- 1Lewis Honors College, University of Kentucky, Lexington, KY, United States
- 2Department of Forestry and Natural Resources, University of Kentucky, Lexington, KY, United States
- 3Northern Research Station, USDA Forest Service, Grand Rapids, MN, United States
Introduction: Timber harvests influence coarse woody debris (CWD) dynamics both initially and long-term—contributing a significant amount of CWD as slash immediately after harvest, but also removing some or all of the mature trees necessary to produce CWD over time. Whereas shelterwood and other similar timber harvest systems retain varying amounts of the overstory, preserving CWD production after harvest, commercial clearcutting essentially eliminates sources of fresh CWD until regenerating trees are large enough to contribute CWD through fallen limbs or trunks, often decades after harvest. Forestry best management practices (BMPs) are critical for reducing the water quality impacts of timber harvest, but their effects on riparian and stream CWD are not well understood.
Methods: The current project explores CWD dynamics (surveyed in 2001, 2004, 2009, and 2020) in three eastern Kentucky watersheds receiving differing timber harvest treatments in 1983: unharvested control, BMPs (clearcut with a protected streamside management zone), and no BMPs (clearcut).
Results: Analysis of hydrology data over the period of record demonstrated significant flooding in 2004 that likely “reset” CWD in the study watersheds. Coarse woody debris volume was higher in control (3.33 m3/ha) than no BMP (1.03 m3/ha) in 2020, with CWD accumulation rates ranging from 0.039 m3/ha in the no BMP watershed to 0.19 m3/ha in the control.
Discussion: While not significantly different, CWD volume was nominally higher in the BMP watershed than the No BMP watershed, suggesting that, in addition to their many other benefits, streamside management zones help facilitate CWD provisioning during stand initiation after a commercial clearcut harvest. Furthermore, this study suggests that provisioning of CWD may not recover after clearcut harvesting for 100 years or more.
1. Introduction
Coarse woody debris (CWD) consists of down and dead woody material equal to or greater than 10 cm diameter (Idol et al., 2001; McClure et al., 2004). This material originates as a result of natural (e.g., disease, insect damage, wildfire, ice storms, hurricanes) and anthropogenic disturbances (e.g., timber harvests) (Jia-Bing et al., 2005). Coarse woody debris serves as important habitat for flora and fauna (Harmon et al., 1986; McMinn and Crossley, 1993). Wildlife species from small mammals to birds to macroinvertebrates inhabit and/or use woody material as both temporary and permanent shelter and feeding substrate (McMinn and Crossley, 1993; Loeb, 1996; Tirpak et al., 2006). Coarse woody debris can also directly influence understory plant species composition by regulating conditions at ground level, such as sunlight, moisture, and herbivory risk (Forrester et al., 2012). In addition to these effects, CWD plays a major role in biochemical processes in the forest floor, particularly through carbon and nitrogen cycling (Oberle et al., 2020). Organic carbon and nitrogen stored in CWD biomass are mobilized by fungi and other detritivores, enriching and supporting soil microbial and plant communities (Morrison et al., 2004; Norden et al., 2004; Fraver et al., 2013; Jones et al., 2019).
Coarse woody debris performs additional functions in stream and riparian areas. For example, riparian CWD provides critical habitat for riparian-associated species, such as herpetofauna (Otto et al., 2013), and serves as a buffer between forests and streams, reducing erosion risk and slowing and filtering surface runoff (Holmes et al., 2010). As CWD enters streams from the riparian area, it may initiate physical and chemical changes within stream ecosystems (Nakamura and Swanson, 1993). Coarse woody debris provides habitat structure for many freshwater invertebrate, fish, and amphibian species (Fausch and Northcote, 1992; Everett and Ruiz, 1993; Phillips and Kilambi, 1994a,b; Sweka and Hartman, 2006), provides shade (Siderhurst et al., 2010), and contributes dissolved and particulate organic matter to the aquatic food web (Gurnell et al., 1995). Hydrologically, CWD can form debris dams, altering streamflow paths and modifying flooding regimes (Merten et al., 2013; McDade et al., 2020).
Timber harvests often create a pulse of CWD immediately following logging, through the contribution of slash left on site (McCarthy and Bailey, 1994). However, after the initial contribution of CWD through slash, future CWD contributions in harvested stands usually are reduced unless the logging practice leaves sufficient remnant trees (e.g., shelterwood harvest systems) or until regenerating trees are large enough to produce CWD (Muller and Liu, 1991; Idol et al., 2001; Wang et al., 2005; Gangloff et al., 2015; Burton et al., 2016). In clearcut harvested stands, this lag period could last for decades, especially for large diameter CWD (McCarthy and Bailey, 1994; Webster and Jenkins, 2005). Furthermore, in topographically complex areas, CWD levels may vary with slope position, either increasing in higher slope positions (Webster and Jenkins, 2005) or accumulating in downslope positions (Rubino and McCarthy, 2003; Parker and Hart, 2014). Streams and riparian areas in harvested watersheds may have low CWD stocks once CWD produced during logging decays or is exported from the watershed by flooding, potentially altering ecosystem structure and function across the forest floor and within the stream channel. Conversely, because timber harvest within streamside management zones (SMZs) is restricted to some extent in many jurisdictions (Witt et al., 2016), streams and riparian areas in watersheds harvested using BMPs (such as limited disturbance and harvesting) may experience more consistent levels of CWD over time due to reserves remaining in the SMZ.
Throughout the Appalachian Region of North America, and particularly in the Cumberland Plateau of Kentucky and Tennessee, timber harvest is a key anthropogenic process controlling coarse woody debris dynamics (McCarthy and Bailey, 1994). The Cumberland Plateau is characterized by steep slopes, biodiverse hardwood forests (Braun, 1950), and extensive stream networks comprised primarily of headwater streams (Villines et al., 2015) that are vulnerable to sedimentation if timber harvests are not conducted with appropriate protections (Aust and Blinn, 2004). Forestry best management practices (BMPs) in the Cumberland Plateau emphasize stream protection by maintaining an unharvested buffer along streams (a streamside management zone, SMZ), carefully constructing roads and trails, and minimizing soil disturbance (Aust and Blinn, 2004). Although minimizing activity in the SMZ significantly reduces erosion risk, sediment contribution (Bowker et al., 2020), and other sources of water quality impairment (Witt et al., 2016), the effects of BMPs on CWD dynamics in the Appalachian Region are not well-understood.
