- 1Mountain Tai Forest Ecosystem Research Station of State Forestry and Grassland Administration, Forestry College, Shandong Agricultural University, Tai’an, Shandong, China
- 2Shandong Academy of Forestry, Jinan, Shandong, China
Introduction: Inputs of additional organic matter to the soil will accelerate or inhibit the decomposition of soil organic carbon (SOC), resulting in a priming effect (PE), which is a key mechanism affecting soil carbon (C) cycling. The impact mechanism of changes in soil properties on the PE is still unclear after vegetation restoration; in particular, the contribution of C pools with different turnover rates to the PE has not been distinguished and quantified.
Methods: In this study, the secondary shrub (SB) (Vitex negundo var. heterophylla) formed by the enclosure of barren grassland was selected as the research object, and the barren grassland (GL) was taken as the control. Equal amounts of 13C-labeled glucose were added to the topsoil for a 45-day incubation experiment to measure the PE. Moreover, soil samples were destructively sampled to explore the fate of new C and changes in POC and MAOC fractions during incubation.
Results: After 45 days of incubation, most of the new C formed by glucose flowed to MAOC, with 95.45% in SB soil and 92.29% in GL soil. In the experiment, all soils showed a positive PE. The PE, POC mineralization and MAOC accumulation were higher in SB soil than in GL soil. During incubation, the mineralization of POC was positively correlated with the PE and made a major contribution to the PE. Partial correlation analysis showed that after vegetation restoration, SB further promoted the mineralization of POC by increasing the soil moisture, fungal diversity and necromass C of bacteria, which led to an increase in PE.
Conclusion: The SB mainly enhanced PE by increasing soil fungal diversity and mineralization of POC. And increasing PE due to the SB may lead to an increase in soil C emissions. Therefore, we need to adopt forest management and other measures to address the potential risks of increased soil C emissions in the vegetation restoration process.
1. Introduction
Soil carbon (C) sequestration was listed by the United Nations as one of the key ways to achieve “carbon neutrality” (IPCC., 2023). Vegetation restoration has been proven to be an effective measure to enhance soil C sequestration capacity (Deng et al., 2013; Yan et al., 2022). However, due to the complex characteristics of soil organic carbon (SOC), the potential mechanism of SOC changes caused by vegetation restoration is still unclear, and there are certain obstacles to predicting global soil C cycling.
In general, SOC content is determined by the balance between its mineralization and sequestration (Bradford et al., 2008; Guidi et al., 2014), and vegetation restoration disrupts the original balance of the soil C pool (Poeplau and Don, 2013). After vegetation restoration, the vegetation cover area and the quantity (thickness) of litter increases, which could lead to moisture retention and humidification (Wang et al., 2011; Cui et al., 2020), resulting in a more stable microbial community structure and larger biomass in the soil (Dong et al., 2021; Cai et al., 2022). However, recent reports have indicated that the proportion of dormant microorganisms will increase with vegetation restoration. The above view holds that vegetation restoration enhances the stability of the soil C pool, resulting in a larger proportion of the highly stable C pool in soil (Gu et al., 2019; Song et al., 2021; Zhang et al., 2022), less available energy and higher C limits to microorganisms (Fontaine et al., 2011; Chen et al., 2019). Dormant microorganisms would be activated, resulting in increased decomposition of SOC (Chen et al., 2018), producing what is known as a positive priming effect (PE) when additional organic matter (OM) is added to the soil. A positive PE could promote SOC mineralization and lead to the loss of soil organic matter (SOM), while a negative PE could inhibit SOC mineralization and thus accumulate SOM (Lyu et al., 2018). After vegetation restoration, the soil is more stable, and the microbial activation potential is greater, which is expected to produce a stronger positive PE (Chen et al., 2019; Zhang et al., 2022). PE is one of the key mechanisms affecting soil C cycling (Guenet et al., 2018; Bastida et al., 2019). It is important to accurately characterize the PE and elucidate the related mechanism of PE change for predicting the change in C storage during vegetation restoration (Guenet et al., 2018; Chen et al., 2019).
The PE is influenced by a variety of biotic and abiotic mechanisms, such as nutrient availability, climate, elevation, soil type, and plant and microbial properties (Bernard et al., 2022). The ease or difficulty of decomposing the C pool determines the differential utilization of SOC fractions by activated microorganisms (Lehmann et al., 2020), which is also the most direct influencing factor on the PE. At present, the most popular classification method of SOC fractions is to divide them into the particulate organic C (POC) and mineral-associated organic C (MAOC) fractions according to their turnover time (Mueller and Koegel-Knabner, 2008; Lavallee et al., 2019). POC is a mixture of partially decomposed plants and their decomposition byproducts, which are easily decomposed by microorganisms and have a short average residence time in soil (Poeplau et al., 2018; Lugato et al., 2021). In contrast, MAOC is mainly composed of organic C derived from byproducts of microbial metabolism or necromass combined with soil minerals (Kögel-Knabner et al., 2008). MAOC is protected by chemical bonds associated with minerals and is a stable fraction with long turnover times (Kleber et al., 2015; Cotrufo et al., 2019). The POC and MAOC fractions together constitute the main body of SOC (Cotrufo et al., 2019). However, the difference in the contribution of the POC and MAOC fractions to the PE has not been effectively distinguished in laboratory experiments with glucose addition. Since the POC and MAOC fractions usually undergo significant changes during vegetation restoration (Trigalet et al., 2016; Hu et al., 2021; Xiang et al., 2022), the impact of vegetation restoration on the above issues also needs to be included in studies.
The value of the PE is the difference in mineralization between soil with and without exogenous OM addition (Kuzyakov et al., 2000; Bernard et al., 2022). The mineralization difference is often traceable according to the main PE theory. The theory of preferential substrate utilization suggests that microorganisms will temporarily stop decomposing macromolecular substances in soil as they prioritize the use of simpler and more easily decomposable exogenous OM (Kuzyakov and Bol, 2006; Blagodatskaya et al., 2007; Bernard et al., 2022). This situation will lead to a negative soil PE, and the mineralization of exogenous OM is the main cause of this part of the mineralization difference. The nitrogen (N) mining hypothesis suggests that activated microorganisms need soil N to provide nutrients after exogenous OM addition (Razanamalala et al., 2018; Bernard et al., 2022). This means that the mineralization difference in the N mining hypothesis is caused by SOC fractions with higher N content, possibly the POC or MAOC in the soil or the newly added exogenous OM. When the N content of exogenous OM is higher, the N mining hypothesis overlaps with the substrate priority utilization theory. At the same time, N addition will lead to soil acidification, which will destroy the MAOC and make it easier for microorganisms to utilize it (Chen et al., 2020; Püspök et al., 2023). Of course, the oxalic acid contained in root exudates will also produce the same result (Keiluweit et al., 2015). In these cases, the mineralization difference is caused more by the mineralization of MAOC. Due to its unstable properties, POC or dissolved organic carbon (DOC) plays a leading role in explaining the mineralization difference in experiments with controlled soil acidification or N addition (Blagodatskaya et al., 2011; Liu et al., 2022). In conclusion, the different properties of exogenous OM and SOC fractions lead to the complexity of the PE mechanism. These mechanisms need to be distinguished on the basis of specific experiments, especially in laboratory incubation experiments where glucose is typically used as an exogenous OM.
Continuous human disturbance has led to the degradation of mountain forests into barren grasslands (Liu et al., 2011), and the barren grasslands will gradually undergo succession to secondary shrubs after enclosure. As the basis for restoring forest vegetation, secondary shrubs are the key vegetation type for secondary succession to proceed to the next stage, especially in areas with relatively harsh ecological environments. Secondary shrubs significantly improve soil quality (Zhang et al., 2015; Cai et al., 2022). Zhang et al. (2015) found that secondary shrubs increased soil C storage in a study of secondary vegetation succession after farmland abandonment in karst areas of Southwest China. In the Loess Plateau region of China, Cai et al. (2022) found that the content of SOC, total nitrogen (TN), and microbial biomass of topsoil increased during the shrub stage of vegetation restoration in abandoned land. However, some studies have also pointed out that secondary shrubs reduced SOC storage (Guidi et al., 2014; Parker et al., 2021). So far, it is uncertain how secondary shrubs affect soil carbon pools, which requires considering the impact of PE.
We took secondary shrubs as the study object, which were formed by the succession of barren grassland after enclosure in the limestone mountains of northern China, and barren grassland without enclosure measures was used as the control. This was done to study the mechanism underlying the influence of secondary shrubs on the PE. Equal amounts of 13C-labeled glucose were added to the topsoil for a 45-day incubation experiment to measure the PE. Moreover, soil samples were destructively sampled to explore the changes in the POC and MAOC fractions during incubation. The objectives of this study were (1) to characterize the PE of secondary shrub and barren grassland and verify whether the PE is affected by vegetation restoration; (2) to quantify the changes in the soil POC and MAOC fractions during 45 days of incubation and trace the fate of exogenous C by labeling it with 13C isotopes; and (3) to explore the relationship between the change in soil properties and the change in PE after barren grassland succession to secondary shrub. Our hypotheses were as follows: (1) Compared to barren grassland soil, secondary shrub soil has more dormant microorganisms and greater potential for microbial activation. The PE will be enhanced after vegetation is restored to secondary shrubs in barren grasslands. (2) POC will be preferentially utilized by activated microorganisms because it is more easily mineralized, and secondary shrub soil will lose more POC during incubation. (3) Soil properties usually affect the utilization of soil C by microorganisms. Under exogenous C input, the differential utilization of the POC and MAOC fractions by microorganisms will mediate the influence of soil property changes on the PE after vegetation restoration.
