- Ontario Forest Research Institute, Ontario Ministry of Natural Resources, and Forestry, Sault Ste. Marie, ON, Canada
The effects of daylength on bud dormancy release varied throughout the dormant period in black spruce (Picea mariana (Mill.) B.S.P.) seedlings. In phase one of this trial, seedlings exposed to 8 h photoperiods to induce terminal buds were then intermittently transferred to 12, 16, 18 or 24 h photoperiods to determine dormancy status, evaluated by observing rates of terminal budburst. Buds were in a state of endodormancy initially after short-day induction, as indicated by their inability to quickly break bud when placed in longer daylengths. The time required for budburst decreased as time after bud initiation increased. Time to terminal budburst also decreased linearly with longer photoperiods. In phase two of the investigation, beginning 9 weeks after terminal bud initiation, seedlings began receiving chilling at +5°C to promote the transition from endo- to ecodormancy. During chilling, groups of seedlings were periodically removed from the cold and placed into warm temperatures at 8, 12, 16, 18 or 24 h photoperiods to observe the rate of budburst. Time to budburst in an 8 h photoperiod decreased logarithmically with the duration of chilling, decreasing more rapidly over the first four weeks of chilling and thereafter more slowly. Photoperiod always affected the rates of budburst: with eight weeks of chilling, longer photoperiods resulted in faster rates of budburst; in contrast, after 16 weeks of chilling, budburst was faster in shorter photoperiods. These results are of practical significance in tree nurseries where controlling bud dormancy release is important. In addition, this trial provides empirical observations of bud dormancy release that contribute to the understanding of environmental control of this aspect of the tree developmental cycle, which may affect tree phenology as the climate changes.
1 Introduction
Factors controlling bud dormancy in tree species are of interest due to the potential changes in timing of budburst that may be induced by warming climates. In addition, species translocations that move more southerly species northward to latitudes with different daylengths across the growing season also have the potential to affect budburst (Casmey et al., 2022). In temperate regions where cold temperatures are sufficient to fulfill the chilling requirement, warmer temperatures in the spring cause budburst to happen sooner (Singh et al., 2017). On the other hand, if there is a substantial shortfall in cold exposure over the winter to break dormancy, spring budburst can be delayed (Fu et al., 2015). Decreased wintertime cold temperatures and increased springtime warm temperatures not only can change the dormancy status of buds, but also have the potential to induce ecodormancy at different photoperiods, which can itself affect dormancy release (Fu et al., 2019). The responses of trees to these factors and their interactions can be complex and there is a need for experimental observations for many species to better understand how climate change may affect tree growth (Hänninen et al., 2019).
Buds pass through a series of physiological conditions that control the timing of growth resumption in the spring. After buds form, they require an external stimulus to pass from a state of physiological dormancy (termed endodormancy or rest) to a condition where buds remain dormant because of environmental conditions (ecodormancy or quiescence). In temperate regions, the external dormancy release stimulus is usually provided by cold temperatures. In trees that are adapted to their climatic environment, sufficient chilling generally is received to put trees into an ecodormant state by the time temperatures begin warming in the spring, although year-to-year variation in spring temperatures lead to earlier or later budbreak (Menzel et al., 2001; Polgar and Primack, 2011). It is also well known that the rate of budburst in response to warm temperatures varies with the amount of chilling received (e.g., Dennis, 2003). There is concern that warmer winters may delay the resumption of shoot elongation in the spring because chilling requirements have not fully met, reducing the environmental fitness of trees (Heide, 1993; Hänninen and Tanino, 2011; Polgar and Primack, 2011). Photoperiod can interact with winter chilling and spring warming to affect the date on which budburst happens, with exposure to either shorter daylengths if seedlings achieve ecodormancy earlier or longer daylengths if ecodormancy is delayed due to incomplete chilling (Fu et al., 2019). While it is theoretically true that photoperiod can interact with climate warming to affect rates of budburst (Polgar and Primack, 2011), limited evidence is available to show that appreciable photoperiodic effects on budburst timing will occur in many species, while budburst substantially varies from year-to-year depending on the occurrence of warm temperatures. This study provides evidence of the potential effects of photoperiod on budburst timing with climate warming for black spruce.
Typically, when non-dormant shoots of young perennial woody plants receive a bud induction stimulus, changes occur in plant hormones, carbohydrates and phytochrome (Chao et al., 2007). These changes alter plant phenology, most noticeably by the formation of budscales instead of leaves at the terminal shoot apices, which in most conifer species are at first identifiable by the formation of living white budscales (cf. Templeton et al., 1993). As these first budscales mature their tissues die and turn brown, after which buds remain largely unchanged visually through the rest of their dormancy cycle. Despite their unvarying appearance, conifer buds at first continue to produce additional budscales and, at a certain point in their development, budscale formation ceases and the shoot tip forms an embryonic shoot, consisting of needle primordia surrounding an apical meristem. Throughout the period during which buds are present, the shoot apex passes through changes in genetic, biochemical and physiological factors (e.g., Faust et al., 1997; Rinne et al., 1997; Horvath, 2010; Cooke et al., 2012) that eventually lead to the growth of the enclosed embryonic shoot in the spring.
Many studies of bud dormancy have examined buds that are transitioning from endodormancy to ecodormancy, and those studies predominantly examine either how chilling promotes this dormancy phase transition and/or the response to warm temperatures that leads to budburst and the resumption of shoot elongation. In the present study, not only is the effect of chilling on the endodormancy-ecodormancy transition evaluated, but also the comparatively less well understood changes in dormancy around the time of bud initiation as buds are first entering endodormancy. These evaluations are made by examining budburst in response to daylength and chilling using a model system of uniformly grown, similarly sized and physiologically alike first-year black spruce (Picea mariana (Mill.) B.S.P.) seedlings.
2 Materials and methods
Seeds of a northeastern Ontario black spruce seed source were sown in early July. The resulting seedlings were grown in a greenhouse in Maple, Ontario, with greenhouse temperatures of 20 to 25°C, both day and night. Natural light was extended to 18 h using high-pressure sodium lamps (approximately 70 μmoles m−2 s−1 PPFD).