In this study, the volume, distribution, and state of decay of CWD were assessed in three watersheds involved in a forestry BMP study in Robinson Forest in the 1980s (Arthur et al., 1998; McClure et al., 2004). Each watershed in the study received a unique timber harvest treatment in the original study: watershed A was an uncut control (control); watershed B (BMP) was clearcut logged with a protected 15.2 m riparian buffer zone and retirement of logging roads after harvest (e.g., installation of water control structures, revegetation); and watershed C was clearcut without the use of BMPs (no BMP). Both clearcut watersheds were harvested for commercial sawtimber, with a follow-up treatment cutting and leaving on site all stems <5 cm (Arthur et al., 1998). The initial harvesting of these watersheds occurred in 1983, when stands in all three watersheds were estimated to be 70+ years old (McClure et al., 2004), and the sites were left untouched for 18 years post-harvest (Arthur et al., 1998). In 2001, McClure et al. (2004) reported that both in-stream and riparian CWD volume was greater in both harvested watersheds than in the unharvested control, attributed to the slash left during harvest. They also noted higher CWD abundance in stream than riparian plots, attributed to CWD sloughing downslope and accumulating in the stream. Finally, they reported that CWD was more decayed in the no BMP watershed, compared to the BMP or control watersheds, a relationship they attributed to the lack of new CWD recruitment in the no BMP watershed (McClure et al., 2004). This paper reports on data from additional CWD surveys conducted in 2004, 2009, and 2020, representing 21, 26, and 37 years post-harvest. At this point, we expected CWD produced during harvest to have decayed or been washed out of the harvested watersheds. We thus hypothesized that CWD volume and biomass in the watershed harvested without BMPs would be lower than in the control or the watershed harvested with BMPs. We also expected any CWD present in the watershed harvested without BMPs to be in a state of advanced decay due to a lack of significant large CWD inputs; thus, we hypothesized that decay class will be higher in harvested watersheds than the unharvested control.
2. Methods and materials
2.1. Site description
This study was conducted within three small headwater watersheds located at the University of Kentucky’s Robinson Forest (37°27 N, 83°8 W) (Figure 1). Robinson Forest, situated in southeastern Kentucky’s Breathitt, Knott, and Perry counties within the Cumberland Plateau physiographic province, was acquired by the University of Kentucky after being commercially harvested in the early 1900s (Overstreet, 1984). It is a characteristic mixed mesophytic forest (Braun, 1950), dominated by oaks (Quercus spp.), hickories (Carya spp.), and yellow poplar (Liriodendron tulipifera), with pines (Pinus spp.) on drier ridgetops (Overstreet, 1984). Climate at Robinson Forest is temperate and humid, with an average annual temperature of 13.6°C and mean annual precipitation of 1,121 mm (Sena et al., 2021). Elevation ranges from 245 to 475 m (Arthur et al., 1998). Sandstone, shale, and siltstone parent formations support well-drained Grigsby–Rowdy loams, as well as stony Cloverlick–Shelocta–Kimper/Handshoe–Fedscreek–Shelocta complexes (Williamson and Barton, 2020). The site’s terrain varies in slope from its stream topography (7%–22%) to its steeper riparian bank borders (33%–88%). Streams within each studied watershed are first-order perennial streams that join to form a stream called Field Branch, which is nested within the Clemons Fork watershed (Figure 1; McClure et al., 2004). Although surface mining for coal is a common and important driver of land use change in the Appalachian Region, including in tracts of land adjacent to Robinson Forest, the study watersheds did not have a history of surface mining.
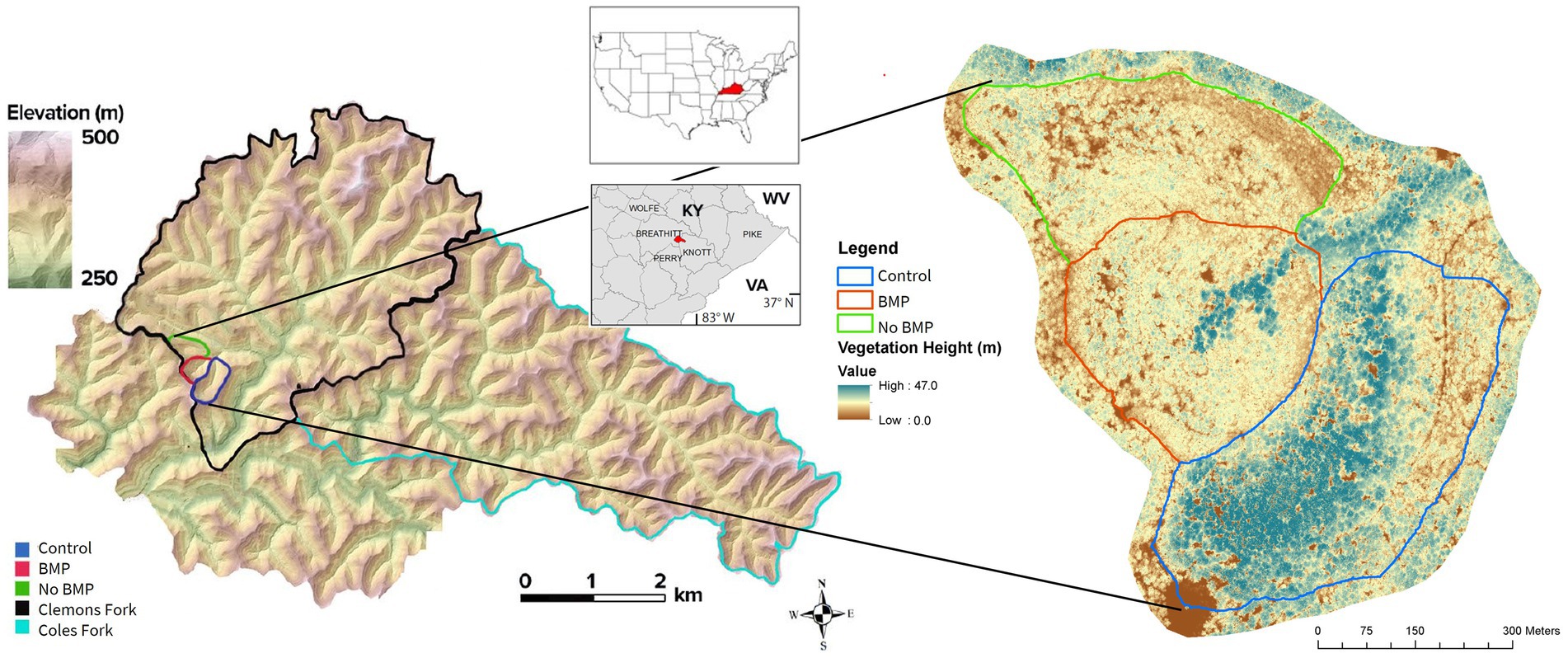
Figure 1. Map of study watersheds, nested within Clemons Fork in Robinson Forest, eastern Kentucky, United States. Also shown is Coles Fork, a large, minimally disturbed watershed often paired with Clemons Fork. Vegetation height of study watersheds was estimated by LiDAR in 2013. The weir at Field Branch (located at the confluence of the studied streams) is located at 37° 28′ 12.78″ N, 83° 08′ 42.22″ W.
