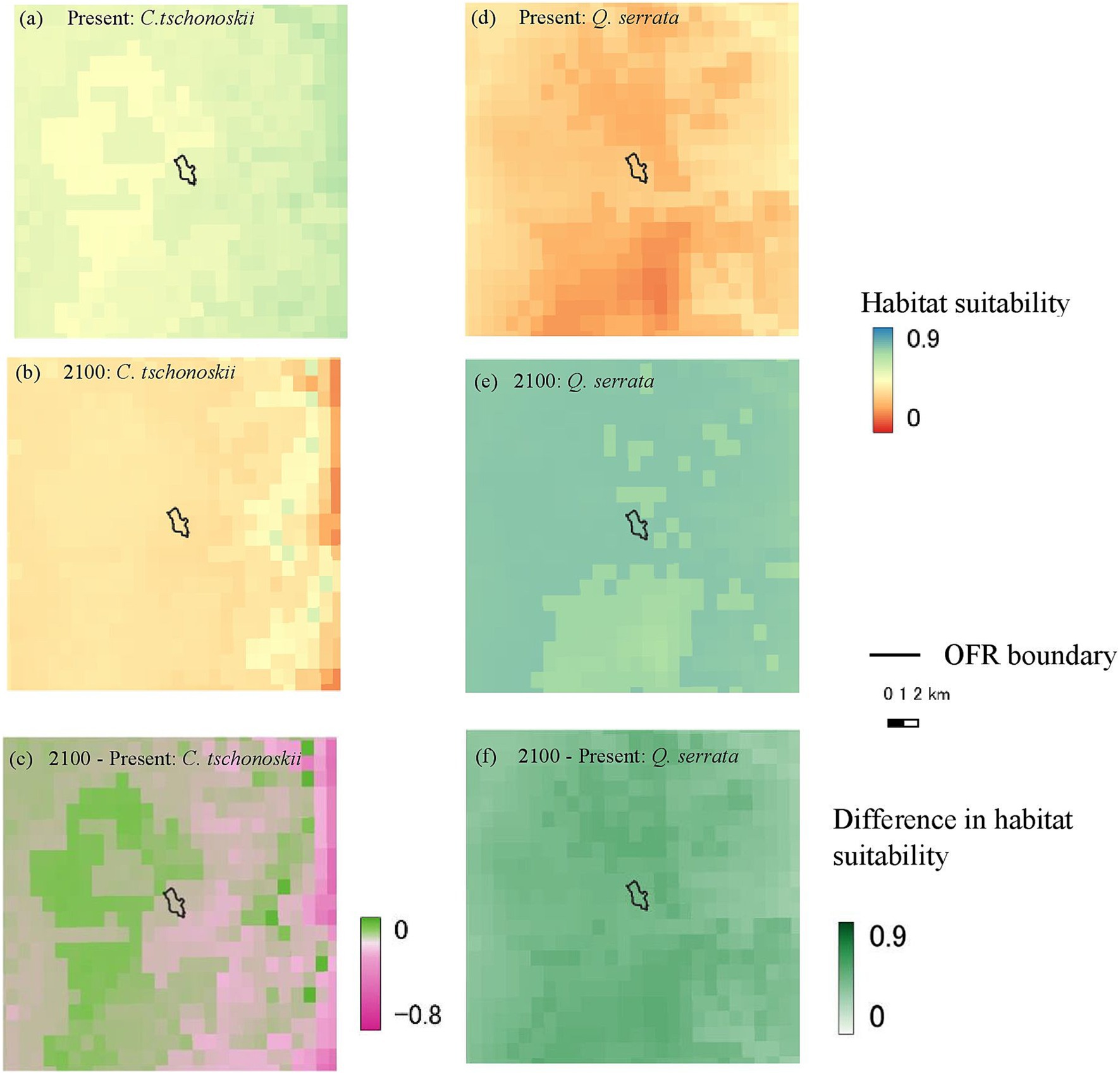
Figure 4. Habitat suitability and difference of habitat suitability maps in and around OFR created using Maxent under the current climate and predicted climate change (SSP5-8.5 pessimistic prediction characterized by high population growth and high levels of greenhouse gas concentrations by the end of 2,100) in 2100 for two species: C. tschonoskii and Q. serrata.
