- 1Department of Biology, High Point University, High Point, NC, United States
- 2Department of Biology, Faculty of Natural Sciences, Universidad del Rosario, Bogotá, Colombia
- 3Department of Biology, Wake Forest University, Winston-Salem, NC, United States
Observations and models indicate that human activity is altering cloud patterns on a global scale. Clouds impact incident visible and infrared radiation during both day and night, driving daily and seasonal variability in plant temperatures—a fundamental driver of all physiological processes. To understand the impacts of changing cloud patterns on essential plant-based processes such as carbon sequestration and food production, changes in local cloud regimes must be linked, via ecophysiology, with affected plant systems. This review provides a comprehensive treatment of cloud effects (apart from precipitation) on fundamental ecophysiological processes that serve as the basis of plant growth and reproduction. The radiative effects of major cloud types (cumulus, stratus, cirrus) are differentiated, as well as their relative impacts on plant microclimate and physiology. Cloud regimes of major climate zones (tropical, subtropical, temperate, polar) are superimposed over recent changes in cloud cover and primary productivity. The most robust trends in changing global cloud patterns include: (i) the tropical rain belt (comprised mostly of deep convective clouds) is narrowing, shifting latitudinally, and strengthening, corresponding with shorter but more intense rainy seasons, increased clouds and precipitation in some parts of the tropics, and decreases in others; (ii) tropical cyclones are increasing in intensity and migrating poleward; (iii) subtropical dry zones are expanding, resulting in fewer clouds and drier conditions at these latitudes; (iv) summer mid-latitude storm tracks are weakening and migrating poleward, and clouds in temperate regions are decreasing; and (v) clouds over the Arctic are increasing. A reduction in coastal fog and low clouds (including those associated with montane cloud forests) have also been observed, although these trends can be partially attributed to local patterns of deforestation, urbanization, and/or reductions in aerosols associated with clean air initiatives. We conclude by highlighting gaps in the cloud-ecophysiology literature in order to encourage future research in this under-studied area.
1 Introduction
Clouds cover approximately 71% of the Earth’s surface and play an important role in the energy balance of the planet (L’Ecuyer et al., 2019). By reflecting incoming shortwave radiation back into space, clouds cool the planet during the day, and through absorption and re-emission of longwave, infrared radiation emitted by the Earth’s surface and atmosphere, also contribute to its warming. Tropospheric warming can further impact cloud phase (liquid/ice) and height, either dampening or amplifying warming (i.e., cloud feedback effects) (Ceppi et al., 2017; Ceppi and Nowack, 2021). Through these radiative effects, clouds contribute to daily and seasonal variability in plant temperatures, a fundamental driver of all physiological processes (Figure 1). Clouds also impact plant water status directly, through foliar uptake of fog/cloud water, and indirectly, as irradiance impacts evaporation and soil moisture (Figure 1).
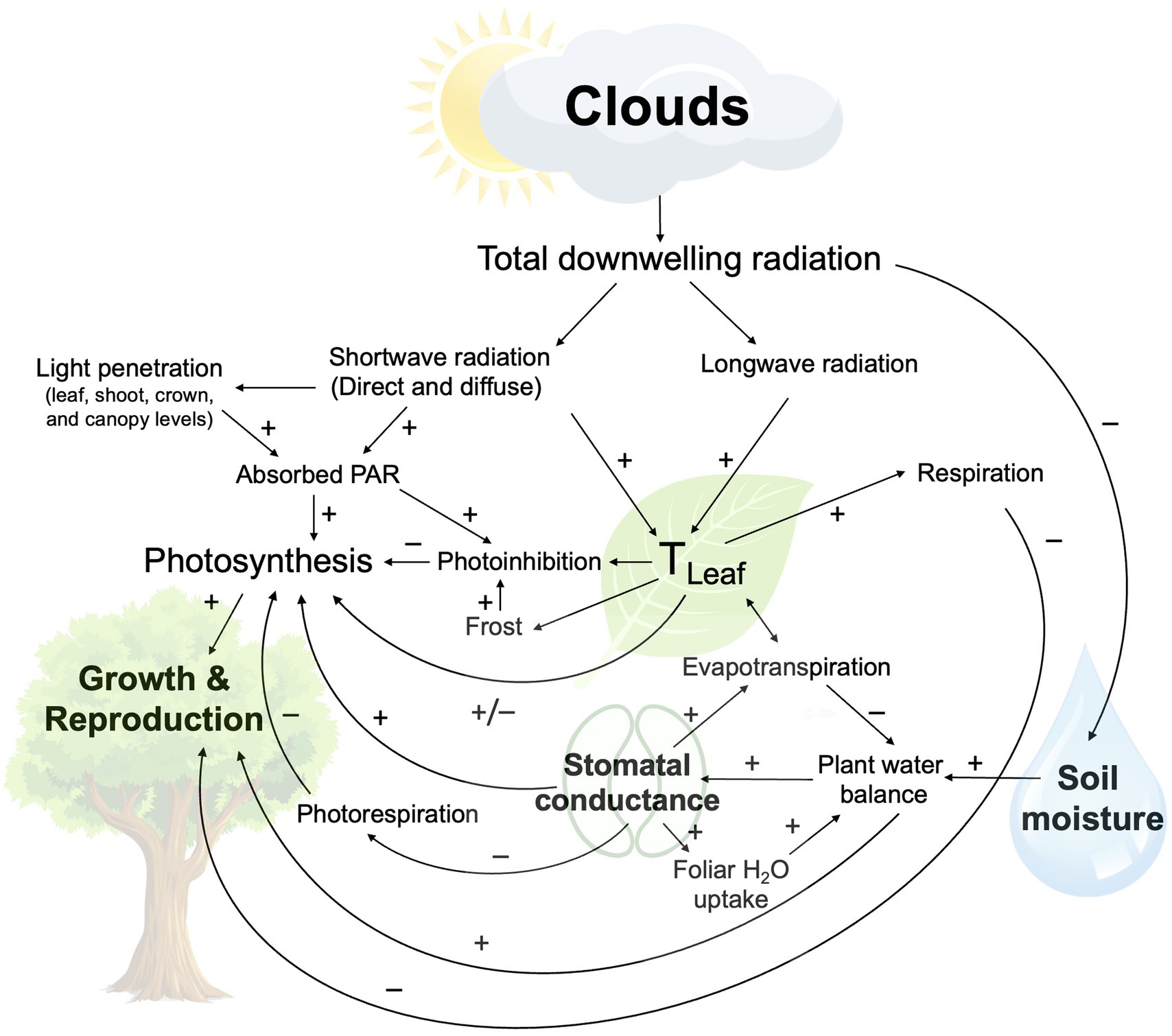
Figure 1. Schematic summary of cloud effects on daytime plant ecophysiology (excluding precipitation). Included are photosynthetically active radiation (PAR) and leaf temperature (TLeaf).
Changes in clouds under CO2-forced warming have been considered one the most critically important, but also most challenging, aspects of climate modeling (Warren et al., 2007). Cloud feedback effects, specifically, represent the single largest source of uncertainty in climate models (Ceppi et al., 2017). For these reasons, clouds are considered a “wild card” of successful global climate change models (Scholes et al., 2015). Yet, while uncertainty remains regarding the sensitivity of cloud feedback effects, robust predictions regarding changes in global cloud patterns have emerged over the past decade. Some of these track changes in large-scale air circulation patterns associated with warmer temperatures (Norris et al., 2016; Grise and Davis, 2020), while others are associated with local factors (e.g., deforestation, aerosols associated with pollution, urban heat island effects) (Yan et al., 2020; Watson-Parris et al., 2022; Xu et al., 2022). Linking changes in global and regional cloud patterns with the predicted responses of affected plant systems is critical for developing accurate carbon assimilation models (Schneider et al., 2017), as well as informing local and global policy makers, conservation efforts, and agricultural communities.
The effects of clouds and aerosols on plant microclimate and productivity were reviewed by Kanniah et al. (2012) and more recently, Durand et al. (2021). These reviews focused primarily on shortwave radiative impacts that affect photosynthesis and productivity, such as diffuse light fertilization and changes in spectral composition. However, their treatment of clouds was largely generalized. As acknowledged by both studies, cloud radiative effects vary markedly with factors such as cloud-base altitude, phase state, number of cloud layers, tropospheric moisture content, size of water droplets or ice particles, and the concentration and physical properties of condensation nuclei. Furthermore, physiological impacts of clouds extend beyond the shortwave radiative effects on photosynthesis, and also include longwave radiative impacts (which are especially important at nighttime), as well as direct and indirect impacts on plant water status. In the current review, we aim to add to the discussion of cloud impacts on plant physiology in the context of climate change by: (i) differentiating between the radiative impacts of different major cloud types (e.g., cirrus, cumulus, stratus) (Section 2); (ii) expanding the discussion of cloud effects on shortwave (SW) radiation and plant productivity to include longwave and water-related impacts (excluding precipitation) (Section 3); and (iii) overlaying changes in global cloud cover with observed and predicted ecophysiological responses of plants in affected ecosystems (Section 4).
2 Cloud radiative effects vary by cloud regime
A substantial amount of research has evaluated the impacts of sunlight incidence on individual leaves due to leaf orientation, mutual shading among leaves of the same plant, or from shading from neighboring individuals of the same or other species (summarized in Loreto et al., 2004; Niinemets and Sack, 2006). However, fewer studies have considered changes in these same dynamics in incident sunlight due to clouds. Geographic regions vary distinctly in local cloud regimes—a term which encompasses both total cloud amount and the relative frequency of occurrence of major cloud types (Jakob and Tselioudis, 2003). Because specific cloud regimes tend to exhibit comparable micro- and macro-physical properties (Sedlar et al., 2021), classifying cloud effects by regime can be useful for extrapolating physiological findings to lesser-studied, but meteorologically-similar ecosystems, as well as fine-tuning predicted responses of native, invasive, and agricultural plant systems to climate change.
Clouds may be characterized by height (low, mid, and high-level clouds), optical depth (i.e., the ratio of irradiance at the surface relative to a clear day), or form (e.g., cumulus, stratus, and cirrus; Figure 2). Low clouds (cloud top pressure, or Pc > 680 mb) are primarily composed of water droplets, and include cumulus and low stratus clouds (e.g., fog). Mid-level clouds are often multi-state, comprised of both water droplets and/or ice crystals, and include high cumulus and stratus (i.e., altocumulus and altostratus, respectively) (Chen et al., 2000). High clouds (Pc < 440 mb) are composed of ice particles, and include cirrus, cirrostratus, and anvil-topped clouds associated with deep convection (Chen et al., 2000). While ground-level measurements of shortwave (270–3,000 nm) radiation under major cloud types are readily available, measurements of photosynthetically active wavebands specifically (i.e., 400–700 nm), are more scattered in the literature. In the sections below, we take care to differentiate between SW and photosynthetically active radiation (PAR) measurements, in order to highlight gaps in the literature.
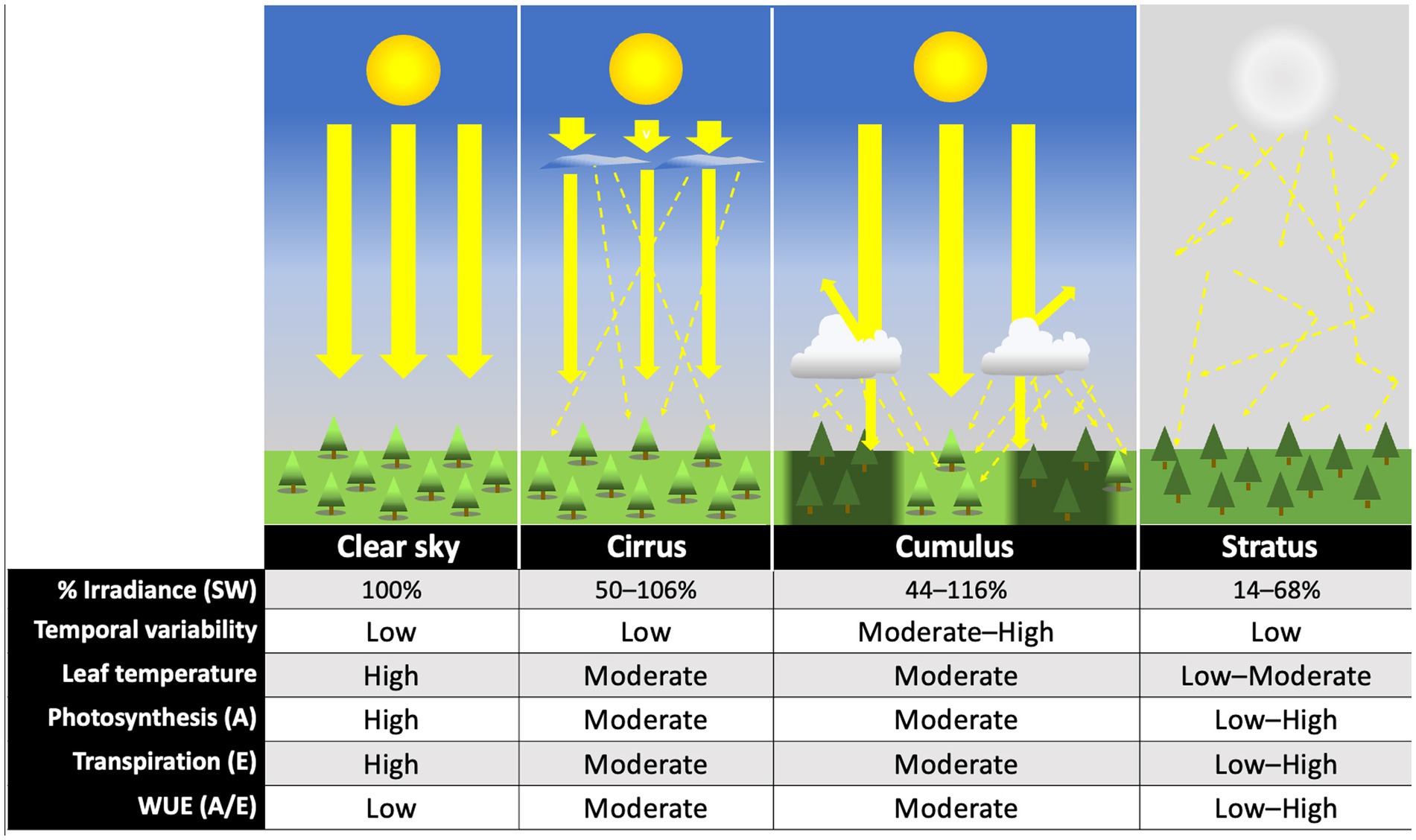
Figure 2. Comparative effects of major cloud types on light environment and plant physiological processes. Illustrations represent generalized effects of each cloud type on direct (large, solid yellow arrows) and diffuse (thin, dashed yellow arrows) irradiance. Percent transmitted shortwave (SW) (270–3,000 nm) radiation relative to clear sky values under each cloud type are based on 10th–90th percentile measurements for high (cirrus), low cumulus, and low stratus reported in Figure 7 of Sedlar et al. (2021). Temporal variability is based on the standard deviation of scaled observed irradiance for cirrus, stratus, and cumulus skies reported as bounding boxes in Figure 5 of Duchon and O’Malley (1999). “Low” values represent a standard deviation below 100 W m−2 during a continuous 21 min interval, “medium” between 101–300, and “high” above 301 W m−2. Leaf temperature and physiological effects are theoretical (rough estimates), and represent predicted, relative responses of a C3 plant to changes in light quality and quantity, under optimal temperature and water conditions. Photosynthetic carbon assimilation (A) and transpiration (E) assume individual-plant (not ecosystem-level) measurements. Instantaneous water use efficiency, WUE (A/E), is also estimated based on these values. Images not drawn to scale.
2.1 Sunlight intensity
Clouds can generate a remarkable range of sunlight intensities at the Earth’s surface compared to clear sky conditions, often well beyond the variation dictated by latitude or time of day (Gu et al., 2001). During the daytime, albedo effects of clouds exceed their reduction of longwave radiation emissions into space (greenhouse effects), resulting in a net cooling effect on the planet (Ceppi and Nowack, 2021). Nearly all clouds reduce direct-beam, shortwave radiation incident on the Earth’s surface when they obstruct the solar disk (albedo effects), perhaps with the exception of subvisual cirrus clouds (Sassen, 2002). Cloud shade is greatest when cloud cover fraction is high, clouds are in the vicinity of the solar disk, the sun is low on the horizon, and clouds exhibit high optical thickness (i.e., greater opacity; calculated as the logarithm of the ratio of incident to transmitted radiation) (Chen et al., 2000; Tzoumanikas et al., 2016). Optical thickness can be affected by phase state and size of cloud particles, and interaction with aerosols (Ceppi et al., 2017; Durand et al., 2021; Sedlar et al., 2021). Clouds composed of large water droplets have the highest optical thickness and include low stratus clouds (including fog and cloud immersion), clouds associated with deep convection (e.g., cumulus congestis), and rain-bearing (nimbus) clouds (Chen et al., 2000; Sedlar et al., 2021).
The effects of different major cloud types on transmission of total shortwave radiation are summarized in Figure 2, based on the findings of Sedlar et al. (2021). No single study to our knowledge has similarly characterized the effects of different cloud types on transmission of photosynthetically active wavelengths specifically. Direct and diffuse photosynthetic photon flux densities (PPFDs) under cloud immersion/fog have been reported in several studies (e.g., Letts and Mulligan, 2005; Eugster et al., 2006; Reinhardt and Smith, 2008a,b; Letts et al., 2010; Berry and Smith, 2012), and correspond with moderate to dramatic reductions in PAR, often interspersed with bright intervals during cloud gaps (e.g., Sanchez et al., 2014b). Photosynthetic photon flux densities associated with fair-weather cumulus clouds are also available, and indicate a wide range of optical densities for this cloud type (transmitting 24–94% clear sky PPFDs) (Hughes et al., 2015; Hernandez-Moreno et al., 2017; Bayeur et al., 2018). No studies to our knowledge have reported continuous PPFD measurements under deep convection clouds (although see Figure 3), nimbus clouds, cirrus clouds, or contrails. The latter is important since contrails associated with air traffic are expected to continue increasing at a rate of 2–5% per annum through 2050 (Penner et al., 1999; Schröder et al., 2000). Also, cirrus cloud thinning is a geo-engineering strategy proposed for mitigating global warming (Mitchell and Finnegan, 2009).
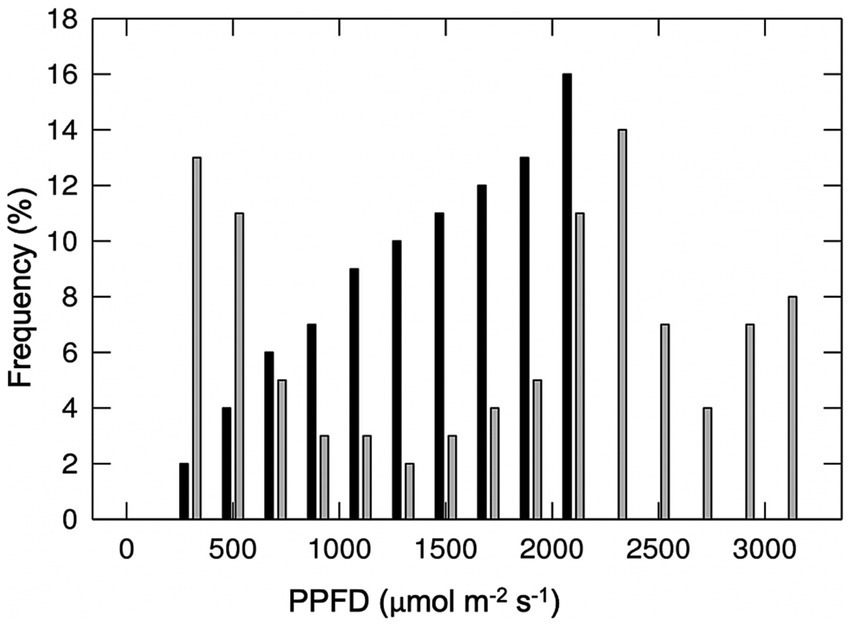
Figure 3. Frequency distribution of total (direct plus diffuse) photosynthetic photon flux density (PPFD) during a clear day (black bars) and a typical afternoon (1,200–1,800 h) with cumulonimbus clouds (grey bars) measured on July 26 and August 17, 2017 (3,654 m elevation), Medicine Bow Mountains, southeast Wyoming, United States (from Smith and Berry, 2013).
In addition to reducing incoming SW radiation through albedo effects, broken or scattered clouds may also amplify incoming sunlight to levels that exceed clear sky measurements (e.g., Duchon and O’Malley, 1999; Hughes et al., 2015; Sedlar et al., 2021). This is due to the combination of direct and diffuse radiation that occurs when sunlight reflected off nearby clouds combines with direct-beam sunlight transmitted between the clouds (discussed further in Section 2.2.1). Indeed, one of the classification criteria used by Duchon and O’Malley (1999) to identify cumulus clouds using surface-measurements was the presence of at least one value in the time series that exceeded clear sky values. Sedlar et al. (2021) similarly reported SW radiation values >120% clear sky levels on measurement days with low cumulus clouds. The effect of alternating high and low solar intensities on photosynthesis is revisited in our discussion of temporal dynamics (Section 2.5) and photoinhibition (Section 3.2.5).