2. Materials and methods
2.1. Study site and soil sampling
The study site is located in the Dongshan watershed area (E 117°47′, N 35°45′), Xintai city, south-central Shandong Province, China. The Dongshan watershed has a warm, semihumid continental climate. The annual average temperature and precipitation are 13.2°C and 715.2 mm, respectively. This area belongs to the limestone mountainous zone of northern China, with a soil type of Eutriccambisols (FAO/UNESCO System of Soil Classification) and a soil layer thickness of 15–30 cm. The limestone mountain soil layer is thin, and its unique structure is easily prone to soil loss, resulting in drought and barren soil. The study site has experienced human interference, severe vegetation degradation, and poor soil and water scarcity and is distributed with a large area of secondary shrub and barren grassland. The secondary shrub (SB) was formed by enclosing and restoring the barren grassland (GL). At the SB site, the dominant shrub species is Vitex negundo var. heterophylla. The GL is adjacent to the SB site and was not enclosed, and the dominant grasses are Selaginella sinensis (Desv.) Spring and Achnatherum extremiorientale. The SB was selected as the research object, and the GL was taken as the control in this study.
In June 2022, three 20 m × 20 m plots were established at the SB and GL sites. We collected soil samples from the topsoil (0–20 cm) from five random positions in each plot and mixed them as one composite sample. Then, the soils were taken back to the laboratory, where the coarse roots and visible residues were removed with a 2-mm sieve. The soils were used for the measurement of soil properties and for further incubation. The soil properties measured in the experiment are shown in Table 1.
2.2. Experimental design and laboratory incubation
Fresh soil (600 g dry weight equivalent) was weighed into a 2-L airtight plastic container. The soil moisture (SM) was adjusted to 50% of the maximum water capacity by adding deionized water before the experimental incubation. All soil samples were preincubated at 25°C for one week. This was done to activate microorganisms in the soil before the addition of glucose. After preincubation, a 60-g sample of dry soil was weighed, divided into three parts and packed in a 50-mL centrifugal tube. We uniformly added 13C-labeled glucose solution (2 SOC%, 3 atom%) to the divided soil, which could simulate litter input. The glucose-added soils were treated as the treatment group, namely, secondary shrub + glucose (SB + G) and barren grassland + glucose (GL + G). The control group only received an equal amount of deionized water and were termed secondary shrub + water (SB + W) and barren grassland + water (GL + W). After adding the solution, the SM reached 60% of the maximum water capacity.
Three centrifuge tubes containing soil samples, 1 centrifuge tube containing NaOH (20 mL, 2 M), and 1 centrifuge tube containing deionized water were placed into a 1-L black airtight plastic can. Three empty containers containing only NaOH and deionized water were set up to calculate the background carbon dioxide (CO2) of the container. All containers were incubated in an incubator at 25°C for 45 days. The soil samples were continuously supplemented with water during the incubation period. The NaOH in the container was used to trap CO2 released by soil respiration and was replaced at 1, 3, 5, 9, 13, 20, 30, and 45 days of incubation. The replaced NaOH was divided into two parts to measure the 13C abundance and the total amount of trapped CO2. The 13C abundance was determined on an isotope ratio mass spectrometer (Delta V IRMS, Thermo Scientific, Bremen, Germany), and the trapped CO2 was determined by the standard 2 M hydrochloric acid titration.
While collecting the CO2, 48 containers were used to determine the content of SOC fractions during the incubation process. The treatment of the soil samples was consistent with the above incubation experiment. At 1, 5, 13, 30 and 45 days, the soil samples representing all treatments were destructively sampled (the soil samples on Day 45 were taken from the soils after incubation was completed). The soil samples obtained from destructive sampling were separated by wet sieving to determine the content of the POC and MAOC fractions. Overall, a total of 63 incubation containers were set up in the experiment (2 types of vegetation × 2 treatments × 3 repetitions +3 blanks +2 types of vegetation × 2 treatments × 3 repetitions × 4 destructive samples).
2.3. Soil microbial community and necromass carbon analysis
The soil microbial community structure and diversity were sequenced by single-ended sequencing. Primers 338F and 806R were used to amplify the V3-V4 regions of the bacterial 16S rRNA gene. Primers ITS1was used to amplify the fungal ITS region (Zhang et al., 2023).
Glucosamine (GluN), galactosamine (GalN), and muramic acid (MurA) were measured to estimate the necromass carbon of fungi [NC (F)] and the necromass carbon of bacteria [NC (B)]. The specific method was to collect amino sugars from each air-dried soil sample containing more than 0.3 mg of nitrogen, and then the amino sugars were determined by gas chromatography with a GC-FID instrument (Agilent 6890A, USA) equipped with an HP-5 fused silica column. NC (F) and NC (B) were estimated according to the formula provided by Liang et al. (2019):
where NC (F) is the necromass carbon of fungi and NC (B) is the necromass carbon of bacteria. The molecular weights of glucosamine (GluN) and muramic acid (MurA) are 179.2 and 251.2, respectively. A conversion coefficient of 9 was used to convert GluN to NC (F), and a conversion coefficient of 45 was used to convert MurA to NC (B) (Appuhn and Joergensen, 2006; Joergensen, 2018). The units of NC (F), NC (B), MurA and GluN are μg g−1.
2.4. Calculation of the priming effect
The total CO2 emission (Ct) from the soil with glucose addition was derived from the glucose mineralization (Cglu) and native SOC decomposition.
where Ct is the total mineralized amount of soil with glucose addition, and its unit is μg C g−1 soil; V0 is the amount of hydrochloric acid needed to titrate the mineralized content of the control soil, in mL; V1 is the amount of hydrochloric acid needed to titrate the amount of soil mineralization treated by glucose, in mL; C is the concentration of standard hydrochloric acid in mol L−1; 12 is the molar mass of C; 1,000 is the conversion factor; 60 is the dried soil weight; δ13Ct is the δ13 value of the soil with glucose addition; Cglu is the amount of CO2 released by 13C-labeled glucose, and δ13Cglu is the δ13 value of glucose; Cck is the amount of CO2 released by the control soil, δ13Cck is the δ13 value of the control soil; and PE is the priming effect, and the unit is μg C g−1 soil.
2.5. Changes in SOC fractions
The wet sieving method modified by Bradford et al. (2008) was used for soil fractionation. In short, 10 g of air-dried soils was shaken with 30 mL of a sodium hexametaphosphate solution for 18 h to disperse aggregates, rinsed and the POC fraction (>53 μm) and MAOC fraction (<53 μm) were separated with a 53-μm sieve. All SOC fractions were dried before the percentage and content of SOC fractions were analyzed. The average mass recovery of the SB and GL SOC was 88.79 and 88.75%, respectively.
The percentage (f) of glucose-derived C in bulk and SOC fractions was calculated according to a simple isotopic mixing model provided by Liao et al. (2022):
where δ13Ct is the δ13C value of each C fraction in the treatments with glucose addition; δ13Cck is the δ13C value of each C fraction in the control; newly formed POC represents the POC formed from glucose; and newly formed MAOC represents the MAOC formed from glucose.
The POC of PE was used to indicate the change in the POC fraction, and the MAOC of PE was used to indicate the change in the MAOC fraction during incubation. Since the PE is calculated by soil CO2 release, the increase in CO2 release represents the increase in SOC loss, so the calculation formula of the POC of PE and of the MAOC of PE in soil is opposite to that of PE:
where POC of PE is the change in POC during incubation; Ct (POC) is the content of POC in the treatment with glucose addition; and Cck (POC) is the content of POC in the control. MAOC of PE, Ct (MAOC) and Cck (MAOC) were the same as above. The unit of the above factors is μg C g−1 soil.
2.6. Statistical analysis
After data collection, one-way ANOVA was used to compare differences in the soil properties, PE, and change in SOC fractions between SB and GL. Pearson correlation analysis was used to analyze the correlation between the PE and the change in SOC fractions and properties. Then, partial correlation analysis was used to test how soil properties affect the PE through the change in SOC fractions. The relationship between PE and soil properties was explored with the change in SOC fractions as control factors. The greater the difference in the partial correlation coefficient between the zero-order and controlling correlation, the stronger the effect of the factor being controlled. The above analysis was conducted with SPSS 23 software, and the mapping was performed with Origin 2021 software.
3. Results
3.1. Soil properties
According to the characteristics of the soil samples before incubation, the MAOC content in the SB soil (14.51 ± 0.25 mg g−1) was higher than that in the GL soil (13.77 ± 0.30 mg g−1) (p < 0.05, Table 1). In addition, the SM, POC/MAOC, total N to total P ratio (N/P), fungal Shannon and Chao1 indexes, NC (F) and NC (B) in the SB soil were also higher than those in the GL soil (p < 0.05, Table 1).