4 Discussion
This study identified the climatic variables that seem most important for determining the habitat suitability of major tree species at present, and under two different future climate change scenarios. The majority of species, including Carpinus. cordata, Carpinus. laxiflora, A. amoenum, S. obassia, Carpinus. tschonoskii, F. crenata, Castanea. crenata, Cerasus. leveilleana, and Cornus. controversa, are affected by winter temperatures (BIO06 and BIO11), which is the most important factor affecting the future distribution of the species. Projected future changes for each species centered around the OFR under two SSP scenarios (a scenario implying low greenhouse gas forcing in the future [SSP2] and a fossil fuel development scenario [SSP5] for 2050 and 2,100) suggest that the distributions of tree species in and around OFR will change until the end of the 21st century. Under both climate scenarios, some structural changes are related to the species composition of the assembly of subcanopy species. However, the uncertainties and potential sources of error in model predictions may arise from complex biotic and abiotic interactions within the community, which are often challenging to capture accurately. Incorporating additional variables, such as terrain and soil characteristics, may only lead to modest improvements in model performance, as these factors might not fully account for the intricate ecological dynamics influencing species distributions.
The literature suggests that the structural complexity of the canopy in old-growth forests facilitates greater resource use efficiency while maintaining significant carbon storage potential (Hardiman et al., 2013; Obata et al., 2023). Near OFR, the subcanopy species C. cordata, A. rufinerve, and S. obassia will disappear completely by 2,100 under SSP5–8.5 while the remaining two subcanopy species (Carpinus japonica and Carpinus tschonoskii) will markedly decrease. In the 2,100 climate mitigation scenario, a similar trend is observed for two subcanopy species: S. obassia and C. cordata. The distribution of the canopy species F. crenata will shrink drastically by the end of this century. Another study of the possible future of F. crenata in Japan identified vulnerable habitats at low elevations on both the Sea of Japan and Pacific Ocean sides (Matsui et al., 2018). The distributions of the canopy species Betula grossa, A. amoenum, C. controversa, and K. septemlobus will shrink remarkably according to projections.
With the potential reductions in the abundance of subcanopy species, the forest structure is expected to change. However, prolonged exposure to unsuitable environmental conditions can lead to the physiological deterioration of trees, disrupting the rhythms of flowering, fruiting, and fertility (Selås et al., 2002; Wright et al., 2022). Consequently, the growth of the next generation of fruiting and juvenile trees may be hindered, resulting in a decline in population density (Niinemets, 2010). Concurrently, competition from species better adapted to warmer environments (e.g., evergreen broadleaved species) may induce changes in forest species composition and structure, accompanied by increased invasion and establishment from external sources, such as the growth of warm-temperate tree species (Lindner et al., 2010).
Stand structure has important implications for forest ecosystems, including the maintenance of wildlife habitat, sustaining biodiversity, nutrient cycling, regulating climate via carbon storage, and affecting forest regeneration (Brassard and Chen, 2006). In this sense, changing climate will likely affect the stability of the plant assembly and the habitats of various mammals and birds will also change. Accordingly, species diversity in the ecosystem surrounding OFR is expected to decrease. Based only on our findings, however, we cannot make a general statement regarding forest ecosystem stability throughout Japan. There may be places where climate change is working against Q. serrata and favoring subcanopy species.
Overall, Q. serrata is projected to remain stable and even expand its distribution, even under extreme climate conditions in 2100. Notably, it is the only one of the 17 species that expands its distribution area under both the climate adaptation and mitigation scenarios (Figure 4). These results suggest a simplified species composition of the canopy layer and a reduced abundance of subcanopy species in OFR in the future. Q. serrata occurs in the intermediate temperate forest zone and is widely distributed in lower and warmer areas, particularly in less snowy environments (Matsui et al., 2018). A demographic study of the life history of OFR indicated that Quercus species may continue to dominate the future landscape, primarily due to the higher survivorship at diameters at breast height of 10–40 cm (Wijenayake et al., 2023). The canopy species A. pictum and C. crenata will also remain stable under SSP5 in 2100.