2.2 Directionality
On completely clear days, sunlight is composed primarily of direct (or collimated) beams of sunlight. Clouds and aerosols increase Rayleigh and Mie scattering, changing its directionality and rendering sunlight more diffuse (for more detailed information, see Durand et al., 2021). The directionality of sunlight is an important factor influencing penetration of sunlight into closed crowns and canopies (Alton et al., 2007). The diffuse fraction of sunlight approaches 100% when the outline of the solar disk is completely obscured by the cloud (Durand et al., 2021). The diffuse fraction of total PPFD ranges between 8–16% on completely clear days, 20–40% on partly cloudy days in between cloud shade intervals, and 60–100% under cumulus cloud shade or overcast skies (Dye, 2004; Urban et al., 2014; Hughes et al., 2015; Hernandez-Moreno et al., 2017). No studies to our knowledge have reported the diffuse fraction of photosynthetically active radiation (PAR) specifically under cirrus clouds or contrails. The impacts of diffuse PPFDs on photosynthesis are discussed in Section 3.2.2.
2.2.1 Combining direct and diffuse-beam radiation: the “cloud gap effect”
As previously mentioned, the combination of direct beam with diffuse sunlight reflected by nearby clouds can result in PPFDs higher than clear sky levels during cloud gaps on cloudy days, even exceeding the Solar Constant (measured outside the atmosphere) (Schade et al., 2007; Piedehierro et al., 2014; Sanchez et al., 2014b; Hughes et al., 2015). This phenomenon has been referred to as the ‘cloud gap effect’ (Gu et al., 1999, 2001). According to Piacentini et al. (2011), the requisites for cloud enhancement of sunlight include (1) a cumulus cloud field that is not directly obstructing the solar disk, (2) 50–90% cloud cover, and (3) dense clouds that favor multiple scattering events. However, contrails and cirrus clouds have also been reported to cause solar enhancement (Duchon and O’Malley, 1999; Feister and Shields, 2005; Tzoumanikas et al., 2016), and some studies have observed enhancement even when the solar disk is partly obscured (e.g., Schade et al., 2007; Mateos et al., 2013).
2.2.2 Focusing and amplifying sunlight: sun leaves and cumulonimbus clouds
Sunlight enhancement of nearly 1.4x clear sky total PPFDs have been observed in association with cumulonimbus clouds (Smith and Berry, 2013; Figure 3). Similarly, extraordinary values have been reported for sunlight data collected in a Brazilian grassland meadow (Gu et al., 2001), above a tropical forest (Dye et al., 2008), and in the Andean páramo (Sanchez et al., 2014b). While these high values may be attributed to processes similar to the cloud gap effect (Körner, 2003), it is also possible that large cumulonimbus clouds may generate photon amplification via channeling of photons through lengthy (kilometers-long) cloud tunnels, or, at the edges of grouped cumulonimbus clouds (SG Warren, pers. comm.). Similar propagation of absorbed sunlight occurs within the palisade cells of leaves, which focus photons through the column-like cells to maximize penetration into the lamina and photosynthetic cells deeper in the leaf (Smith et al., 1997). There have also been reports of enhanced photosynthesis in aquatic algae due to ocean wave-action that generated extraordinarily large amplifications of sunlight and greater sunlight penetration into the water column (Evans et al., 2008).
2.3 Spectral quality
Clouds and aerosols change the spectral quality of sunlight, which can also impact plant physiological processes. This topic was reviewed thoroughly by previous authors (Kanniah et al., 2012; Durand et al., 2021), and so here we only summarize major trends. Briefly, when normalized against total irradiance, cloudy skies are found to be enriched in shorter (ultraviolet, blue, and green) and deficient in longer (red, far red) wavebands, relative to clear skies (Durand et al., 2021). These effects are most pronounced under low solar elevation angles, and appear to occur irrespectively of cloud type, having been reported under overcast, “cloudy,” and cloud-immersed conditions (Dye, 2004; Urban et al., 2007; Reinhardt et al., 2010). However, under very thick clouds, these enhancements decline due to increased non-selective scattering (Dye, 2004; Urban et al., 2007). Enhanced blue to red (B:R) ratios under cloud also do not appear to extend into the understory, where B:R is relatively higher under clear sky conditions (Endler, 1993; Durand et al., 2021). Because stomata are especially responsive to blue light (detected by blue/UV-A-detecting cryptochromes and phototropins), cloudy conditions could increase stomatal opening in low leaf area index (LAI) habitats or above the canopy, leading to further enhancements in photosynthesis, while the opposite response would be expected in the understory (Shimazaki et al., 2007; Lawson, 2009; Dengel and Grace, 2010).
The absorption of far-red radiation at 728 nm by water vapor also leads to higher R:FR in both the top of the canopy and understory habitats under cloudy skies compared to clear (e.g., Endler, 1993; Capers and Chazdon, 2004; Reinhardt et al., 2010). The red (R) to far-red (FR) ratio is important for regulating physiological processes mediated by phytochrome, e.g., seedling germination and development, circadian rhythms, flowering, and shade avoidance (reviewed in Han et al., 2007). Through mechanisms that have not yet been elucidated, both phytochromes and cryptochromes appear to be involved, alongside temperature cues, in photoperiodic and phenological processes including bud burst and bud set (citations in Brelsford et al., 2019).
2.4 Longwave radiation
During both day and night, the water droplets that compose clouds strongly absorb longwave (infrared) radiation emitted by the Earth’s surface and lower troposphere, re-emitting these wavelengths either into space (i.e., top-of-atmosphere longwave radiative fluxes) or downward into the atmosphere (greenhouse effect) (Alados-Arboledas et al., 1995; Wielicki et al., 1995). Clouds are effective insulators because liquid water droplets absorb thermal radiation 1,000 times more strongly than water vapor (Stephens, 2005). Even ice clouds, which tend to be relatively transparent to incoming shortwave radiation (low albedo, low optical depth), absorb and re-emit outgoing longwave radiation, resulting in a net-positive (warming) effect on the atmosphere (Gasparini and Lohmann, 2016). Accordingly, cirrus cloud thinning is one proposed strategy for mitigating future warming due to climate change (Lohmann and Gasparini, 2017).
While top-of-the atmosphere heat exchange is restricted to high clouds, low-altitude clouds (e.g., stratocumulus, cumulus, and altostratus) exert the greatest warming effects on plants at the surface (Chen et al., 2000; Mülmenstädt et al., 2018; L’Ecuyer et al., 2019). Fully overcast skies at night have been estimated to increase longwave radiation at Earth’s surface up to 100 W m−2 (Feygel'son, 1988; Harrison et al., 1993). The warming of the lower atmosphere by low clouds further increases evaporation and water vapor content in the lower atmosphere, strengthening the greenhouse effect and inducing even more surface warming (i.e., positive water vapor feedback; Held and Soden, 2000; Garratt, 2001). Humidity of the lower atmosphere can also influence the capacity of clouds to warm the surface. Because water vapor in the lower atmosphere absorbs and scatters downwelling radiation before it reaches the surface, high water vapor content can mitigate transfer of thermal energy from clouds to plants. It has been estimated that the atmosphere becomes essentially opaque to downwelling radiation at water vapor contents above ca. 30 kg m−2 (Stephens et al., 2012).
2.5 Temporal dynamics
The rapidity of temporal changes between cloud shade and cloud gaps can impact plant ecophysiology as well. As previously discussed, differences in PPFD between cloud gaps and cloud shade can be dramatic, especially when PPFDs during cloud gaps exceed clear-sky values (Sections 2.2.1, 2.2.2). In the forest, temporal fluctuations in incident PPFDs on clear days are associated with shadows cast by individual plant leaves within a crown or canopy. These alternating values of high and low PPFDs can occur over seconds (e.g., “sunflecks”; Chazdon and Pearcy, 1986), to minutes and hours (“sunpatches”; Smith and Berry, 2013). Some authors have noted the parallels in temporal changes in light environment under cloud cover with those imposed by the canopy in the forest, as broken cloud cover can cause variable temporal exposure to cloud shade lasting seconds (small or fast-moving clouds) to tens of minutes to hours (for larger or slower-moving clouds) (Smith and Berry, 2013). However, not all clouds are associated with dramatic temporal fluctuations in irradiance. Indeed, Duchon and O’Malley (1999) used low variance in PPFD over a 21 min window as a criterion for differentiating stratus and cirrus clouds (low standard deviation) from cumulus clouds (high).
Eastman and Warren (2014) reported that cloud types also tend to have distinct temporal dynamics within the span of a day. Notably, cumulus clouds worldwide (at least over land) tend to form in the afternoon, as the ground warms and convection results in formation of cumulus clouds. Stratiform clouds tend to be most abundant in the morning, when Earth’s boundary layer is coolest, resulting in condensation and formation of fog and other stratiform clouds. The presence of any aerosols in the atmosphere promotes nucleation of cloud droplets and formation of low clouds in the morning (Rosenfeld et al., 2008); however, in the afternoon and evening, warming of the atmosphere by darkly-colored aerosols may inhibit low cloud formation (Ten Hoeve et al., 2012).
It is also important to recognize that the temporal variability in incident sunlight intensity and directionality due to clouds and aerosols is superimposed over similar changes in incident sunlight that occurs within individual plants and among neighboring plants on clear days (Smith and Berry, 2013). These cloud dynamics and corresponding variability in sunlight intensity and temporal variability, as well as the amount of diffuse versus direct sunlight incidence, add multiple levels of complexity for understanding and predicting the ecophysiological impacts of clouds now and in the future. Thus, mathematical models attempting to simulate accurately the magnitude and temporal variation of cloud effects on sunlit leaf areas and corresponding plant productivity have been a continuing challenge.
3 Ecophysiological effects of clouds
3.1 Differentiating cloud from precipitation effects
One challenge in interpreting the effects of local cloud patterns on plant ecophysiology is the precipitation that often accompanies cloud cover, and the corresponding increases in soil moisture and accompanying nutrient uptake. Clearly, this greater uptake of soil water and nutrients could mask any direct effects of clouds on a species ecophysiology, e.g., photosynthetic carbon gain and transpirational water loss. To differentiate between these effects, we restrict our discussion here to impacts directly or indirectly involving clouds, excluding precipitation.
3.2 Daytime
3.2.1 Leaf temperature
Temperature is a fundamental driver of all physiological processes in plants (Figure 1). During the daytime, the cooling effects of cloud shade directly and indirectly impact photosynthesis (Section 3.2.2), respiration and photorespiration (Section 3.2.3), and plant water status (Section 3.2.4). Daytime cooling effects further extend into the understory, where clouds can ameliorate the potentially extreme temperatures associated with direct sunlight patches combined with typically low wind speeds (low convective heat dissipation) (Watling et al., 1997). Conversely, in colder ecosystems or seasons, reduced leaf temperatures could also reduce photosynthesis and increase vulnerability to photo-oxidative stress (Section 3.2.5). In the sections below, we expand on and integrate these temperature effects into plant physiological processes, while differentiating between effects by major cloud type (if known).
3.2.2 Photosynthesis
Much data exists describing effects of clouds on photosynthesis at the leaf, shoot, and ecosystem levels, although gaps in the literature remain (especially for cirrus clouds and contrails). Kanniah et al. (2012) provides a useful table summarizing results of previous studies examining cloud and aerosol effects on canopy-level ecosystem productivity. The extent to which clouds impact photosynthesis on any structural scale depends on many factors, including air and soil temperature, plant water status, cloud optical thickness, canopy structure, and plant physiological constraints (e.g., light-saturation point), which are discussed below.
Perhaps the most obvious (and well-studied) effect of clouds on photosynthesis is their attenuation of downwelling irradiance. Clouds with low optical thickness (e.g., cirrus, thin cumulus or altostratus) may transmit sufficient PPFDs to saturate instantaneous photosynthesis at the leaf or shoot level (i.e., >500 μmol m−2 s−1 for most C3 plants) (Fan et al., 1995; Larcher, 2003;Johnson and Smith, 2008; Sanchez et al., 2016). Conversely, significant reductions in instantaneous photosynthesis of sun-exposed leaves have been reported under heavy cloud shade associated with cumulus clouds (Knapp and Smith, 1988; Hughes et al., 2015), overcast skies (Sanchez et al., 2014a, 2016; Hernandez-Moreno et al., 2017; Bayeur et al., 2018), and cloud immersion (Letts and Mulligan, 2005; Reinhardt and Smith, 2008a,b; Letts et al., 2010).
Enhanced diffuse light associated with clouds (Section 2.2) can at least partially offset reductions in total PPFDs by increasing sunlight distribution within crowns, stands, and canopies through diffuse light fertilization effects (Zhang et al., 2020). Light-saturation values of photosynthesis in shade-adapted species and shade-acclimated leaves range between 100–500 μmol m−2 s−1 (Larcher, 2003), and so even slight increases in irradiance in shaded microsites can lead to significant gains in carbon fixation. A more evenly-distributed radiation load in forest canopies is a major driver of enhanced light use efficiency, LUE (mol C mol− 1 photons) under cloudy conditions, although reduced respiration rates associated with cooler leaf temperatures and enhanced stomatal conductance resulting from a reduced vapor pressure deficit (particularly in the afternoon) are also recognized as important factors (Alton et al., 2007; Zhang et al., 2020). Higher LUE under cloudy vs. clear sky conditions has been documented for numerous plant functional groups and ecosystems (citations in Kanniah et al., 2012; Durand et al., 2021), with the most dramatic enhancements occurring in ecosystems characterized by high leaf area index (LAI), corresponding with high ratios of shaded to sun-lit foliage (Greenwald et al., 2006; Alton, 2008). However, these effects may reverse under CO2 doubling, through processes not yet fully understood (Urban et al., 2014).
It is important to note that increased LUE associated with diffuse light fertilization by clouds/aerosols is not always sufficient to offset reductions in productivity associated with lower total PPFDs (Alton et al., 2007). Alton (2008) reported consistent, significant declines in net ecosystem productivity and carbon sequestration in a wide variety of ecosystems under heavy cloud cover characterized by <40% clear sky PPFDs, despite elevated diffuse light fractions. However, under light cloud cover (<30% attenuation), the same study demonstrated slightly (ca. 10%) higher productivity in forested ecosystems relative to clear skies. These results suggest a “Goldilocks effect” for cloud cover, whereby maximum ecosystem productivity occurs under an intermediate amount of cloud. Similarly, diffuse PPFDs were reported to be brightest during broken, thin clouds with hourly sunshine amounts ranging 0.3–0.5 sunshine hours per hour (Page, 2012).
Cloud effects on photosynthesis also depend on the light saturation point (LSP) of plant species within the ecosystem, which can vary with plant functional type, water and nutrient status, leaf area, leaf inclination, crown structure, etc. For example, several studies have shown that broad-leafed angiosperms show significant reductions in photosynthesis under overcast or cloud-immersed conditions compared to co-occurring conifers (Johnson and Smith, 2008; Sanchez et al., 2016). C4 grasses and crops, too, tend to exhibit greater reductions in photosynthetic carbon gain under cloud cover compared to C3 species at similar irradiances (Figure 4; Still et al., 1999; Niyogi et al., 2004; Alton, 2008; Ye et al., 2020).
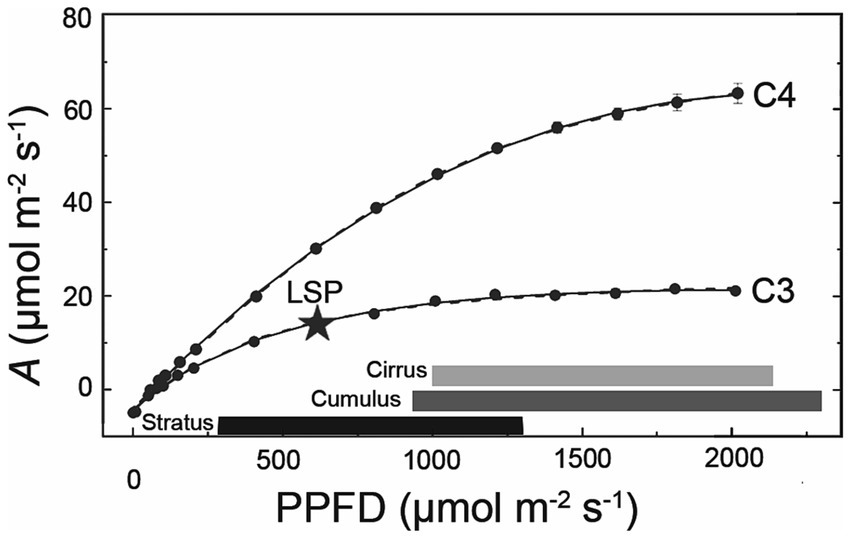
Figure 4. Plants vary in their photosynthetic responses to cloud cover. Shown here are photosynthetic light response curves for a C3 (soybean; Glycine max) and C4 species (grain amaranth; Amaranthus hypochondriacus), adapted from Ye et al. (2020). The star indicates the light saturation point (LSP, estimated as 90% maximum A) of the C3 species. Horizontal bars represent rough range of PPFDs transmitted under three major cloud types (calculated using % clear sky transmittance values from Figure 2, assuming clear sky PPFD of 2000 μmol m−2 s−1).
Clouds also influence photosynthesis indirectly through effects on leaf temperature (Figure 1). During intervals of cloud shade, leaves cool proportionally to the duration and optical density of the cloud, reducing rates of temperature-dependent biochemical processes including respiration, photorespiration, and photosynthesis, as well as evapotranspiration (Farquhar et al., 1980; Urban et al., 2007; Hughes et al., 2015). In cases where air temperatures exceed photosynthetic optima, however, cloud shade may increase photosynthesis (Baldocchi and Harley, 1995; Steiner and Chameides, 2005). Cooler leaf temperatures under cloud shade also reduce the leaf-to-air vapor pressure deficit (VPD), thereby decreasing rates of evaporative water loss (Hughes et al., 2015; Section 3.2.4). Higher plant water potentials promote sustained stomatal conductance (gs) and CO2 uptake, enhancing carbon gain while mitigating costs associated with photorespiration and photoinhibition (Urban et al., 2014). This is especially important in the afternoon, when low mid-day water potentials may lead to stomatal closure and mid-day depression of photosynthesis. Indeed, while diffuse light fertilization effects drive enhanced gs under cloud cover in the morning (when plant water stress is minimal), a lower leaf-to-air VPD is the primary driver of enhanced gs under cloud cover during afternoon (Freedman et al., 2001; Urban et al., 2014; Zhang et al., 2020).
Finally, there is some evidence that plants adapted to different light environments (including cloud regimes) exhibit leaf anatomical characteristics which maximize light absorption in that environment. For example, high-light adapted C3 plants often exhibit thicker leaves and multiple layers of vertically-oriented palisade cells, which efficiently facilitate distribution of direct beam sunlight through the leaf lamina (Vogelmann and Martin, 1993). Under diffuse light, however, light use efficiency in such species declines due to increased reflection off the leaf surface and a decrease in light penetration (Brodersen and Vogelmann, 2010; Earles et al., 2017). Some canopy tree species adapted to montane cloud forests, in contrast, exhibit up to 100% greater photosynthesis under diffuse light (Berry and Goldsmith, 2020), although the mechanisms responsible for photosynthetic enhancement were not described. However, variation exists within communities, even between plants in the same successional stage. For example, although three of the eight early-successional tropical canopy species examined by Berry and Goldsmith (2020) showed significantly higher net photosynthesis under diffuse light conditions, two species showed higher net photosynthesis under direct light conditions, and three species showed no significant differences between treatments. In general, much more research is needed to understand how plants have adapted to their local cloud regimes at these lower structural levels.
3.2.3 Respiration and photorespiration
Declines in photosynthetic carbon gain under cloudy conditions with low PPFDs may be offset to some degree by reduced respiration rates associated with cooler temperatures (Gu et al., 1999; Urban et al., 2007; Knohl and Baldocchi, 2008). Alton (2008) demonstrated that cooling-induced reductions in ecosystem respiration under cloudy skies offset declines in gross primary productivity (GPP) by approximately one third during the growing season in 38 Fluxnet sites representing six plant functional types.
Lower leaf temperatures afforded by cloud shade may also reduce photorespiration on hot days by slowing enzymatic reactions, increasing solubility of CO2, and reducing leaf to air vapor pressure deficits; the latter enhances leaf water balance, promoting stomatal opening and CO2 uptake (Figure 1; Ku and Edwards, 1977; Urban et al., 2014). The cooling effects of cloud shade are especially important for C3 plants under drought conditions and during the afternoon hours, when low water potentials promote stomatal closure (Freedman et al., 2001; Urban et al., 2012, 2014; Sanchez et al., 2016).
3.2.4 Water balance
Clouds are known to have beneficial effects on plant water status through several direct and indirect mechanisms. As discussed in Section 3.2.2, lower leaf temperatures and corresponding reductions in leaf-to-air VPD reduce transpiration under daytime clouds and allow plants to maintain higher plant water potentials, which are reflected in enhanced gs and CO2 uptake. Reports of cloud effects on plant water potentials are sparse (most notably lacking are measurements for grasses and deciduous trees, and many tropical ecosystems). However, there is some evidence that partly cloudy (cumulus), overcast (stratiform), and cloud immersion all buffer declines in mid-day water potentials (e.g., Reinhardt and Smith, 2008a,b; Urban et al., 2012; Berry and Smith, 2014; Hughes et al., 2015; Sanchez et al., 2016), which may help extend carbon gain over the growing season (assuming irradiance is not limiting).