In mid-November, daylength was reduced to 8 h using manually applied blackout curtains between 1,600 and 0800 h. Temperatures during both day and night ranged between 20 to 25°C. The blackout curtains remained closed on weekends, at which time light was provided between 0800 and 1,600 h only by high-pressure sodium lamps. Twice-weekly destructive examination of 20 seedlings began when the 8 h photoperiod treatment commenced. For these seedlings, the vegetative apex of the primary shoots was dissected and examined under a dissecting microscope to determine whether budscales had formed. The bi-weekly destructive sampling continued until 100% of the seedlings in a sample were observed to have terminal buds, which occurred three weeks after beginning the 8 h photoperiod.
Phase I of the experiment (examining budburst at different daylengths but without chilling, during bud morphogenesis and the development of endodormancy) began at the time of 100% bud initiation. At that time, 20 seedlings were transferred from the 8 h glasshouse cubicle to each of four other glasshouse cubicles with daylengths of 12, 16, 18 or 24 h. Further transfers of 20 seedlings from the 8 h cubicle to cubicles with other daylengths occurred over the following nine weeks after bud initiation. Specifically, the 16 h and 24 h cubicles each received 20 seedlings on weeks 1, 2, 4 and 9. And, on weeks 4 and 9, 20 seedlings were also transferred from the 8 h cubicle to both the 12 and 18 h cubicles. A summary of the treatment combinations used in Phase I is shown in Table 1. On weekdays, curtains were opened daily in all glasshouse cubicles to provide eight hours of natural sunlight (0800 to 1,600 h). Air temperatures in all cubicles ranged between 20 and 25°C both day and night, with temperatures under blackout more likely to be toward the higher end of this range during sunny days. Daylengths in the photoperiod treatments were extended an equal number of hours before 0800 h and after 1,600 h to give the required number of hours of artificial lighting from high-pressure sodium lamps. Seedlings in each photoperiod treatment were examined non-destructively twice weekly to assess budburst of the main stem terminal bud. Budburst was the point at which bud scales had parted and enlarging needles emerged from the bud were oriented perpendicular to the stem (similar to stage 5 of budburst in Rossi and Bousquet (2014)).
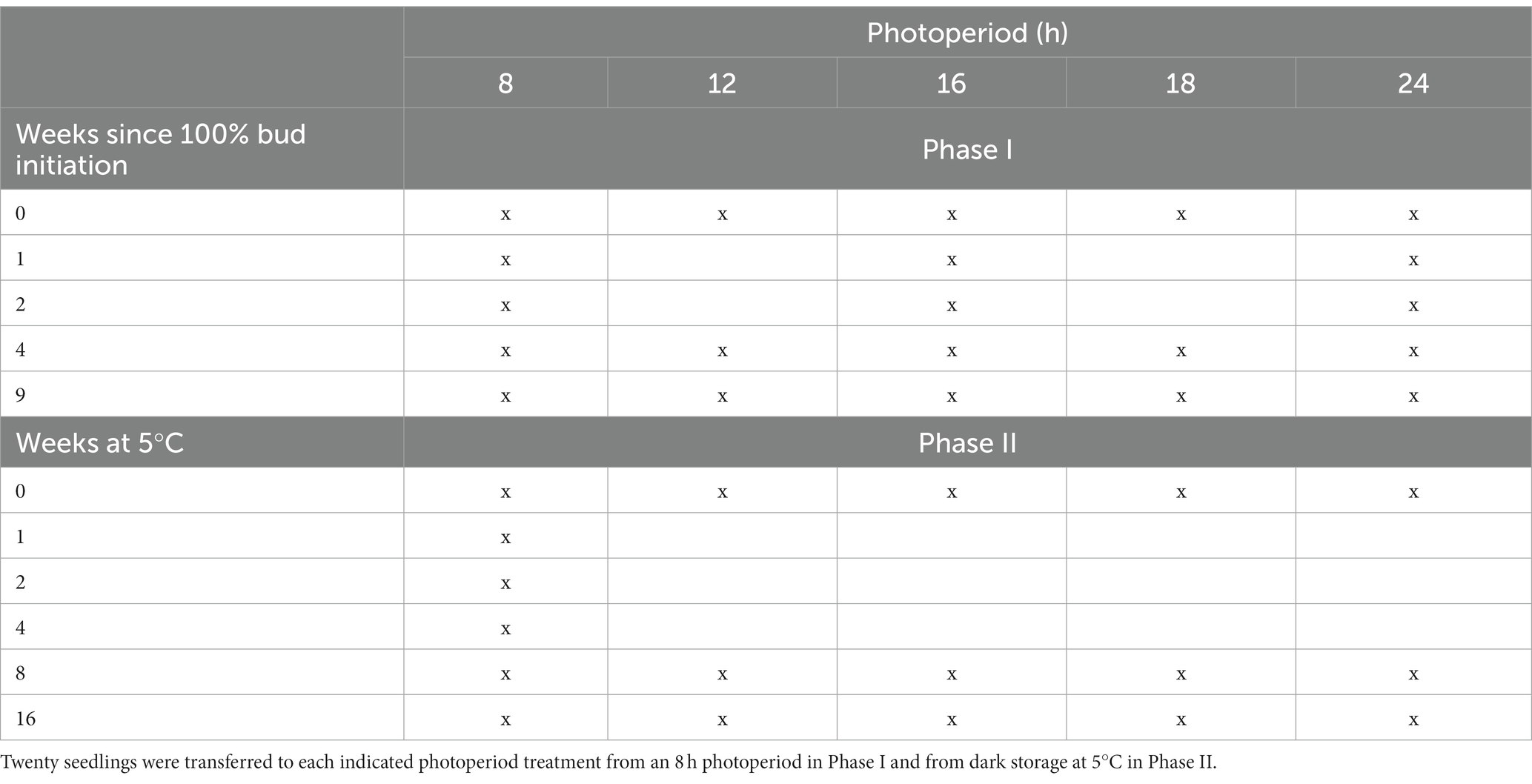
Table 1. Summary of treatment combinations used in Phase I (during the development of endodormancy) and in Phase II (during the transition from endodormancy to ecodormancy).