3.2. Priming effect of SOC
After 45 days of incubation, both the SB and GL soils showed a positive PE, and the PE increased rapidly in the early stage and tended to be stable in the later stage. The SB + G showed a cumulative release of 1308.67 μg C g−1 soil CO2, and the SB + W showed a cumulative release of 940.00 μg C g−1 soil CO2 (Figure 1A). The GL + G showed a cumulative release of 1004.00 μg C g−1 soil CO2, and the GL + W showed a cumulative release of 795.33 μg C g−1 soil CO2 (Figure 1B). The cumulative PE in the SB soil (344.21 μg C g−1 soil) was higher than that in the GL soil (188.25 μg C g−1 soil) within 45 days (p < 0.001) (Figures 1C,D).
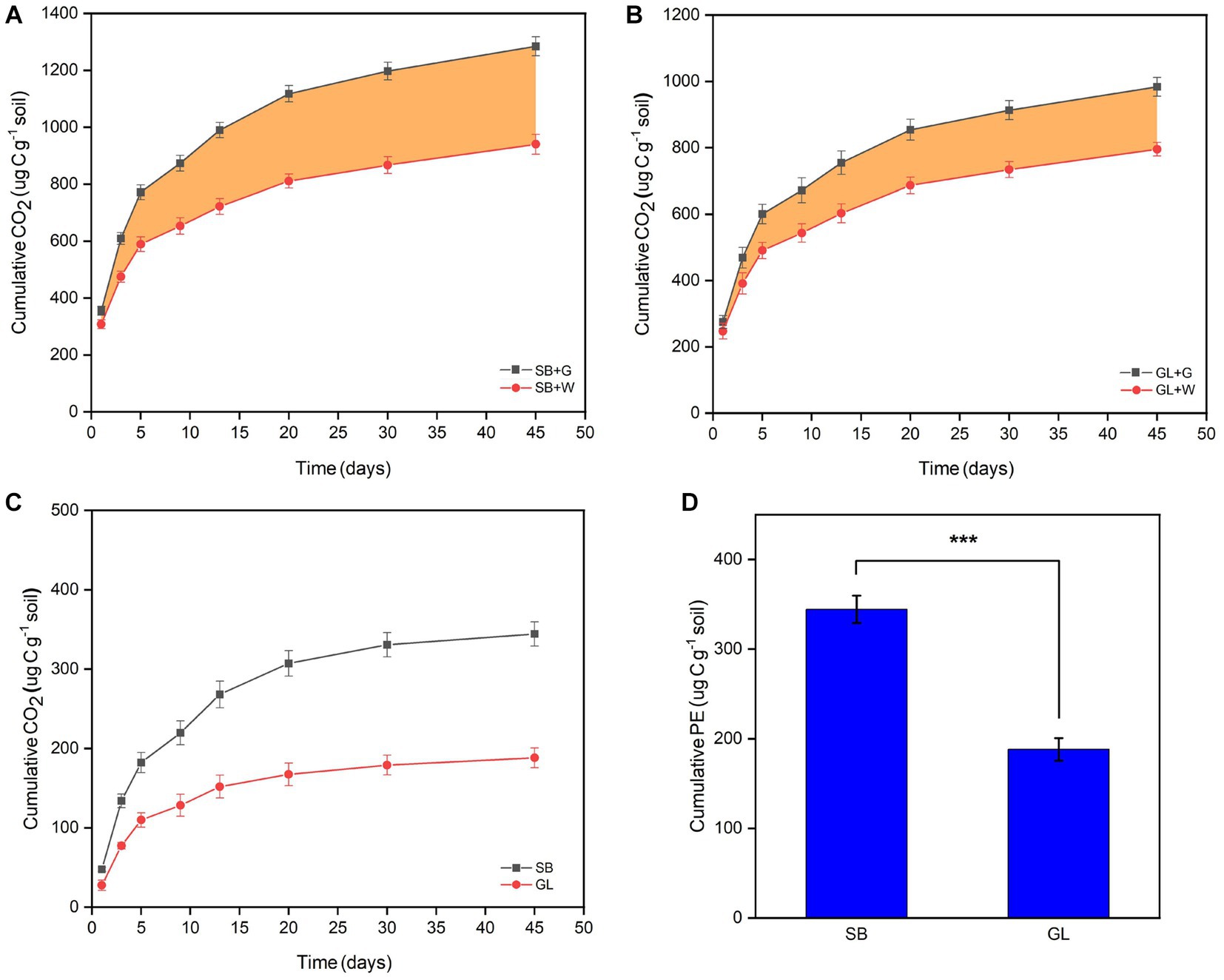
Figure 1. Cumulative CO2 release from soil organic carbon in secondary shrub (A) and barren grassland (B) treated with glucose or distilled water, the shaded portion represents the priming effect amount; Cumulative priming effect amount curve of secondary shrub and barren grassland soil in 45 days (C); Cumulative priming effect amount of secondary shrub and barren grassland soil in 45 days (D); SB: secondary shrub; GL: barren grassland; SB + G: secondary shrub soil with added glucose; SB + W: secondary shrub soil with added distilled water; GL + G: barren grassland soil with added glucose; GL + W: barren grassland soil with added distilled water; PE: priming effect.
3.3. Dynamic changes in the POC and MAOC fractions in soil
The results showed that after 45 days of incubation, the MAOC formed by glucose (82.71 μg C g−1 soil) accounted for 95.45% of the newly formed SOC, and the POC formed by glucose (3.99 μg C g-1 soil) accounted for 4.55% of the newly formed SOC in the SB soil (Figures 2A,C). The MAOC formed by glucose (86.34 μg C g−1 soil) accounted for 92.29% of the newly formed SOC, and the POC formed by glucose (7.21 μg C g−1 soil) accounted for 7.71% of the SOC in the GL soil (Figures 2B,D).
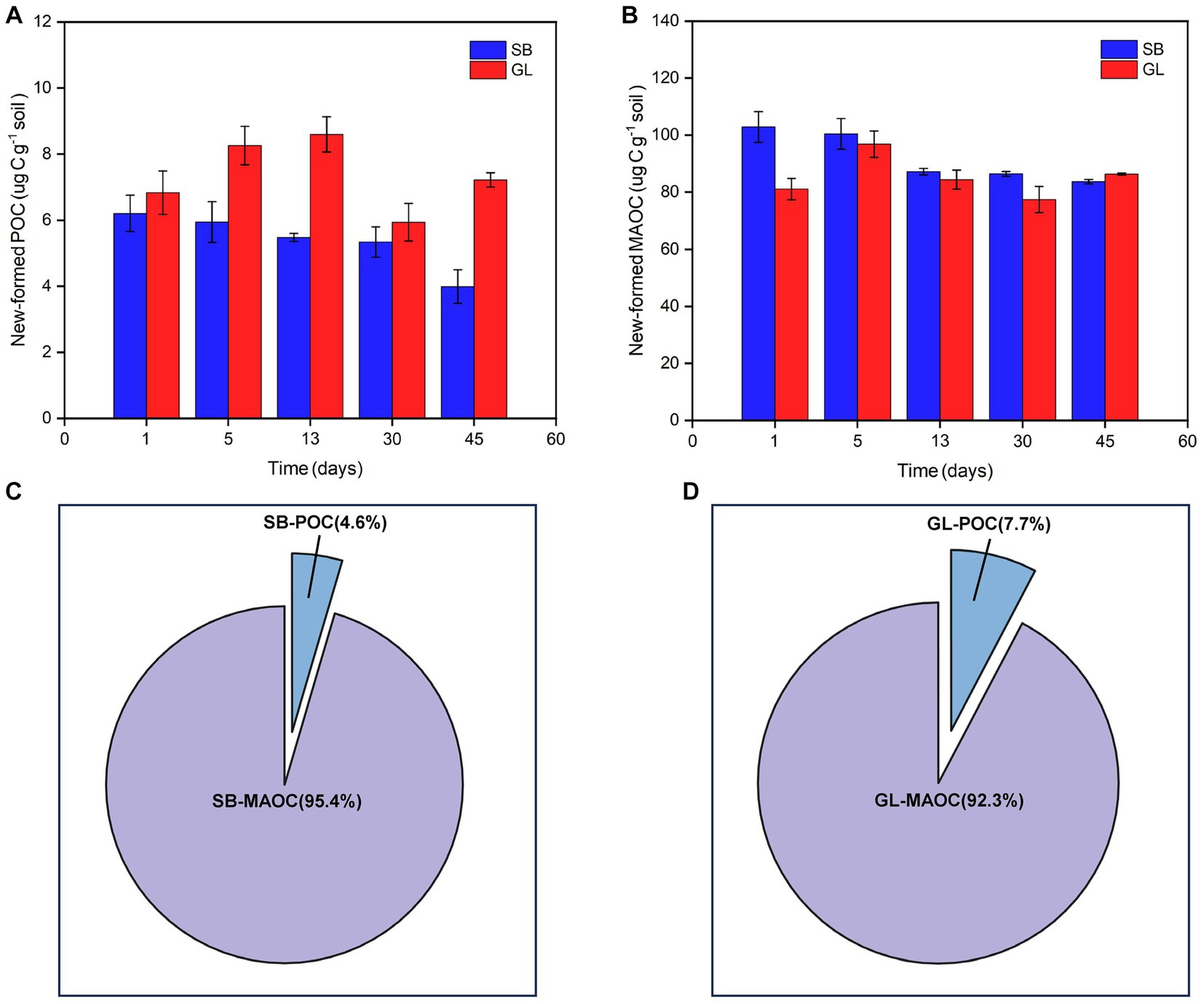
Figure 2. The content of POC (A) and MAOC (B) formed by glucose at various time points. After 45 days, the proportion of POC and MAOC formed by glucose in newly formed SOC in secondary shrubs (C) and grassland (D) soil. POC: particulate organic carbon; MAOC: mineral-associated organic carbon; New-formed POC: the new POC formed by glucose; New-formed MAOC: the new MAOC formed by glucose; SB: secondary shrub; GL: barren grassland; SB-POC: POC in secondary shrub soil; SB-MAOC: MAOC in secondary shrub soil; GL-POC: POC in barren grassland soil; GL-MAOC: MAOC in barren grassland soil.