Water use efficiency (WUE, calculated as A/E) is an important parameter for native plant adaptation, as well as for calculating agricultural yield and associated irrigation costs. If absorbed PAR is sufficient to saturate photosynthesis (A), reduced evapotranspiration (E) owing to cooler leaf temperatures can result in higher WUE compared to clear days (e.g., Young and Smith, 1983; Fan et al., 1995; Lamaud et al., 1996; Rochette et al., 1996; Freedman et al., 2001). Similarly, reduced E under intermittent cloud shade on partly cloudy days has been shown to carry over into brighter, between-cloud intervals, resulting in increased WUE during cloud gaps compared to clear days (Freedman et al., 2001; Hughes et al., 2015; Kivalov and Fitzjarrald, 2019). However, reductions in A under heavier cloud shade (i.e., below light-saturating levels) can reduce WUE despite lower E (Johnson and Smith, 2008; Reinhardt and Smith, 2008a,b; Sanchez et al., 2014b; Hughes et al., 2015).
The increased WUE afforded by intermediate levels of cloud shade could be considered another example of the “Goldilocks effect” in cloud-plant interactions, whereby too little cloud shade reduces WUE by increasing E, and too much cloud shade reduces WUE by reducing A. This latter point is complicated, however, by the fact that plant species vary dramatically in light requirements needed to offset respiration demands and saturate photosynthesis (Larcher, 2003). Hence, species with high light saturation points (LSP) may show declines in WUE while co-occurring species with lower LSP exhibit increased WUE under the same level of cloud cover (e.g., Johnson and Smith, 2008; Sanchez et al., 2016). Similarly, while some species maintain gs during short term variations in PPFDs, others track light levels closely, especially when drought stressed, opening stomata during high light and closing them during shade periods (Knapp and Smith, 1988; Knapp and Smith, 1990). In the latter case, increases in A upon return of high light are restricted by the lag in stomatal opening.
Perhaps the most direct effect of clouds on plant water status is uptake of fog water, either through leaves (primarily as vapor through stomata, but also directly through the cuticle) or through the soil in the form of fog drip (Weathers et al., 2020; Guzmán-Delgado et al., 2021). Fog, or low-cloud immersion, is common in valleys, coastal forests and deserts along nearshore-coastal upwelling zones with strong subsidence, as well as tropical and temperate montane cloud forests (e.g., Figure 5; Weathers et al., 2020). Immersion events can be daily in mountain cloud-forest communities (e.g., elfin forest), or may be periodic according to the day or season (e.g., coastal forests) (Scholl et al., 2011). Foliar uptake during fog or low cloud immersion has been documented in a broad variety of species, including bryophytes, ferns, grasses, shrubs, temperate deciduous and coniferous trees, and epiphytes (Limm et al., 2009; Ebner et al., 2011; Eller et al., 2013; Goldsmith et al., 2013; Berry et al., 2014a,b; Schreel et al., 2019). In addition to being an important source of water, fog also increases nutrient and pollution deposition (Weathers et al., 2020 and citations therein). However, in combination with precipitation, fog may also promote waterlogging of soils, leading to water stress reflected in stomatal closure but not shoot water potential (Green and Jane, 1983). Similarly, saturating VPDs may reduce the ability of plants to take up soil nutrients, due to lack of a vapor pressure gradient between the leaf and the air sufficient to drive transpirational pull (Gisleröd et al., 1987; Del Amor and Marcelis, 2004).
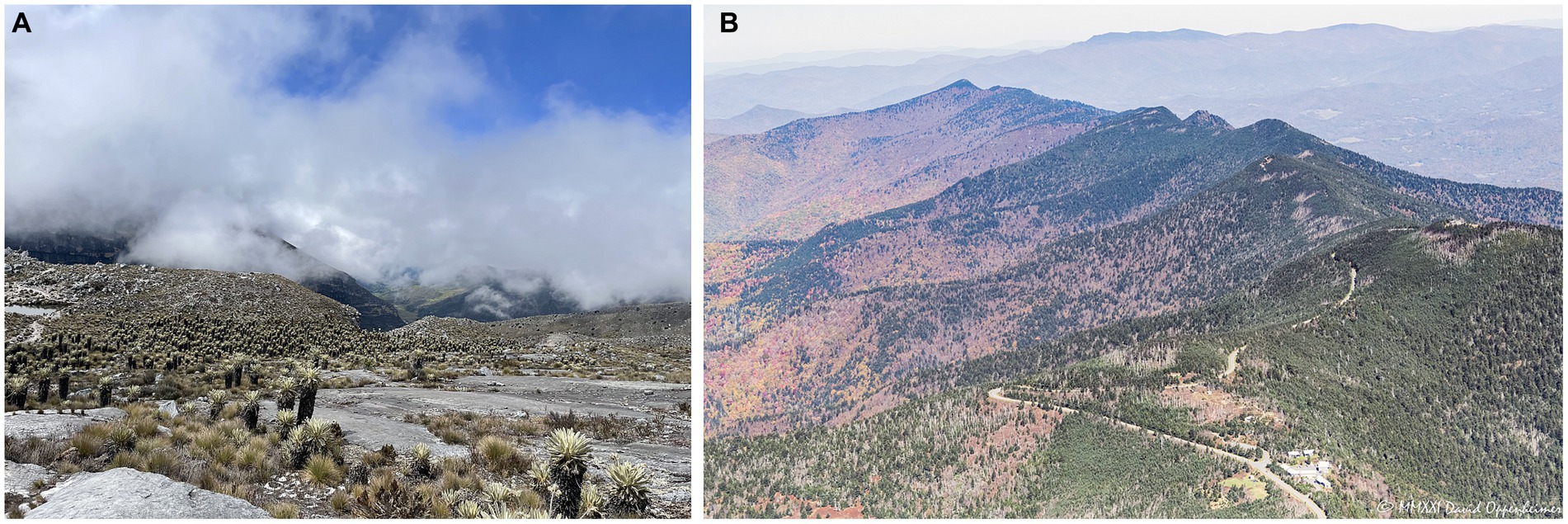
Figure 5. Montane ecosystems adapted to frequent cloud immersion face greater vulnerability to drought and frost stress with lifting cloud bases. Examples include the páramo in the Andes mountains of South America (A), and refugial spruce-fir forests in the Appalachian mountains of North America (B). Photos by Adriana Sanchez (A) and David Oppenheimer (B).
3.2.5 Photoinhibition
Full sunlight levels are commonly recognized as inhibitory to photosynthesis (i.e., photoinhibition), especially at low temperatures (Krause, 1994). When energy absorption is in excess of that which can be processed through the Calvin cycle, a deficiency in available electron substrates results, leading to closure of reaction centers and increased energy transfer from chlorophyll to atmospheric oxygen, formation of singlet oxygen and associated reactive oxygen species, and ultimately photo-oxidative damage (reviewed in Goh et al., 2012). Energy dissipation mechanisms may be employed under such circumstances, e.g., photorespiration, the Mehler-peroxidase pathway, the xanthophyll cycle, etc. (Heber et al., 1996; Gururani et al., 2015). Because these processes are competitive with photochemistry, they are collectively termed ‘non-photochemical quenching’ (NPQ), and degree of engagement can be quantified using chlorophyll fluorescence parameters (Rosenqvist and van Kooten, 2003).
Rapid temporal changes in PPFD have been shown to induce photoinhibition of photosynthesis, although the magnitude of these reductions is highly variable, depending on genetic constraints imposed by, e.g., stomatal induction, Rubisco activitation, regeneration of RuBP, diffusional limitations, leaf and stomatal movement, and relaxation rates of NPQ, as well as the duration and intensity of both past and present sunlight exposure (Chazdon and Pearcy, 1986; Ooba et al., 2003; Alter et al., 2012; Kaiser et al., 2016; Morales and Kaiser, 2020; Zhang et al., 2021). Are plants adapted to dynamic sky conditions better equipped to tolerate and utilize dramatic changes in PPFDs compared to plants adapted to more uniformly sunny environments (as seen in late successional vs. early-successional tree species, e.g., Zhang et al., 2012)? No studies to our knowledge have tested this hypothesis. However, under alternating cloud shade and cloud gap intervals associated with partly cloudy afternoons typical for the Rocky mountains, spruce and fir saplings exhibited only slight changes in PSII quantum yield (ΦPSII) during cloud gaps (< 0.1 units), suggesting minimal photoinhibition of photosynthesis (Hughes et al., 2015). Similarly, Sanchez and Smith (2015) showed only mild declines (<20%) in maximum PSII quantum yield efficency in the light-adapted state (Fv’/Fm′) under alternating periods of artificially applied low (250 μmol m−2 s−1) and extremely high PPFD’s (3,500 μmol m−2 s−1) in the alpine herb Caltha leptosepala at the same field site. No studies to our knowledge have tested the photoinhibitory response of plants adapted to clear sky conditions (e.g., desert plants) to fluctuating light levels. We were also unable to find studies measuring photoinhibition under intermittent cirrus clouds (or contrails).
3.2.6 Leaf and stomatal tracking
It now appears that exposure to full sunlight at midday is avoided by a large majority of broadleaf, vascular plant species via passive leaf wilting or changes in leaf orientation (Loreto et al., 2004; Niinemets and Sack, 2006). Such leaf movements can temper sunlight incidence from 100% full sun to less than a few percent (Reifsnyder and Lull, 1965; Huang et al., 1985). If direct sunlight incidence is not avoided, daily photosynthetic carbon gain may be limited by photoinhibition, photorespiration, and/or stomal closure associated with rising leaf temperatures and increased transpirational water loss (Loreto et al., 2004). Leaf movements have been observed in response to cloud-modulated changes in irradiance, and rate changes in leaf orientation of up to an angular degree per second have been measured in response to rapidly moving clouds (Huang et al., 1985). As described in Section 3.2.4, stomata also appear to track clouds similarly to sunflecks. Do plants adapted to dynamic cloud regimes similarly exhibit leaf movements in response to alternating intervals of sun/shade? Overall, this topic remains poorly understood.
3.3 Nighttime
The impacts of nighttime compared to daytime clouds is an understudied area with potentially important ecophysiological impacts on plants, beyond the important temperature effects that dictate growth season length. Below we summarize effects of clouds on nighttime leaf temperatures, and corresponding effects on respiration, phenological signaling, frost, and dew formation. We note also that nighttime clouds can also potentially interfere with plant circadian clocks, which can be based on moonlight (Breitler et al., 2020).
3.3.1 Nighttime plant temperature
At night, low clouds can trap infrared radiation and increase surface temperatures, and mitigate frost damage associated with cold nights and clear mornings. However, the influence of specific cloud types on nighttime plant ecophysiology has not been evaluated comprehensively. At sites in the Rocky Mountains, United States, measured leaf temperatures on clear nights with typically still air resulted in near-freezing leaf temperatures several degrees below air temperature (Jordan and Smith, 1994, 1995a; Germino and Smith, 2000). Similarly, reduced ecosystem respiration has been reported under cooler nighttime temperatures in multiple forest systems (Alton et al., 2007; Alton, 2008).
Changes in plant structure, architecture, and microsite preferences that are adaptive for avoiding nighttime sky exposure have been observed, separate from daytime sun exposure, although these most often occur mutually (Jordan and Smith, 1995b). Confusion also emerges due to the similarity in plant responses to avoid full sunlight and those required to avoid the cold night sky. Both slope orientation and sheltering vegetation appeared to have dramatic effects on the intensity and frequency of frost episodes during summer (Christersson, 1985). The greatest survival of conifer seedlings occurs at microsites with low sky exposure, despite the greater competition for soil water generated by the presence of the surrounding vegetation (Germino and Smith, 2000, 2001). Indeed, seedling survival is increased most (>90%) by a facilitated reduction in sky exposure, and not by water competition with the adjacent plants (also see Callaway et al., 2002; Smith et al., 2003). Experimental watering did not alter the positive response of establishing treeline seedlings of Abies, Picea, and Pinus species compared to experimental elimination of plant cover, supporting the role of sky exposure (rather than moisture-limitations) in controlling ecotonal tree seedling establishment (Maher and Germino, 2006). However, we also note that seedlings of deciduous treeline species appear to thrive in more exposed microsites, which could be related to their avoidance of the cold winter season owing to leaf shed (Hughes et al., 2009).
Finally, nighttime minimums also serve as a primary phenological signal initiating the beginning and/or end of the annual growing season in temperate ecosystems. This topic is discussed further in Section 4.3.
3.3.2 Frost
Seasonal frost damage and the strong effects of nighttime longwave radiation exchange on minimum leaf temperatures were described by Christersson (1985), in a summary of the importance of summer frost damage to both agricultural species and native tree species in Sweden. Severe frost damage at night was observed in native poplar species of the Rocky Mountains of Canada and the United States, especially when warmer daytime temperatures were followed by freezing nights (Zalasky, 1976). Less frost damage occurred in a variety of species (e.g., crops, ferns, and Vaccinium spp.) if individual plants were sheltered from clear night skies (Bannister, 1973). The importance of clear-sky exposure on summer frost occurrence has also been documented as influencing growth and establishment of tamarack trees and black spruce (Dang et al., 1992). Radiation frosts have also been associated with inverted treelines in frost hollows of Southeastern Australia (Paton, 1988) and in Western Australia where re-vegetation of mined and agricultural areas using Eucalyptus tree seedlings occurs. Multiple leader production in response to radiation frost damage to apical buds is now recognized as a primary cause of substantial reduction in productivity in forests throughout the world (Gross, 1983; Raitio, 1987; Holopainen, 1990).
3.3.3 Dew
Leaf temperatures below air temperature may also result in condensation events and leaf surface wetting (i.e., dew formation), typically during the early morning hours when water status and photosynthesis are at their daily maximum (except in cold-limited times and seasons). Almost universally, plants respond to the possibility of severe restriction in gas exchange across a water film by repelling water into droplets (Smith and McClean, 1989; Letts and Mulligan, 2005). Nevertheless, a host of plant species are known to depend on dew formation on leaves for hydration (Berry and Smith, 2014). This process would be curtailed by warmer nighttime temperatures associated with increased cloud cover, preventing colder leaf temperatures needed to reach the dewpoint.
3.4 Combined effects of nighttime and daytime clouds
There is direct evidence that the combined stresses of near-freezing leaf temperatures, usually occurring just before sunrise, combined with high incident sunlight at daybreak, can result in a strong and lasting negative impact on photosynthetic performance in a variety of plant species (Lundmark and Hällgren, 1987; Örlander, 1993; Adir et al., 2003). Such excessive sun exposure can result in particularly strong reductions in daily photosynthesis and subsequent mortality, especially in first-year tree seedlings in the field (Krause, 1994; Germino and Smith, 1999; Alves et al., 2002; Hernandez Velasco and Mattsson, 2020). Just as neighboring plants are known to protect others from both high daytime insolation plus the cold nighttime sky (Leuning, 1988, 1989; Ball et al., 1997; Egerton et al., 2000; Germino et al., 2002; Maher et al., 2005), clouds may also buffer the transition between night and day.
Under cold conditions, plants often maintain some degree of sustained non-photochemical quenching through cold nights, which can help mitigate photo-oxidative damage the following day (reviewed in Verhoeven, 2014). Photosynthetic photoinhibition resulting from the combination of both low nighttime temperatures and high morning irradiance (natural or simulated) has been reported for some native tree species, including Eucalyptus (Holly et al., 1994; Davidson et al., 2004); Fagus and Fraxinus (Einhorn et al., 2004); Picea (Kitao et al., 2004); Mediterranean tree species (Martínez-Ferri et al., 2004); and conifer seedlings (Germino and Smith, 1999).
4 Changing cloud patterns
Clouds are not distributed evenly around the globe, either in frequency or type (Eastman and Warren, 2013; L’Ecuyer et al., 2019; Figure 6A). Latitudinal trends in cloud cover are governed primarily by largescale patterns of convection driven by uneven heating of the earth’s surface. Rising temperatures, disproportionately in the Arctic, impact the thermal gradients which drive these air circulation patterns, affecting cloud height, frequency, morphology, and distribution worldwide (summarized by climatic zone in the following sections). Eastman and Warren (2013) provide a useful surface-based cloud climatological atlas describing diurnal, seasonal, and multi-decadal trends for each major cloud type over a 39 year period.1
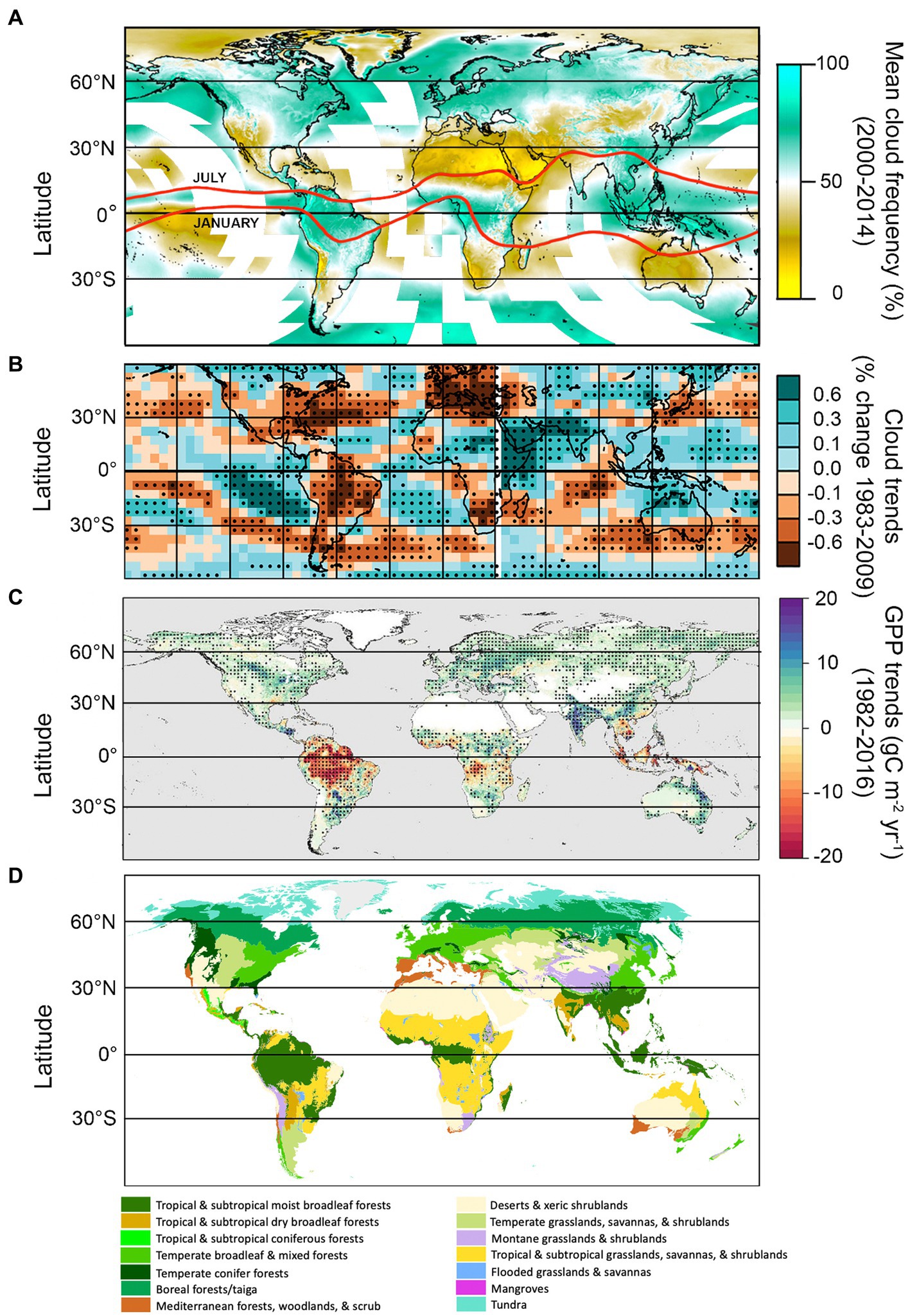
Figure 6. Global cloud frequency, cloud trends, gross primary productivity (GPP) trends, and the major terrestrial biomes. Global mean cloud frequency data (A) adapted from Figure 1 of Wilson and Jetz (2016), and based on twice-daily satellite-derived cloud observations at 1-km resolution, taken between 2000 and 2014. Monthly data are available at http://www.earthenv.org/cloud. Red lines represent the approximate position of the intertropical convergence zone (ITCZ) in July and January (adapted from Yan, 2005). (B) Represents % change in cloud amount between 1983 and 2009 (relative to the 60° S–60° N mean), based on Coupled Model Intercomparison Project (CMIP5) historical simulations with all radiative forcings included (adapted from Figure 1C in Norris et al., 2016). Black dots represent statistically significant trends (p < 0.05). Gross primary productivity trends (1982–2016), (C) from an optimum remote sensing light use efficiency model, adapted from Madani et al. (2020); black dots represent statistically significant trends (p < 0.05). Biomes map (D) adapted from Olson et al. (2001).