Phase II of the experiment (budburst at different daylengths after the completion of bud morphogenesis and during the chilling-induced transition from endodormancy to ecodormancy) began nine weeks after 100% bud initiation. At that time, most of the trays of seedlings in the 8 h glasshouse were placed in large plastic bags and moved to a + 5°C dark chamber (except for twenty seedlings that remained in the 8 h glasshouse to determine time to budburst without chilling at an 8 h daylength). (Thus, week 9 in Phase I is the same as 0 weeks of chilling in Phase II). The effects of the chilling x photoperiod interaction on bud dormancy release were observed by evaluating time to budburst for seedlings removed from cold storage and placed into some combinations of 8, 12, 16, 18 or 24 h photoperiods after 1, 2, 4, 8 or 16 weeks, with 20 seedlings in each photoperiod x cold storage duration combination used. The treatment combinations used in Phase II are shown in Table 1. Day and night temperatures were in all cases between 20 and 25°C.
Statistical assessment of photoperiod and chilling effects on bud dormancy was by means of regression analysis using SigmaPlot software (version 10).
3 Results
In Phase I of the experiment, the effect of photoperiod on rate of budburst differed with time since bud initiation (Figure 1). While time to budburst decreased linearly with longer photoperiods (r2 was 0.94 on week 0, 0.97 on week 4 and 0.82 on week 9), the differences among photoperiods in time to budburst diminished with the time progression since bud initiation (Figure 2). For example, on week 0, the point at which all seedlings had recently initiated buds, the difference in days to budburst between seedlings in an 8 h versus a 24 h daylength was about 74 days, while on weeks 4 and 9, the differences were about 50 and 22 days, respectively. Trees that remained in an 8 h photoperiod at warm temperatures eventually broke bud given sufficient time, requiring on average 94 days (Figures 2, 8 h treatment).
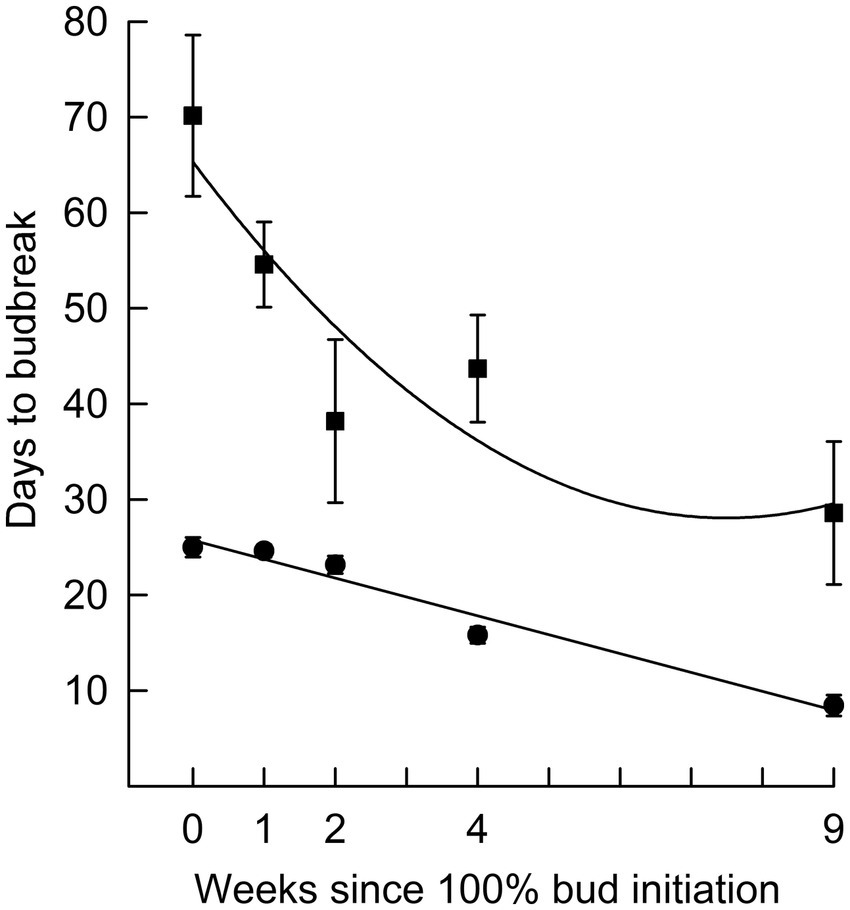
Figure 1. Average and standard error of the number of days to terminal budburst for black spruce seedlings in 16 h (■; r2 = 0.82) and 24 h (●; r2 = 0.96) photoperiods over time after bud initiation. Error bars for 24 h are mostly hidden by symbols.