Taking proactive steps to understand how trees respond to climate, through well-planned tests in specific areas, could bring tangible advantages in the future (Rehfeldt et al., 2014). The findings in Appendix 2 can help identify suitable conservation strategies for the tree species examined here. For example, for the subcanopy species that are expected to undergo considerable loss in the future, efforts could focus on facilitating range expansion to the northwest of OFR, which may enhance their stability facing the consequences of rapid climate change. Furthermore, the northern range limits of evergreen broadleaved tree species are projected to expand to higher elevations and northwards into areas dominated by Quercus (Nakao et al., 2011; Matsui et al., 2018). Our study also suggests that the Fagaceae will show resilience and expand their distributions, particularly Q. serrata, and especially under extreme climate conditions in 2100.
The species C. controversa and C. crenata had lower AUC values, which is a study limitation. C. controversa is a fast-growing, shade-intolerant pioneer species that may have lower correlations with climate variables. C. crenata is a valuable food resource related to agroforestry and it would be affected by past socioeconomic activities rather than bioclimate variables (Freitas et al., 2022). Therefore, it is important to note that the distribution changes are not only affected by climate factors but also by socioeconomic factors such as anthropogenic disturbances (Koyama et al., 2021), and land use history, which could have a lasting impact on forest resilience. For species such as C. controversa with potentially long seed dispersal distance (Wijenayake et al., 2024), the incorporation of spatially dynamic processes such as seed dispersal models should also be important to improve model prediction from landscape perspective. Long-distance dispersal of seeds significantly influences plant population dynamics via enabling vulnerable species to colonize more suitable distant habitats; however, our understanding of its implications at a landscape scale remains limited. For this reason, we excluded seed dispersal factors to minimize complexity. Climate change will increase disturbances in forests, but these disturbances should not be seen only as a threat to stability. They have the potential to enhance tree species diversity, with positive impacts on productivity and other ecosystem functions (Silva Pedro et al., 2016), while leading to changes in habitat quality (Hovenden and Williams, 2010). These vulnerabilities are not necessarily a realistic picture of the area around OFR. Note, however, that the future changes in this area may not be as severe due to topographic features. The current dominant tree species will remain similar, although their relative dominance will change with the climate.
To verify the generality of findings from previous related studies, we figured out sharply contrasting outcomes. Hansen et al. (2001) examined the potential impacts of human-induced climate change on forest biodiversity in the eastern U.S., revealing that habitat suitability for tree species varies based on their individual responses to environmental changes. Their study predicted a northward shift in habitat for many species, ranging from 100 to 530 km, with some species extending their optimal habitats beyond the U.S. border. This contrasts with our findings, where a high-resolution ecosystem-based climate envelope model predicts climate change impacts on forests in British Columbia, emphasizing climate as the primary driver of plant distribution (Hamann and Wang, 2006). By 2050, a significant proportion of present-day beech and sessile oak forests in zonal positions at low altitudes in Central Europe may fall outside their current bioclimatic niches. However, in our case the current dominant tree species are expected to remain, their relative dominance is likely to shift with changing climatic conditions. We believe that our study expanded the generality of knowledge of this field.