In addition to global patterns of convection, regional factors also impact cloud formation over land. These include mountains (orographic lifting), transpiration from vegetation, land cover change, and the presence of condensation nuclei (e.g., aerosols, pollution, salt spray) (Boucher et al., 2013; Ray, 2013; Yan et al., 2020; Spiridonov and Ćurić, 2021). Clouds nucleated on aerosols may be brighter, as they tend to be composed of higher concentrations of smaller water droplets (Rosenfeld et al., 2008). Such clouds are longer-lived and less likely to form precipitation (Ten Hoeve et al., 2012 and citations therein). “Brown clouds” comprised of carbonaceous aerosols have also been associated with large-scale deforestation in many locations around the globe (Ramanathan et al., 2007). Darkly-colored aerosols, including those associated with forest fires, can promote cloud formation at low concentrations by acting as condensation nuclei; however, cooling effects are reversed at higher concentrations, as atmospheric warming favors evaporation of low clouds and formation of high clouds from resulting updrafts (Ten Hoeve et al., 2012; Liu et al., 2020). Notably, reductions in atmospheric pollutants associated with clean-air initiatives have been implicated in localized reductions in low cloud cover and optical thickness at several locations around the globe (Yan et al., 2020; Watson-Parris et al., 2022).
Deforestation also impacts cloud formation regionally by interrupting the recycling of soil water back into the atmosphere via evapotranspiration, and reducing atmospheric water vapor available for cloud formation and precipitation (Staal et al., 2020; Xu et al., 2022). There is some evidence, however, that small-scale deforestation may increase clouds and precipitation locally, owing to convective circulation induced by land surface heterogeneity, although this effect diminishes with increasing size of the deforested area (Souza et al., 2000; Khanna et al., 2017). Warmer surface temperatures in deforested areas also increase the altitude at which overlying clouds form (Lawton et al., 2001; Ray, 2013), similarly to urban heat islands (Williams et al., 2015; Yan et al., 2020).
Finally, most models agree that cloud top and cloud base heights are increasing on average globally, although the magnitude of the latter varies widely regionally and across models (Prein and Heymsfield, 2020; Zelinka et al., 2020). These changes have been attributed to a variety of global and regional factors, including warmer air temperatures (which increase the height at which condensation occurs), pollution, urbanization, and interruption of the hydrological cycle by deforestation (Lawton et al., 2001; Ray, 2013; Williams et al., 2015; Yan et al., 2020). However, cloud base heights are not increasing everywhere. In fact, in regions where atmospheric moisture, clouds, and precipitation are increasing, clouds bases have been lowering (e.g., India; Figure 6B). The most dramatic lifting of cloud bases occurs in regions with atmospheric drying (e.g., tropical America and Africa; Los et al., 2021). As described previously, regional patterns of air pollution and deforestation also play a role.
In the sections below, observed and predicted trends in cloud cover over land for the major climate zones (tropics, subtropics, temperate, and polar) are summarized, incorporating both global and regional factors. Potential ecophysiological impacts of changing cloud patterns on dominant plant ecosystems are discussed in broad terms (i.e., in terms of anticipated impacts on major functional groups, e.g., broadleaf deciduous trees, C3 and C4 crops, montane cloud forest species, etc.), and interpreted by overlaying patterns of GPP (Figure 6C) and its environmental drivers (Figure 7).
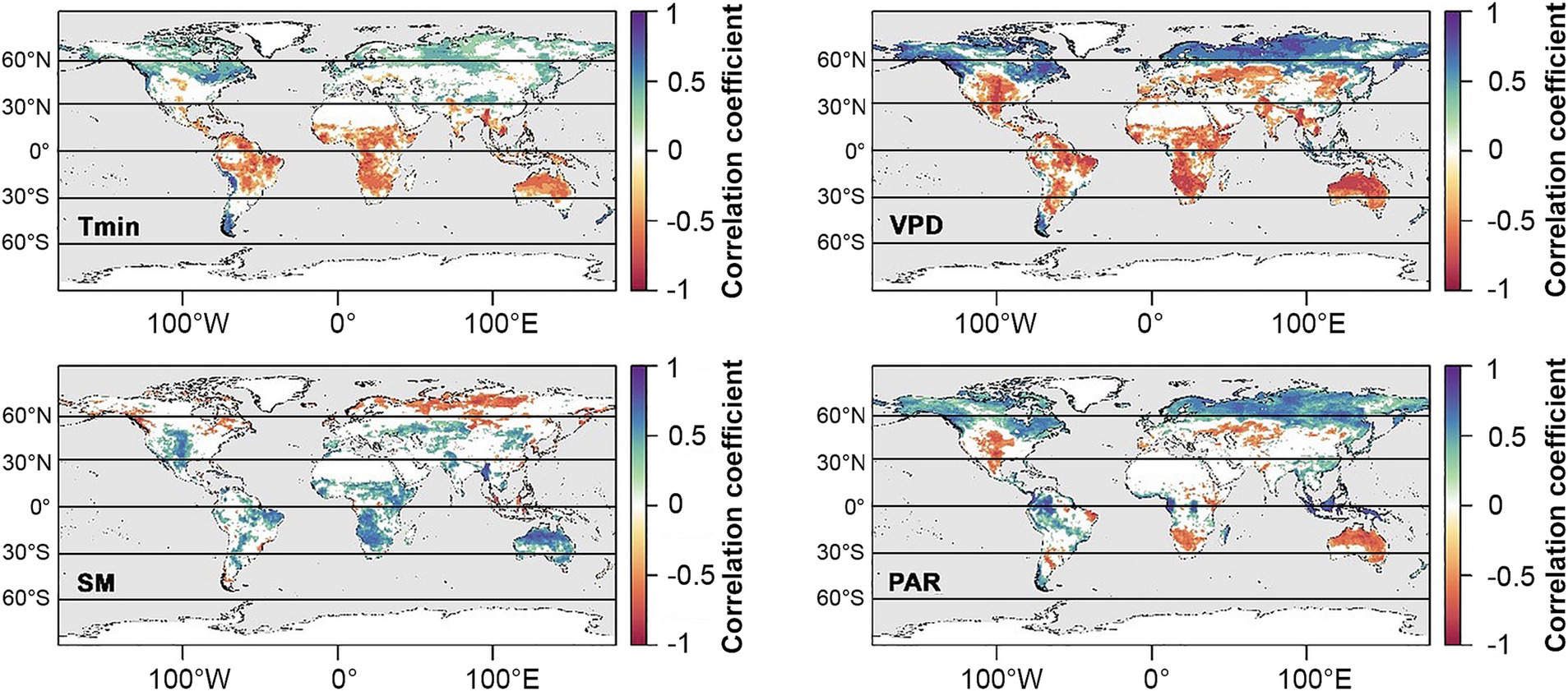
Figure 7. Dominant climatic factors affecting GPP between 1982 and 2016 (from Madani et al., 2020). Colors indicate Pearson correlations of minimum daily temperature (Tmin), vapor pressure deficit (VPD), soil moisture (SM), and photosynthetically active radiation (PAR). Nonsignificant correlations are not included.
4.1 Tropics
High solar radiation coupled with an abundance of warm ocean water favors formation of deep convective clouds (cumulonimbus, congestus, and associated cirrus clouds) in the tropics, with a diurnal maximum occurring around mid-day (Eastman and Warren, 2014). Nearly all cloud types are more common in the tropics than other latitudes, although those associated with deep convection dominate (Sassen and Wang, 2008, 2012; Li et al., 2015; L’Ecuyer et al., 2019). The resulting band of moist, heated air near the equator forms the ascending arm of the Hadley cells, and is a major driver of global air circulation patterns. At its center lies the intertropical convergence zone (ITCZ), a roughly 80–300 km wide band where the trade winds from the Northern and Southern Hemispheres converge (Yan, 2005). The ITCZ accounts for an estimated 32% of global precipitation (Kang et al., 2018). Coupled Model Intercomparison Project (CMIP5) models show robust support for a CO2-induced deepening and narrowing of the ITCZ, in a process referred to as the “deep-tropics squeeze” (Lau and Kim, 2015; Byrne and Schneider, 2016; Su et al., 2017, 2020; Zhou et al., 2019). This process results in deeper, stronger convection at the center of the ITCZ, but fewer high clouds (and less precipitation) at the margins of the convection zone (Figure 8; Zhou et al., 2011; Chou et al., 2013; Lau and Kim, 2015; Norris et al., 2016; Su et al., 2017). Accordingly, general circulation models are in robust agreement that the length of the rainy season will shorten in the tropics under 1.5o–2°C warming, with approximately 10% of the tropics expected to experience rainy seasons which are 6–7 days shorter (Saeed et al., 2018). In terms of direct cloud effects (apart from precipitation), fewer clouds at the margins of the convective zone will correspond with greater downwelling solar (as in, greater downwelling solar radiation) radiation during the day, which could increase primary productivity in PAR-limited regions of the tropics where moisture is abundant (e.g., western Congo). However, in PAR-limited regions where clouds are increasing (e.g., much of southeast Asia), GPP will likely continue to decline (Figures 6, 8; Norris et al., 2016; Madani et al., 2020). In contrast, primary productivity the Amazon is primarily moisture-limited, and reductions in cloud cover over recent decades have corresponded with declines in GPP (Figures 6, 8; Norris et al., 2016; Madani et al., 2020).
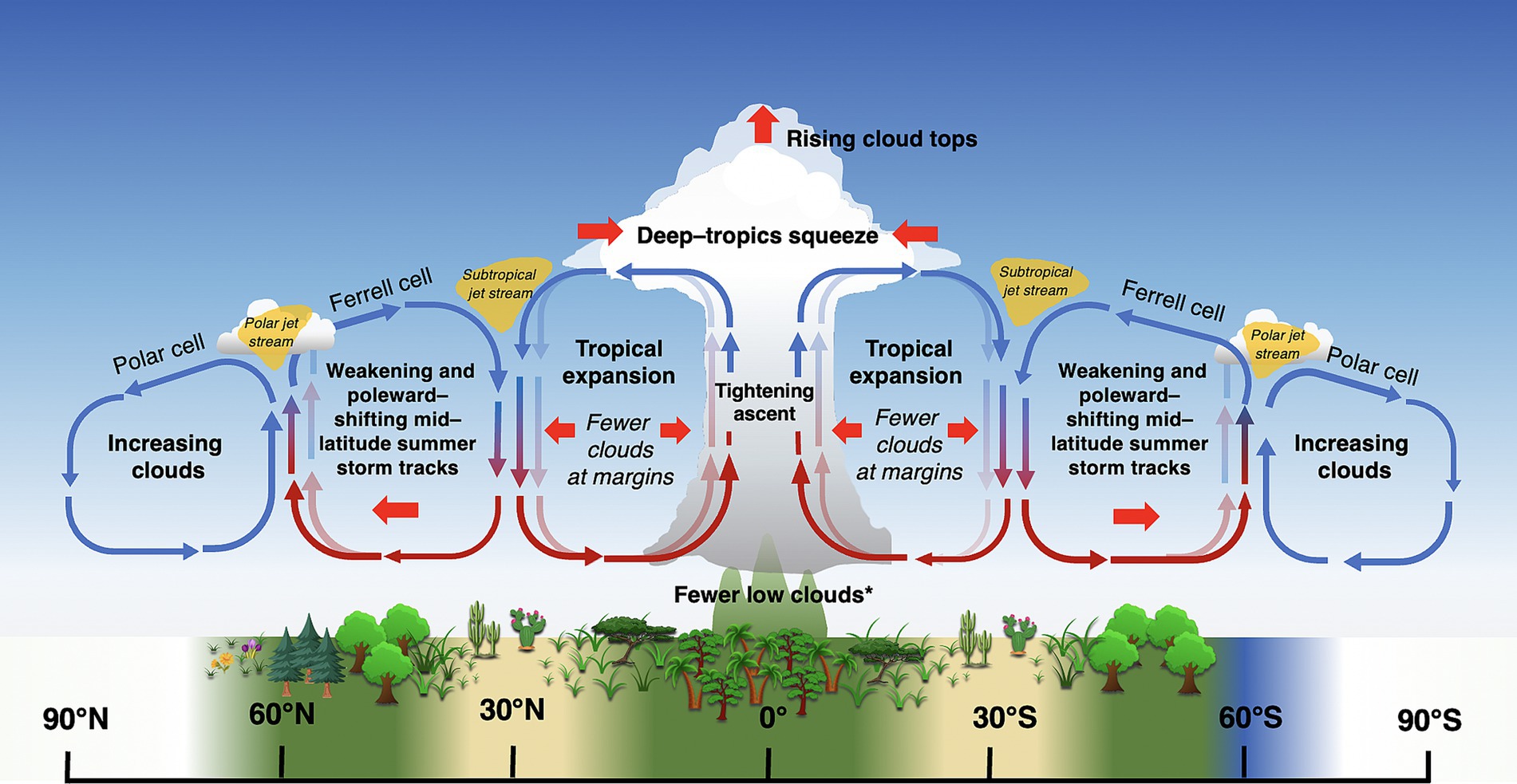
Figure 8. Summary of predicted changes in large-scale air circulation patterns under global warming. Lighter arrows represent previous air circulation patterns, while solid arrows represent new patterns under climate warming. Positions of climate belts and corresponding biomes are approximate (note seasonal position of ITCZ in Figure 6A). Thin red and blue arrows represent warm and cool air, respectively. *Exceptions include regions with increasing moisture (Los et al., 2021). Image not drawn to scale.
The ITCZ migrates north and south seasonally towards the warmer hemisphere, forming a “tropical rain belt”—a region that is home to 40% of the world’s population, and some of the most biodiverse ecosystems on earth (Denniston et al., 2016). In addition to a narrower, deeper ITCZ, there is strong evidence that its annual north–south migration path is both contracting and shifting (Zhou et al., 2019; Mamalakis et al., 2021). Recent contraction of the tropical rain belt has been attributed to enhanced equatorial and sea surface temperature warming in the deep tropics (Zhou et al., 2019). The direction of its shift varies longitudinally, with a northward shift occurring over the Indian Ocean and Africa, and a southward shift over the oceans surrounding South America (Mamalakis et al., 2021). Significant increases in GPP in India in recent decades (Figures 6B,C) have been associated with precipitation-driven increases in photosynthesis by crops, which make up the majority of the land area in the country (Verma et al., 2022). Rainfall during the Indian summer monsoon is also predicted to increase and become more variable with warming temperatures by CMIP6 models (Katzenberger et al., 2021). No significant shift in the ITCZ position over the Amazon has been projected by CMIP6 models, although significant shifts to the south are forecasted in the surrounding oceans (Mamalakis et al., 2021). However, deforestation and aerosols associated with forest fires have been shown to negatively impact regional cloud formation and precipitation (Wang et al., 2009; Khanna et al., 2017; Liu et al., 2020; Staal et al., 2020; Xu et al., 2022). As described previously, forests are an important source of atmospheric moisture, and play an important role in the hydrological cycle. The tropics have experienced the greatest loss in forested area in the past century, primarily due to agricultural expansion and cattle ranching (Hansen et al., 2013), which has had severe, potentially irreversible, impacts on the hydrological cycle (Xu et al., 2022).
Rising cloud base height poses a special threat to tropical montane cloud forest ecosystems (e.g., Figure 5A). which depend on moisture inputs associated with low-level clouds to maintain plant water balance, especially during the winter dry season (Still et al., 1999). Low clouds also reduce vulnerability to frost in these systems (Helmer et al., 2019). The relationship between cloud base height and species composition are well documented (Still et al., 1999; Sklenář et al., 2008; Hulshof et al., 2020). In locations where clouds cover the tops of low mountains (i.e., where upward migration is impossible), montane forests will likely contract, due to drier conditions and competition from upward-shifting lowland species (Bruijnzeel et al., 2011; Taylor and Kumar, 2016). In regions with vegetation above the current cloud band, there may be opportunities for species to migrate. Under moderate CO2 emissions scenarios, neotropical tropical montane cloud forests are expected to contract and dry dramatically (57% decline in area) as early as 2040, with 70% of paramo being either dry or subject to tree invasion by 2040 as well (Helmer et al., 2019; Figure 5A). An increase in cloud-base height of 250 m, predicted under 2°C warming, has also been estimated to reduce tropical cloud forest extent in N Kenya by 50–100% (Los et al., 2019), and some models anticipate that tropical cloud forest climates will disappear entirely by 2,100 (Williams et al., 2007). However, Los et al. (2021) found evidence of lifting-cloud bases only in tropical montane cloud forests of the Americas and parts of Africa; cloud bases in tropical montane cloud forests in Asia, in contrast, declined during the same period, corresponding with regional increases in moisture.
4.2 Subtropics
As air masses high in the atmosphere move towards the poles, they cool and sink in the subtropical latitudes (~23.5 to 35° N/S). Climate in these regions is sunny and dry, due to subsidence (sinking, compressing, and heating of air masses), and it is at these latitudes that many of the earth’s deserts occur. Accordingly, most cloud types over land are relatively scarce around the 30° latitudes (Rossow and Schiffer, 1999; Sassen and Wang, 2008). Altostratus and altocumulus clouds have been reported as the dominant cloud type in Northern and Southern Hemisphere deserts, respectively (Li et al., 2015), although discerning cloud types over deserts can be difficult due to the presence of airborne dust, which may be misidentified as clouds (Choi et al., 2010). Coastal deserts situated next to colder oceans in these regions often experience morning fog and low clouds on a daily basis (Warren et al., 2007), and many native plants depend on this fog as a moisture source (discussed in Section 3.2.4).
An abundance of evidence, including observations and simulations, indicates that the descending edge of Hadley cells (and associated subtropical arid conditions) are moving poleward in a process known as “Hadley cell expansion” or “tropical expansion” (Figure 8; Lu et al., 2007; Bender et al., 2012; Grise and Polvani, 2016; Norris et al., 2016; Grise and Davis, 2020). A variety of factors, both natural and anthropogenic, contribute to tropical expansion, including increasing greenhouse gases and warmer sea surface temperatures (Tao et al., 2016; Rollings and Merlis, 2021), depletion of stratospheric ozone over Antarctica (Waugh et al., 2015), El-Niño Southern Oscillation (Amaya et al., 2018), the Pacific Decadal Oscillations, and aerosols (Allen et al., 2012, 2014). Recently, however, Yang et al. (2020) demonstrated that the width of the tropics closely tracks the movement of oceanic midlatitude meridional sea surface temperature gradient (MMTG), and concluded the poleward shift of the MMTG was the primary factor driving tropical expansion. Estimates of the rate of poleward tropical expansion range from 0.25°–3° latitude decade−1 (citations in Rollings and Merlis, 2021), with the Southern Hemisphere Hadley cell expanding more rapidly than the Northern (Tao et al., 2016).
Expansion of Hadley cells corresponds with a poleward shift of the subtropical subsidence zone, resulting in reduced clouds and more arid conditions at the poleward edges of the horse latitudes (Scheff and Frierson, 2012; Fu, 2015; Marvel et al., 2015; Liu et al., 2016; Norris et al., 2016) (Figure 8). Global arid and semiarid drylands across the globe have been expanding since the mid-twentieth century, and are expected to continue to expand under high greenhouse gas emissions scenarios (Feng and Fu, 2013). Reduced cloud cover would increase sunlight incidence and evaporation in plants and soils in these regions, possibly increasing photosynthesis in the short term, but increasing drought stress (and reducing carbon gain) in the long term (e.g., Hu et al., 2010). Plant communities at these latitudes could be expected to shift to favor more high-light, drought-adapted species.
Water stress associated with reductions in subtropical clouds may be offset somewhat by intensification of equatorial winds and tropical cyclones (hurricanes/typhoons), which are expected to intensify and move poleward under CO2-forced warming (Byun and Hamlet, 2018; Zhou et al., 2021). These winds and associated ocean currents transport heat and moisture poleward from the tropics, and are responsible for much of the cloud cover and precipitation on the eastern coasts of continents, as well as some western coasts (e.g., Western Europe) (Gimeno et al., 2012). Climate models are in agreement that CO2-forced warming drives an intensification and poleward shift in tropical cyclones, although frequency trends vary by ocean basin (citations in Sobel et al., 2016; Vecchi et al., 2021), with storm frequencies decreasing in southern Indian and South Pacific Oceans, and increasing in the North Atlantic (Bhatia et al., 2019; Kossin et al., 2020; Murakami et al., 2020). Forests too may offset reductions in clouds somewhat by promoting local cloud formation (e.g., Teuling et al., 2017), as may a heterogeneous landscape (Souza et al., 2000).
4.3 Midlatitudes
In the temperate midlatitudes (35° to 56°N and S), cyclonic storms fueled by westerly winds bring moist air from oceans onto land, accounting for over 70% of total precipitation in regions such as Europe and North America (Hawcroft et al., 2012). Middle clouds (altostratus and altocumulus) are widely distributed at these latitudes, while high clouds (cirrus, cumulonimbus) are more unevenly distributed, their appearance corresponding with factors that generate thunderstorms, e.g., orographic lifting, deep convection, and synoptic jet stream (Sassen, 2002; Sassen and Wang, 2008). Nimbostratus clouds are also more common in these latitudes than in the tropics (Sassen and Wang, 2008).