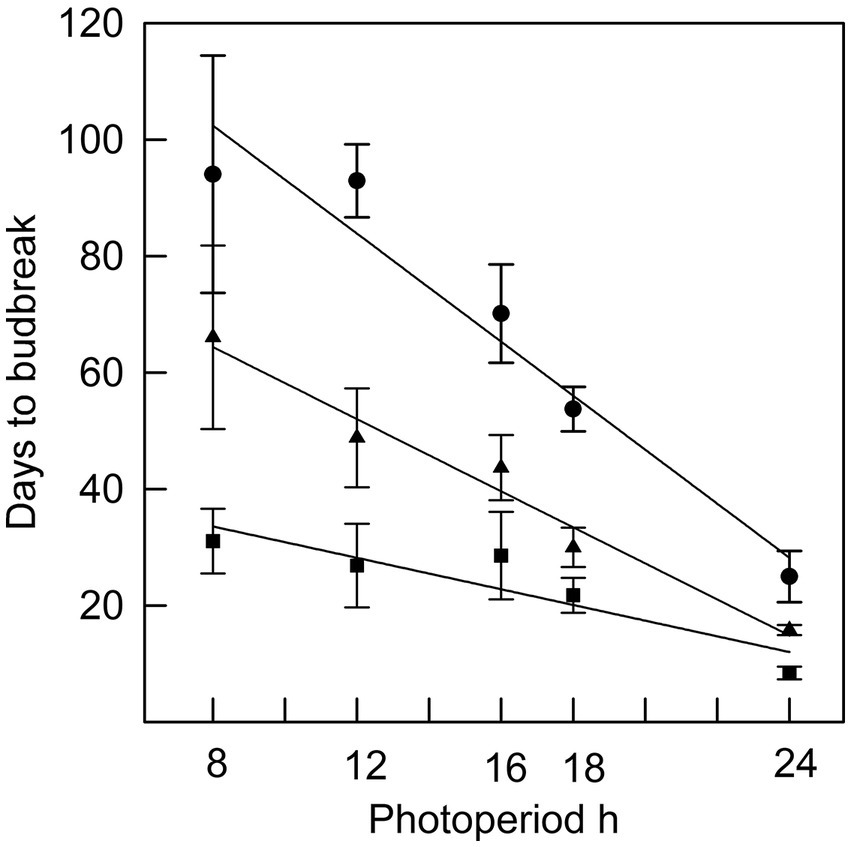
Figure 2. The relationship between photoperiod and days to bud break for seedlings placed in different photoperiods at the time of bud initiation (●; r2 = 0.94), four weeks after bud initiation (▲; r2 = 0.97) or nine weeks after bud initiation (■; r2 = 0.82). Error bars are standard errors.
In Phase II of the experiment, the effect of chilling on reducing the time to budburst was strongest in the first four weeks. This is clearly shown in Figure 3, in which budburst in an 8 h photoperiod is displayed: the time to budburst decreased from an average of 31 days with no chilling to about nine days after four weeks of chilling, with 12 more weeks of chilling reducing time to budburst only by about four additional days. Daylength influenced the rate of budburst regardless of the amount of chilling, although the photoperiodic effect was diminished with 4 or more weeks of chilling (comparing the result for trees 9 weeks after bud initiation in Figure 2, which shows the photoperiodic effect with no chilling versus Figure 4, indicating a reduced photoperiodic effect with eight weeks of chilling). After eight weeks of chilling there was a significant linear trend of faster budburst in longer photoperiods (Figure 4). However, with 16 weeks of chilling, the trend was reversed, with a significant linear trend of slower budburst at longer photoperiods (Figure 5). An apparently anomalous result with 16 weeks of chilling for the 12 h photoperiod reduced the slope of the relationship between photoperiod and rate of budbreak (dotted line in Figure 5) but not the overall pattern of slower budbreak with longer photoperiods (r2 = 0.93 without and 0.52 with the 12 h photoperiod, respectively). Tables of goodness of fit and significance of regression equations are shown for all regressions in Supplementary Table S1.
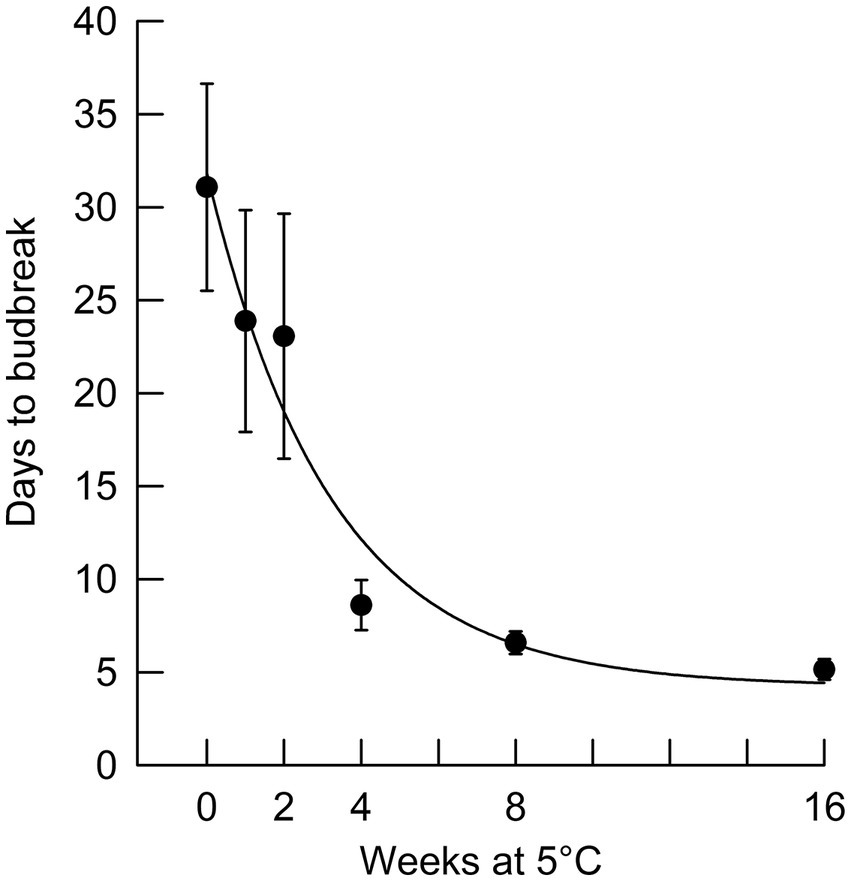
Figure 3. Number of days to terminal budburst (with standard error bars) for black spruce seedlings removed from chilling at different times and placed in a growing environment with an 8 h photoperiod (r2 = 0.95).