As described by Arthur et al. (1998), each experimental watershed was assigned a unique silvicultural treatment in autumn of 1983. Watershed A (control) served as an unharvested control. Watershed B (BMP) was clearcut harvested but with a protected 50-foot (15.2 m) unharvested riparian buffer (Arthur et al., 1998). Watershed C (no BMP) was clearcut harvested without BMPs in place—no riparian buffer zone was protected, and trees were clearcut down to the streamside. Harvest treatments were still evident in a 2013 LiDAR survey (Figure 1), 30 years after harvest—trees in the unharvested control were taller than those in the other watersheds, except for the riparian buffer in the BMP watershed (Figure 1). Height distribution class data demonstrated a more even distribution across height classes in the control watershed with maximum canopy height at 42.5 m, whereas the clearcut watershed demonstrated a dominance by lower height classes (15–22.5 m) and a maximum of 27.5 m (Figure 1). The BMP watershed was also dominated by lower height classes, but maximum height ranged to 37.5 m in the protected streamside management zones (Figure 1).
2.2. Field data approach & collection
Coarse woody debris was surveyed at the study sites in 2001, 2004, 2009, and 2020, in 24 plots (8 plots on each side of the stream, and 8 plots within the stream) in each watershed. Stream plots were 25 m long and as wide as the stream channel. Riparian plots were established on either side of the stream, starting at the stream edge and extending 15.2 meters upslope. Due to varying stream topography, individual riparian plots were not identical; however, each plot measured approximately 15.2 meters × 25 meters. The total length of the surveyed stream reach, from the flume at the downstream end to the most upstream plot, was 200 meters (McClure et al., 2004).
For each piece of CWD (at least 10 cm in diameter), calipers were used to measure diameter at three points (each end and approximately in the middle), and length was recorded from end-to-end measurements with measuring tapes. Volume was calculated from mean diameter and length data using the equation for volume of a cylinder. Decay class was visually estimated according to a 1–5 scale, with a score of 1 indicating fresh-fallen debris and 5 describing CWD in advanced decay with no structural integrity (McClure et al., 2004). Samples judged to be within class 5 were disregarded due to overall lack of structural integrity and inability to support proper diameter measurements, consistent with McClure et al. (2004). Biomass was estimated from volume using a decay-specific density conversion factor calculated by McClure et al. (2004): 0.50, 0.45, 0.41, and 0.29 g/cm3 for decay classes 1–4, respectively.
2.3. Hydrology data collection and processing
To better understand streamflow dynamics in these watersheds, especially including significant flooding events, streamflow data were assembled from the Robinson Forest Environmental Monitoring Network records for Field Branch. All three watersheds in the study drain to Field Branch. Streamflow has been monitored in Field Branch using a v-notched weir since 1971. Stage height was recorded on stripcharts until systems were upgraded to electronic data loggers in 2009. Data were assembled and checked for potential errors as described in Sena et al. (2021). Briefly, streamflow data spanning as much of the surveyed period as possible (1971–2019) were visually assessed using graphs in Excel to identify potentially corrupted data (e.g., inaccurate data recorded by a malfunctioning data logger). These errant data were excluded as missing data for further analysis. Compiled and edited streamflow data were aggregated to daily and monthly minimum, maximum, and mean values (cfs) using custom scripts in R (R Core Team, 2021). To describe streamflow at the site, 5th, 50th, and 95th percentile mean daily streamflow were identified by month within decade (for decades starting in 1971, 1981, 1991, 2001, and 2011), using custom scripts in R (R Core Team, 2021), and a frequency analysis was conducted to identify the recurrence interval of major flood events occurring during the study period.
2.4. Data analyses
Data from the four surveys (2001, 2004, 2009, and 2020) were assembled from hard copy and digitized records and checked for accuracy and completeness using Excel and R. Data were aggregated to plot-level in R using the “summarize” function. Briefly, mean length, diameter, and decay class were calculated for each plot to permit evaluation of any shifts in the size and/or decay class of individual pieces over time and across watersheds. Mean decay class was not weighted by CWD size. However, to better understand plot-level CWD dynamics, total CWD volume and biomass were assessed at the plot level by summing the volume and biomass estimates of all the pieces in each plot. Plot-level volume and biomass were subsequently standardized by plot area. To calculate an annual CWD accumulation rate, CWD volume in 2004 (the minimum CWD volume found during the survey period) was subtracted from CWD volume in 2020, and the difference was divided by 16 years. To evaluate potential differences in CWD stocks across size classes, we also computed summary statistics (mean ± SD volume per piece, total volume) by diameter size classes (<25 cm, 25–65 cm, >65 cm), similar to McCarthy and Bailey (1994).
Data were analyzed for effects of silvicultural treatment, time since harvest, and their interaction using a two-way ANOVA. To meet model assumptions of normally distributed residuals, volume and biomass data were log-transformed. Preliminary analysis using a mixed models approach failed to converge due to a lack of variance in the random error term (variance among plots within watershed); accordingly, the model was simplified to a linear model (using the “lm” function in R). Significant ANOVA results (p < 0.05) were followed up with pairwise Tukey tests.
3. Results
The treatment × year interaction was significant (F = 2.46, df = 6, p = 0.026) for CWD volume in riparian plots (Figure 2). Pairwise comparisons found a significant treatment effect only in the 2020 survey year, for which volume was higher in the control (3.33 m3/ha) than the no BMP watershed (1.03 m3/ha; p = 0.009; Table 1). Riparian CWD volume in all three watersheds decreased significantly from 2001 to 2004, then recovered significantly from 2004 to 2009. Coarse woody debris volume in 2020 was significantly higher than the 2004 minimum in the control and BMP watersheds, but not in the no BMP watershed. Coarse woody debris volume increased at an average annual rate of 0.19 m3/ha/year in the control watershed, nearly 2.5× higher than the accumulation rate in the BMP watershed (0.076 m3/ha/year) and 4.7× higher than the accumulation rate in the no BMP watershed (0.039 m3/ha/year). In stream plots, CWD volume was significantly different only across years (F = 10.5, df = 3, p < 0.001), with volume decreasing significantly from 2001 to 2004, but not significantly changing from 2004 levels by 2020 (Figure 2). Nominally, CWD increased at an annual rate of 0.24 m3/ha/year in the control watershed stream, slightly less than that of the BMP watershed (0.30 m3/ha/year). In contrast, CWD volume decreased slightly from 2004 to 2020 in the no BMP watershed, at an annual rate of 0.22 m3/ha/year.