One robust prediction by climate models is a widespread reduction in clouds at midlatitudes, which can be at least partially attributed to a weakening equator-to-pole thermal gradient (Zelinka et al., 2012; Coumou et al., 2015; Norris et al., 2016). This translates into seasonally weaker westerly winds and storm tracks in the Northern Hemisphere, favoring the buildup of hot and dry conditions over continents during summer (O’Gorman, 2010; Barnes and Polvani, 2013; Coumou et al., 2015, 2018; Petrie et al., 2015; Chang et al., 2016). Declining cloud trends over land are driven primarily by reductions in middle to high clouds (Eastman and Warren, 2013). Increased surface irradiance owing to fewer clouds could potentially increase photosynthesis in PAR-limited species or systems where moisture is not limiting. However, with the exception of North American boreal forests, GPP in the temperate zone is more frequently moisture-limited than PAR-limited (Figure 7; Madani et al., 2020), and so ecosystem impacts of reduced clouds in the mid-latitudes are more likely to be negative. For example, weakening of mid-latitude summer storm tracks could further exacerbate drought and wildfires in water-limited regions such as western to central North America and the Mediterranean, impacting native systems as well as the agriculturally-important crop and pasture lands that sustain these regions (Ramankutty et al., 2008). Increased surface irradiance owing to fewer clouds could potentially increase photosynthesis in plants adapted to dry conditions and high PPFDs such as grasses and C4 crops (Figure 4). However, GPP tends to be limited by soil moisture and VPD in the temperate zone (Figure 7; Madani et al., 2020), and so ecosystem impacts are more likely to be negative.
There is also abundant evidence from decades of climate models that midlatitude storm tracks are shifting poleward, with the largest shifts occurring in the Southern Hemisphere (Figure 8; reviewed in Shaw, 2019). Poleward expansion of tropical cyclones, too, have already caused significant damage to boreal forests as far as 50°N (Korznikov et al., 2023). These latitudinal shifts have been linked to numerous complex thermodynamic mechanisms associated with human activity, including stratospheric ozone depletion over the Antarctic, and rising CO2 levels (e.g., poleward expansion of Hadley cells, increasing extratropical tropopause height, and changes in cloud radiative effects with the resulting upward shift of high clouds) (Lee and Feldstein, 2013; Mbengue and Schneider, 2018; Li et al., 2019; Shaw, 2019). A shift in clouds and precipitation to more polar latitudes may reduce primary productivity in these ecosystems, which are already limited by low PAR (Figure 7; Boucher et al., 2013; Madani et al., 2020). However, if cloud cover is light, increased radiation sharing by the canopy, combined with cooler leaf temperatures and reduced respiration, could increase Net Ecosystem Productivity (NEP) relative to clear skies (Alton, 2008).
Rising cloud bases in the mid-latitudes have the potential to impact temperate montane cloud forest ecosystems. For example, refugial spruce-fir forests of the Appalachian mountains (Figure 5B) occur only on the highest peaks (i.e., above ~1,500 m elevation), and derive up to 31% of plant water from fog during morning cloud immersion (Berry et al., 2014a). Because these populations have already reached the altitudinal limits imposed by topography, a lifting cloud base could potentially lead to extinction of this unique ecosystem and its many endemic species (Richardson et al., 2003).
Warmer temperatures over the last century have also had impacts on phenology of temperate deciduous species, including agriculturally important fruit and nut trees. Although the dynamics of phenological changes vary across space and time, leaf-out and flowering generally occur earlier in warmer years (Cleland et al., 2007; Polgar and Primack, 2011). Accordingly, woody species in temperate regions across the world are advancing their timing of leaf-out at an estimated two weeks earlier on average than in the 18th and 19th centuries (Vitasse et al., 2022). An early bud break can increase a plant’s vulnerability to late season frost, resulting in significant losses to agricultural yield if flowers are severely damaged (as in Kunz and Blanke, 2022). Many temperate species, including fruit trees, also have minimum-chilling requirements, and rising night-time temperatures associated with global warming could impact dormancy and productivity of such species, especially on the equatorial ends of their ranges (reviewed in Salama et al., 2021). Rising nighttime temperatures could also benefit invasive plant species with lower chilling requirements, giving them a head-start over native species with higher chilling requirements (Polgar et al., 2014). A reduction in clouds may offset these and other processes impacted by rising nighttime temperatures to some degree, as more longwave energy is allowed to escape into the atmosphere (Section 3.3).
Warmer temperatures owing to fewer daytime clouds can also impact the timing of autumn leaf senescence. However, these effects are more complex than those driving spring leaf-out, since drought and nutrient status also play a role (Estiarte and Peñuelas, 2015; Seyednasrollah et al., 2020; Wang et al., 2022). It has been estimated that fall/senescence in autumn is now delayed 1–2.4 days decade−1 corresponding with rising temperatures (citations in Estiarte and Peñuelas, 2015). However, a recent study suggests that early-late summer asymmetry in warming can yield opposing effects on the timing of senescence, with warmer pre-solstice temperatures accelerating senescence (by 1.9 ± 0.1 days per °C), and warmer post-solstice temperatures delaying it (2.6 ± 0.1 days per °C; Zohner et al., 2023). A longer growing season could potentially result in greater carbon uptake, but could also potentially promote drought stress (Hu et al., 2010). Drought stress, especially during autumn, has also been shown to advance leaf senescence (Chen et al., 2020; Seyednasrollah et al., 2020), as has N deficiency, which often accompanies drought (Rouphael et al., 2012; Wang et al., 2022).
Finally, some researchers have argued that a weaker equatorial-pole thermal gradient could weaken and destabilize the polar jet streams, increasing the likelihood of extreme cold snaps during winter in midlatitudes as the polar vortex meanders into temperate latitudes (e.g., Kim et al., 2014). However, this hypothesis was based largely on observational data following a run of historically cold winters for North America and Europe, and has not been supported by the most recent models (Blackport and Screen, 2020).
4.4 Polar latitudes
In general, cloud height decreases towards the poles, as dew point temperatures can be achieved at lower altitudes. There is also marked increase in optical thickness of clouds from 60° poleward (Rossow and Schiffer, 1999), as well as a scarcity of high clouds (cumulonimbus and cirrus; Sassen and Wang, 2008). Precipitation in polar latitudes is most commonly associated with nimbostratus clouds.
It is well-known that temperatures at the poles are increasing more rapidly than the lower latitudes (more than 3x faster than the global average since 1979 according to Rantanen et al., 2022). This process, termed “Arctic amplification,” has traditionally been attributed primarily to albedo feedback effects, which occur when melting sea ice exposes darker water beneath (Screen and Simmonds, 2010a; Serreze and Barry, 2011). However, increased poleward heat transport, intraseasonal cycling of heat, and changing clouds also contribute (citations in Boeke and Taylor, 2018; Yamanouchi and Takata, 2020). With rising temperatures, cold-temperature limitations to GPP in the polar latitudes have been loosening, resulting in longer growing seasons and enhanced productivity—a process termed “Arctic greenification” (Figures 6C, 8; Madani et al., 2020).
Arctic plant species differ widely in their response to changes in climate and nutrient availability (e.g., Chapin and Shaver, 1985; citations in Dormann and Woodin, 2002). A meta-analysis of field experiments examining the response of various Arctic plant functional groups to environmental manipulation found few consistent trends, although shrubs appeared positively impacted by warming (Dormann and Woodin, 2002), consistent with reports of “Arctic shrubification” (Mekonnen et al., 2021; although see Kremers et al., 2015). Tundra grasses also tend to thrive under both fertilization and elevated temperature treatments, while forbs show little to no response to warming or nutrient addition (Dormann and Woodin, 2002; Kremers et al., 2015). Warming and human activity have also facilitated species invasions, thus competition with non-native plants is another challenge for formerly-isolated, high-latitude native species (Rew et al., 2020). Boreal forests too are advancing poleward, facilitated by warmer temperatures, deeper snowpack, increased soil nutrient availability, and stronger winds that can facilitate long-distance seed dispersal (e.g., Dial et al., 2022). At their southern boundaries, however, rising temperatures threaten the growth of young conifers (Reich et al., 2022), while adult trees are vulnerable to fire, logging, and disease (Hansen et al., 2013).
In addition to rising temperatures, Arctic cloud amount is also increasing, due to greater poleward heat transport combined with mid-latitude moisture intrusions and greater evaporation from warming oceans (Sejas et al., 2014; Kim et al., 2016). However, the radiative effects of Arctic clouds differ in sign according to the season. During the summer, increased cloud albedo results in a net cooling effect on the Earth’s surface, while during late fall and winter, the trapping of longwave radiation emitted by the Earth and its warming oceans, combined with low incoming radiation associated with the winter season, result in a net warming effect (Boeke and Taylor, 2018; Yamanouchi and Takata, 2020). Accordingly, Arctic amplification is greatest during late fall and early winter, as well closer to the Earth’s surface (Screen and Simmonds, 2010a,b).
Because low PAR in another factor significantly limiting GPP at high latitudes (Figure 7; Dormann and Woodin, 2002; Madani et al., 2020), an increase in low, stratospheric clouds would moderately to dramatically reduce downwelling SW irradiance, resulting in lower PAR, cooler leaf temperatures, and other downstream effects (Figure 1). As in other systems, impacts of reduced downwelling PAR on plant carbon gain vary according to plant species and functional type, although reductions tend to be greatest in those with high light saturation points (Figure 4). For example, Chapin and Shaver (1985) demonstrated a reduction in growth under ~50% PPFDs for canopy but not understory species in arctic tussock and wet meadow tundra communities during summer. Forbs tend to be negatively impacted by reductions in PAR (Dormann and Woodin, 2002), and increasing clouds combined with greater competition for sunlight with encroaching shrubs and taller grasses could pose a challenge for this functional group. Productivity in boreal forest also declines significantly under >70% cloudy skies (Fan et al., 1995; Alton, 2008), while alpine shrublands appear relatively insensitive to increases in cloud cover (Letts et al., 2005; Alton et al., 2007). Lower incoming surface irradiance would also increase soil moisture and reduce evaporation, although this is unlikely to benefit plants since soil moisture is not a significant factor limiting GPP at high latitudes (the two are, rather, inversely correlated; Figure 7).
While diffuse light fertilization associated with an increase in clouds may offset losses in GPP somewhat in high LAI systems like the boreal forest, low LAI ecosystems such as the tundra appear to benefit the least (Section 3.2.2; Alton, 2008). Increased light penetration into tundra and boreal canopies under light cloud cover increases photosynthesis for understory plants in normally light-limited microsites (Young and Smith, 1983), although again, these trends may reverse under heavier cloud cover (Fan et al., 1995).
5 Conclusion
Given the changes in global clouds that are currently underway, it is critical to understand how plants have adapted to their native cloud regimes. In this review we aimed to provide a basic framework for beginning to interconnect the complex relationships between plants, clouds, and climate change. The continued study of plant-cloud interactions, especially for native plants but also for agricultural plants and invasive species, is strongly encouraged. It is also recommended that future plant ecophysiological studies record the specific type (s) of cloud present during measurement, rather than simply “cloudy” conditions. Continuous measurements of direct and diffuse PPFDs under different cloud regimes are also needed, both inside and outside the canopy. The impacts of nighttime compared to daytime clouds is another understudied area with potentially important ecophysiological impacts on plants, beyond the important temperature effects that dictate length of the growing season. Finally, measurements of cloud effects on plant water potentials are also lacking, especially for grasses and deciduous trees, and many tropical ecosystems.
Author contributions
NH: Conceptualization, Visualization¸ Writing – original draft, Writing – review & editing. AS: Investigation, Writing – review & editing. ZB: Investigation, Visualization, Writing – review & editing. WS: Conceptualization, Funding acquisition, Visualization¸ Writing – original draft, Writing – review & editing.
Funding
The author(s) declare financial support was received for the research, authorship, and/or publication of this article. This paper was published with the financial support of High Point University (NH) and Wake Forest University (WS); the Division of Integrative Organismal Systems, Physiological and Structural Systems, National Science Foundation, United States, Grant Nos. IOS-11122092 (WS) and IOS-1122064 (NH).
Acknowledgments
The authors thank the reviewers of this manuscript, whose comments and suggestions greatly enhanced the quality of this paper.
Conflict of interest
The authors declare that the research was conducted in the absence of any commercial or financial relationships that could be construed as a potential conflict of interest.
Publisher’s note
All claims expressed in this article are solely those of the authors and do not necessarily represent those of their affiliated organizations, or those of the publisher, the editors and the reviewers. Any product that may be evaluated in this article, or claim that may be made by its manufacturer, is not guaranteed or endorsed by the publisher.
Footnotes
References
Adir, N., Zer, H., Shochat, S., and Ohad, I. (2003). Photoinhibition–a historical perspective. Photosynth. Res. 76, 343–370. doi: 10.1023/A:1024969518145
Alados-Arboledas, L., Vida, J., and Olmo, F. J. (1995). The estimation of thermal atmospheric radiation under cloudy conditions. Int. J. Climatol. 15, 107–116. doi: 10.1002/joc.3370150111
Allen, R. J., Norris, J. R., and Kovilakam, M. (2014). Influence of anthropogenic aerosols and the Pacific decadal oscillation on tropical belt width. Nat. Geosci. 7, 270–274. doi: 10.1038/ngeo2091
Allen, R. J., Sherwood, S. C., Norris, J. R., and Zender, C. S. (2012). Recent northern hemisphere tropical expansion primarily driven by black carbon and tropospheric ozone. Nature 485, 350–354. doi: 10.1038/nature11097
Alter, P., Dreissen, A., Luo, F. L., and Matsubara, S. (2012). Acclimatory responses of Arabidopsis to fluctuating light environment: comparison of different sunfleck regimes and accessions. Photosynth. Res. 113, 221–237. doi: 10.1007/s11120-012-9757-2
Alton, P. B. (2008). Reduced carbon sequestration in terrestrial ecosystems under overcast skies compared to clear skies. Agric. For. Meteorol. 148, 1641–1653. doi: 10.1016/j.agrformet.2008.05.014
Alton, P. B., North, P. R., and Los, S. O. (2007). The impact of diffuse sunlight on canopy light-use efficiency, gross photosynthetic product and net ecosystem exchange in three forest biomes. Glob. Chang. Biol. 13, 776–787. doi: 10.1111/j.1365-2486.2007.01316.x
Alves, P. L. D. C., Magalhães, A. C., and Barja, P. R. (2002). The phenomenon of photoinhibition of photosynthesis and its importance in reforestation. Bot. Rev. 68, 193–208. doi: 10.1663/0006-8101(2002)068[0193:TPOPOP]2.0.CO;2
Amaya, D. J., Siler, N., Xie, S. P., and Miller, A. J. (2018). The interplay of internal and forced modes of Hadley cell expansion: lessons from the global warming hiatus. Clim. Dyn. 51, 305–319. doi: 10.1007/s00382-017-3921-5
Baldocchi, D. D., and Harley, P. C. (1995). Scaling carbon dioxide and water vapour exchange from leaf to canopy in a deciduous forest. II. Model testing and application. Plant Cell Environ. 18, 1157–1173. doi: 10.1111/j.1365-3040.1995.tb00626.x
Ball, M. C., Egerton, J. J. G., Leuning, R., Cunningham, R. B., and Dunne, P. (1997). Microclimate above grass adversely affects spring growth of seedling snow gum (Eucalyptus pauciflora). Plant Cell Environ. 20, 155–166. doi: 10.1046/j.1365-3040.1997.d01-61.x
Bannister, P. (1973). A note on some observations on frost damage in the field, with particular reference to various ferns. Trans. Bot. Soc. Edinburgh 42, 111–113.
Barnes, E. A., and Polvani, L. (2013). Response of the midlatitude jets, and of their variability, to increased greenhouse gases in the CMIP5 models. J. Clim. 26, 7117–7135. doi: 10.1175/JCLI-D-12-00536.1
Bayeur, N. M., Carpenter, K. L., and Hughes, N. M. (2018). Shade tolerance: an additional factor affecting the distribution of mountain beech and silver beech in New Zealand? Trees 32, 539–547. doi: 10.1007/s00468-018-1654-7
Bender, F. A., Ramanathan, V., and Tselioudis, G. (2012). Changes in extratropical storm track cloudiness 1983–2008: observational support for a poleward shift. Clim. Dyn. 38, 2037–2053. doi: 10.1007/s00382-011-1065-6
Berry, Z. C., and Goldsmith, G. R. (2020). Diffuse light and wetting differentially affect tropical tree leaf photosynthesis. New Phytol. 225, 143–153. doi: 10.1111/nph.16121
Berry, Z. C., Hughes, N. M., and Smith, W. K. (2014a). Cloud immersion: an important water source for spruce and fir saplings in the southern Appalachian Mountains. Oecologia 174, 319–326. doi: 10.1007/s00442-013-2770-0
Berry, Z. C., and Smith, W. K. (2012). Cloud pattern and water relations in Picea rubens and Abies fraseri, southern Appalachian Mountains, USA. Agric. For. Meteorol. 162-163, 27–34. doi: 10.1016/j.agrformet.2012.04.005
Berry, Z. C., and Smith, W. K. (2014). Experimental cloud immersion and foliar water uptake in saplings of Abies fraseri and Picea rubens. Trees 28, 115–123. doi: 10.1007/s00468-013-0934-5
Berry, Z. C., White, J. C., and Smith, W. K. (2014b). Foliar uptake, carbon fluxes and water status are affected by the timing of daily fog in saplings from a threatened cloud forest. Tree Physiol. 34, 459–470. doi: 10.1093/treephys/tpu032
Bhatia, K. T., Vecchi, G. A., Knutson, T. R., Murakami, H., Kossin, J., Dixon, K. W., et al. (2019). Recent increases in tropical cyclone intensification rates. Nat. Commun. 10:635. doi: 10.1038/s41467-019-08471-z
Blackport, R., and Screen, J. A. (2020). Weakened evidence for midlatitude impacts of Arctic warming. Nat. Clim. Chang. 10, 1065–1066. doi: 10.1038/s41558-020-00954-y
Boeke, R. C., and Taylor, P. C. (2018). Seasonal energy exchange in sea ice retreat regions contributes to differences in projected Arctic warming. Nat. Commun. 9:5017. doi: 10.1038/s41467-018-07061-9
Boucher, O., Randall, D., Artaxo, P., Bretherton, C., Feingold, G., Forster, P., et al. (2013). “Clouds and aerosols” in Climate change 2013: the physical science basis. Contribution of working group I to the fifth assessment report of the intergovernmental panel on climate change. eds. T. F. Stocker, D. Qin, G.-K. Plattner, M. Tignor, S. K. Allen, and J. Boschung, et al. (Cambridge, United Kingdom and New York, NY, USA: Cambridge University Press)
Breitler, J. C., Djerrab, D., Leran, S., Toniutti, L., Guittin, C., Severac, D., et al. (2020). Full moonlight-induced circadian clock entrainment in Coffea arabica. BMC Plant Biol. 20, 24–11. doi: 10.1186/s12870-020-2238-4
Brelsford, C. C., Nybakken, L., Kotilainen, T. K., and Robson, T. M. (2019). The influence of spectral composition on spring and autumn phenology in trees. Tree Physiol. 39, 925–950. doi: 10.1093/treephys/tpz026
Brodersen, C. R., and Vogelmann, T. C. (2010). Do changes in light direction affect absorption profiles in leaves? Funct. Plant Biol. 37, 403–412. doi: 10.1071/FP09262
Bruijnzeel, L. A., Mulligan, M., and Scatena, F. N. (2011). Hydrometeorology of tropical montane cloud forests: emerging patterns. Hydrol. Process. 25, 465–498. doi: 10.1002/hyp.7974
Byrne, M. P., and Schneider, T. (2016). Narrowing of the ITCZ in a warming climate: physical mechanisms. Geophys. Res. Lett. 43, 11–350. doi: 10.1002/2016GL070396
Byun, K., and Hamlet, A. F. (2018). Projected changes in future climate over the Midwest and Great Lakes region using downscaled CMIP5 ensembles. Int. J. Climatol. 38, e531–e553. doi: 10.1002/joc.5388
Callaway, R. M., Brooker, R. W., Choler, P., Kikvidze, Z., Lortie, C. J., Michalet, R., et al. (2002). Positive interactions among alpine plants increase with stress. Nature 417, 844–848. doi: 10.1038/nature00812
Capers, R. S., and Chazdon, R. L. (2004). Rapid assessment of understory light availability in a wet tropical forest. Agric. For. Meteorol. 123, 177–185. doi: 10.1016/j.agrformet.2003.12.009
Ceppi, P., Brient, F., Zelinka, M. D., and Hartmann, D. L. (2017). Cloud feedback mechanisms and their representation in global climate models. Wiley Interdiscip. Rev. Clim. Change 8:e465. doi: 10.1002/wcc.465
Ceppi, P., and Nowack, P. (2021). Observational evidence that cloud feedback amplifies global warming. Proc. Natl. Acad. Sci. 118:e2026290118. doi: 10.1073/pnas.2026290118
Chang, E. K., Ma, C. G., Zheng, C., and Yau, A. M. (2016). Observed and projected decrease in northern hemisphere extratropical cyclone activity in summer and its impacts on maximum temperature. Geophys. Res. Lett. 43, 2200–2208. doi: 10.1002/2016GL068172
Chapin, F. S. III, and Shaver, G. R. (1985). Individualistic growth response of tundra plant species to environmental manipulations in the field. Ecology 66, 564–576. doi: 10.2307/1940405
Chazdon, R. L., and Pearcy, R. W. (1986). Photosynthetic responses to light variation in rainforest species. Oecologia 69, 524–531. doi: 10.1007/BF00410358
Chen, L., Hänninen, H., Rossi, S., Smith, N. G., Pau, S., Liu, Z., et al. (2020). Leaf senescence exhibits stronger climatic responses during warm than during cold autumns. Nat. Clim. Chang. 10, 777–780. doi: 10.1038/s41558-020-0820-2
Chen, T., Rossow, W. B., and Zhang, Y. (2000). Radiative effects of cloud-type variations. J. Clim. 13, 264–286. doi: 10.1175/1520-0442(2000)013<0264:REOCTV>2.0.CO;2
Choi, Y. S., Lindzen, R. S., Ho, C. H., and Kim, J. (2010). Space observations of cold-cloud phase change. Proc. Natl. Acad. Sci. 107, 11211–11216. doi: 10.1073/pnas.1006241107
Chou, C., Chiang, J. C., Lan, C. W., Chung, C. H., Liao, Y. C., and Lee, C. J. (2013). Increase in the range between wet and dry season precipitation. Nat. Geosci. 6, 263–267. doi: 10.1038/ngeo1744
Christersson, L . (1985). Frost damage during the growing season. In Plant adaptation workshop, Tromsoe (Norway), 4–9 Sep 1983. Norwegian University Press.