4.1 Future aspirations
We used a species distribution model (SDM) approach to analyze the compositional changes in species of old-growth forest landscapes under future climate conditions, but the accuracy of the projected changes needs to be checked. It is necessary to continue monitoring actual changes of OFR and adjust the model accordingly to improve the model prediction. Furthermore, this study did not consider surrounding land use. In OFR, the primary landscape transitioned from expansive grasslands and broadleaved forests to a mosaic of fragmented secondary forests and coniferous plantations (Miyamoto et al., 2011). The canopy trees in plantations remain evergreen, creating consistently darker conditions compared to the varying light conditions in natural old-growth forests, where the light conditions change seasonally (Gonzales and Nakashizuka, 2010). This difference would be a barrier to regeneration by limiting recruitment, particularly for canopy species. It is ideal to integrate studies of the seed dispersal distances of each species to detect barriers to regeneration (Wijenayake et al., 2024). Furthermore, for local forest planning, it may be useful to designate corridors based on climate change scenarios and land-use changes. It is essential to explore strategies that balance biodiversity conservation with climate change mitigation and to validate their effectiveness (Hirata et al., 2024). By integrating models that connect the economy, land use, and biodiversity, we can understand how different socioeconomic factors affect biodiversity directly or indirectly (Ohashi et al., 2019). Incorporating nature-based solutions is essential for understanding the impacts of climate change on species distributions and forest ecosystem dynamics.
5 Conclusion
This study demonstrated the utility of species distribution models for addressing knowledge gaps regarding changes in major tree species under varying climate change scenarios. We project that the canopy species Q. serrata will become more dominant than other canopy species by 2,100 under the baseline climate scenario, potentially leading to shifts in the species composition of the OFR. Consequently, the stability of forest ecosystems and local species diversity may be compromised under future climate change scenarios. This information is crucial for developing vegetation monitoring, ecosystem management, and climate change adaptation pathways. Further refinements should consider factors such as topography and land use changes in the surrounding landscape.
Data availability statement
Publicly available datasets were analyzed in this study. This data can be found here: Japan Biodiversity Mapping Project (J-BMP) database.
Author contributions
PW: Conceptualization, Data curation, Formal analysis, Methodology, Visualization, Writing – original draft. TS: Data curation, Methodology, Software, Validation, Writing – review & editing. AH: Methodology, Software, Validation, Writing – review & editing. TeM: Methodology, Software, Validation, Visualization, Writing – review & editing. YK: Data curation, Resources, Validation, Writing – review & editing. TaM: Conceptualization, Funding acquisition, Investigation, Project administration, Resources, Supervision, Writing – review & editing.
Funding
The author(s) declare that financial support was received for the research, authorship, and/or publication of this article. The JSPS KAKEN supported this work under grant 21H04946.
Acknowledgments
The authors thank Dr. Kazuaki Tsuchiya, for his various support, guidance, and encouragement.
Conflict of interest
The authors declare that the research was conducted in the absence of any commercial or financial relationships that could be construed as a potential conflict of interest.
Generative AI statement
The authors declare that no Gen AI was used in the creation of this manuscript.
Publisher’s note
All claims expressed in this article are solely those of the authors and do not necessarily represent those of their affiliated organizations, or those of the publisher, the editors and the reviewers. Any product that may be evaluated in this article, or claim that may be made by its manufacturer, is not guaranteed or endorsed by the publisher.