Similar to the calculation of PE, the changes in the POC and MAOC fractions were obtained by subtracting the SOC fractions of the glucose-treated group from the sum of the SOC fractions formed by glucose and the SOC fractions of the deionized water-treated soil. After the incubation period of 45 days, there was a net loss of POC, and the mean change in POC was 492.54 and 252.21 μg C g−1 soil in the SB and GL soils, respectively. There was a net accumulation of MAOC, and the mean change in MAOC was −214.03 and − 117.75 μg C g−1 soil in the SB and GL soils, respectively. There were significant differences in the POC and MAOC fractions between the SB and GL soils (p < 0.001) (Figures 3C,F).
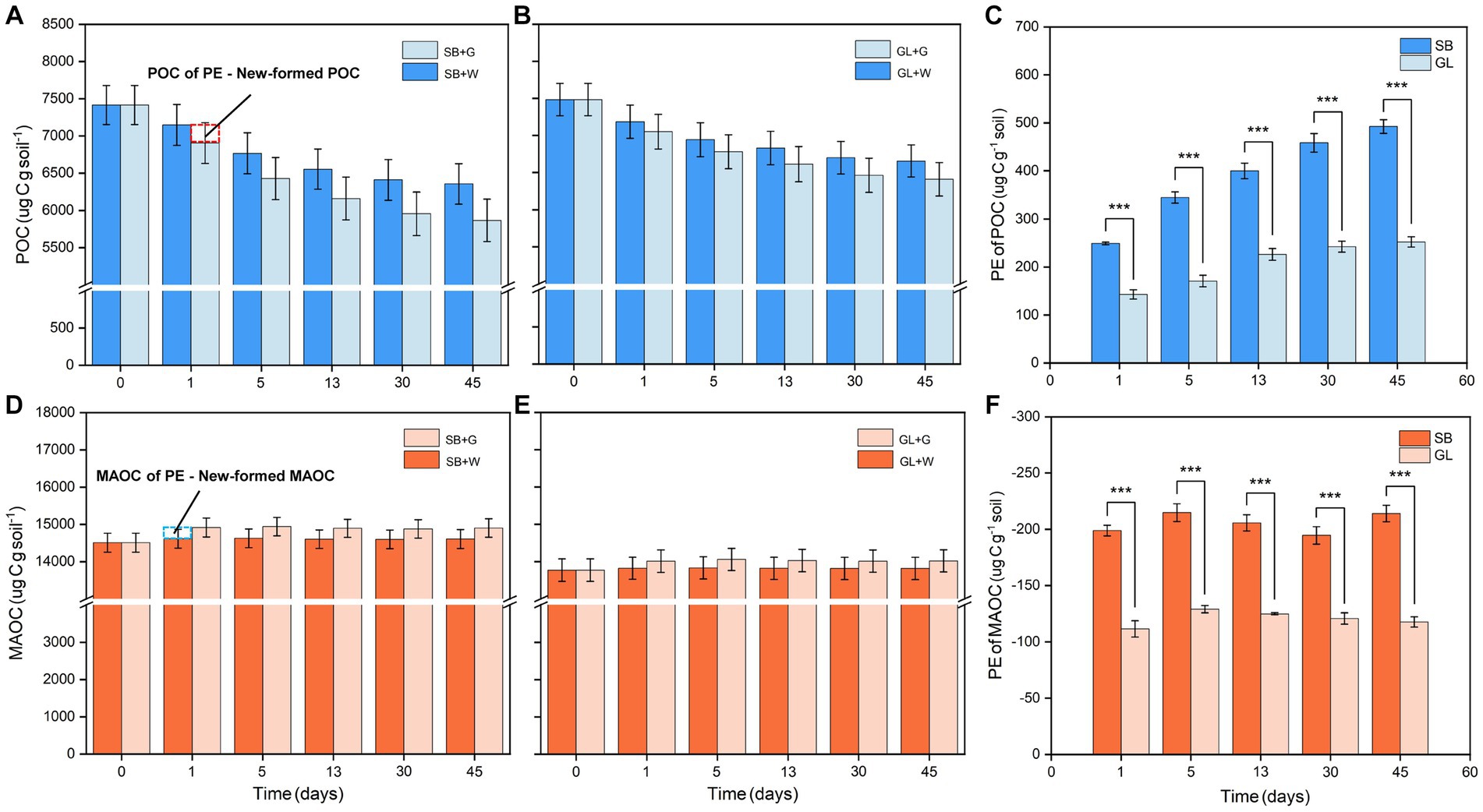
Figure 3. Soil POC content in secondary shrub (A) and barren grassland (B) with glucose or distilled water added at each time point; Soil MAOC content in secondary shrub (D) and barren grassland (E) with glucose or distilled water added at each time point; Change contents of POC (C) and MAOC (F) in secondary shrub and barren grassland soils at each time point; The marked portion indicates the difference of the amount of two carbon fractions between the soil with added distilled water and the soil with added glucose, it also represents the difference between the change of the carbon fractions and the amount of carbon fractions formed from glucose, according to the formula of “POC or MAOC of PE = −[Ct (POC or MAOC) - newly formed POC or MAOC - Cck (POC or MAOC)],” it is calculated that “POC or MAOC of PE - New-formed POC or MAOC = Cck (POC or MAOC) - Ct (POC or MAOC)”; POC: particulate organic carbon; MAOC: mineral-associated organic carbon; New-formed POC: the new POC formed by glucose; New-formed MAOC: the new MAOC formed by glucose; POC of PE: changes of POC in the priming effect; MAOC of PE: changes of MAOC in the priming effect; SB: secondary shrub; GL: barren grassland; SB + G: secondary shrub soil with added glucose; SB + W: secondary shrub soil with added distilled water; GL + G: barren grassland soil with added glucose; GL + W: barren grassland soil with added distilled water; PE: priming effect.
According to the changes in SOC fractions at the five selected destructive sampling time points, more POC of SB was lost than POC of GL (Figures 3A–C). Similarly, more MAOC accumulated in the SB soil than in the GL soil (Figure 3F). The MAOC in both soils showed a tendency to decrease in some periods (Figures 3D–F). The changes in the POC and MAOC fractions in the SB and GL soils gradually slowed over time and finally stabilized (Figures 3C,F).
3.4. Correlation between the priming effect, changes in SOC fractions, and soil properties
The correlation analysis showed that the SM, fungal Shannon and Chao1 indexes, and NC (B) were positively correlated with the PE and the POC of PE. The SM, fungal Shannon and Chao1 indexes, NC (F) and NC (B) were negatively correlated with the newly formed POC, newly formed MAOC and MAOC of PE. The N/P was positively correlated with the POC of PE and negatively correlated with the MAOC of PE (Figure 4A).