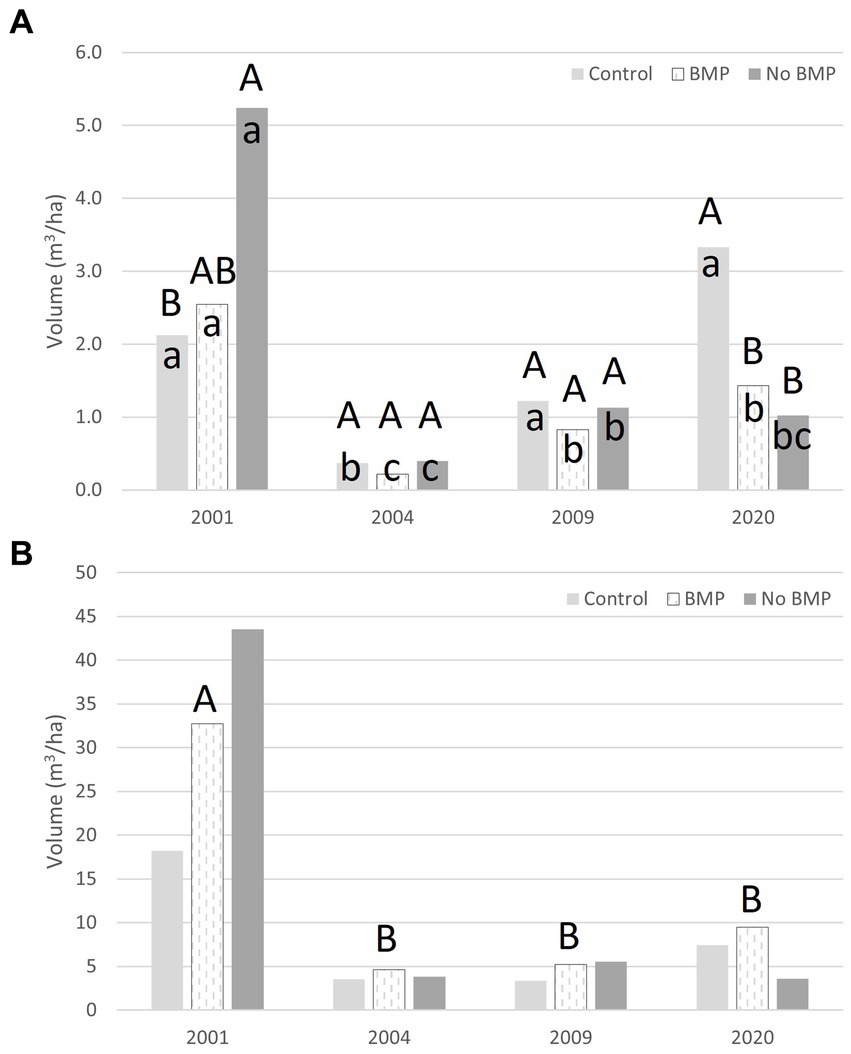
Figure 2. Mean CWD volume by year and silvicultural treatment in riparian (A) and stream (B) plots. The BMP watershed was clearcut harvested with protected streamside management zones. The no BMP watershed was clearcut harvested with no streamside protection. The control watershed was left unharvested. Harvest treatments were implemented in 1983, and coarse woody debris was surveyed in 2001, 2004, 2009, and 2020. All watersheds were in the Field Branch watershed in Robinson Forest, eastern Kentucky, United States. Lowercase letters (abc) denote significant treatment effects within year, while uppercase letters (ABC) denote significant year effects within treatment.
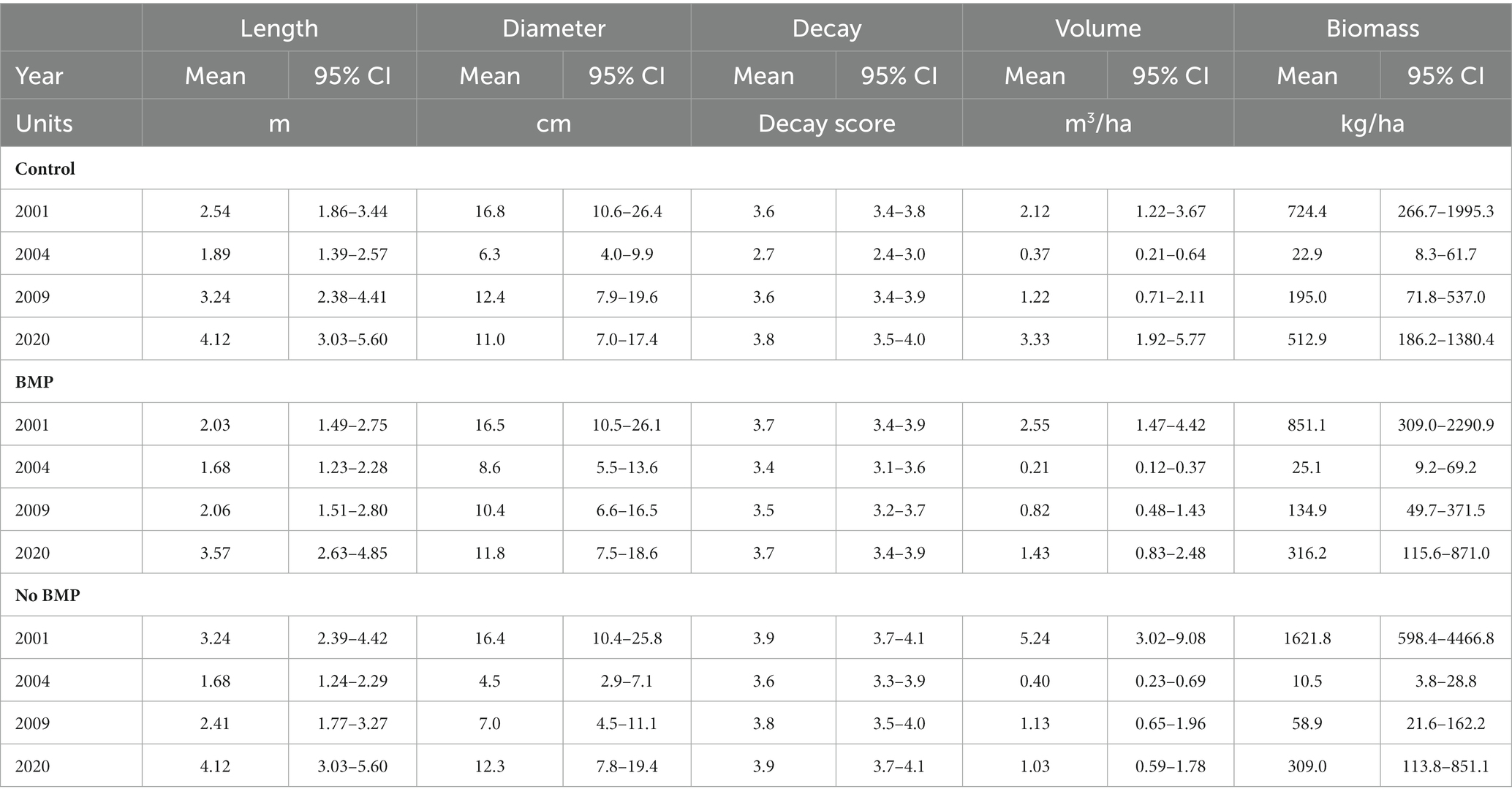
Table 1. Characteristics of coarse woody debris distribution in riparian plots in three watersheds in Robinson Forest.