Supplementary material
The Supplementary material for this article can be found online at: https://www.frontiersin.org/articles/10.3389/ffgc.2024.1501987/full#supplementary-material
Footnotes
1. ^https://biodiversity-map.thinknature-japan.com/index.html
2. ^https://qgis.org/ja/site/index.html
3. ^https://www.worldclim.org/
4. ^https://biodiversityinformatics.amnh.org/open_source/maxent/
References
Anderson, J. T., and Song, B. H. (2020). Plant adaptation to climate change—where are we? J. Syst. Evol. 58, 533–545. doi: 10.1111/jse.12649
Brassard, B. W., and Chen, H. Y. (2006). Stand structural dynamics of north American boreal forests. Crit. Rev. Plant Sci. 25, 115–137. doi: 10.1080/07352680500348857
Canham, C. D., and Murphy, L. (2017). The demography of tree species response to climate: sapling and canopy tree survival. Ecosphere 8:e01701. doi: 10.1002/ecs2.1701
Chu, C., Lutz, J. A., Král, K., Vrška, T., Yin, X., Myers, J. A., et al. (2019). Direct and indirect effects of climate on richness drive the latitudinal diversity gradient in forest trees. Ecol. Lett. 22, 245–255. doi: 10.1111/ele.13175
Colavito, M. M. (2017). Utilising scientific information to support resilient forest and fire management. Int. J. Wildland Fire 26, 375–383. doi: 10.1071/WF16158
Elith, J., and Leathwick, J. R. (2009). Species distribution models: ecological explanation and prediction across space and time. Annu. Rev. Ecol. Evol. Syst. 40, 677–697. doi: 10.1146/annurev.ecolsys.110308.120159
Elith, J., Phillips, S. J., Hastie, T., Dudík, M., Chee, Y. E., and Yates, C. J. (2011). A statistical explanation of MaxEnt for ecologists. Divers. Distrib. 17, 43–57. doi: 10.1111/j.1472-4642.2010.00725.x
Freitas, T. R., Santos, J. A., Silva, A. P., Martins, J., and Fraga, H. (2022). Climate change projections for bioclimatic distribution of Castanea sativa in Portugal. Agronomy 12:1137. doi: 10.3390/agronomy12051137
Gilliam, F. S. (2016). Forest ecosystems of temperate climatic regions: from ancient use to climate change. New Phytol. 212, 871–887. doi: 10.1111/nph.14255
Gonzales, R. S., and Nakashizuka, T. (2010). Broad-leaf species composition in Cryptomeria japonica plantations with respect to distance from natural forest. For. Ecol. Manag. 259, 2133–2140. doi: 10.1016/j.foreco.2010.02.028
Guisan, A., and Zimmermann, N. E. (2000). Predictive habitat distribution models in ecology. Ecol. Model. 135, 147–186. doi: 10.1016/S0304-3800(00)00354-9
Hamann, A., and Wang, T. (2006). Potential effects of climate change on ecosystem and tree species distribution in British Columbia. Ecology 87, 2773–2786. doi: 10.1890/0012-9658(2006)87[2773:PEOCCO]2.0.CO;2
Hanewinkel, M., Cullmann, D. A., Michiels, H. G., and Kändler, G. (2014). Converting probabilistic tree species range shift projections into meaningful classes for management. J. Environ. Manag. 134, 153–165. doi: 10.1016/j.jenvman.2014.01.010
Hansen, A. J., Neilson, R. P., Dale, V. H., Flather, C. H., Iverson, L. R., Currie, D. J., et al. (2001). Global change in forests: responses of species, communities, and biomes: interactions between climate change and land use are projected to cause large shifts in biodiversity. Bioscience 51, 765–779. doi: 10.1641/0006-3568(2001)051[0765:GCIFRO]2.0.CO;2
Hardiman, B. S., Gough, C. M., Halperin, A., Hofmeister, K. L., Nave, L. E., Bohrer, G., et al. (2013). Maintaining high rates of carbon storage in old forests: a mechanism linking canopy structure to forest function. For. Ecol. Manag. 298, 111–119. doi: 10.1016/j.foreco.2013.02.031
Harger, J. R. E. (1993). Potential limits of human dominated fossil energy based global ecosystems. Chemosphere 27, 907–945. doi: 10.1016/0045-6535(93)90062-A
Hijmans, R. J., Cameron, S. E., Parra, J. L., Jones, P. G., and Jarvis, A. (2005). Very high resolution interpolated climate surfaces for global land areas. Int. J. Climatol. 25, 1965–1978. doi: 10.1002/joc.1276
Hirata, A., Ohashi, H., Hasegawa, T., Fujimori, S., Takahashi, K., Tsuchiya, K., et al. (2024). The choice of land-based climate change mitigation measures influences future global biodiversity loss. Commun. Earth Environ. 5:259. doi: 10.1038/s43247-024-01433-4
Hovenden, M. J., and Williams, A. L. (2010). The impacts of rising CO2 concentrations on Australian terrestrial species and ecosystems. Austral Ecol. 35, 665–684. doi: 10.1111/j.1442-9993.2009.02074.x
IPBES, W. (2019). “Intergovernmental science-policy platform on biodiversity and ecosystem services,” in Summary for policy makers of the global assessment report on biodiversity and ecosystem services of the intergovernmental science-policy platform on biodiversity and ecosystem services. Bonn, Germany: IPBES Secretariat.