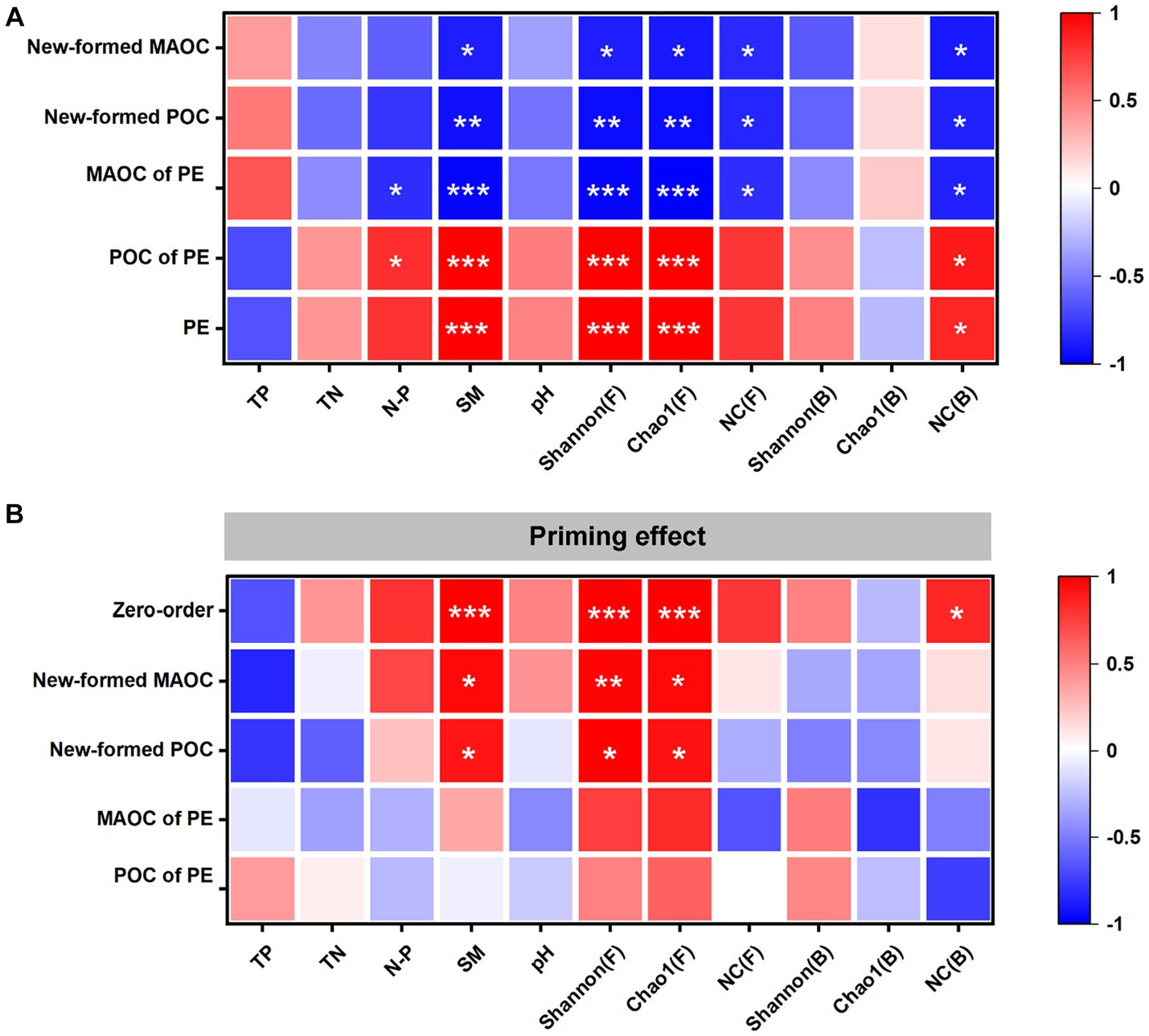
Figure 4. Correlation analysis of the soil properties with the changes in soil organic carbon (SOC) fractions and the cumulative priming effect (PE) (A). Partial correlations between the PE and the soil properties after controlling the changes in SOC fractions (B). The x-axis shows the zero-order (without controlling any factors) and the factors being controlled. The y-axis shows the factors. POC: particulate organic carbon; MAOC: mineral-associated organic carbon; POC of PE: changes of POC in the priming effect; MAOC of PE: changes of MAOC in the priming effect; New-formed POC: the new POC formed by glucose; New-formed MAOC: the new MAOC formed by glucose; TP: total phosphorus; TN: total nitrogen; N/P: total nitrogen to total phosphorus ratio; SM: Soil moisture content; Shannon(F): Shannon index of fungi; Chao1(F): Chao1 index of fungi; NC(F): Necromass carbon of fungi; Shannon(B): Shannon index of bacteria; Chao1(B): Chao1 index of bacteria; NC(B): Necromass carbon of bacteria. *p < 0.05, **p < 0.01 and ***p < 0.001.
Through further partial correlation analysis, it was found that the SM, fungal Shannon and Chao1 indexes, and NC (B) were positively correlated with the PE without controlling for any factors. The SM, NC (B), and fungal Shannon and Chao1 indexes had no significant correlation with the PE when the POC of PE and MAOC of PE were controlled. NC (B) had no significant correlation with PE when the newly formed POC and newly formed MAOC were controlled (Figure 4B).
4. Discussion
4.1. Distribution of exogenous carbon in SOC fractions
After incubation, only a small part of the exogenous C was mineralized into CO2, and the rest was mostly left in the soil. We added equal amounts of 13C-labeled glucose to the soil and then measured the δ13C values of the POC and MAOC fractions at each destructive sampling time point over a 45-day period. In this way, the fate and content of exogenous C in SOC fractions could be determined. It was found that most of the new C formed by glucose in the SB and GL soils flowed to MAOC after incubation. This C could account for more than 90% of the total SOC formed by glucose (Figures 2C,D), which was similar to the results of earlier studies (Cotrufo et al., 2015; Liao et al., 2022). Liao et al. (2022) studied the PE of coniferous and broadleaf forests and found that most of the C formed by glucose was distributed in MAOC after incubation, with 90.8% in coniferous forest soil and 83.2% in broadleaf forest soil. Cotrufo et al. (2015) added labeled litter to soil to track its fate and found that the proportion of stable SOC formed by litter decomposition reached as high as 94%. The fate of glucose could be traced due to the easily decomposable properties of glucose and the formation pathway of MAOC. Part of the glucose, which provided energy for microbial growth and reproduction, was processed into small molecules and fixed in MAOC (Lehmann and Kleber, 2015; Sokol and Bradford, 2018). At the same time, some glucose and NC of microbes were also combined with soil minerals to form MAOC (Liang et al., 2017; Chen et al., 2020; Cotrufo et al., 2022).
Unexpectedly, after removing the mineralized glucose (19.77 ~ 20.22 μg C g−1 soil), the glucose remaining in the POC and MAOC fractions (86.52 ~ 93.77 μg C g−1 soil) also accounted for only a small portion of the total added glucose (500 μg C g−1 soil). The experimental data of Liao et al. (2022) also revealed a similar situation to ours, and their results showed that after the soil was separated by wet sieving, the newly formed SOC (300 ~ 350 μg C g−1 soil) accounted for approximately a quarter of the total amount of glucose added (1,300 ~ 1,600 μg C g−1 soil). There seemed to be a threshold for soil fixation of exogenous C during the production of the PE. We speculated that this threshold was related to the following mechanism: the utilization of exogenous C by soil microorganisms will have a saturation value, which was probably limited by nutrients such as N (Castellano et al., 2015; Angst et al., 2023). When the utilization of exogenous C by soil microorganisms reached the saturation value, the remaining glucose existed in the soil in a separate form and then dissolved together with DOC during the wet sieving process. Whether the threshold is related to the type or content of exogenous OM is still unclear, and further experiments need to be designed to explore their relationship.
4.2. Effects of secondary shrub on the priming effect and SOC fraction mineralization
The results of the PE experiment confirmed the first hypothesis; that is, the PE of soil was enhanced after vegetation restoration in the form of secondary shrub. Specifically, there were more dormant microorganisms in the SB than in the GL, and the former had greater microbial activation potential. More activated microorganisms mean a greater potential for soil C mineralization under exogenous OM input. From the above viewpoint, it could be concluded that the PE of SB is stronger than that of GL. By measuring the background values of soil properties before incubation, we found that the fungal community diversity of the SB soil was higher, which supported the above result to a certain extent (Table 1). In addition, a number of studies on PE have shown that increased microbial biomass is an important indicator of enhanced PE (Kuzyakov, 2010; Wang et al., 2014; Feng et al., 2021), which was similar to our findings.
We confirmed our second hypothesis that activated microorganisms preferentially used POC and that the SB soil lost more POC during incubation. In general, with increasing incubation time, there was a net loss of POC and a net accumulation of MAOC in the soil. Especially after the input of exogenous OM, the soil lost more POC while also accumulating more MAOC. Moreover, the SB soil lost more POC and accumulated more MAOC than the GL soil in the glucose-added treatment group and in the control group. The cometabolism of soil original C and exogenous OM mineralized by microorganisms may be the main reason for the net loss of POC (Yu et al., 2018; Perveen et al., 2019). POC is more easily mineralized by microorganisms (Rocci et al., 2021), so it is the SOC fraction preferentially utilized by microorganisms during incubation, which explained the increased POC loss after the input of exogenous OM. Interestingly, our results indicated that the decrease in POC was accompanied by the accumulation of MAOC, so the net accumulation of MAOC was likely to come from POC. Some previous studies support our view, and the conversion of POC to MAOC has been reported during the SOC mineralization process (Samson et al., 2020; Vidal et al., 2021). In addition, the view that POC is considered a precursor to the formation of MAOC could also support our results (Lehmann and Kleber, 2015; Angst et al., 2023).
SB could promote POC mineralization, which was the reason why the PE of the SB soil was stronger than that of the GL soil. The experimental results also indicated that the mineralization of soil POC and the cumulative PE showed a highly consistent trend of change during the 45 days of incubation. Although the sum of cumulative PE and the net accumulation of MAOC was greater than the net mineralization of POC, this could be explained by the fact that there was also a certain amount of decomposition of the MAOC and DOC fractions during this process. For example, the NC of microbes in MAOC was often reused by microorganisms (Liang et al., 2019; Cui et al., 2020), and this view was supported by a slight decrease in newly formed MAOC in some periods during incubation (Figure 2B). In addition, when Blagodatskaya et al. (2011) studied the changes in DOC in a PE experiment, they found that the amount of DOC gradually decreased with incubation, which was significantly positively correlated with a positive PE. In summary, SB could enhance the PE, which was mainly caused by the increased mineralization of POC. Therefore, there was a risk of exogenous OM input leading to a decrease in soil C sequestration capacity during vegetation restoration. Some studies have found that soil C sequestration capacity was unchanged or decreased in the early stage of vegetation restoration (Hartley et al., 2012; Zhao et al., 2023), and our conclusion may be one reason for these results.