Riparian CWD biomass was significantly different across years (F = 34.2, df = 3, p < 0.001; Table 1), but treatment and their interaction were not significant. Biomass decreased significantly from 2001 (1,000 kg/ha) to 2004 (18.2 kg/ha), then increased significantly in 2009 (117 kg/ha) and recovered to levels statistically similar to 2001 in 2020 (372 kg/ha). Similarly, stream CWD biomass was significantly associated with year only (F = 6.1, df = 3, p < 0.001), decreasing from 2001 (10,000 kg/ha) to 2004 (692 kg/ha), but did not significantly change from 2004 to 2020 (Table 2).
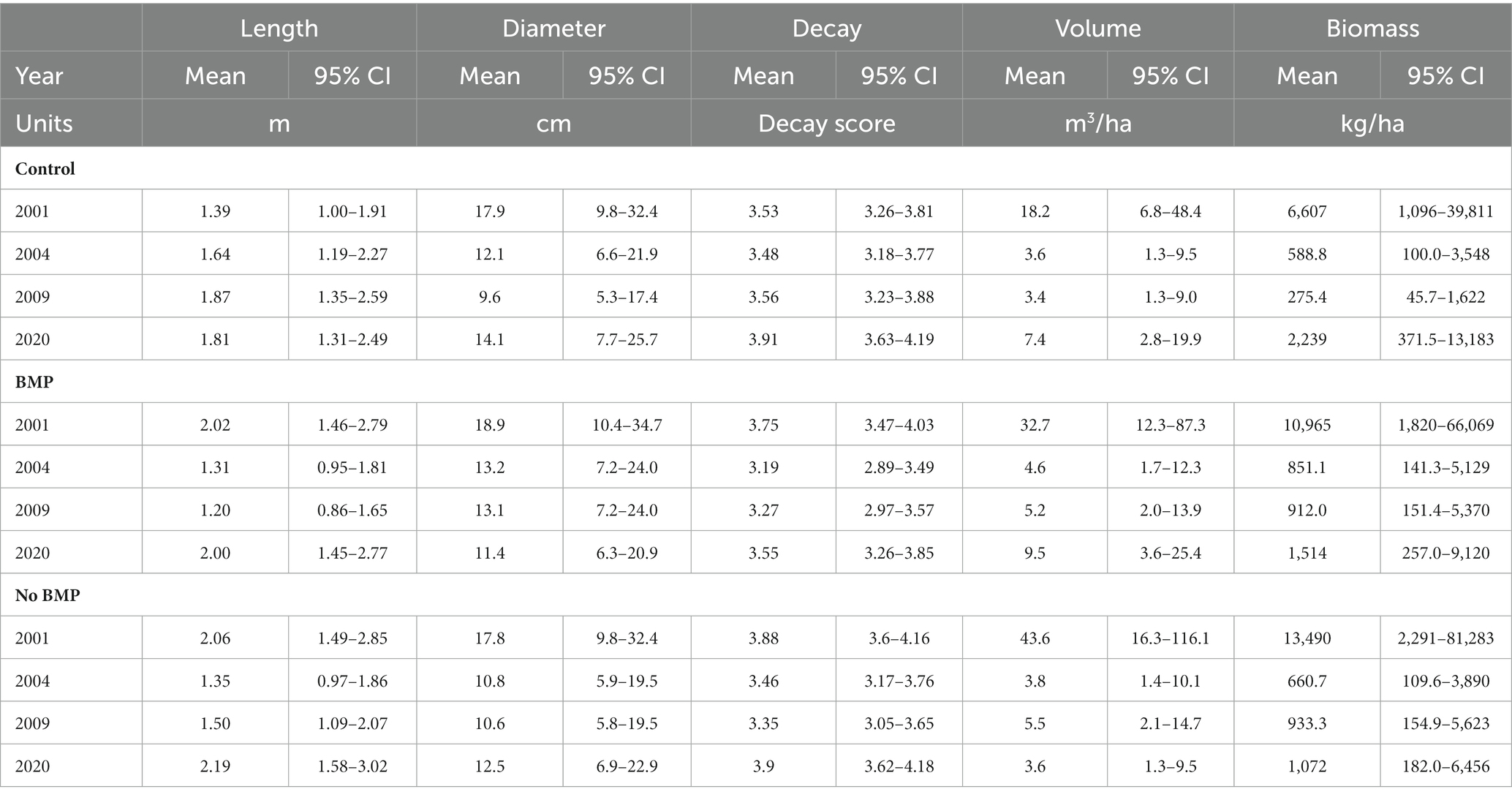
Table 2. Characteristics of coarse woody debris distribution in stream plots in three watersheds in Robinson Forest.
In riparian plots, the treatment × year interaction was significant for unweighted mean CWD decay class (F = 2.65, df = 6, p = 0.018; Figure 3). A treatment effect was significant only for 2004, when CWD decay was more advanced in BMP (3.4) and no BMP watersheds (3.6) than in the control watershed (2.7). Year effects were significant within watersheds only for the control watershed, where decay class decreased from 2001 (3.6) to 2004 (2.7), then increased in 2009 (3.6) and stayed similar through 2020 (3.8). Stream CWD decay class was significantly different for year only (F = 6.86, df = 3, p = 0.0004), decreasing from 2001 (3.72) to 2004 (3.38), then increasing to near-2001 levels in 2020 (3.79; Figure 3).