Jump, A. S., Marchant, R., and Peñuelas, J. (2009). Environmental change and the option value of genetic diversity. Trends Plant Sci. 14, 51–58. doi: 10.1016/j.tplants.2008.10.002
Koyama, A., Uchida, K., Ozeki, M., Iwasaki, T., Nakahama, N., and Suka, T. (2021). Conservation of endangered and rare plants requires strategies additional to deer-proof fencing for conservation of sub-alpine plant diversity. Appl. Veg. Sci. 24:e12553. doi: 10.1111/avsc.12553
Lindner, M., Maroschek, M., Netherer, S., Kremer, A., Barbati, A., Garcia-Gonzalo, J., et al. (2010). Climate change impacts, adaptive capacity, and vulnerability of European forest ecosystems. For. Ecol. Manag. 259, 698–709. doi: 10.1016/j.foreco.2009.09.023
Macreadie, P. I., Costa, M. D., Atwood, T. B., Friess, D. A., Kelleway, J. J., Kennedy, H., et al. (2021). Blue carbon as a natural climate solution. Nat. Rev. Earth Environ. 2, 826–839. doi: 10.1038/s43017-021-00224-1
Masaki, T., Kitagawa, R., Nakashizuka, T., Shibata, M., and Tanaka, H. (2021). Interspecific variation in mortality and growth and changes in their relationship with size class in an old-growth temperate forest. Ecol. Evol. 11, 8869–8881. doi: 10.1002/ece3.7720
Masaki, T., Suzuki, W., Niiyama, K., Iida, S., Tanaka, H., and Nakashizuka, T. (1992). Community structure of a species-rich temperate forest, Ogawa forest reserve, Central Japan. Vegetatio 98, 97–111. doi: 10.1007/BF00045549
Matsui, T., Nakao, K., Higa, M., Tsuyama, I., Kominami, Y., Yagihashi, T., et al. (2018). Potential impact of climate change on canopy tree species composition of cool-temperate forests in Japan using a multivariate classification tree model. Ecol. Res. 33, 289–302. doi: 10.1007/s11284-018-1576-2
Miyamoto, A., Sano, M., Tanaka, H., and Niiyama, K. (2011). Changes in forest resource utilization and forest landscapes in the southern Abukuma Mountains, Japan during the twentieth century. J. For. Res. 16, 87–97. doi: 10.1007/s10310-010-0213-x
Mizoguchi, Y., Morisawa, T., and Ohtani, Y. (2002). Climate in ogawa forest reserve. Ogawa Forest Reserve of Japan: Diversity and interaction in a temperate forest community, 11–18.