4.3. Mechanism by which secondary shrub enhances the priming effect
Many studies have indicated that vegetation restoration will lead to changes in soil properties (Jiao et al., 2011; Shaoxuan et al., 2016), which may be the main reason that SB enhanced the PE. The results of this study indicated that the change in the PE was related to the change in the SM, fungal diversity and NC of bacteria (Figure 4A). Moreover, the results of partial correlation analysis indicated that the above factors were related to the PE through the amount of POC mineralization during incubation (Figure 4B). These results suggested that the changes in soil properties caused by SB enhanced the PE by increasing POC mineralization, which supported the third hypothesis.
The soil microorganisms were an important factor influencing the PE (Whitaker et al., 2014; Yu et al., 2020; Zhang et al., 2022). In this study, the increase in fungal diversity played a key role in enhancing the PE of SB compared with bacterial diversity (Figure 4B). In general, fungi were more efficient than bacteria in utilizing refractory compounds (Bernard et al., 2022), such as cellulose, lignocellulose and chitin (Fernandez and Kennedy, 2015). After vegetation restoration, woody litter usually comprised the main part of the SB soil, so the diversity of the fungal community involved in the decomposition of refractory compounds was larger in the SB soil.
Secondary shrub could increase the accumulation of litter on the surface, which can capture and trap rainfall, and stronger water retention will increase the biomass and activity of soil microorganisms (Wang et al., 2011; Cui et al., 2020). At the same time, changes in vegetation cover types can also affect microbial biomass by changing the content of soil nutrients, which is a key mechanism affecting the PE in many studies (Chen et al., 2014; Zhang F. et al., 2022). In terms of our research results, although N/P to some extent stimulated the mineralization of POC (Figure 4A), it did not affect the PE. In this study, SM was the most important factor affecting microbial biomass compared to soil nutrients. The higher NC (F) and NC (B) in the SB soil confirmed this conclusion (Table 1) because more NC of microbes meant more microbial biomass in the soil (Wang et al., 2021). More microbial biomass meant that there were more dormant microbes in the SB soil because the energy restriction in the SB soil was more severe (Joergensen and Wichern, 2018). After adding exogenous OM to the SB soil, more dormant microorganisms were activated, leading to more POC mineralization in the soil. Similarly, Liu et al. (2022) found that enhanced microbial activity reduced the POC of topsoil by studying the changes in SOC fractions after frozen soil thawing on the Tibetan Plateau. In addition, Zhang F. et al. (2022) found that microbial biomass was positively correlated with PE, which could also support our view. In general, the role of fungi was greater than that of bacteria in the mechanism of PE enhancement by changing the microbial diversity in the SB during vegetation restoration. Because the positive feedback of PE on increased fungal diversity may lead to a potential risk of increased soil C emissions, it is necessary to focus on assessing changes in soil fungal diversity during vegetation restoration. A deeper understanding of the relationship between soil microbes and PE will help us to reduce soil greenhouse gas emissions through forest management and other measures during vegetation restoration.
5. Conclusion
In summary, the results of this study indicated that SB enhanced the PE, which was mainly driven by the mineralization of different SOC fractions and changes in environmental factors. According to the incubation experiment, the increase in POC mineralization in SB soil was the main reason for its enhanced PE. From the perspective of environmental factors, the change in microbial diversity was the main driving factor enhancing the PE of SB, and the effect of fungi was greater than that of bacteria. Because the SB enhanced the potential risks of increased soil C emissions through the PE, it is necessary to focus on the dynamics of additional C input during vegetation restoration. In addition, further exploring the relevant mechanisms for enhancing the PE of SB soil, especially its microbial mechanisms, will help deepen our understanding of the relationship between vegetation restoration and the soil C pool.
Data availability statement
The original contributions presented in the study are included in the article/supplementary material, further inquiries can be directed to the corresponding author.
Author contributions
QY: Conceptualization, Data curation, Formal analysis, Investigation, Methodology, Software, Validation, Visualization, Writing – original draft, Writing – review & editing. ZZ: Formal analysis, Investigation, Methodology, Supervision, Validation, Visualization, Writing – original draft, Writing – review & editing. YH: Investigation, Writing – original draft, Writing – review & editing. MH: Writing – original draft, Writing – review & editing. GW: Writing – original draft. XD: Resources, Supervision, Writing – review & editing. QW: Methodology, Supervision, Validation, Writing – review & editing. PG: Funding acquisition, Methodology, Project administration, Resources, Supervision, Visualization, Writing – review & editing.
Funding
The author(s) declare financial support was received for the research, authorship, and/or publication of this article. Demonstration and Promotion of Forest Biological Fire Blocking Forest Belt Construction Technology (no. LU [2023] TG008) and Mount Tai Forest Ecosystem National Positioning Observation Research Station Monitoring Subsidy Project (no. 2018-LYPT-DW-053).
Conflict of interest
The authors declare that the research was conducted in the absence of any commercial or financial relationships that could be construed as a potential conflict of interest.
Publisher’s note
All claims expressed in this article are solely those of the authors and do not necessarily represent those of their affiliated organizations, or those of the publisher, the editors and the reviewers. Any product that may be evaluated in this article, or claim that may be made by its manufacturer, is not guaranteed or endorsed by the publisher.
Abbreviations
SOC, Soil organic carbon; PE, Priming effect; POC, Particulate organic carbon; MAOC, Mineral-associated organic carbon; SB, Secondary shrub; GL, Barren grassland; OM, Organic matter; DOC, Dissolved organic carbon; SM, Soil moisture; NC, Necromass carbon.
References
Angst, G., Mueller, K. E., Castellano, M. J., Vogel, C., Wiesmeier, M., and Mueller, C. W. (2023). Unlocking complex soil systems as carbon sinks: multi-pool management as the key. Nat. Commun. 14, 1–8. doi: 10.1038/s41467-023-38700-5
Appuhn, A., and Joergensen, R. (2006). Microbial colonisation of roots as a function of plant species. Soil Biol. Biochem. 38, 1040–1051. doi: 10.1016/j.soilbio.2005.09.002
Bastida, F., García, C., Fierer, N., Eldridge, D. J., Bowker, M. A., Abades, S., et al. (2019). Global ecological predictors of the soil priming effect. Nat. Commun. 10:3481. doi: 10.1038/s41467-019-11472-7
Bernard, L., Basile-Doelsch, I., Derrien, D., Fanin, N., Fontaine, S., Guenet, B., et al. (2022). Advancing the mechanistic understanding of the priming effect on soil organic matter mineralisation. Funct. Ecol. 36, 1355–1377. doi: 10.1111/1365-2435.14038
Blagodatskaya, E. V., Blagodatsky, S. A., Anderson, T. H., and Kuzyakov, Y. (2007). Priming effects in Chernozem induced by glucose and N in relation to microbial growth strategies. Appl. Soil Ecol. 37, 95–105. doi: 10.1016/j.apsoil.2007.05.002