Cleland, E. E., Chuine, I., Menzel, A., Mooney, H. A., and Schwartz, M. D. (2007). Shifting plant phenology in response to global change. Trends Ecol. Evol. 22, 357–365. doi: 10.1016/j.tree.2007.04.003
Coumou, D., Di Capua, G., Vavrus, S., Wang, L., and Wang, S. (2018). The influence of Arctic amplification on midlatitude summer circulation. Nat. Commun. 9, 1–12. doi: 10.1038/s41467-018-05256-8
Coumou, D., Lehmann, J., and Beckmann, J. (2015). The weakening summer circulation in the northern hemisphere midlatitudes. Science 348, 324–327. doi: 10.1126/science.1261768
Dang, Q. L., Lieffers, V. J., and Rothwell, R. L. (1992). Effects of summer frosts and subsequent shade on foliage gas exchange in peatland tamarack and black spruce. Can. J. For. Res. 22, 973–979. doi: 10.1139/x92-130
Davidson, N. J., Battaglia, M., and Close, D. C. (2004). Photosynthetic responses to overnight frost in Eucalyptus nitens and E. globulus. Trees 18, 245–252. doi: 10.1007/s00468-003-0298-3
Del Amor, F. M., and Marcelis, L. F. M. (2004). Regulation of growth and nutrient uptake under different transpiration regimes. In International symposium on soilless culture and hydroponics 697 (pp. 523–528).
Dengel, S., and Grace, J. (2010). Carbon dioxide exchange and canopy conductance of two coniferous forests under various sky conditions. Oecologia 164, 797–808. doi: 10.1007/s00442-010-1687-0
Denniston, R. F., Ummenhofer, C. C., Wanamaker, A. D., Lachniet, M. S., Villarini, G., Asmerom, Y., et al. (2016). Expansion and contraction of the indo-Pacific tropical rain belt over the last three millennia. Sci. Rep. 6:34485. doi: 10.1038/srep34485
Dial, R. J., Maher, C. T., Hewitt, R. E., and Sullivan, P. F. (2022). Sufficient conditions for rapid range expansion of a boreal conifer. Nature 608, 546–551. doi: 10.1038/s41586-022-05093-2
Dormann, C. F., and Woodin, S. J. (2002). Climate change in the Arctic: using plant functional types in a meta-analysis of field experiments. Funct. Ecol. 16, 4–17. doi: 10.1046/j.0269-8463.2001.00596.x
Duchon, C. E., and O’Malley, M. S. (1999). Estimating cloud type from pyranometer observations. J. Appl. Meteorol. 38, 132–141. doi: 10.1175/1520-0450(1999)038<0132:ECTFPO>2.0.CO;2
Durand, M., Murchie, E. H., Lindfors, A. V., Urban, O., Aphalo, P. J., and Robson, T. M. (2021). Diffuse solar radiation and canopy photosynthesis in a changing environment. Agric. For. Meteorol. 311:108684. doi: 10.1016/j.agrformet.2021.108684
Dye, D. G. (2004). Spectral composition and quanta-to-energy ratio of diffuse photosynthetically active radiation under diverse cloud conditions. J. Geophys. Res. Atmos. 109, D10203. doi: 10.1029/2003JD004251
Dye, D. G., Kobayashi, H., Wu, P., Syamsudin, F., Sulistyowati, R., and Sarodja, D. (2008). Cloud-induced variability of photosynthetically active radiation, tropical forest PAR absorption and photosynthesis in Central Borneo. In AGU spring meeting abstracts (2008, pp. B33A–B05A).
Earles, J. M., Théroux-Rancourt, G., Gilbert, M. E., McElrone, A. J., and Brodersen, C. R. (2017). Excess diffuse light absorption in upper mesophyll limits CO2 drawdown and depresses photosynthesis. Plant Physiol. 174, 1082–1096. doi: 10.1104/pp.17.00223
Eastman, R., and Warren, S. G. (2013). A 39-yr survey of cloud changes from land stations worldwide 1971–2009: long-term trends, relation to aerosols, and expansion of the tropical belt. J. Clim. 26, 1286–1303. doi: 10.1175/JCLI-D-12-00280.1
Eastman, R., and Warren, S. G. (2014). Diurnal cycles of cumulus, cumulonimbus, stratus, stratocumulus, and fog from surface observations over land and ocean. J. Clim. 27, 2386–2404. doi: 10.1175/JCLI-D-13-00352.1
Ebner, M., Miranda, T., and Roth-Nebelsick, A. (2011). Efficient fog harvesting by Stipagrostis sabulicola (Namib dune bushman grass). J. Arid Environ. 75, 524–531. doi: 10.1016/j.jaridenv.2011.01.004
Egerton, J. J., Banks, J. C., Gibson, A., Cunningham, R. B., and Ball, M. C. (2000). Facilitation of seedling establishment: reduction in irradiance enhances winter growth of Eucalyptus pauciflora. Ecology 81, 1437–1449. doi: 10.1890/0012-9658(2000)081[1437:FOSERI]2.0.CO;2
Einhorn, K. S., Rosenqvist, E., and Leverenz, J. W. (2004). Photoinhibition in seedlings of Fraxinus and Fagus under natural light conditions: implications for forest regeneration? Oecologia 140, 241–251. doi: 10.1007/s00442-004-1591-6
Eller, C. B., Lima, A. L., and Oliveira, R. S. (2013). Foliar uptake of fog water and transport belowground alleviates drought effects in the cloud forest tree species, Drimys brasiliensis (W interaceae). New Phytol. 199, 151–162. doi: 10.1111/nph.12248
Endler, J. A. (1993). The color of light in forests and its implications. Ecol. Monogr. 63, 1–27. doi: 10.2307/2937121
Estiarte, M., and Peñuelas, J. (2015). Alteration of the phenology of leaf senescence and fall in winter deciduous species by climate change: effects on nutrient proficiency. Glob. Chang. Biol. 21, 1005–1017. doi: 10.1111/gcb.12804
Eugster, W., Burkard, R., Holwerda, F., Scatena, F. N., and Bruijnzeel, L. S. (2006). Characteristics of fog and fogwater fluxes in a Puerto Rican elfin cloud forest. Agric. For. Meteorol. 139, 288–306. doi: 10.1016/j.agrformet.2006.07.008
Evans, M. A., Mac Intyre, S., and Kling, G. W. (2008). Internal wave effects on photosynthesis: experiments, theory, and modeling. Limnol. Oceanogr. 53, 339–353. doi: 10.4319/lo.2008.53.1.0339
Fan, S. M., Goulden, M. L., Munger, J. W., Daube, B. C., Bakwin, P. S., Wofsy, S. C., et al. (1995). Environmental controls on the photosynthesis and respiration of a boreal lichen woodland: a growing season of whole-ecosystem exchange measurements by eddy correlation. Oecologia 102, 443–452. doi: 10.1007/BF00341356
Farquhar, G. D., von Caemmerer, S., and Berry, J. A. (1980). A biochemical model of photosynthetic CO2 assimilation in leaves of C3 species. Planta 149, 78–90. doi: 10.1007/BF00386231
Feister, U., and Shields, J. (2005). Cloud and radiance measurements with the VIS/NIR daylight whole sky imager at Lindenberg (Germany). Meteorol. Zeitschrift (Berlin) 14, 627–639. doi: 10.1127/0941-2948/2005/0066
Feng, S., and Fu, Q. (2013). Expansion of global drylands under a warming climate. Atmos. Chem. Phys. 13, 10081–10094. doi: 10.5194/acp-13-10081-2013
Feygel'son, Y. M. (1988). Infrared radiation fluxes in the presence of cirrus clouds. Izv. Atmos. Ocean. Phys. 24:430.
Freedman, J. M., Fitzjarrald, D. R., Moore, K. E., and Sakai, R. K. (2001). Boundary layer clouds and vegetation–atmosphere feedbacks. J. Clim. 14, 180–197. doi: 10.1175/1520-0442(2001)013<0180:BLCAVA>2.0.CO;2
Fu, R. (2015). Global warming-accelerated drying in the tropics. Proc. Natl. Acad. Sci. 112, 3593–3594. doi: 10.1073/pnas.1503231112
Garratt, J. R. (2001). Clear-sky longwave irradiance at the Earth’s surface—evaluation of climate models. J. Clim. 14, 1647–1670. doi: 10.1175/1520-0442(2001)014<1647:CSLIAT>2.0.CO;2
Gasparini, B., and Lohmann, U. (2016). Why cirrus cloud seeding cannot substantially cool the planet. J. Geophys. Res. Atmos. 121, 4877–4893. doi: 10.1002/2015JD024666
Germino, M. J., and Smith, W. K. (1999). Sky exposure, crown architecture, and low-temperature photoinhibition in conifer seedlings at alpine treeline. Plant Cell Environ. 22, 407–415. doi: 10.1046/j.1365-3040.1999.00426.x
Germino, M. J., and Smith, W. K. (2000). Differences in microsite, plant form, and low-temperature photoinhibition in alpine plants. Arct. Antarct. Alp. Res. 32, 388–396. doi: 10.1080/15230430.2000.12003382
Germino, M. J., and Smith, W. K. (2001). Relative importance of microhabitat, plant form and photosynthetic physiology to carbon gain in two alpine herbs. Funct. Ecol. 15, 243–251. doi: 10.1046/j.1365-2435.2001.00506.x
Germino, M. J., Smith, W. K., and Resor, A. C. (2002). Conifer seedling distribution and survival in an alpine-treeline ecotone. Plant Ecol. 162, 157–168. doi: 10.1023/A:1020385320738
Gimeno, L., Stohl, A., Trigo, R. M., Dominguez, F., Yoshimura, K., Yu, L., et al. (2012). Oceanic and terrestrial sources of continental precipitation. Rev. Geophys. 50, 2012RG000389. doi: 10.1029/2012RG000389
Gisleröd, H. R., Selmer-Olsen, A. R., and Mortensen, L. M. (1987). The effect of air humidity on nutrient uptake of some greenhouse plants. Plant Soil 102, 193–196. doi: 10.1007/BF02370702
Goh, C. H., Ko, S. M., Koh, S., Kim, Y. J., and Bae, H. J. (2012). Photosynthesis and environments: photoinhibition and repair mechanisms in plants. J. Plant Biol. 55, 93–101. doi: 10.1007/s12374-011-9195-2
Goldsmith, G. R., Matzke, N. J., and Dawson, T. E. (2013). The incidence and implications of clouds for cloud forest plant water relations. Ecol. Lett. 16, 307–314. doi: 10.1111/ele.12039
Green, T. G. A., and Jane, G. T. (1983). Diurnal patterns of water potential in the evergreen cloud forests of the Kaimai ranges, North Island, New Zealand. N. Z. J. Bot. 21, 379–389. doi: 10.1080/0028825X.1983.10428570
Greenwald, R., Bergin, M. H., Xu, J., Cohan, D., Hoogenboom, G., and Chameides, W. L. (2006). The influence of aerosols on crop production: a study using the CERES crop model. Agric. Syst. 89, 390–413. doi: 10.1016/j.agsy.2005.10.004
Grise, K. M., and Davis, S. M. (2020). Hadley cell expansion in CMIP6 models. Atmos. Chem. Phys. 20, 5249–5268. doi: 10.5194/acp-20-5249-2020
Grise, K. M., and Polvani, L. M. (2016). Is climate sensitivity related to dynamical sensitivity? J. Geophys. Res. Atmos. 121, 5159–5176. doi: 10.1002/2015JD024687
Gross, H. L. (1983). Injuries to terminal shoots cause multiple-leadered nursery seedlings. Sault Ste. Marie, ON: Great Lakes Forest Research Centre.
Gu, L., Fuentes, J. D., Garstang, M., Da Silva, J. T., Heitz, R., Sigler, J., et al. (2001). Cloud modulation of surface solar irradiance at a pasture site in southern Brazil. Agric. For. Meteorol. 106, 117–129. doi: 10.1016/S0168-1923(00)00209-4
Gu, L., Fuentes, J. D., Shugart, H. H., Staebler, R. M., and Black, T. A. (1999). Responses of net ecosystem exchanges of carbon dioxide to changes in cloudiness: results from two North American deciduous forests. J. Geophys. Res. Atmos. 104, 31421–31434.
Gururani, M. A., Venkatesh, J., and Tran, L. S. P. (2015). Regulation of photosynthesis during abiotic stress-induced photoinhibition. Mol. Plant 8, 1304–1320. doi: 10.1016/j.molp.2015.05.005
Guzmán-Delgado, P., Laca, E., and Zwieniecki, M. A. (2021). Unravelling foliar water uptake pathways: the contribution of stomata and the cuticle. Plant Cell Environ. 44, 1728–1740. doi: 10.1111/pce.14041
Han, Y. J., Song, P. S., and Kim, J. L. (2007). Phytochrome-mediated photomorphogenesis in plants. J. Plant Biol. 50, 230–240. doi: 10.1007/BF03030650
Hansen, M. C., Potapov, P. V., Moore, R., Hancher, M., Turubanova, S. A., Tyukavina, A., et al. (2013). High-resolution global maps of 21st-century forest cover change. Science 342, 850–853. doi: 10.1126/science.1244693
Harrison, E. F., Minnis, P., Barkstrom, B. R., and Gibson, G. G. (1993). “Radiation budget at the top of the atmosphere” R. J. Gurney, J. L. Foster, and C. Parkinson, Eds. in Atlas of satellite observations related to global change, Cambridge University Press 19–38.
Hawcroft, M. K., Shaffrey, L. C., Hodges, K. I., and Dacre, H. F. (2012). How much northern hemisphere precipitation is associated with extratropical cyclones? Geophys. Res. Lett. 39, L24809. doi: 10.1029/2012GL053866
Heber, U., Bligny, R., Streb, P., and Douce, R. (1996). Photorespiration is essential for the protection of the photosynthetic apparatus of C3 plants against photoinactivation under sunlight. Bot. Acta 109, 307–315. doi: 10.1111/j.1438-8677.1996.tb00578.x
Held, I. M., and Soden, B. J. (2000). Water vapor feedback and global warming. Annu. Rev. Energy Environ. 25, 441–475. doi: 10.1146/annurev.energy.25.1.441
Helmer, E. H., Gerson, E. A., Baggett, L. S., Bird, B. J., Ruzycki, T. S., and Voggesser, S. M. (2019). Neotropical cloud forests and páramo to contract and dry from declines in cloud immersion and frost. PloS One 14:e0213155. doi: 10.1371/journal.pone.0213155
Hernandez Velasco, M., and Mattsson, A. (2020). Light shock stress after outdoor sunlight exposure in seedlings of Picea abies (L.) karst and Pinus sylvestris L. pre-cultivated under LEDs—possible mitigation treatments and their energy consumption. Forests 11:354. doi: 10.3390/f11030354
Hernandez-Moreno, J. M., Bayeur, N. M., Coley, H. D., and Hughes, N. M. (2017). Clouds homogenize shoot temperatures, transpiration, and photosynthesis within crowns of Abies fraseri (Pursh.) Poiret. Oecologia 183, 667–676. doi: 10.1007/s00442-016-3799-7
Holly, C., Laughlin, G. P., and Ball, M. C. (1994). Cold-induced photoinhibition and design of shelters for establishment of eucalypts in pasture. Aust. J. Bot. 42, 139–147. doi: 10.1071/BT9940139
Holopainen, J. K. (1990). The relationship between multiple leaders and mechanical and frost damage to the apical meristem of scots pine seedlings. Can. J. For. Res. 20, 280–284. doi: 10.1139/x90-041
Hu, J. I. A., Moore, D. J., Burns, S. P., and Monson, R. K. (2010). Longer growing seasons lead to less carbon sequestration by a subalpine forest. Glob. Chang. Biol. 16, 771–783. doi: 10.1111/j.1365-2486.2009.01967.x
Huang, R. S., Smith, W. K., and Yost, R. S. (1985). Influence of vesicular-arbuscular mycorrhiza on growth, water relations, and leaf orientation in Leucaena leucocephala (lam.) de wit. New Phytol. 99, 229–243. doi: 10.1111/j.1469-8137.1985.tb03652.x
Hughes, N. M., Carpenter, K. L., Cook, D. K., Keidel, T. S., Miller, C. N., Neal, J. L., et al. (2015). Effects of cumulus clouds on microclimate and shoot-level photosynthetic gas exchange in Picea engelmannii and Abies lasiocarpa at treeline, medicine Bow Mountains, Wyoming, USA. Agric. For. Meteorol. 201, 26–37. doi: 10.1016/j.agrformet.2014.10.012
Hughes, N. M., Johnson, D. M., Akhalkatsi, M., and Abdaladze, O. (2009). Characterizing Betula litwinowii seedling microsites at the alpine-treeline ecotone, central greater Caucasus Mountains, Georgia. Arct. Antarct. Alp. Res. 41, 112–118. doi: 10.1657/1523-0430-41.1.112
Hulshof, C. M., Waring, B. G., Powers, J. S., and Harrison, S. P. (2020). Trait-based signatures of cloud base height in a tropical cloud forest. Am. J. Bot. 107, 886–894. doi: 10.1002/ajb2.1483
Jakob, C., and Tselioudis, G. (2003). Objective identification of cloud regimes in the tropical western Pacific. Geophys. Res. Lett. 30:2082. doi: 10.1029/2003GL018367
Johnson, D. M., and Smith, W. K. (2008). Cloud immersion alters microclimate, photosynthesis and water relations in Rhododendron catawbiense and Abies fraseri seedlings in the southern Appalachian Mountains, USA. Tree Physiol. 28, 385–392. doi: 10.1093/treephys/28.3.385
Jordan, D. N., and Smith, W. K. (1994). Energy balance analysis of nighttime leaf temperatures and frost formation in a subalpine environment. Agric. For. Meteorol. 71, 359–372. doi: 10.1016/0168-1923(94)90020-5
Jordan, D. N., and Smith, W. K. (1995a). Microclimate factors influencing the frequency and duration of growth season frost for subalpine plants. Agric. For. Meteorol. 77, 17–30. doi: 10.1016/0168-1923(95)02233-N
Jordan, D. N., and Smith, W. K. (1995b). Radiation frost susceptibility and the association between sky exposure and leaf size. Oecologia 103, 43–48. doi: 10.1007/BF00328423
Kaiser, E., Morales, A., Harbinson, J., Heuvelink, E., Prinzenberg, A. E., and Marcelis, L. F. (2016). Metabolic and diffusional limitations of photosynthesis in fluctuating irradiance in Arabidopsis thaliana. Sci. Rep. 6:31252. doi: 10.1038/srep31252
Kang, S. M., Shin, Y., and Xie, S. P. (2018). Extratropical forcing and tropical rainfall distribution: energetics framework and ocean Ekman advection. Npj Clim. Atmos. Sci. 1, 1–10. doi: 10.1038/s41612-017-0004-6
Kanniah, K. D., Beringer, J., North, P., and Hutley, L. (2012). Control of atmospheric particles on diffuse radiation and terrestrial plant productivity: a review. Prog. Phys. Geogr. 36, 209–237. doi: 10.1177/0309133311434244
Katzenberger, A., Schewe, J., Pongratz, J., and Levermann, A. (2021). Robust increase of Indian monsoon rainfall and its variability under future warming in CMIP6 models. Earth Syst. Dynam. 12, 367–386. doi: 10.5194/esd-12-367-2021
Khanna, J., Medvigy, D., Fueglistaler, S., and Walko, R. (2017). Regional dry-season climate changes due to three decades of Amazonian deforestation. Nat. Clim. Chang. 7, 200–204. doi: 10.1038/nclimate3226
Kim, K. Y., Hamlington, B. D., Na, H., and Kim, J. (2016). Mechanism of seasonal Arctic Sea ice evolution and Arctic amplification. Cryosphere 10, 2191–2202. doi: 10.5194/tc-10-2191-2016
Kim, B. M., Son, S. W., Min, S. K., Jeong, J. H., Kim, S. J., Zhang, X., et al. (2014). Weakening of the stratospheric polar vortex by Arctic Sea-ice loss. Nat. Commun. 5, 4646–4648. doi: 10.1038/ncomms5646
Kitao, M., Qu, L., Koike, T., Tobita, H., and Maruyama, Y. (2004). Increased susceptibility to photoinhibition in pre-existing needles experiencing low temperature at spring budbreak in Sakhalin spruce (Picea glehnii) seedlings. Physiol. Plant. 122, 226–232. doi: 10.1111/j.1399-3054.2004.00393.x
Kivalov, S. N., and Fitzjarrald, D. R. (2019). Observing the whole-canopy short-term dynamic response to natural step changes in incident light: characteristics of tropical and temperate forests. Bound.-Layer Meteorol. 173, 1–52. doi: 10.1007/s10546-019-00460-5
Knapp, A. K., and Smith, W. K. (1988). Effect of water stress on stomatal and photosynthetic responses in subalpine plants to cloud patterns. Am. J. Bot. 75, 851–858. doi: 10.1002/j.1537-2197.1988.tb13508.x
Knapp, A. K., and Smith, W. K. (1990). Stomatal and photosynthetic responses to variable sunlight. Physiol. Plant. 78, 160–165. doi: 10.1111/j.1399-3054.1990.tb08731.x
Knohl, A., and Baldocchi, D. D. (2008). Effects of diffuse radiation on canopy gas exchange processes in a forest ecosystem. J. Geophys. Res. Biogeo. 113:G02023. doi: 10.1029/2007JG000663
Körner, C. (2003). Alpine plant life: functional plant ecology of high mountain ecosystems (2nd ed.) Springer Berlin, Heidelberg
Korznikov, K., Kislov, D., Doležal, J., and Altman, J. (2023). Poleward migration of tropical cyclones induced severe disturbance of boreal forest above 50°. Sci. Total Environ. 890:164376. doi: 10.1016/j.scitotenv.2023.164376
Kossin, J. P., Knapp, K. R., Olander, T. L., and Velden, C. S. (2020). Global increase in major tropical cyclone exceedance probability over the past four decades. Proc. Natl. Acad. Sci. 117, 11975–11980. doi: 10.1073/pnas.1920849117
Krause, G. H. (1994). “Photoinhibition induced by low temperatures” in Photoinhibition of photosynthesis, from molecular mechanism to the field, Baker, N.R., and Bowyer, J.R., Eds (Oxford, UK: BIOS Scientific Publishers Ltd) 331–348.