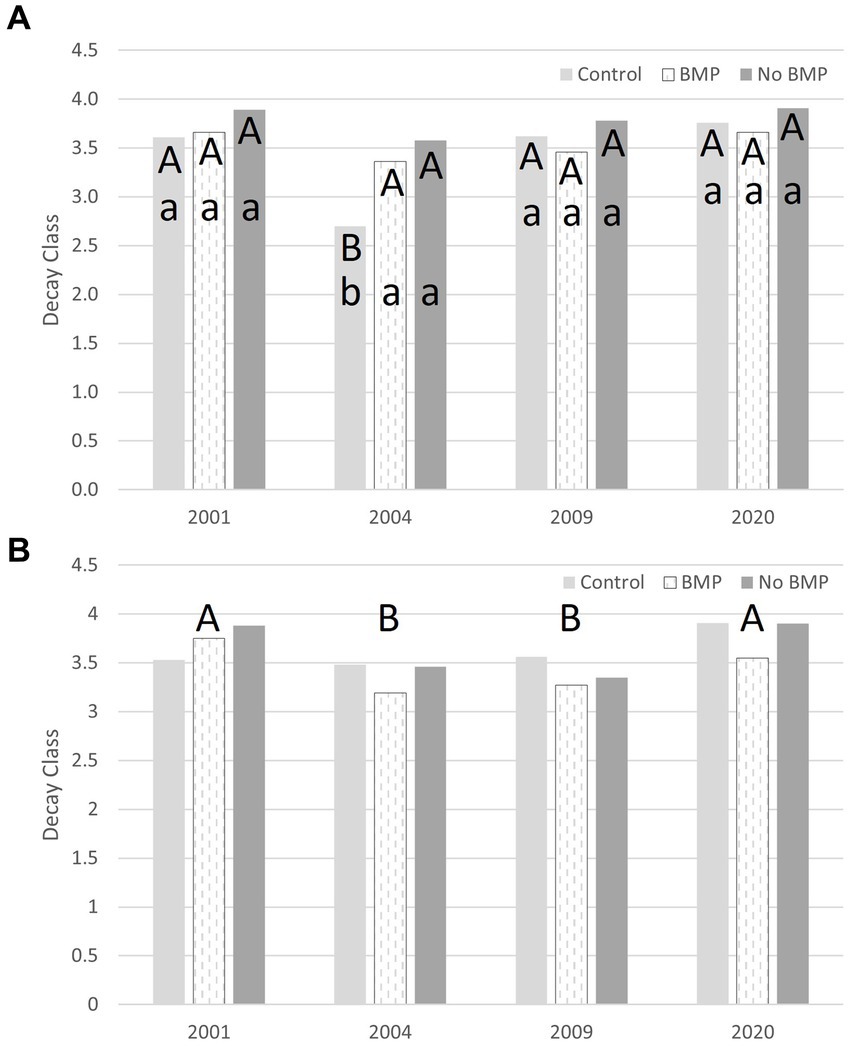
Figure 3. Mean CWD decay class by year and silvicultural treatment in riparian (A) and stream (B) plots. The BMP watershed was clearcut harvested with protected streamside management zones. The no BMP watershed was clearcut harvested with no streamside protection. The control watershed was left unharvested. Harvest treatments were implemented in 1983, and coarse woody debris was surveyed in 2001, 2004, 2009, and 2020. All watersheds were in the Field Branch watershed in Robinson Forest, eastern Kentucky, United States. Lowercase letters (abc) denote significant treatment effects within year, while uppercase letters (ABC) denote significant year effects within treatment.
The majority of surveyed pieces of CWD were from smaller diameter classes (<25 cm) in all study years and watersheds (Table 3). Coarse woody debris pieces of intermediate size (25–65 cm) contributed greater total volume than pieces <25 cm in the control and BMP watersheds in 2001, 2009, and 2020. In contrast, CWD pieces 25–65 cm in diameter contributed less total volume than pieces <25 cm in the no BMP watershed in all study years, as well as in all watersheds in 2004. Only three CWD pieces >65 cm were recorded across all study years and watersheds, and all three were found in the control watershed in 2009 and 2020.
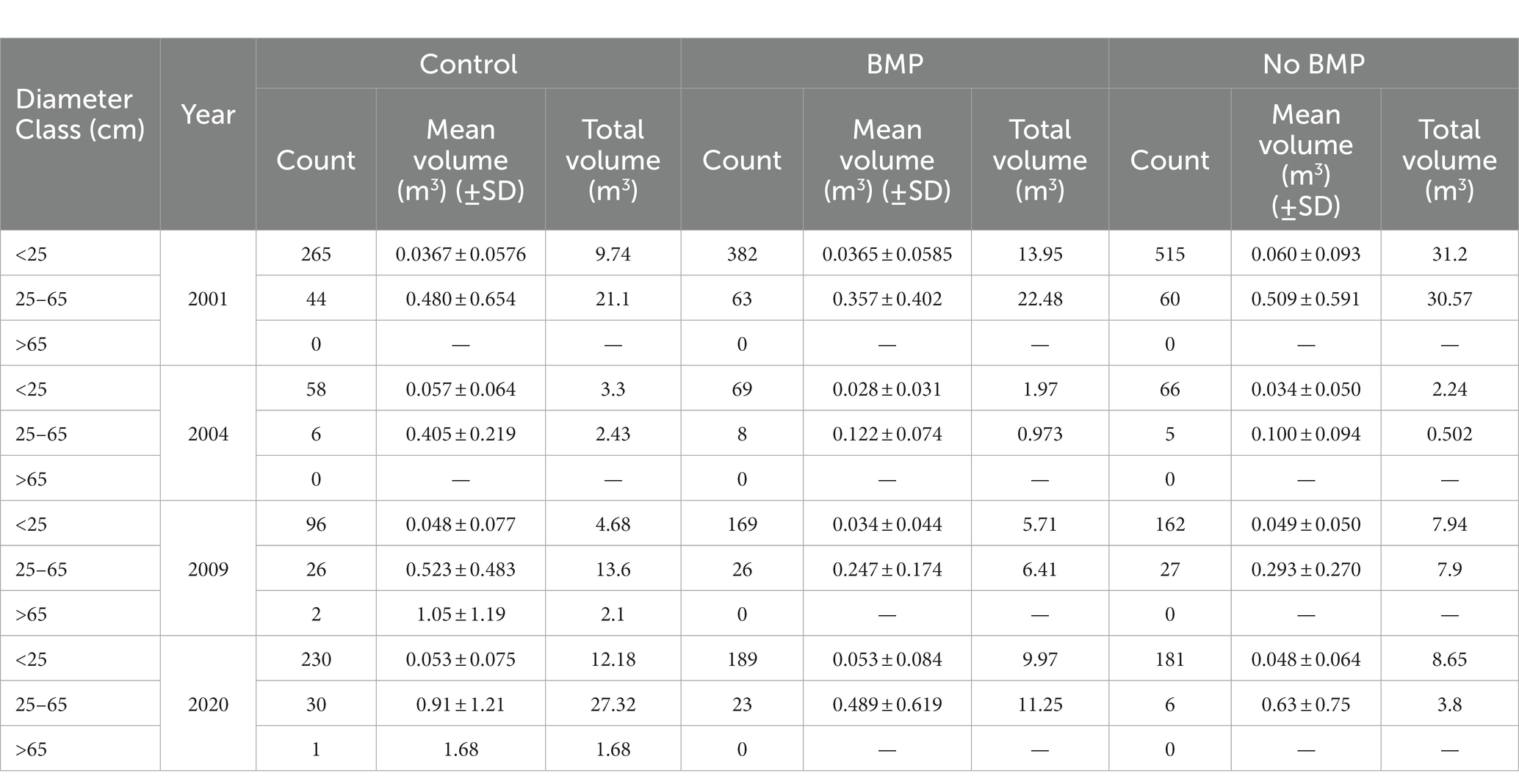
Table 3. Coarse woody debris count, mean volume, and total volume by diameter size class; data are pooled across all plots (riparian and stream) within the given watershed (control, BMP, or no BMP).