Blagodatskaya, E., Yuyukina, T., Blagodatsky, S., and Kuzyakov, Y. (2011). Three-source-partitioning of microbial biomass and of CO2 efflux from soil to evaluate mechanisms of priming effects. Soil Biol. Biochem. 43, 778–786. doi: 10.1016/j.soilbio.2010.12.011
Bradford, M. A., Fierer, N., and Reynolds, J. F. (2008). Soil carbon stocks in experimental mesocosms are dependent on the rate of labile carbon, nitrogen and phosphorus inputs to soils. Funct. Ecol. 22, 964–974. doi: 10.1111/j.1365-2435.2008.01404.x
Cai, X., Zhang, D., Wang, Y., Diao, L., Cheng, X., Luo, Y., et al. (2022). Shift in soil microbial communities along ~160 years of natural vegetation restoration on the loess plateau of China. Appl. Soil Ecol. 173:104394. doi: 10.1016/j.apsoil.2022.104394
Castellano, M. J., Mueller, K. E., Olk, D. C., Sawyer, J. E., and Six, J. (2015). Integrating plant litter quality, soil organic matter stabilization, and the carbon saturation concept. Glob. Chang. Biol. 21, 3200–3209. doi: 10.1111/gcb.12982
Chen, L., Liu, L., Mao, C., Qin, S., Wang, J., Liu, F., et al. (2018). Nitrogen availability regulates topsoil carbon dynamics after permafrost thaw by altering microbial metabolic efficiency. Nat. Commun. 9:3951. doi: 10.1038/s41467-018-06232-y
Chen, L., Liu, L., Qin, S., Yang, G., Fang, K., Zhu, B., et al. (2019). Regulation of priming effect by soil organic matter stability over a broad geographic scale. Nat. Commun. 10:5112. doi: 10.1038/s41467-019-13119-z
Chen, R., Senbayram, M., Blagodatsky, S., Myachina, O., Dittert, K., Lin, X., et al. (2014). Soil C and N availability determine the priming effect: microbial N mining and stoichiometric decomposition theories. Glob. Chang. Biol. 20, 2356–2367. doi: 10.1111/gcb.12475
Chen, J., Xiao, W., Zheng, C., and Zhu, B. (2020). Nitrogen addition has contrasting effects on particulate and mineral-associated soil organic carbon in a subtropical forest. Soil Biol. Biochem. :142. doi: 10.1016/j.soilbio.2020.107708
Cotrufo, M. F., Haddix, M. L., Kroeger, M. E., and Stewart, C. E. (2022). The role of plant input physical-chemical properties, and microbial and soil chemical diversity on the formation of particulate and mineral-associated organic matter. Soil Biol. Biochem. :168. doi: 10.1016/j.soilbio.2022.108648
Cotrufo, M. F., Ranalli, M. G., Haddix, M. L., Six, J., and Lugato, E. (2019). Soil carbon storage informed by particulate and mineral-associated organic matter. Nat. Geosci. 12, 989–994. doi: 10.1038/s41561-019-0484-6
Cotrufo, M. F., Soong, J. L., Horton, A. J., Campbell, E. E., Haddix, M. L., Wall, D. H., et al. (2015). Formation of soil organic matter via biochemical and physical pathways of litter mass loss. Nat. Geosci. 8, 776–779. doi: 10.1038/ngeo2520
Cui, Y., Wang, X., Zhang, X., Ju, W., Duan, C., Guo, X., et al. (2020). Soil moisture mediates microbial carbon and phosphorus metabolism during vegetation succession in a semiarid region. Soil Biol. Biochem. 147:107814. doi: 10.1016/j.soilbio.2020.107814
Cui, J., Zhu, Z., Xu, X., Liu, S., Jones, D. L., Kuzyakov, Y., et al. (2020). Carbon and nitrogen recycling from microbial necromass to cope with C:N stoichiometric imbalance by priming. Soil Biol. Biochem. 142:107720. doi: 10.1016/j.soilbio.2020.107720
Deng, L., Wang, K.-B., Chen, M.-L., Shangguan, Z.-P., and Sweeney, S. (2013). Soil organic carbon storage capacity positively related to forest succession on the loess plateau, China. Catena 110, 1–7. doi: 10.1016/j.catena.2013.06.016
Dong, X., Gao, P., Zhou, R., Li, C., Dun, X., and Niu, X. (2021). Changing characteristics and influencing factors of the soil microbial community during litter decomposition in a mixed Quercus acutissima Carruth. And Robinia pseudoacacia L. forest in Northern China. Catena :196. doi: 10.1016/j.catena.2020.104811
Feng, J., Tang, M., and Zhu, B. (2021). Soil priming effect and its responses to nutrient addition along a tropical forest elevation gradient. Glob. Chang. Biol. 27, 2793–2806. doi: 10.1111/gcb.15587
Fernandez, C. W., and Kennedy, P. G. (2015). Revisiting the ‘Gadgil effect’: do interguild fungal interactions control carbon cycling in forest soils? New Phytol. 209, 1382–1394. doi: 10.1111/nph.13648
Fontaine, S., Henault, C., Aamor, A., Bdioui, N., Bloor, J. M. G., Maire, V., et al. (2011). Fungi mediate long term sequestration of carbon and nitrogen in soil through their priming effect. Soil Biol. Biochem. 43, 86–96. doi: 10.1016/j.soilbio.2010.09.017
Gu, X., Fang, X., Xiang, W., Zeng, Y., Zhang, S., Lei, P., et al. (2019). Vegetation restoration stimulates soil carbon sequestration and stabilization in a subtropical area of southern China. Catena 181:104098. doi: 10.1016/j.catena.2019.104098
Guenet, B., Camino-Serrano, M., Ciais, P., Tifafi, M., Maignan, F., Soong, J. L., et al. (2018). Impact of priming on global soil carbon stocks. Glob. Chang. Biol. 24, 1873–1883. doi: 10.1111/gcb.14069
Guidi, C., Vesterdal, L., Gianelle, D., and Rodeghiero, M. (2014). Changes in soil organic carbon and nitrogen following forest expansion on grassland in the southern Alps. For. Ecol. Manag. 328, 103–116. doi: 10.1016/j.foreco.2014.05.025
Hartley, I. P., Garnett, M. H., Sommerkorn, M., Hopkins, D. W., Fletcher, B. J., Sloan, V. L., et al. (2012). A potential loss of carbon associated with greater plant growth in the European Arctic. Nat. Clim. Chang. 2, 875–879. doi: 10.1038/nclimate1575
Hu, P., Zhang, W., Chen, H., Li, D., Zhao, Y., Zhao, J., et al. (2021). Soil carbon accumulation with increasing temperature under both managed and natural vegetation restoration in calcareous soils. Sci. Total Environ. 767:145298. doi: 10.1016/j.scitotenv.2021.145298
IPCC (2023) in Climate change 2023: Synthesis report. Contribution of working groups I, II and III to the sixth assessment report of the intergovernmental panel on climate change. eds. H. Lee and J. Romero (Geneva, Switzerland: IPCC), 35–115.
Jiao, F., Wen, Z.-M., and An, S.-S. (2011). Changes in soil properties across a chronosequence of vegetation restoration on the loess plateau of China. Catena 86, 110–116. doi: 10.1016/j.catena.2011.03.001
Joergensen, R. G. (2018). Amino sugars as specific indices for fungal and bacterial residues in soil. Biol. Fertil. Soils 54, 559–568. doi: 10.1007/s00374-018-1288-3
Joergensen, R. G., and Wichern, F. (2018). Alive and kicking: why dormant soil microorganisms matter. Soil Biol. Biochem. 116, 419–430. doi: 10.1016/j.soilbio.2017.10.022
Keiluweit, M., Bougoure, J. J., Nico, P. S., Pett-Ridge, J., Weber, P. K., and Kleber, M. (2015). Mineral protection of soil carbon counteracted by root exudates. Nat. Clim. Chang. 5, 588–595. doi: 10.1038/nclimate2580
Kleber, M., Eusterhues, K., Keiluweit, M., Mikutta, C., Mikutta, R., and Nico, P. S. (2015). Mineral–organic associations: formation, properties, and relevance in soil environment. Adv. Agron. 130, 1–140. doi: 10.1016/bs.agron.2014.10.005
Kögel-Knabner, I., Guggenberger, G., Kleber, M., Kandeler, E., Kalbitz, K., Scheu, S., et al. (2008). Organo-mineral associations in temperate soils: integrating biology, mineralogy, and organic matter chemistry. J. Plant Nutr. Soil Sci. 171, 61–82. doi: 10.1002/jpln.200700048
Kuzyakov, Y. (2010). Priming effects: interactions between living and dead organic matter. Soil Biol. Biochem. 42, 1363–1371. doi: 10.1016/j.soilbio.2010.04.003
Kuzyakov, Y., and Bol, R. (2006). Sources and mechanisms of priming effect induced in two grassland soils amended with slurry and sugar. Soil Biol. Biochem. 38, 747–758. doi: 10.1016/j.soilbio.2005.06.025
Kuzyakov, Y., Friedel, J. K., Stahr, K. K., Friedel, J. K., and Stahr, K. (2000). Review of mechanisms and quantification of priming effects. Soil Biol. Biochem. 32, 32, 1485, 1485–1498.