Kremers, K. S., Hollister, R. D., and Oberbauer, S. F. (2015). Diminished response of arctic plants to warming over time. PLoS One, 10, e0116586.
Ku, S. B., and Edwards, G. E. (1977). Oxygen inhibition of photosynthesis: II. Kinetic characteristics as affected by temperature. Plant Physiology, 5, 991–999.
Kunz, A., and Blanke, M. (2022). “60 years on”—effects of climatic change on tree phenology—a case study using pome fruit. Horticulturae 8:110. doi: 10.3390/horticulturae8020110
L’Ecuyer, T. S., Hang, Y., Matus, A. V., and Wang, Z. (2019). Reassessing the effect of cloud type on Earth’s energy balance in the age of active spaceborne observations. Part I: top of atmosphere and surface. J. Clim. 32, 6197–6217. doi: 10.1175/JCLI-D-18-0753.1
Lamaud, E., Brunet, Y., and Berbigier, P. (1996). Radiation and water use efficiencies of two coniferous forest canopies. Phys. Chem. Earth 21, 361–365. doi: 10.1016/S0079-1946(97)81124-X
Larcher, W. (2003). “Gas exchange in plants” in Physiological plant ecology: ecophysiology and stress physiology of functional groups (Berlin, Germany: Springer Science and Business Media), 91–138.
Lau, W. K., and Kim, K. M. (2015). Robust Hadley circulation changes and increasing global dryness due to CO2 warming from CMIP5 model projections. Proc. Natl. Acad. Sci. 112, 3630–3635. doi: 10.1073/pnas.1418682112
Lawson, T. (2009). Guard cell photosynthesis and stomatal function. New Phytol. 181, 13–34. doi: 10.1111/j.1469-8137.2008.02685.x
Lawton, R. O., Nair, U. S., Pielke, R. A. Sr., and Welch, R. M. (2001). Climatic impact of tropical lowland deforestation on nearby montane cloud forests. Science 294, 584–587. doi: 10.1126/science.1062459
Lee, S., and Feldstein, S. B. (2013). Detecting ozone-and greenhouse gas–driven wind trends with observational data. Science 339, 563–567. doi: 10.1126/science.1225154
Letts, M. G., Lafleur, P. M., and Roulet, N. T. (2005). On the relationship between cloudiness and net ecosystem carbon dioxide exchange in a peatland ecosystem. Ecoscience 12, 53–69. doi: 10.2980/i1195-6860-12-1-53.1
Letts, M. G., and Mulligan, M. (2005). The impact of light quality and leaf wetness on photosynthesis in north-west Andean tropical montane cloud forest. J. Trop. Ecol. 21, 549–557. doi: 10.1017/S0266467405002488
Letts, M. G., Mulligan, M., Rincón-Romero, M. E., and Bruijnzeel, L. A. (2010). “Environmental controls on photosynthetic rates of lower montane cloud forest vegetation in South-Western Colombia” Bruijnzeel, L. A., Scatena, F. N., and Hamilton, L.S., Eds.in Tropical montane cloud forests: science for conservation and management (Cambridge: Cambridge University Press), 465–478.
Leuning, R. (1988). Leaf temperatures during radiation frost part II. A steady state theory. Agric. For. Meteorol. 42, 135–155. doi: 10.1016/0168-1923(88)90073-1
Leuning, R. (1989). Leaf energy balances: developments and applications. Philos. Trans. R Soc. Lond. B Biol. Sci. 324, 191–206.
Li, J., Huang, J., Stamnes, K., Wang, T., Lv, Q., and Jin, H. (2015). A global survey of cloud overlap based on CALIPSO and cloud sat measurements. Atmos. Chem. Phys. 15, 519–536. doi: 10.5194/acp-15-519-2015
Li, Y., Thompson, D. W., Bony, S., and Merlis, T. M. (2019). Thermodynamic control on the poleward shift of the extratropical jet in climate change simulations: the role of rising high clouds and their radiative effects. J. Clim. 32, 917–934. doi: 10.1175/JCLI-D-18-0417.1
Limm, E. B., Simonin, K. A., Bothman, A. G., and Dawson, T. E. (2009). Foliar water uptake: a common water acquisition strategy for plants of the redwood forest. Oecologia 161, 449–459. doi: 10.1007/s00442-009-1400-3
Liu, L., Cheng, Y., Wang, S., Wei, C., Pöhlker, M. L., Pöhlker, C., et al. (2020). Impact of biomass burning aerosols on radiation, clouds, and precipitation over the Amazon: relative importance of aerosol–cloud and aerosol–radiation interactions. Atmos. Chem. Phys. 20, 13283–13301. doi: 10.5194/acp-20-13283-2020
Liu, R., Liu, S. C., Shiu, C. J., Li, J., and Zhang, Y. (2016). Trends of regional precipitation and their control mechanisms during 1979–2013. Adv. Atmos. Sci. 33, 164–174. doi: 10.1007/s00376-015-5117-4
Lohmann, U., and Gasparini, B. (2017). A cirrus cloud climate dial? Science 357, 248–249. doi: 10.1126/science.aan3325
Loreto, F., Baker, N. R., and Ort, D. R. (2004). “Chloroplast to leaf” in Photosynthetic adaptation Smith, W. K., Vogelmann, T. C., and Critchley, C., Eds.(New York, NY: Springer), 231–261.
Los, S. O., Street-Perrott, F. A., Loader, N. J., and Froyd, C. A. (2021). Detection of signals linked to climate change, land-cover change and climate oscillators in tropical montane cloud forests. Remote Sens. Environ. 260:112431. doi: 10.1016/j.rse.2021.112431
Los, S. O., Street-Perrott, F. A., Loader, N. J., Froyd, C. A., Cuní-Sanchez, A., and Marchant, R. A. (2019). Sensitivity of a tropical montane cloud forest to climate change, present, past and future: Mt. Marsabit, N. Kenya. Quat. Sci. Rev. 218, 34–48. doi: 10.1016/j.quascirev.2019.06.016
Lu, J., Vecchi, G. A., and Reichler, T. (2007). Expansion of the Hadley cell under global warming. Geophys. Res. Lett. 34:L06805. doi: 10.1029/2006GL028443
Lundmark, T., and Hällgren, J. E. (1987). Effects of frost on shaded and exposed spruce and pine seedlings planted in the field. Can. J. For. Res. 17, 1197–1201. doi: 10.1139/x87-184
Madani, N., Parazoo, N. C., Kimball, J. S., Ballantyne, A. P., Reichle, R. H., Maneta, M., et al. (2020). Recent amplified global gross primary productivity due to temperature increase is offset by reduced productivity due to water constraints. AGU Adv. 1:e2020AV000180. doi: 10.1029/2020AV000180
Maher, E. L., and Germino, M. J. (2006). Microsite differentiation among conifer species during seedling establishment at alpine treeline. Ecoscience 13, 334–341. doi: 10.2980/i1195-6860-13-3-334.1
Maher, E. L., Germino, M. J., and Hasselquist, N. J. (2005). Interactive effects of tree and herb cover on survivorship, physiology, and microclimate of conifer seedlings at the alpine tree-line ecotone. Can. J. For. Res. 35, 567–574. doi: 10.1139/x04-201
Mamalakis, A., Randerson, J. T., Yu, J. Y., Pritchard, M. S., Magnusdottir, G., Smyth, P., et al. (2021). Zonally contrasting shifts of the tropical rain belt in response to climate change. Nat. Clim. Chang. 11, 143–151. doi: 10.1038/s41558-020-00963-x
Martínez-Ferri, E., Manrique, E., Valladares, F., and Balaguer, L. (2004). Winter photoinhibition in the field involves different processes in four co-occurring Mediterranean tree species. Tree Physiol. 24, 981–990. doi: 10.1093/treephys/24.9.981
Marvel, K., Zelinka, M., Klein, S. A., Bonfils, C., Caldwell, P., Doutriaux, C., et al. (2015). External influences on modeled and observed cloud trends. J. Clim. 28, 4820–4840. doi: 10.1175/JCLI-D-14-00734.1
Mateos, D., Antón, M., Valenzuela, A., Cazorla, A., Olmo, F. J., and Alados-Arboledas, L. (2013). Short-wave radiative forcing at the surface for cloudy systems at a midlatitude site. Tellus B Chem. Phys. Meteorol. 65:21069. doi: 10.3402/tellusb.v65i0.21069
Mbengue, C., and Schneider, T. (2018). Linking Hadley circulation and storm tracks in a conceptual model of the atmospheric energy balance. J. Atmos. Sci. 75, 841–856. doi: 10.1175/JAS-D-17-0098.1
Mekonnen, Z. A., Riley, W. J., Berner, L. T., Bouskill, N. J., Torn, M. S., Iwahana, G., et al. (2021). Arctic tundra shrubification: a review of mechanisms and impacts on ecosystem carbon balance. Environ. Res. Lett. 16:053001. doi: 10.1088/1748-9326/abf28b
Mitchell, D. L., and Finnegan, W. (2009). Modification of cirrus clouds to reduce global warming. Environ. Res. Lett. 4:045102. doi: 10.1088/1748-9326/4/4/045102
Morales, A., and Kaiser, E. (2020). Photosynthetic acclimation to fluctuating irradiance in plants. Front. Plant Sci. 11:268. doi: 10.3389/fpls.2020.00268
Mülmenstädt, J., Sourdeval, O., Henderson, D. S., L'Ecuyer, T. S., Unglaub, C., Jungandreas, L., et al. (2018). Using CALIOP to estimate cloud-field base height and its uncertainty: the Cloud Base altitude spatial extrapolator (CBASE) algorithm and dataset. Earth Syst. Sci. Data 10, 2279–2293. doi: 10.5194/essd-10-2279-2018
Murakami, H., Delworth, T. L., Cooke, W. F., Zhao, M., Xiang, B., and Hsu, P. C. (2020). Detected climatic change in global distribution of tropical cyclones. Proc. Natl. Acad. Sci. 117, 10706–10714. doi: 10.1073/pnas.1922500117
Niinemets, Ü., and Sack, L. (2006). “Structural determinants of leaf light-harvesting capacity and photosynthetic potentials” Progress in Botany. 67, 385–419.
Niyogi, D., Chang, H. I., Saxena, V. K., Holt, T., Alapaty, K., Booker, F., et al. (2004). Direct observations of the effects of aerosol loading on net ecosystem CO2 exchanges over different landscapes. Geophys. Res. Lett. 31:L20506. doi: 10.1029/2004GL020915
Norris, J. R., Allen, R. J., Evan, A. T., Zelinka, M. D., O’Dell, C. W., and Klein, S. A. (2016). Evidence for climate change in the satellite cloud record. Nature 536, 72–75. doi: 10.1038/nature18273
O’Gorman, P. A. (2010). Understanding the varied response of the extratropical storm tracks to climate change. Proc. Natl. Acad. Sci. 107, 19176–19180. doi: 10.1073/pnas.1011547107
Olson, D. M., Dinerstein, E., Wikramanayake, E. D., Burgess, N. D., Powell, G. V., Underwood, E. C., et al. (2001). Terrestrial ecoregions of the world: a new map of life on earth: a new global map of terrestrial ecoregions provides an innovative tool for conserving biodiversity. Bio Sci. 51, 933–938. doi: 10.1641/0006-3568(2001)051[0933:TEOTWA]2.0.CO;2
Ooba, M., Takagi, K., and Takahashi, H. (2003). Numerical simulation of the time-averaged rate of photosynthesis in a leaf under alternating light. J. Agric. Meteorol. 59, 259–267. doi: 10.2480/agrmet.59.259
Örlander, G. (1993). Shading reduces both visible and invisible frost damage to Norway spruce seedlings in the field. For. Int. J. For. Res. 66, 27–36. doi: 10.1093/forestry/66.1.27
Page, J. (2012). “The role of solar-radiation climatology in the design of photovoltaic systems” Eds: Markvart, T., and Castañer, L. in Practical handbook of photovoltaics (Cambridge, MA: Academic Press), 573–643.
Paton, D. M. (1988). Genesis of an inverted treeline associated with a frost hollow in South-Eastern Australia. Aust. J. Bot. 36, 655–663. doi: 10.1071/BT9880655
Penner, J. E., Lister, D., Griggs, D. J., Dokken, D. J., and McFarland, M. (1999). Aviation and the global atmosphere: A special report of the intergovernmental panel on climate change. Cambridge: Cambridge University Press.
Petrie, R. E., Shaffrey, L. C., and Sutton, R. (2015). Atmospheric response in summer linked to recent Arctic Sea ice loss. Q. J. R. Meteorol. Soc. 141, 2070–2076. doi: 10.1002/qj.2502
Piacentini, R. D., Salum, G. M., Fraidenraich, N., and Tiba, C. (2011). Extreme total solar irradiance due to cloud enhancement at sea level of the NE Atlantic coast of Brazil. Renew. Energy 36, 409–412. doi: 10.1016/j.renene.2010.06.009
Piedehierro, A. A., Antón, M., Cazorla, A., Alados-Arboledas, L., and Olmo, F. J. (2014). Evaluation of enhancement events of total solar irradiance during cloudy conditions at Granada (southeastern Spain). Atmos. Res. 135-136, 1–7. doi: 10.1016/j.atmosres.2013.08.008
Polgar, C., Gallinat, A., and Primack, R. B. (2014). Drivers of leaf-out phenology and their implications for species invasions: insights from Thoreau's Concord. New Phytol. 202, 106–115. doi: 10.1111/nph.12647
Polgar, C. A., and Primack, R. B. (2011). Leaf-out phenology of temperate woody plants: from trees to ecosystems. New Phytol. 191, 926–941. doi: 10.1111/j.1469-8137.2011.03803.x
Prein, A. F., and Heymsfield, A. J. (2020). Increased melting level height impacts surface precipitation phase and intensity. Nat. Clim. Chang. 10, 771–776. doi: 10.1038/s41558-020-0825-x
Raitio, H. (1987). Site elevation differences in frost damage to scots pine (Pinus sylvestris). For. Ecol. Manag. 20, 299–306. doi: 10.1016/0378-1127(87)90086-7
Ramanathan, V., Ramana, M. V., Roberts, G., Kim, D., Corrigan, C., Chung, C., et al. (2007). Warming trends in Asia amplified by brown cloud solar absorption. Nature 448, 575–578. doi: 10.1038/nature06019
Ramankutty, N., Evan, A. T., Monfreda, C., and Foley, J. A. (2008). Farming the planet: 1. Geographic distribution of global agricultural lands in the year 2000. Glob. Biogeochem. Cycles 22:GB1003. doi: 10.1029/2007GB002952
Rantanen, M., Karpechko, A. Y., Lipponen, A., Nordling, K., Hyvärinen, O., Ruosteenoja, K., et al. (2022). The Arctic has warmed nearly four times faster than the globe since 1979. Commun. Earth Environ. 3:168. doi: 10.1038/s43247-022-00498-3
Ray, D. K. (2013). “Tropical montane cloud forests” in Vulnerability of water resources to climate (Amsterdam: Elsevier Inc.), 79–85.
Reich, P. B., Bermudez, R., Montgomery, R. A., Rich, R. L., Rice, K. E., Hobbie, S. E., et al. (2022). Even modest climate change may lead to major transitions in boreal forests. Nature 608, 540–545. doi: 10.1038/s41586-022-05076-3
Reifsnyder, W. E., and Lull, H. W. (1965). Radiant energy in relation to forests (1344). US Department of Agriculture, Forest Service.
Reinhardt, K., and Smith, W. K. (2008a). Impacts of cloud immersion on microclimate, photosynthesis and water relations of Abies fraseri (Pursh.) Poiret in a temperate mountain cloud forest. Oecologia 158, 229–238. doi: 10.1007/s00442-008-1128-5
Reinhardt, K., and Smith, W. K. (2008b). Leaf gas exchange of understory spruce–fir saplings in relict cloud forests, southern Appalachian Mountains, USA. Tree Physiol. 28, 113–122. doi: 10.1093/treephys/28.1.113
Reinhardt, K., Smith, W. K., and Carter, G. A. (2010). Clouds and cloud immersion alter photosynthetic light quality in a temperate mountain cloud forest. Botany 88, 462–470. doi: 10.1139/B10-008
Rew, L. J., McDougall, K. L., Alexander, J. M., Daehler, C. C., Essl, F., Haider, S., et al. (2020). Moving up and over: redistribution of plants in alpine, Arctic, and Antarctic ecosystems under global change. Arct. Antarct. Alp. Res. 52, 651–665. doi: 10.1080/15230430.2020.1845919
Richardson, A. D., Denny, E. G., Siccama, T. G., and Lee, X. (2003). Evidence for a rising cloud ceiling in eastern North America. J. Clim. 16, 2093–2098. doi: 10.1175/1520-0442(2003)016<2093:EFARCC>2.0.CO;2
Rochette, P., Desjardins, R. L., Pattey, E., and Lessard, R. (1996). Instantaneous measurement of radiation and water use efficiencies of a maize crop. Agron. J. 88, 627–635. doi: 10.2134/agronj1996.00021962008800040022x
Rollings, M., and Merlis, T. M. (2021). The observed relationship between Pacific SST variability and Hadley cell extent trends in reanalyses. J. Clim. 34, 2511–2527. doi: 10.1175/JCLI-D-20-0410.1
Rosenfeld, D., Lohmann, U., Raga, G. B., O'Dowd, C. D., Kulmala, M., Fuzzi, S., et al. (2008). Flood or drought: how do aerosols affect precipitation? Science 321, 1309–1313. doi: 10.1126/science.1160606
Rosenqvist, E., and van Kooten, O. (2003). “Chlorophyll fluorescence: a general description and nomenclature” DeEll, J.R., and Toivonen, P.M.A. (eds) in Practical applications of chlorophyll fluorescence in plant biology (Boston, MA: Springer), 31–77.
Rossow, W. B., and Schiffer, R. A. (1999). Advances in understanding clouds from ISCCP. Bull. Am. Meteorol. Soc. 80, 2261–2287. doi: 10.1175/1520-0477(1999)080<2261:AIUCFI>2.0.CO;2
Rouphael, Y., Cardarelli, M., Schwarz, D., Franken, P., and Colla, G. (2012). “Effects of drought on nutrient uptake and assimilation in vegetable crops” in Plant responses to drought stress: from morphological to molecular features. ed. R. Aroca , (Springer-Verlag Berlin Heidelberg) 171–195.