Mo, L., Zohner, C. M., Reich, P. B., and Liang, J., Miguel, S. de, Nabuurs, G. J., Renner, S. S., et al., (2023). Integrated global assessment of the natural forest carbon potential. Nature, 624, 92–101, doi: 10.1038/s41586-023-06723-z
Nakao, K., Higa, M., Tsuyama, I., Matsui, T., Horikawa, M., and Tanaka, N. (2013). Spatial conservation planning under climate change: using species distribution modeling to assess priority for adaptive management of Fagus crenata in Japan. J. Nat. Conserv. 21, 406–413. doi: 10.1016/j.jnc.2013.06.003
Nakao, K., Matsui, T., Horikawa, M., Tsuyama, I., and Tanaka, N. (2011). Assessing the impact of land use and climate change on the evergreen broad-leaved species of Quercus acuta in Japan. Plant Ecol. 212, 229–243. doi: 10.1007/s11258-010-9817-7
Nakashizuka, T., Iida, S., Tanaka, H., Shibata, M., Abe, S., Masaki, T., et al. (1992). Community dynamics of Ogawa Forest reserve, a species rich deciduous forest, Central Japan. Vegetatio 103, 105–112. doi: 10.1007/BF00047696
Niinemets, Ü. (2010). Responses of forest trees to single and multiple environmental stresses from seedlings to mature plants: past stress history, stress interactions, tolerance and acclimation. For. Ecol. Manag. 260, 1623–1639. doi: 10.1016/j.foreco.2010.07.054
Obata, A., Yoshida, T., and Hiura, T. (2023). Estimation of stand biomass and species-specific biomass in Japanese northern mixed forests in 1920–1930s: understanding environmental factors affecting carbon sequestration before recent climate change. Ecol. Indic. 154:110495. doi: 10.1016/j.ecolind.2023.110495
Ohashi, H., Hasegawa, T., Hirata, A., Fujimori, S., Takahashi, K., Tsuyama, I., et al. (2019). Biodiversity can benefit from climate stabilization despite adverse side effects of land-based mitigation. Nat. Commun. 10:5240. doi: 10.1038/s41467-019-13241-y
Ohsawa, M. (1993). Latitudinal pattern of mountain vegetation zonation in southern and eastern Asia. J. Veg. Sci. 4, 13–18. doi: 10.2307/3235728
Pettorelli, N., Graham, N. A., Seddon, N., da Cunha, M., Bustamante, M., Lowton, M. J., et al. (2021). Time to integrate global climate change and biodiversity science-policy agendas. J. Appl. Ecol. 58, 2384–2393. doi: 10.1111/1365-2664.13985
Rehfeldt, G. E., Leites, L. P., St Clair, J. B., Jaquish, B. C., Sáenz-Romero, C., López-Upton, J., et al. (2014). Comparative genetic responses to climate in the varieties of Pinus ponderosa and Pseudotsuga menziesii: clines in growth potential. For. Ecol. Manag. 324, 138–146. doi: 10.1016/j.foreco.2014.02.041
Scheffer, M., Hirota, M., Holmgren, M., Van Nes, E. H., and Chapin, F. S. 3rd. (2012). Thresholds for boreal biome transitions. Proc. Natl. Acad. Sci. 109, 21384–21389. doi: 10.1073/pnas.1219844110
Selås, V., Piovesan, G., Adams, J. M., and Bernabei, M. (2002). Climatic factors controlling reproduction and growth of Norway spruce in southern Norway. Can. J. For. Res. 32, 217–225. doi: 10.1139/x01-192
Shibata, M., Masaki, T., Tanaka, H., Niiyama, K., Iida, S., Abe, S., et al. (2010). Effects of abiotic and biotic factors and stochasticity on tree regeneration in a temperate forest community. Ecoscience 17, 137–145. doi: 10.2980/17-2-3163
Shiono, T., Kubota, Y., and Kusumoto, B. (2021). Area-based conservation planning in Japan: the importance of OECMs in the post-2020 global biodiversity framework. Global Ecol. Conserv. 30:e01783. doi: 10.1016/j.gecco.2021.e01783
Shitara, T., Fukui, S., Matsui, T., Momohara, A., Tsuyama, I., Ohashi, H., et al. (2021). Climate change impacts on migration of Pinus koraiensis during the quaternary using species distribution models. Plant Ecol. 222, 843–859. doi: 10.1007/s11258-021-01147-z
Silva Pedro, M., Rammer, W., and Seidl, R. (2016). A disturbance-induced increase in tree species diversity facilitates forest productivity. Landsc. Ecol. 31, 989–1004. doi: 10.1007/s10980-015-0317-y