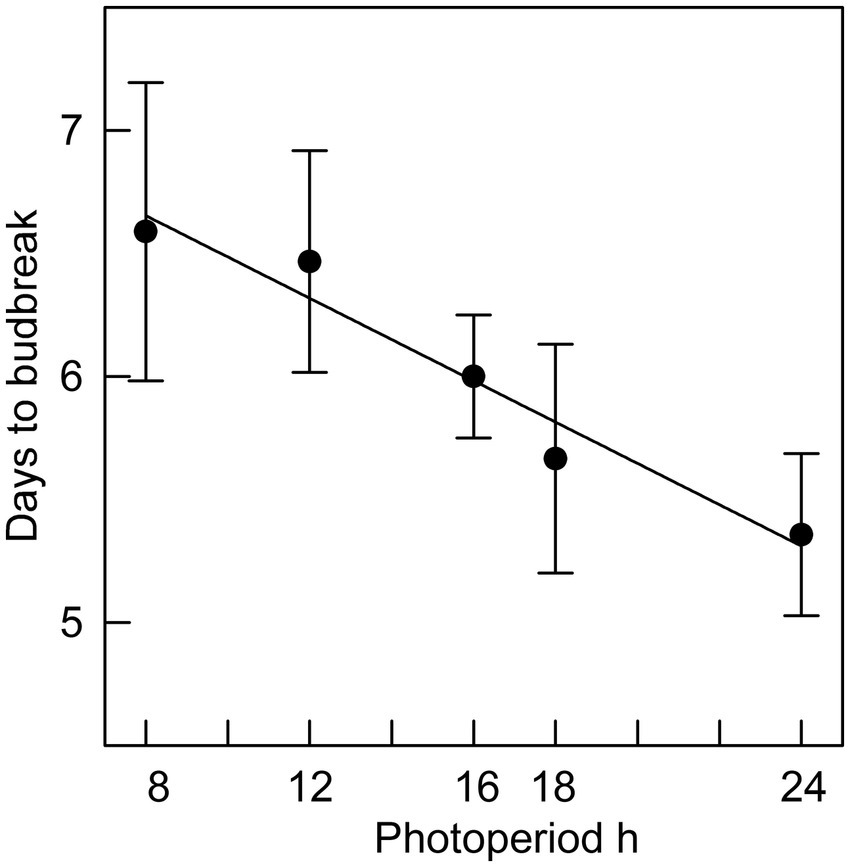
Figure 4. The relationship between photoperiod and days to bud break for seedlings placed in different photoperiods after 8 weeks of chilling (r2 = 0.95; error bars are standard errors).
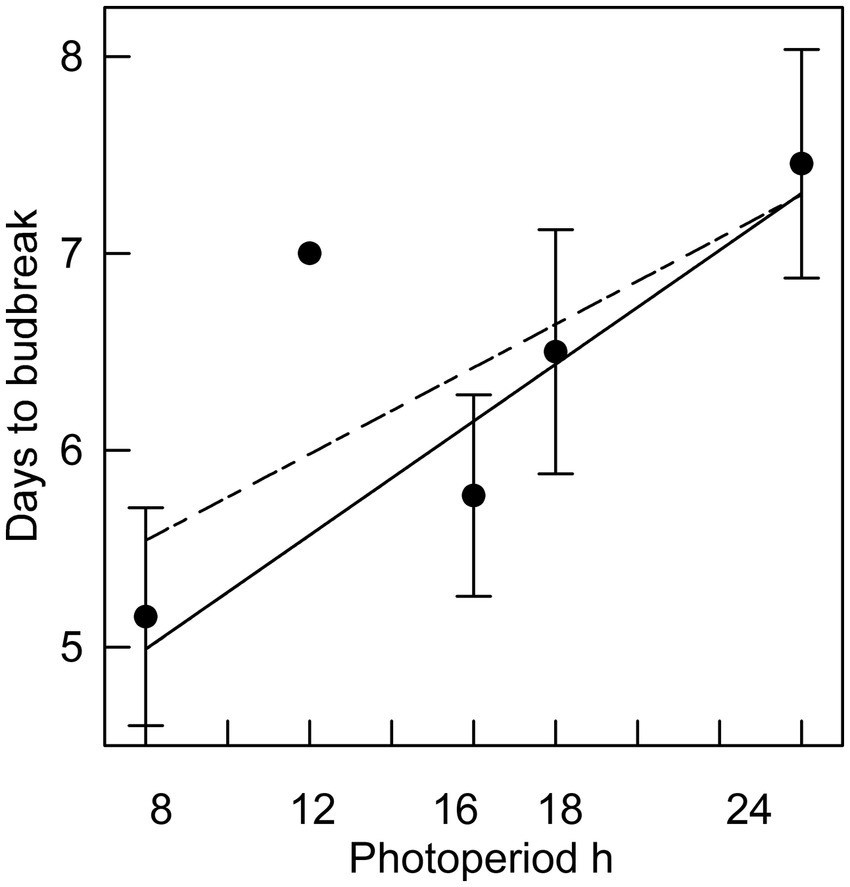
Figure 5. The relationship between photoperiod and days to bud break for seedlings placed in different photoperiods after 16 weeks of chilling (r2 = 0.93 excluding 12 h photoperiod). Error bars are standard errors. Solid line excludes 12 h photoperiod; dashed line includes 12 h photoperiod (r2 = 0.52).
4 Discussion
This study describes bud dormancy for black spruce seedlings through a full dormancy cycle, beginning at the point buds are just initiated and ending with buds that are fully released from endodormancy by chilling and dormancy is entirely due to ecodormancy. This contrasts with most dormancy studies, which focus on one of three periods during the bud dormancy cycle: 1. In some cases they examine the induction of buds (with few considering dormancy release shortly after bud induction); 2. In other instances, studies examine factors promoting the transition from bud endodormancy to ecodormancy, usually in response to chilling; or 3. Dormancy studies focus on the number of heat units required to release buds from ecodormancy leading to budburst. The present trial describes black spruce bud dormancy through a full developmental cycle, specifically: In the early stage of bud formation, beginning at the point buds are initiated (designated Week 0); during intense meristematic activity within the bud when needle primordia are rapidly initiating (up to about Week 4); and after bud morphogenesis is complete and as buds transition from endodormancy to ecodormancy, prompted by chilling (Week 9 in Figure 1 and beyond).
To better understand how bud dormancy changes throughout the dormancy cycle, a range of photoperiods were used, since different daylengths have either dormancy-inducing or dormancy-breaking effects and the requirement for chilling to break dormancy can be reduced by long days (Myking and Heide, 1995). Including photoperiod in the experiment also addresses the need identified by Hänninen et al. (2019) for experimental results describing the role of photoperiod in regulating the transition from endodormancy to ecodormancy and bud break.