Mean daily streamflow varied seasonally, with low flows (5th percentile) averaging 0.07–0.12 cfs1 from February to April but averaging 0.001–0.004 cfs from June to November. Similarly, 50th percentile flows averaged 0.285–0.350 cfs from February to April and 0.02 to 0.07 from June to November. High flows (95th percentile) demonstrated less seasonal variability and ranged from 0.36 to 4.15 cfs. Daily maximum streamflow exceeded 50 cfs only 9 times during the period of record (1971–2019), four times prior to logging in 1983 and five times after. After 1983, these events occurred in May 1984 (51.8 cfs), May 2004 (113.0 cfs), May 2009 (two events: 55.0 and 262.1 cfs, respectively) and July 2015 (70.9 cfs), of which the 2004 and 2009 floods are most relevant for this study (Figure 4). The 2009 flood (262.1 cfs) was the largest flood event in the 48 years period of record; thus, the estimated recurrence interval for this event was 49 years. The estimated recurrence interval for the 2004 flood was 16.3 years.
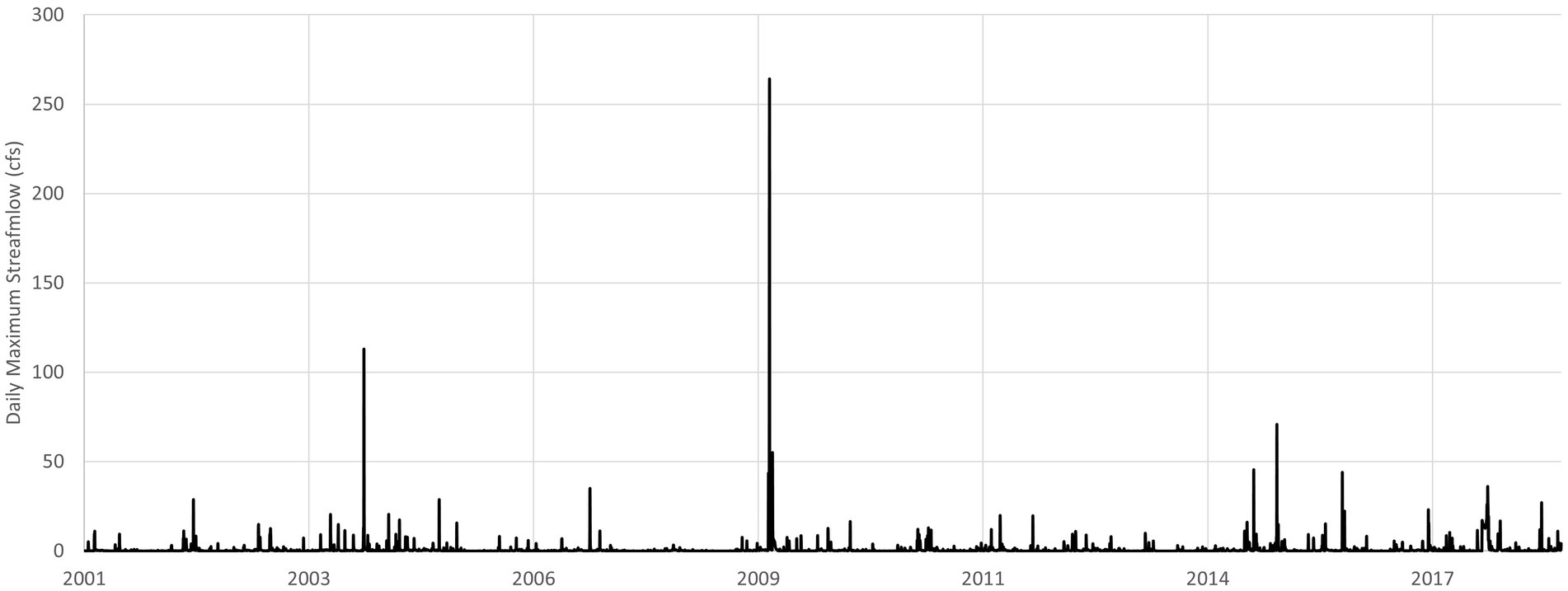
Figure 4. Daily maximum streamflow (cfs) for Field Branch, in which the study watersheds are subwatersheds, from January 2001 to December 2018.
4. Discussion
We observed relatively few differences in CWD stocks among treatments 21 to 37 years post-harvest. Among the differences that we observed were significantly elevated CWD volume in riparian plots in the control watershed compared to the no BMP watershed, with only nominally higher CWD volume in the BMP watershed. However, we note that serious floods occurring during the study period likely influenced CWD levels in both stream and riparian plots. In general, CWD volume and biomass decreased significantly from 2001 to 2004, then increased through 2020. The significant decrease in CWD volume and biomass from 2001 to 2004 was likely associated with a major flood event occurring in May 2004. Shortly after this flood, we observed high water marks well into the riparian area of the study watersheds, supporting the idea that the flood rapidly exported both stream and riparian CWD from these watersheds. Furthermore, export of both riparian and stream CWD during the flood of 2004 is consistent with the literature documenting accumulation of CWD in lower topographic positions in steep watersheds (Rubino and McCarthy, 2003; Parker and Hart, 2014)—many of the riparian CWD pieces observed in 2001 were adjacent to or partially in the stream, rendering them particularly vulnerable to export by flooding. Alongside the rapid export of CWD from stream and riparian areas, we noted a shift in streambed material from sand and fine sediments to bedrock and occasional cobble—the streambeds were essentially scoured by this flood event. Subsequent floods in 2009 and 2015 likely did not have a similar effect because much of the CWD that existed in 2001 had been removed from the streams in 2004 and recruitment of new CWD after that time was not sufficient to have a similar effect. Importantly, although volume did tend to increase from 2004 to 2020, we note that this increase was slight—riparian CWD volume achieved near 2001 levels by 2020 only in the control watershed, but CWD volume lagged below 2001 levels in all other treatments for both stream and riparian plots, suggesting that recruitment of new CWD in these treated watersheds was low.