Tanaka, N., Matsui, T., Shimada, K., Yagihashi, T., and Taoda, H. (2005). Constructing vegetation database useful for assessing impact of climate changes in Japan. J. Agri. Meteorol. 60, 433–438. doi: 10.2480/agrmet.433
Tanaka, N., Nakao, K., Tsuyama, I., Higa, M., Nakazono, E., and Matsui, T. (2012). Predicting the impact of climate change on potential habitats of fir (Abies) species in Japan and on the east Asian continent. Procedia Environ. Sci. 13, 455–466. doi: 10.1016/j.proenv.2012.01.039
Tang, C. Q., Matsui, T., Ohashi, H., Dong, Y. F., Momohara, A., Herrando-Moraira, S., et al. (2018). Identifying long-term stable refugia for relict plant species in East Asia. Nat. Commun. 9:4488. doi: 10.1038/s41467-018-06837-3
Tang, Y., Winkler, J. A., Viña, A., Liu, J., Zhang, Y., Zhang, X., et al. (2018). Uncertainty of future projections of species distributions in mountainous regions. PLoS One 13:e0189496. doi: 10.1371/journal.pone.0189496
Thom, D., Rammer, W., and Seidl, R. (2017). The impact of future forest dynamics on climate: interactive effects of changing vegetation and disturbance regimes. Ecol. Monogr. 87, 665–684. doi: 10.1002/ecm.1272
Thompson, I., Mackey, B., McNulty, S., and Mosseler, A. (2009). Forest resilience, biodiversity, and climate change. Montreal: secretariat of the convention on biological diversity.
Warren, R., Price, J., Graham, E., Forstenhaeusler, N., and VanDerWal, J. (2018). The projected effect on insects, vertebrates, and plants of limiting global warming to 1.5 C rather than 2 C. Science 360, 791–795. doi: 10.1126/science.aar3646
Wijenayake, P. R., Masaki, T., and Hirata, A. (2024). Effects of stochastic intraspecific seed dispersal variation on dispersal distance predictions in a temperate forest in Japan. J. Asia-Pacific Biodiversity 17, 800–806. doi: 10.1016/j.japb.2024.06.004
Wijenayake, P. R., Masaki, T., Shibata, M., and Kubota, Y. (2023). Does life form affect tree species assembly? A demographic study across the life history of a temperate forest in Japan. Ecosphere 14:e4579. doi: 10.1002/ecs2.4579
Wright, B. R., Franklin, D. C., and Fensham, R. J. (2022). The ecology, evolution and management of mast reproduction in Australian plants. Aust. J. Bot. 70, 509–530. doi: 10.1071/BT22043
Yonekura, K., and Kajita, T. (2003). Plant Japanese name-scientific name index YList. Available at:http://ylist.info/index.html. Accessed March 20, 2024.
Yoshinaga, S., Takahashi, M., and Aizawa, S. (2002). “Landforms and soil characteristics in Ogawa Forest reserve” in Diversity and interaction in a temperate forest community: Ogawa forest Reserve of Japan (Springer Japan: Tokyo), 19–26.
Keywords: climate change, Ogawa Forest Reserve, tree species distribution, canopy, subcanopy species
Citation: Wijenayake PR, Shitara T, Hirata A, Matsui T, Kubota Y and Masaki T (2025) Changes in forest ecosystem stability under climate change in a temperate landscape. Front. For. Glob. Change. 7:1501987. doi: 10.3389/ffgc.2024.1501987
Edited by:
Iva Hůnová, Czech Hydrometeorological Institute, CzechiaReviewed by:
Zongzheng Chai, Guizhou University, ChinaFrank S. Gilliam, University of West Florida, United States
Copyright © 2025 Wijenayake, Shitara, Hirata, Matsui, Kubota and Masaki. This is an open-access article distributed under the terms of the Creative Commons Attribution License (CC BY). The use, distribution or reproduction in other forums is permitted, provided the original author(s) and the copyright owner(s) are credited and that the original publication in this journal is cited, in accordance with accepted academic practice. No use, distribution or reproduction is permitted which does not comply with these terms.
*Correspondence: Pavithra Rangani Wijenayake, cGF2aXRocmEucmFuZ2FuaUBnbWFpbC5jb20=
†PRESENT ADDRESS: Pavithra Rangani Wijenayake,National Institute for Environmental Studies, Tsukuba, Japan