Budburst was slowest (and, by definition, dormancy greatest) at the time buds were initiated. Studies of the ability to break bud by recently initiated buds are rare (e.g., Dormling et al., 1968; Qamaruddin et al., 1995; Vince-Prue et al., 2001). In this trial, exposing actively growing seedlings to an 8 h photoperiod induced terminal bud initiation, as expected (e.g., Maurya and Bhalerao, 2017). Dormling et al. (1968) observed that two- or three-weeks exposure to 8 h days resulted in long-lasting bud dormancy in Norway spruce (Picea abies L.) seedlings and that budbreak was faster at that developmental stage in longer photoperiods. Similarly, in this trial, when seedlings were exposed to a 24 h photoperiod, recently initiated buds broke on average after about four weeks, compared with about nine and a half weeks to break bud in a 16 h photoperiod. Bud dormancy decreased with additional time at 8 h, but over time bud burst continued to be faster in longer daylengths. We note that the differences in time to budburst are attributable to photoperiod and not to differences in temperature, since temperatures in all instances ranged between 20 and 25°C, avoiding the possibility of confounding effects of temperature on daily heat sums that may have occurred had there been a substantial day:night temperature differential. This contrasts with other studies (e.g., Zohner et al., 2016) in which night temperatures were colder than day temperatures. However, in natural environments, where night temperatures are usually cooler than day temperatures, temperature fluctuations may interact with photoperiod to speed up the rate of budburst after chilling requirements have been met (e.g., Partanen et al., 1998), and that warm night temperature (as was used in the current trial) can have less effect on the rate of budburst compared with warm day temperature (Fu et al., 2016).
Terminal buds that were initiated and entered endodormancy and remained in an 8 h photoperiod eventually broke bud given sufficient time, even without chilling to provide a dormancy-releasing stimulus. It is well-known that buds will resume growth in an 8 h photoperiod after receiving chilling or some other dormancy breaking treatment (e.g., Laube et al., 2014). However, the ability of buds to flush while in an inductive photoperiod without a dormancy breaking treatment is not widely recognized, even though a similar result was obtained by Dormling et al. (1968) for Norway spruce and Man et al. (2017, 2021) for numerous boreal and temperate North American tree species. These results indicate that “time” itself can substitute for cold temperatures and allow bud endodormancy to be released.
This contrasts with the commonly held view, such as that stated by Maurya and Bhalerao (2017), that “once dormancy is established, growth will not start under favorable conditions unless dormancy is terminated by exposure to dormancy-breaking signals, which, in many boreal and temperate trees, include prolonged exposure to low temperature.” Hannerz et al. (2003) similarly indicate that release from bud dormancy requires a period of chilling. However, we interpret these statements by Maurya and Bhalerao (2017) and Hannerz et al. (2003) as referring to the resumption of vigorous growth, as previous studies of boreal and temperate species show that trees with small amounts of chilling may flush, but with high proportions of abnormally flushed buds and stunted new shoot and leaf expansion (Man et al., 2017, 2021).
We observed that daylength affected budbreak at all dormancy stages. Longer photoperiods hastened budburst throughout endodormancy and ecodormancy, the sole exception being after 16 weeks of chilling, when longer photoperiods were antagonistic to budburst (see Figure 5). Differences in rates of budburst between photoperiods decreased with chilling, but after even 16 weeks of chilling, when endodormancy was minimal if not fully relieved, there was a significant correlation between photoperiod and days to budburst. Although the differences in time to budbreak at different photoperiods during ecodormancy have little practical significance, the existence of these differences may provide clues to some underlying physiological mechanisms. The presence of a photoperiodic effect during ecodormancy in this trial contrasts with the results of Laube et al. (2014), who report that a photoperiod effect on budbreak was only observed in trees that had not had their chilling requirement fulfilled.
The slower budburst at longer photoperiods found after 16 weeks of chilling in this trial is rarely observed, with one of the few other examples being reported by Partanen et al. (2001). Almost all other studies indicate that longer photoperiods decrease time to budbreak (e.g., Campbell, 1978; Basler and Körner, 2014; Rossi, 2014). Fu et al. (2019) suggest that daylength acts as a signal to cause budbreak to happen near a species-dependent optimal time of year, by increasing the heat requirement for budbreak when daylength is too short (i.e., prior to the optimal time for budbreak) and reducing the heat requirement if daylength is too long (i.e., after the optimal budbreak time). In the current trial, longer photoperiods retarded budburst in trees which had the greatest amount of chilling. This could be an adaptive trait, since a natural setting with a long, cool spring could be associated with an elevated risk of a damaging late spring frost. In that case, having longer daylengths provide a retrograde signal delaying budbreak would reduce the risk of spring frost damage. Such a mechanism is one interpretation of the results of this trial and, so far as we are aware, the existence of such an adaptive trait has not been reported.
However, this is not the first observation of a chilling-modulated response to photoperiod. Fu et al. (2019) hypothesized that a “long daylength effect” occurs in some deciduous tree species when cold springs delay budbreak, with faster budbreak occurring because trees have increased sensitivity to warm temperatures under increasing daylengths, reflected in a reduced requirement for growing degree days to resume growth. While our observed retrograde mechanism (slower rate of budburst with prolonged chilling) is the opposite of what Fu et al. (2019) observed, it is possible that both observations may be due to a common, as yet unexplained mechanism. In addition, our trial used one-year-old seedlings and it is also conceivable that the retrograde effect of photoperiod is a juvenile characteristic.