Lavallee, J. M., Soong, J. L., and Cotrufo, M. F. (2019). Conceptualizing soil organic matter into particulate and mineral-associated forms to address global change in the 21st century. Glob. Chang. Biol. 26, 261–273. doi: 10.1111/gcb.14859
Lehmann, J., Hansel, C. M., Kaiser, C., Kleber, M., Maher, K., Manzoni, S., et al. (2020). Persistence of soil organic carbon caused by functional complexity. Nat. Geosci. 13, 529–534. doi: 10.1038/s41561-020-0612-3
Lehmann, J., and Kleber, M. (2015). The contentious nature of soil organic matter. Nature 528, 60–68. doi: 10.1038/nature16069
Liang, C., Amelung, W., Lehmann, J., and Kästner, M. (2019). Quantitative assessment of microbial necromass contribution to soil organic matter. Glob. Chang. Biol. 25, 3578–3590. doi: 10.1111/gcb.14781
Liang, C., Schimel, J. P., and Jastrow, J. D. (2017). The importance of anabolism in microbial control over soil carbon storage. Nat. Microbiol. 2, 1–6. doi: 10.1038/nmicrobiol.2017.105
Liao, C., Men, X., Wang, C., Chen, R., and Cheng, X. (2022). Nitrogen availability and mineral particles contributed fungal necromass to the newly formed stable carbon pool in the alpine areas of Southwest China. Soil Biol. Biochem. :173. doi: 10.1016/j.soilbio.2022.108788
Liu, F., Qin, S., Fang, K., Chen, L., Peng, Y., Smith, P., et al. (2022). Divergent changes in particulate and mineral-associated organic carbon upon permafrost thaw. Nat. Commun. 13, 1–10. doi: 10.1038/s41467-022-32681-7
Liu, X., Zhang, W., Liu, Z., Qu, F., and Tang, X. (2011). Changes in species diversity and above-ground biomass of shrubland over long-term natural restoration process in the Taihang Mountain in North China. Plant Soil Environ. 57, 505–512. doi: 10.17221/216/2011-pse
Lugato, E., Lavallee, J. M., Haddix, M. L., Panagos, P., and Cotrufo, M. F. (2021). Different climate sensitivity of particulate and mineral-associated soil organic matter. Nat. Geosci. 14, 295–300. doi: 10.1038/s41561-021-00744-x
Lyu, M., Xie, J., Vadeboncoeur, M. A., Wang, M., Qiu, X., Ren, Y., et al. (2018). Simulated leaf litter addition causes opposite priming effects on natural forest and plantation soils. Biol. Fertil. Soils 54, 925–934. doi: 10.1007/s00374-018-1314-5
Mueller, C. W., and Koegel-Knabner, I. (2008). Soil organic carbon stocks, distribution, and composition affected by historic land use changes on adjacent sites. Biol. Fertil. Soils 45, 347–359. doi: 10.1007/s00374-008-0336-9
Parker, T. C., Thurston, A. M., Raundrup, K., Subke, J.-A., Wookey, P. A., and Hartley, I. P. (2021). Shrub expansion in the Arctic may induce large-scale carbon losses due to changes in plant-soil interactions. Plant Soil 463, 643–651. doi: 10.1007/s11104-021-04919-8
Perveen, N., Barot, S., Maire, V., Cotrufo, M. F., Shahzad, T., Blagodatskaya, E., et al. (2019). Universality of priming effect: An analysis using thirty five soils with contrasted properties sampled from five continents. Soil Biol. Biochem. 134, 162–171. doi: 10.1016/j.soilbio.2019.03.027
Poeplau, C., and Don, A. (2013). Sensitivity of soil organic carbon stocks and fractions to different land-use changes across Europe. Geoderma 192, 189–201. doi: 10.1016/j.geoderma.2012.08.003
Poeplau, C., Don, A., Six, J., Kaiser, M., Benbi, D., Chenu, C., et al. (2018). Isolating organic carbon fractions with varying turnover rates in temperate agricultural soils – a comprehensive method comparison. Soil Biol. Biochem. 125, 10–26. doi: 10.1016/j.soilbio.2018.06.025
Püspök, J. F., Zhao, S., Calma, A. D., Vourlitis, G. L., Allison, S. D., Aronson, E. L., et al. (2023). Effects of experimental nitrogen deposition on soil organic carbon storage in Southern California drylands. Glob. Chang. Biol. 29, 1660–1679. doi: 10.1111/gcb.16563
Razanamalala, K., Razafimbelo, T., Maron, P.-A., Ranjard, L., Chemidlin, N., Lelièvre, M., et al. (2018). Soil microbial diversity drives the priming effect along climate gradients: a case study in Madagascar. ISME J. 12, 451–462. doi: 10.1038/ismej.2017.178
Rocci, K. S., Lavallee, J. M., Stewart, C. E., and Cotrufo, M. F. (2021). Soil organic carbon response to global environmental change depends on its distribution between mineral-associated and particulate organic matter: a meta-analysis. Sci. Total Environ. 793:148569. doi: 10.1016/j.scitotenv.2021.148569
Samson, M.-É., Chantigny, M. H., Vanasse, A., Menasseri-Aubry, S., and Angers, D. A. (2020). Coarse mineral-associated organic matter is a pivotal fraction for SOM formation and is sensitive to the quality of organic inputs. Soil Biol. Biochem. :149. doi: 10.1016/j.soilbio.2020.107935
Shaoxuan, H., Zongsuo, L., Ruilian, H., Yong, W., and Guobin, L. (2016). Soil carbon dynamics during grass restoration on abandoned sloping cropland in the hilly area of the loess plateau, China. Catena 137, 679–685. doi: 10.1016/j.catena.2015.01.027
Sokol, N. W., and Bradford, M. A. (2018). Microbial formation of stable soil carbon is more efficient from belowground than aboveground input. Nat. Geosci. 12, 46–53. doi: 10.1038/s41561-018-0258-6
Song, Y., Zhai, J., Zhang, J., Qiao, L., Wang, G., Ma, L., et al. (2021). Forest management practices of Pinus tabulaeformis plantations alter soil organic carbon stability by adjusting microbial characteristics on the loess plateau of China. Sci. Total Environ. 766, 1–8. doi: 10.1016/j.scitotenv.2020.144209
Trigalet, S., Gabarrón-Galeote, M. A., Van Oost, K., and van Wesemael, B. (2016). Changes in soil organic carbon pools along a chronosequence of land abandonment in southern Spain. Geoderma 268, 14–21. doi: 10.1016/j.geoderma.2016.01.014
Vidal, A., Klöffel, T., Guigue, J., Angst, G., Steffens, M., Hoeschen, C., et al. (2021). Visualizing the transfer of organic matter from decaying plant residues to soil mineral surfaces controlled by microorganisms. Soil Biol. Biochem. :160. doi: 10.1016/j.soilbio.2021.108347
Wang, B., Liang, C., Yao, H., Yang, E., and An, S. (2021). The accumulation of microbial necromass carbon from litter to mineral soil and its contribution to soil organic carbon sequestration. Catena 207:105622. doi: 10.1016/j.catena.2021.105622
Wang, Y., Shao, M. A., Zhu, Y., and Liu, Z. (2011). Impacts of land use and plant characteristics on dried soil layers in different climatic regions on the loess plateau of China. Agric. For. Meteorol. 151, 437–448. doi: 10.1016/j.agrformet.2010.11.016
Wang, Q., Wang, S., He, T., Liu, L., and Wu, J. (2014). Response of organic carbon mineralization and microbial community to leaf litter and nutrient additions in subtropical forest soils. Soil Biol. Biochem. 71, 13–20. doi: 10.1016/j.soilbio.2014.01.004
Whitaker, J., Ostle, N., McNamara, N. P., Nottingham, A. T., Stott, A. W., Bardgett, R. D., et al. (2014). Microbial carbon mineralization in tropical lowland and montane forest soils of Peru. Front. Microbiol. 5:720. doi: 10.3389/fmicb.2014.00720
Xiang, H., Luo, X., Zhang, L., Hou, E., Li, J., Zhu, Q., et al. (2022). Forest succession accelerates soil carbon accumulation by increasing recalcitrant carbon stock in subtropical forest topsoils. Catena 212:106030. doi: 10.1016/j.catena.2022.106030
Yan, W., Zhou, Q., Peng, D., Luo, Y., Chen, M., and Lu, Y. (2022). Response of surface-soil quality to secondary succession in karst areas in Southwest China: case study on a limestone slope. Ecol. Eng. 178:106581. doi: 10.1016/j.ecoleng.2022.106581
Yu, Z., Chen, L., Pan, S., Li, Y., Kuzyakov, Y., Xu, J., et al. (2018). Feedstock determines biochar-induced soil priming effects by stimulating the activity of specific microorganisms. Eur. J. Soil Sci. 69, 521–534. doi: 10.1111/ejss.12542
Yu, G., Zhao, H., Chen, J., Zhang, T., Cai, Z., Zhou, G., et al. (2020). Soil microbial community dynamics mediate the priming effects caused by in situ decomposition of fresh plant residues. Sci. Total Environ. 737:139708. doi: 10.1016/j.scitotenv.2020.139708
Zhang, D., Cai, X., Diao, L., Wang, Y., Wang, J., An, S., et al. (2022). Changes in soil organic carbon and nitrogen pool sizes, dynamics, and biochemical stability during ∼160 years natural vegetation restoration on the loess plateau, China. Catena 211:106014. doi: 10.1016/j.catena.2021.106014
Zhang, F., Chen, X., Wang, Q., Zhang, Y., Yao, S., and Zhang, B. (2022). The priming effect dynamics are driven by microbial activation and growth and constrained by the relative availability of input C and soil N. Biol. Fertil. Soils 58, 745–760. doi: 10.1007/s00374-022-01658-5
Zhang, Q., Feng, J., Li, J., Huang, C. Y., Shen, Y., Cheng, W., et al. (2022). A distinct sensitivity to the priming effect between labile and stable soil organic carbon. New Phytol. 237, 88–99. doi: 10.1111/nph.18458
Zhang, Z., Hao, M., Yu, Q., Dun, X., Xu, J., and Gao, P. (2023). The effect of thinning intensity on the soil carbon pool mediated by soil microbial communities and necromass carbon in coastal zone protected forests. Sci. Total Environ. :881. doi: 10.1016/j.scitotenv.2023.163492
Zhang, W., Zhao, J., Pan, F., Li, D., Chen, H., and Wang, K. (2015). Changes in nitrogen and phosphorus limitation during secondary succession in a karst region in Southwest China. Plant Soil 391, 77–91. doi: 10.1007/s11104-015-2406-8
Keywords: vegetation restoration, soil organic carbon, mineral-associated organic carbon, particulate organic carbon, fungal diversity
Citation: Yu Q, Zhang Z, He Y, Hao M, Wang G, Dun X, Wu Q and Gao P (2023) Secondary shrubs promoted the priming effect by increasing soil particle organic carbon mineralization. Front. For. Glob. Change. 6:1288259. doi: 10.3389/ffgc.2023.1288259
Edited by:
Xuebo Zheng, Chinese Academy of Agricultural Sciences, ChinaReviewed by:
Kailou Liu, Jiangxi Institute of Red Soil, ChinaPing Cong, Chinese Academy of Agricultural Sciences, China
Copyright © 2023 Yu, Zhang, He, Hao, Wang, Dun, Wu and Gao. This is an open-access article distributed under the terms of the Creative Commons Attribution License (CC BY). The use, distribution or reproduction in other forums is permitted, provided the original author(s) and the copyright owner(s) are credited and that the original publication in this journal is cited, in accordance with accepted academic practice. No use, distribution or reproduction is permitted which does not comply with these terms.
*Correspondence: Xingjian Dun, ZHhpbmdqaWFuQDE2My5jb20=; Qicong Wu, cWN3dUBzZGF1LmVkdS5jbg==; Peng Gao, Z2FvcGVuZ3lAMTYzLmNvbQ==
†This author have contributed equally to this work and share first authorship