Saeed, F., Bethke, I., Fischer, E., Legutke, S., Shiogama, H., Stone, D. A., et al. (2018). Robust changes in tropical rainy season length at 1.5 C and 2 C. Environ. Res. Lett. 13:064024. doi: 10.1088/1748-9326/aab797
Salama, A. M., Ezzat, A., El-Ramady, H., Alam-Eldein, S. M., Okba, S. K., Elmenofy, H. M., et al. (2021). Temperate fruit trees under climate change: challenges for dormancy and chilling requirements in warm winter regions. Horticulturae 7:86. doi: 10.3390/horticulturae7040086
Sanchez, A., Hughes, N. M., and Smith, W. K. (2014a). Importance of natural cloud regimes to ecophysiology in the alpine species, Caltha leptosepala and Arnica parryi, snowy Range Mountains, Southeast Wyoming, USA. Funct. Plant Biol. 42, 186–197. doi: 10.1071/FP14096
Sanchez, A., Hughes, N. M., and Smith, W. K. (2016). Leaf/shoot level ecophysiology in two broadleaf and two needle-leaf species under representative cloud regimes at alpine treeline. J. Plant Ecol. 9, 762–772. doi: 10.1093/jpe/rtw019
Sanchez, A., Posada, J. M., and Smith, W. K. (2014b). Dynamic cloud regimes, incident sunlight, and leaf temperatures in Espeletia grandiflora and Chusquea tessellata, two representative species of the Andean Páramo, Colombia. Arct. Antarct. Alp. Res. 46, 371–378. doi: 10.1657/1938-4246-46.2.371
Sanchez, A., and Smith, W. K. (2015). No evidence for photoinhibition of photosynthesis in alpine Caltha leptosepala DC. Alp. Bot. 125, 41–50. doi: 10.1007/s00035-015-0146-2
Sassen, K., and Wang, Z. (2008). Classifying clouds around the globe with the cloud sat radar: 1-year of results. Geophys. Res. Lett. 35:L04805. doi: 10.1029/2007GL032591
Sassen, K., and Wang, Z. (2012). The clouds of the middle troposphere: composition, radiative impact, and global distribution. Surv. Geophys. 33, 677–691. doi: 10.1007/s10712-011-9163-x
Schade, N. H., Macke, A., Sandmann, H., and Stick, C. (2007). Enhanced solar global irradiance during cloudy sky conditions. Meteorol. Z. 16, 295–303. doi: 10.1127/0941-2948/2007/0206
Scheff, J., and Frierson, D. M. (2012). Robust future precipitation declines in CMIP5 largely reflect the poleward expansion of model subtropical dry zones. Geophys. Res. Lett. 39:L18704. doi: 10.1029/2012GL052910
Schneider, T., Teixeira, J., Bretherton, C. S., Brient, F., Pressel, K. G., Schär, C., et al. (2017). Climate goals and computing the future of clouds. Nat. Clim. Chang. 7, 3–5. doi: 10.1038/nclimate3190
Scholes, M., Scholes, R. B., and Lucas, M. (2015). “Why are clouds the wild card in climate change?” in Climate change: Briefings from southern Africa (Johannesburg, South Africa: Wits University Press)
Scholl, M., Eugster, W., and Burkard, R. (2011). Understanding the role of fog in forest hydrology: stable isotopes as tools for determining input and partitioning of cloud water in montane forests. Hydrol. Process. 25, 353–366. doi: 10.1002/hyp.7762
Schreel, J. D., von der Crone, J. S., Kangur, O., and Steppe, K. (2019). Influence of drought on foliar water uptake capacity of temperate tree species. Forests 10:562. doi: 10.3390/f10070562
Schröder, F., Kärcher, B., Duroure, C., Ström, J., Petzold, A., Gayet, J. F., et al. (2000). On the transition of contrails into cirrus clouds. J. Atmos. Sci. 57, 464–480. doi: 10.1175/1520-0469(2000)057<0464:OTTOCI>2.0.CO;2
Screen, J. A., and Simmonds, I. (2010a). Increasing fall-winter energy loss from the Arctic Ocean and its role in Arctic temperature amplification. Geophys. Res. Lett. 37:L16707. doi: 10.1029/2010GL044136
Screen, J. A., and Simmonds, I. (2010b). The central role of diminishing sea ice in recent Arctic temperature amplification. Nature 464, 1334–1337. doi: 10.1038/nature09051
Sedlar, J., Riihimaki, L. D., Lantz, K., and Turner, D. D. (2021). Development of a random-forest cloud-regime classification model based on surface radiation and cloud products. J. Appl. Meteorol. Climatol. 60, 477–491. doi: 10.1175/JAMC-D-20-0153.1
Sejas, S. A., Cai, M., Hu, A., Meehl, G. A., Washington, W., and Taylor, P. C. (2014). Individual feedback contributions to the seasonality of surface warming. J. Clim. 27, 5653–5669. doi: 10.1175/JCLI-D-13-00658.1
Serreze, M. C., and Barry, R. G. (2011). Processes and impacts of Arctic amplification: a research synthesis. Glob. Planet. Chang. 77, 85–96. doi: 10.1016/j.gloplacha.2011.03.004
Seyednasrollah, B., Young, A. M., Li, X., Milliman, T., Ault, T., Frolking, S., et al. (2020). Sensitivity of deciduous forest phenology to environmental drivers: implications for climate change impacts across North America. Geophys. Res. Lett. 47:e2019GL086788. doi: 10.1029/2019GL086788
Shaw, T. A. (2019). Mechanisms of future predicted changes in the zonal mean mid-latitude circulation. Curr. Clim. Chang. Rep. 5, 345–357. doi: 10.1007/s40641-019-00145-8
Shimazaki, K. I., Doi, M., Assmann, S. M., and Kinoshita, T. (2007). Light regulation of stomatal movement. Annu. Rev. Plant Biol. 58, 219–247. doi: 10.1146/annurev.arplant.57.032905.105434
Sklenář, P., Bendix, J., and Balslev, H. (2008). Cloud frequency correlates to plant species composition in the high Andes of Ecuador. Basic Appl. Ecol. 9, 504–513. doi: 10.1016/j.baae.2007.09.007
Smith, W. K., and Berry, Z. C. (2013). Sunflecks? Tree Physiol. 33, 233–237. doi: 10.1093/treephys/tpt005
Smith, W. K., Germino, M. J., Hancock, T. E., and Johnson, D. M. (2003). Another perspective on altitudinal limits of alpine timberlines. Tree Physiol. 23, 1101–1112. doi: 10.1093/treephys/23.16.1101
Smith, W. K., and McClean, T. M. (1989). Adaptive relationship between leaf water repellency, stomatal distribution, and gas exchange. Am. J. Bot. 76, 465–469. doi: 10.1002/j.1537-2197.1989.tb11335.x
Smith, W. K., Vogelmann, T. C., DeLucia, E. H., Bell, D. T., and Shepherd, K. A. (1997). Leaf form and photosynthesis. Bio. Sci. 47, 785–793. doi: 10.2307/1313100
Sobel, A. H., Camargo, S. J., Hall, T. M., Lee, C. Y., Tippett, M. K., and Wing, A. A. (2016). Human influence on tropical cyclone intensity. Science 353, 242–246. doi: 10.1126/science.aaf6574
Souza, E. P., Rennó, N. O., and Silva Dias, M. A. (2000). Convective circulations induced by surface heterogeneities. J. Atmos. Sci. 57, 2915–2922. doi: 10.1175/1520-0469(2000)057<2915:CCIBSH>2.0.CO;2
Spiridonov, V., and Ćurić, M. (2021). “Clouds and precipitation” in Fundamentals of meteorology (Berlin: Springer), 135–158.
Staal, A., Flores, B. M., Aguiar, A. P. D., Bosmans, J. H., Fetzer, I., and Tuinenburg, O. A. (2020). Feedback between drought and deforestation in the Amazon. Environ. Res. Lett. 15:044024. doi: 10.1088/1748-9326/ab738e
Steiner, A. L., and Chameides, W. L. (2005). Aerosol-induced thermal effects increase modelled terrestrial photosynthesis and transpiration. Tellus B Chem. Phys. Meteorol. 57, 404–411. doi: 10.3402/tellusb.v57i5.16559
Stephens, G. L. (2005). Cloud feedbacks in the climate system: a critical review. J. Clim. 18, 237–273. doi: 10.1175/JCLI-3243.1
Stephens, G. L., Wild, M., Stackhouse, P. W., L’Ecuyer, T., Kato, S., and Henderson, D. S. (2012). The global character of the flux of downward longwave radiation. J. Clim. 25, 2329–2340. doi: 10.1175/JCLI-D-11-00262.1
Still, C. J., Foster, P. N., and Schneider, S. H. (1999). Simulating the effects of climate change on tropical montane cloud forests. Nature 398, 608–610. doi: 10.1038/19293
Su, H., Jiang, J. H., Neelin, J. D., Shen, T. J., Zhai, C., Yue, Q., et al. (2017). Tightening of tropical ascent and high clouds key to precipitation change in a warmer climate. Nat. Commun. 8:15771. doi: 10.1038/ncomms15771
Su, H., Wu, L., Zhai, C., Jiang, J. H., Neelin, J. D., and Yung, Y. L. (2020). Observed tightening of tropical ascent in recent decades and linkage to regional precipitation changes. Geophys. Res. Lett. 47:e2019GL085809. doi: 10.1029/2019GL085809
Tao, L., Hu, Y., and Liu, J. (2016). Anthropogenic forcing on the Hadley circulation in CMIP5 simulations. Clim. Dyn. 46, 3337–3350. doi: 10.1007/s00382-015-2772-1
Taylor, S., and Kumar, L. (2016). Global climate change impacts on pacific islands terrestrial biodiversity: a review. Trop. Conserv. Sci. 9, 203–223. doi: 10.1177/194008291600900111
Ten Hoeve, J. E., Jacobson, M. Z., and Remer, L. A. (2012). Comparing results from a physical model with satellite and in situ observations to determine whether biomass burning aerosols over the Amazon brighten or burn off clouds. J. Geophys. Res. Atmos. 117:D08203. doi: 10.1029/2011JD016856
Teuling, A. J., Taylor, C. M., Meirink, J. F., Melsen, L. A., Miralles, D. G., Van Heerwaarden, C. C., et al. (2017). Observational evidence for cloud cover enhancement over western European forests. Nat. Commun. 8:14065. doi: 10.1038/ncomms14065
Tzoumanikas, P., Nikitidou, E., Bais, A. F., and Kazantzidis, A. (2016). The effect of clouds on surface solar irradiance, based on data from an all-sky imaging system. Renew. Energy 95, 314–322. doi: 10.1016/j.renene.2016.04.026
Urban, O., Janouš, D., Acosta, M., Czerný, R., Markova, I., Navratil, M., et al. (2007). Ecophysiological controls over the net ecosystem exchange of mountain spruce stand. Comparison of the response in direct vs. diffuse solar radiation. Glob. Chang. Biol. 13, 157–168. doi: 10.1111/j.1365-2486.2006.01265.x
Urban, O., Klem, K., Ač, A., Havránková, K., Holišová, P., Navrátil, M., et al. (2012). Impact of clear and cloudy sky conditions on the vertical distribution of photosynthetic CO2 uptake within a spruce canopy. Funct. Ecol. 26, 46–55. doi: 10.1111/j.1365-2435.2011.01934.x
Urban, O., Klem, K., Holišová, P., Šigut, L., Šprtová, M., Teslová-Navrátilová, P., et al. (2014). Impact of elevated CO2 concentration on dynamics of leaf photosynthesis in Fagus sylvatica is modulated by sky conditions. Environ. Pollut. 185, 271–280. doi: 10.1016/j.envpol.2013.11.009
Vecchi, G. A., Landsea, C., Zhang, W., Villarini, G., and Knutson, T. (2021). Changes in Atlantic major hurricane frequency since the late-19th century. Nat. Commun. 12:4054. doi: 10.1038/s41467-021-24268-5
Verhoeven, A. (2014). Sustained energy dissipation in winter evergreens. New Phytol. 201, 57–65. doi: 10.1111/nph.12466
Verma, A., Chandel, V., and Ghosh, S. (2022). Climate drivers of the variations of vegetation productivity in India. Environ. Res. Lett. 17:084023. doi: 10.1088/1748-9326/ac7c7f
Vitasse, Y., Baumgarten, F., Zohner, C. M., Rutishauser, T., Pietragalla, B., Gehrig, R., et al. (2022). The great acceleration of plant phenological shifts. Nat. Clim. Chang. 12, 300–302. doi: 10.1038/s41558-022-01283-y
Vogelmann, T. C., and Martin, G. (1993). The functional significance of palisade tissue: penetration of directional versus diffuse light. Plant Cell Environ. 16, 65–72. doi: 10.1111/j.1365-3040.1993.tb00845.x
Wang, J., Chagnon, F. J., Williams, E. R., Betts, A. K., Renno, N. O., Machado, L. A., et al. (2009). Impact of deforestation in the Amazon basin on cloud climatology. Proc. Natl. Acad. Sci. 106, 3670–3674. doi: 10.1073/pnas.0810156106
Wang, P., Fu, C., Wang, L., and Yan, T. (2022). Delayed autumnal leaf senescence following nutrient fertilization results in altered nitrogen resorption. Tree Physiol. 42, 1549–1559. doi: 10.1093/treephys/tpac028
Warren, S. G., Eastman, R. M., and Hahn, C. J. (2007). A survey of changes in cloud cover and cloud types over land from surface observations, 1971–96. J. Clim. 20, 717–738. doi: 10.1175/JCLI4031.1
Watling, J. R., Robinson, S. A., Woodrow, I. E., and Osmond, C. B. (1997). Responses of rainforest understorey plants to excess light during sunflecks. Funct. Plant Biol. 24, 17–25. doi: 10.1071/PP96074
Watson-Parris, D., Christensen, M. W., Laurenson, A., Clewley, D., Gryspeerdt, E., and Stier, P. (2022). Shipping regulations lead to large reduction in cloud perturbations. Proc. Natl. Acad. Sci. 119:e2206885119. doi: 10.1073/pnas.2206885119
Waugh, D. W., Garfinkel, C. I., and Polvani, L. M. (2015). Drivers of the recent tropical expansion in the southern hemisphere: changing SSTs or ozone depletion? J. Clim. 28, 6581–6586. doi: 10.1175/JCLI-D-15-0138.1
Weathers, K. C., Ponette-González, A. G., and Dawson, T. E. (2020). Medium, vector, and connector: fog and the maintenance of ecosystems. Ecosystems 23, 217–229. doi: 10.1007/s10021-019-00388-4
Wielicki, B. A., Cess, R. D., King, M. D., Randall, D. A., and Harrison, E. F. (1995). Mission to planet earth: role of clouds and radiation in climate. Bull. Am. Meteorol. Soc. 76, 2125–2153. doi: 10.1175/1520-0477(1995)076<2125:MTPERO>2.0.CO;2
Williams, J. W., Jackson, S. T., and Kutzbach, J. E. (2007). Projected distributions of novel and disappearing climates by 2100 AD. Proc. Natl. Acad. Sci. 104, 5738–5742. doi: 10.1073/pnas.0606292104
Williams, A. P., Schwartz, R. E., Iacobellis, S., Seager, R., Cook, B. I., Still, C. J., et al. (2015). Urbanization causes increased cloud base height and decreased fog in coastal Southern California. Geophys. Res. Lett. 42, 1527–1536. doi: 10.1002/2015GL063266
Wilson, A. M., and Jetz, W. (2016). Remotely sensed high-resolution global cloud dynamics for predicting ecosystem and biodiversity distributions. PLoS Biol. 14:e1002415. doi: 10.1371/journal.pbio.1002415
Xu, X., Zhang, X., Riley, W. J., Xue, Y., Nobre, C. A., Lovejoy, T. E., et al. (2022). Deforestation triggering irreversible transition in Amazon hydrological cycle. Environ. Res. Lett. 17:034037. doi: 10.1088/1748-9326/ac4c1d
Yamanouchi, T., and Takata, K. (2020). Rapid change of the Arctic climate system and its global influences-overview of GRENE Arctic climate change research project (2011–2016). Pol. Sci. 25:100548. doi: 10.1016/j.polar.2020.100548
Yan, Y. Y. (2005). “Intertropical Convergence Zone (ITCZ)” in Encyclopedia of world climatology. Encyclopedia of earth sciences series. ed. J. E. Oliver (Dordrecht: Springer)
Yan, S., Zhu, B., Huang, Y., Zhu, J., Kang, H., Lu, C., et al. (2020). To what extents do urbanization and air pollution affect fog? Atmos. Chem. Phys. 20, 5559–5572. doi: 10.5194/acp-20-5559-2020
Yang, H., Lohmann, G., Lu, J., Gowan, E. J., Shi, X., Liu, J., et al. (2020). Tropical expansion driven by poleward advancing midlatitude meridional temperature gradients. J. Geophys. Res. Atmos. 125:e2020JD033158. doi: 10.1029/2020JD033158
Ye, Z. P., Ling, Y., Yu, Q., Duan, H. L., Kang, H. J., Huang, G. M., et al. (2020). Quantifying light response of leaf-scale water-use efficiency and its interrelationships with photosynthesis and stomatal conductance in C3 and C4 species. Frontiers in Plant Science, 11, 374. doi: 10.3389/fpls.2020.00374
Young, D. R., and Smith, W. K. (1983). Effect of cloudcover on photosynthesis and transpiration in the subalpine understory species Arnica latifolia. Ecology 64, 681–687. doi: 10.2307/1937189
Zalasky, H. (1976). Frost damage in poplar on the prairies. For. Chron. 52, 61–64. doi: 10.5558/tfc52061-2
Zelinka, M. D., Klein, S. A., and Hartmann, D. L. (2012). Computing and partitioning cloud feedbacks using cloud property histograms. Part II: attribution to changes in cloud amount, altitude, and optical depth. J. Clim. 25, 3736–3754. doi: 10.1175/JCLI-D-11-00249.1
Zelinka, M. D., Myers, T. A., McCoy, D. T., Po-Chedley, S., Caldwell, P. M., Ceppi, P., et al. (2020). Causes of higher climate sensitivity in CMIP6 models. Geophys. Res. Lett. 47:e2019GL085782. doi: 10.1029/2019GL085782
Zhang, Y., Bastos, A., Maignan, F., Goll, D., Boucher, O., Li, L., et al. (2020). Modeling the impacts of diffuse light fraction on photosynthesis in ORCHIDEE (v 5453) land surface model. Geosci. Model Dev. 13, 5401–5423. doi: 10.5194/gmd-13-5401-2020
Zhang, Q., Chen, Y. J., Song, L. Y., Liu, N., Sun, L. L., and Peng, C. L. (2012). Utilization of lightflecks by seedlings of five dominant tree species of different subtropical forest successional stages under low-light growth conditions. Tree Physiol. 32, 545–553. doi: 10.1093/treephys/tps043
Zhang, J. Y., Zhang, Q. H., Shuang, S. P., Cun, Z., Wu, H. M., and Chen, J. W. (2021). The responses of light reaction of photosynthesis to dynamic sunflecks in a typically shade-tolerant species Panax notoginseng. Front. Plant Sci. 12:718981. doi: 10.3389/fpls.2021.718981
Zhou, W., Leung, L. R., Song, F., and Lu, J. (2021). Future changes in the Great Plains low-level jet governed by seasonally dependent pattern changes in the North Atlantic subtropical high. Geophys. Res. Lett. 48:e2020GL090356. doi: 10.1029/2020GL090356
Zhou, W., Xie, S. P., and Yang, D. (2019). Enhanced equatorial warming causes deep-tropical contraction and subtropical monsoon shift. Nat. Clim. Chang. 9, 834–839. doi: 10.1038/s41558-019-0603-9
Zhou, Y. P., Xu, K. M., Sud, Y. C., and Betts, A. K. (2011). Recent trends of the tropical hydrological cycle inferred from global precipitation climatology project and international satellite cloud climatology project data. J. Geophys. Res. Atmos. 116:D09101. doi: 10.1029/2010JD015197
Zohner, C. M., Mirzagholi, L., Renner, S. S., Mo, L., Rebindaine, D., Bucher, R., et al. (2023). Effect of climate warming on the timing of autumn leaf senescence reverses after the summer solstice. Science 381:eadf5098. doi: 10.1126/science.adf5098
Glossary
Keywords: cloud forest, deforestation, drought, fog, global warming, phenology, photosynthesis, productivity
Citation: Hughes NM, Sanchez A, Berry ZC and Smith WK (2024) Clouds and plant ecophysiology: missing links for understanding climate change impacts. Front. For. Glob. Change. 7:1330561. doi: 10.3389/ffgc.2024.1330561
Edited by:
Martin D. Venturas, Universidad Politécnica de Madrid, SpainReviewed by:
Brett Raczka, National Center for Atmospheric Research (UCAR), United StatesJesús Rodríguez-Calcerrada, Polytechnic University of Madrid, Spain
Copyright © 2024 Hughes, Sanchez, Berry and Smith. This is an open-access article distributed under the terms of the Creative Commons Attribution License (CC BY). The use, distribution or reproduction in other forums is permitted, provided the original author(s) and the copyright owner(s) are credited and that the original publication in this journal is cited, in accordance with accepted academic practice. No use, distribution or reproduction is permitted which does not comply with these terms.
*Correspondence: Nicole M. Hughes, bmh1Z2hlc0BoaWdocG9pbnQuZWR1