Both chilling and (with the exception noted above) long photoperiods reduced time to budburst. At the beginning of Phase II, a 24 h photoperiod without chilling had the same dormancy-breaking effect as about four weeks of chilling (i.e., on average seedlings broke bud after 8.4 days in 24 h days at the start of Phase II, equivalent to the time for budbreak of 8.6 days for budbreak in an 8 h photoperiod following four weeks of chilling). We conclude that four weeks of chilling at 5°C (equivalent to 672 chilling hours) was adequate to transition seedlings from endo- to ecodormancy, since an additional 12 weeks of chilling (16 weeks total) reduced time to budburst by only an additional four days. In comparison, Man et al. (2017) observed that the chilling requirement for black spruce was met with less than 600 chilling hours, which may reflect provenance differences in chilling requirement. Treatments promoting bud dormancy release can be important in managing greenhouse-grown tree seedlings. Occasionally, terminal buds are inadvertently induced before seedlings have reached their desired height. This study indicates that black spruce seedlings can be induced to resume shoot elongation most rapidly by exposure to 24 h day lengths. Budburst may take from as little as one week to slightly over three weeks, depending on the stage of bud dormancy.
Tao et al. (2021) estimated the dates of potential black spruce budburst with different levels of winter and spring warming. For the average latitude of their sites (47° 59′), they estimate that budburst would have occurred historically on May 28, with dates of budburst following 2, 4 and 6°C of warming falling on May 17, May 6 and April 24, respectively. We estimate that photoperiods on those dates of budburst at the average latitude of their sites would be 15.67 h, 15.27 h, 14.75 h and 14.13 h, respectively. We also estimate, based on our Figure 5 and assuming the chilling requirement to achieve ecodormancy was fully met, that there would be a photoperiod difference of about 1.5 h between the historical and 6°C scenarios. From Figure 5 we estimate that the difference in photoperiod would cause budbreak to occur about half a day earlier with 6°C warming. Whether this difference would be ecologically significant (e.g., making the species more competitive without increasing risk of damaging frost exposure) depends on the phenological responses of co-occurring species. We project from these estimates that for warming of 2° or 4°C that photoperiodic effects on budburst would be negligible.
In summary, bud dormancy decreased with time after bud initiation in the absence of a dormancy-releasing treatment. The effect of photoperiod on the rate of terminal budburst were greatest in seedlings at the time of bud initiation and decreased over time. Four weeks exposure to 5°C, beginning nine weeks after 100% bud initiation, were sufficient to pass buds from endodormancy to ecodormancy, at which time differences in the rate of budburst between 8 h and extended photoperiods were the smallest.
Data availability statement
The raw data supporting the conclusions of this article will be made available by the authors, without undue reservation.
Author contributions
SC: Conceptualization, Formal analysis, Investigation, Methodology, Writing – original draft, Writing – review & editing. RM: Conceptualization, Formal analysis, Writing – review & editing.
Funding
The author(s) declare that no financial support was received for the research, authorship, and/or publication of this article.
Acknowledgments
We thank Peter Menes of the Ontario Ministry of Natural Resources and Forestry for assistance during the study.
Conflict of interest
The authors declare that the research was conducted in the absence of any commercial or financial relationships that could be construed as a potential conflict of interest.
Publisher’s note
All claims expressed in this article are solely those of the authors and do not necessarily represent those of their affiliated organizations, or those of the publisher, the editors and the reviewers. Any product that may be evaluated in this article, or claim that may be made by its manufacturer, is not guaranteed or endorsed by the publisher.
Supplementary material
The Supplementary material for this article can be found online at: https://www.frontiersin.org/articles/10.3389/ffgc.2023.1261112/full#supplementary-material
References
Basler, D., and Körner, C. (2014). Photoperiod and temperature responses of bud swelling and bud burst in four temperate forest tree species. Tree Physiol. 34, 377–388. doi: 10.1093/treephys/tpu021
Campbell, R. K. (1978). “Regulation of bud-burst timing by temperature and photoregime during dormancy” in Proceedings: Fifth North American Forest Biology Workshop eds. C. A. Hollis and A. E. Squillace (Gainesville, FL: University of Florida), 19-33.
Casmey, M., Hamann, A., and Hacke, U. G. (2022). Adaptation of white spruce to climatic risk environments in spring: implications for assisted migration. For. Ecol. Manag. 525:120555. doi: 10.1016/j.foreco.2022.120555
Chao, W. S., Foley, M. E., Horvath, D. P., and Anderson, J. V. (2007). Signals regulating dormancy in vegetative buds. Int J Plant Dev Biol 1, 49–56.
Cooke, J. E. K., Eriksson, M. E., and Junttila, O. (2012). The dynamic nature of bud dormancy in trees: environmental control and molecular mechanisms. Plant Cell Environ. 35, 1707–1728. doi: 10.1111/j.1365-3040.2012.02552.x
Dennis, F. G. (2003). Problems in standardizing methods for evaluating the chilling requirements for the breaking of dormancy in buds of woody plants. HortScience 38, 347–350. doi: 10.21273/HORTSCI.38.3.347
Dormling, I., Gustafsson, A., and Von Wettstein, D. (1968). The experimental control of the life cycle in Picea abies (L.). Karst. Silvae Genet 17, 44–64.
Faust, M., Erez, A., Rowland, L. J., Wang, S. Y., and Norman, H. A. (1997). Bud dormancy in perennial fruit trees: physiological basis for dormancy induction, maintenance and release. HortScience 32, 623–629. doi: 10.21273/HORTSCI.32.4.623
Fu, Y. H., Liu, Y., De Boeck, H. J., Menzel, A., Nijs, I., Peaucelle, M., et al. (2016). Three times greater weight of daytime than of night-time temperature on leaf unfolding phenology in temperate trees. New Phytol. 212, 590–597. doi: 10.1111/nph.14073
Fu, Y. H., Zhang, X., Piao, S., Hao, F., Geng, X., Vitasse, Y., et al. (2019). Daylength helps temperate deciduous trees to leaf-out at the optimal time. Glob. Chang. Biol. 25, 2410–2418. doi: 10.1111/gcb.14633
Fu, Y. H., Zhao, H., Piao, S., Peaucelle, M., Peng, S., Zhou, G., et al. (2015). Declining global warming effects on the phenology of spring leaf unfolding. Nature 526, 104–107. doi: 10.1038/nature15402
Hannerz, M., Ekberg, I., and Norell, L. (2003). Variation in chilling requirements for completing bud rest between provenances of Norway spruce. Silvae Genetica 52, 161–168.
Hänninen, H., Kramer, K., Tanino, K., Zhang, R., Wu, J., and Fu, Y. H. (2019). Experiments are necessary in process-based tree phenology modelling. Trends Plant Sci. 24, 199–209. doi: 10.1016/j.tplants.2018.11.006
Hänninen, H., and Tanino, K. (2011). Tree seasonality in a warming climate. Trends Plant Sci. 16, 412–416. doi: 10.1016/j.tplants.2011.05.001
Heide, O. M. (1993). Growth and dormancy in Norway spruce ecotypes. II. After-effects of photoperiod and temperature on growth and development in subsequent years. Physiol. Plant. 31, 131–139. doi: 10.1111/j.1399-3054.1974.tb03117.x
Horvath, D. (2010). “Bud dormancy and growth” in Plant developmental biology—Biotechnological perspectives. eds. E. C. Pua and M. R. Davey (Berlin Heidelberg: Springer), 53–70.
Laube, J., Sparks, T. H., Estrella, N., Höfler, J., Ankerst, D. P., and Menzel, A. (2014). Chilling outweighs photoperiod in preventing precocious spring development. Glob. Chang. Biol. 20, 170–182. doi: 10.1111/gcb.12360
Man, R., Lu, P., and Dang, Q. L. (2017). Insufficient chilling effects vary among boreal tree species and chilling duration. Front. Plant Sci. 8:1354. doi: 10.3389/fpls.2017.01354
Man, R., Lu, P., and Dang, Q. L. (2021). Effects of insufficient chilling on budburst and growth of six temperate forest tree species in Ontario. New For. 52, 303–315. doi: 10.1007/s11056-020-09795-1
Maurya, J. P., and Bhalerao, R. P. (2017). Photoperiod-and temperature-mediated control of growth cessation and dormancy in trees: a molecular perspective. Ann. Bot. 120, 351–360. doi: 10.1093/aob/mcx061
Menzel, A., Estrella, N., and Fabian, P. (2001). Spatial and temporal variability of the phenological seasons in Germany from 1951 to 1996. Glob. Chang. Biol. 7, 657–666. doi: 10.1111/j.1365-2486.2001.00430.x
Myking, T., and Heide, O. M. (1995). Dormancy release and chilling requirement of buds of latitudinal ecotypes of Betula pendula and B. pubescens. Tree Physiol. 15, 697–704. doi: 10.1093/treephys/15.11.697
Partanen, J., Koski, V., and Hänninen, H. (1998). Effects of photoperiod and temperature on the timing of bud burst in Norway spruce (Picea abies). Tree Physiol. 18, 811–816. doi: 10.1093/treephys/18.12.811
Partanen, J., Leinonen, I., and Repo, T. (2001). Effect of accumulated duration of the light period on bud burst in Norway spruce (Picea abies) of varying ages. Silva Fennica 35, 111–117. doi: 10.14214/sf.608
Polgar, C. A., and Primack, R. B. (2011). Leaf-out phenology of temperate woody plants: from trees to ecosystems. New Phytol. 191, 926–941. doi: 10.1111/j.1469-8137.2011.03803.x
Qamaruddin, M., Ekberg, I., Dormling, I., Norell, L., Clapham, D., and Eriksson, G. (1995). Early effects of long nights on budset, bud dormancy and abscisic acid content in two populations of Picea abies. For. Genet. 2, 207–216.
Rinne, P., Hänninen, H., Kaikuranta, P., Jalonen, J. E., and Repo, T. (1997). Freezing exposure releases bud dormancy in Betula pubescens and B. pendula. Plant Cell Environ. 20, 1199–1204. doi: 10.1046/j.1365-3040.1997.d01-148.x
Rossi, S. (2014). Local adaptations and climate change: converging sensitivity of bud break in black spruce provenances. Int. J. Biometeorol. 59, 827–835. doi: 10.1007/s00484-014-0900-y
Rossi, S., and Bousquet, J. (2014). The bud break process and its variation among local populations of boreal black spruce. Front. Plant Sci. 5:574. doi: 10.3389/fpls.2014.00574
Singh, R. K., Svystun, T., AlDahmash, B., Jönsson, A. M., and Bhalerao, R. P. (2017). Photoperiod-and temperature-mediated control of phenology in trees - a molecular perspective. New Phytol. 213, 511–524. doi: 10.1111/nph.14346
Tao, J., Man, R., and Dang, Q. L. (2021). Earlier and more variable spring phenology projected for eastern Canadian boreal and temperate forests with climate warming. Trees, Forests and People 6:100127. doi: 10.1016/j.tfp.2021.100127
Templeton, C. W. G., Odlum, K. D., and Colombo, S. J. (1993). How to identify bud initiation and count needle primordia in first-year spruce seedlings. For. Chron. 69, 431–437. doi: 10.5558/tfc69431-4
Vince-Prue, D., Clapham, D. H., Ekberg, I., and Norell, L. (2001). Circadian timekeeping for the photoperiodic control of budset in Picea abies (Norway spruce) seedlings. Biol. Rhythm. Res. 32, 479–487. doi: 10.1076/brhm.32.4.479.1336
Keywords: chilling requirement, climatic warming, daylength, dormancy release, ecodormancy, endodormancy, spring bud phenology
Citation: Colombo SJ and Man R (2023) Daylength effects on black spruce bud dormancy release change during endo- and ecodormancy. Front. For. Glob. Change. 6:1261112. doi: 10.3389/ffgc.2023.1261112
Edited by:
Ned Fetcher, Wilkes University, United StatesReviewed by:
David Horvath, Agricultural Research Service (USDA), United StatesDaniel Chmura, Polish Academy of Sciences, Poland
Copyright © 2023 Colombo and Man. This is an open-access article distributed under the terms of the Creative Commons Attribution License (CC BY). The use, distribution or reproduction in other forums is permitted, provided the original author(s) and the copyright owner(s) are credited and that the original publication in this journal is cited, in accordance with accepted academic practice. No use, distribution or reproduction is permitted which does not comply with these terms.
*Correspondence: Stephen J. Colombo, ZWNvdmlld2NvbnN1bHRpbmdAZ21haWwuY29t