In 2001, 18 years post-harvest, McClure et al. (2004) reported that CWD in the no-BMP treatment was in a more advanced state of decay than in the other watersheds, which they attributed to a lack of fresh CWD production in that watershed. For riparian CWD, our study found a significant decrease in CWD decay class from 2001 to 2004 in the control watershed only—we suggest that the retention of large trees throughout this unharvested watershed presented opportunity for fresh treefall between 2001 and 2004. For stream CWD, the significant decrease in decay class from 2001 to 2004 across all watersheds similarly suggests an export of existing CWD and import of fresher material from riparian areas during the May 2004 flood and other events. However, the increase in CWD decay class in all watersheds in both stream and riparian plots from 2004 to 2020 further supports the observation that recruitment of new CWD is limited.
In 2001, McClure et al. (2004) found higher CWD volume in harvested watersheds than in the control. This is consistent with the literature on the effects of clearcut harvesting on CWD volume—for some time after harvest, CWD levels are elevated in harvested watersheds due to the production of slash during the logging process. However, once that slash deteriorates or is exported from the watershed, clearcut stands experience a dearth of CWD due to the absence of trees large enough to produce it (McCarthy and Bailey, 1994). This was demonstrated in our study as an inversion over time—CWD volume was highest in the harvested watersheds in 2001, but highest in the control watershed in 2020. Furthermore, although our study did not find significant differences in CWD volumes between the watershed harvested using BMPs and the watershed harvested without BMPs, we note that 2020 CWD volume was lowest in the clearcut watershed and highest in the unharvested control, with the BMP watershed intermediate. Similarly, average annual riparian CWD recruitment was greatest in the unharvested control, followed by the BMP watershed and then the clearcut watershed. In contrast, annual stream CWD recruitment was highest in the BMP watershed, and negative in the clearcut watershed, with the control intermediate. Although statistically insignificant, these patterns suggest that protecting riparian buffers during clearcut harvesting may facilitate CWD recovery compared to clearcut watersheds with no BMPs, in which CWD production remains low for decades after harvest (McCarthy and Bailey, 1994; Jenkins et al., 2004; Webster and Jenkins, 2005). Continued periodic surveys will be essential to evaluate whether CWD levels in the harvested watersheds diverge further in the future as these stands continue to develop.
In addition, we note that the levels of CWD reported in this study, even in the control watershed, are low compared to other studied sites in the region. Webster and Jenkins (2005) reported CWD volumes in 70–80 years old stands in the Great Smoky Mountains of 21–87 m3/ha in sites with anthropogenic disturbance history, ranging up to 134 m3/ha in minimally disturbed sites. Nearer the current study, Muller and Liu (1991) reported average CWD volumes of 66.3 m3/ha in an old-growth stand of Lilley Cornett Woods in southeastern Kentucky. In contrast, the highest riparian CWD volumes in the control watershed in our study were 3.33 m3/ha—20× less than that reported in undisturbed sites by Muller and Liu (1991) and nearly 50× less than that reported in undisturbed sites by Webster and Jenkins (2005). However, although the watershed used as a control in this watershed was not harvested during this study, it is itself a second-growth stand—when compared to the anthropogenically disturbed sites sampled by Webster and Jenkins (2005), our control site was much more similar, with only ~6× less CWD volume. All of Robinson Forest was commercially harvested in the early 1900s prior to being acquired by the University of Kentucky (Overstreet, 1984); thus, even the most minimally disturbed stands in the forest are second-growth stands at most ~120 years old. In their study, McCarthy and Bailey (1994) found that CWD stocks shifted from smaller material (primarily slash in clearcut stands) to larger size classes as harvested stands develop from clearcut to pole (~25 years) to mature (~75 years) to old (~100 years). Similarly, coarse woody debris surveyed in this study was dominated by smaller diameter material (<25 cm), with relatively few pieces 25–65 cm and only three pieces >65 cm. While we lacked statistical power to test for effects of silvicultural treatment and time since harvesting on size class distributions, our data suggest that total CWD stocks of intermediate size classes recovered to higher levels in control and BMP watersheds than the no BMP watershed, consistent with our assessments of plot-level volume. Similarly, consistent with McCarthy and Bailey (1994), these data illustrate that large-diameter CWD (>65 cm) may not be present in stands until a century or more after clearcut harvesting.
Production of new CWD in harvested watersheds is critical for maintenance of riparian and stream ecosystems, both locally and downstream. Large CWD in streams and riparian areas can modify streamflow patterns (Merten et al., 2013; McDade et al., 2020) and provide critical habitat for aquatic and riparian wildlife species (Otto et al., 2013). Furthermore, CWD produced in uplands can eventually be exported downstream via ephemeral channels, contributing significant volumes of organic matter to downstream food webs (Fritz et al., 2019). Thus, in addition to the water quality improvement benefits of BMPs reported elsewhere (Witt et al., 2016; Bowker et al., 2020), BMPs may further protect streams by safeguarding the future supply of riparian and stream CWD. However, continued surveys into the coming decades will be necessary to evaluate long-term trends in CWD production across the studied treatments.
5. Conclusion
Studying the dynamics of coarse woody debris, from recruitment to retention to export, in headwater streams is difficult to undertake because of time constraints associated with these processes. Understanding CWD changes that may take place in both riparian and stream environments may take decades, if not centuries. This study provided an opportunity to examine CWD content in both medium and young-aged forest stands and to evaluate the role of BMP riparian buffers on CWD dynamics. Moreover, the multi-decade study allowed us to examine the influence of flooding events on CWD distribution between treatments. The no BMP treatment demonstrated once again that anthropogenic disturbance from logging contributed CWD to both the riparian area and the stream. A flood event in 2004 transported most of the stream and riparian CWD in each watershed and essentially “reset” the system with equally low amounts of CWD by treatment. Although CWD recruitment was observed in all treatments after 2004, only the control and BMP treatments saw a significant increase. This is likely a function of stand age. Trees in the no BMP treatment were relatively young in 2020 (37 years), so recruitment levels were low due to the health of the trees and lack of major windstorms or disease/infestations. The BMP treatment, on the other hand, had older trees retained in the riparian area that were of similar age as the control (100+ years) and were likely contributing more CWD due to aging. Still, the 100 years-old mixed hardwood forest was healthy and recruitment levels were relatively low. If long-term maintenance of CWD recruitment levels are important for forest and stream management decisions, then use of riparian BMPs during logging operations may help maintain those functions.
Data availability statement
The raw data supporting the conclusions of this article will be made available by the authors, without undue reservation.
Author contributions
KS: investigation, data curation, writing—original draft, writing—review and editing, and visualization. JF: investigation, data curation, and writing—original draft. WL: formal analysis and writing—review and editing. RK: conceptualization, methodology, funding acquisition, and writing—review and editing. CB: conceptualization, investigation, data curation, supervision, project administration, funding acquisition, and writing—review and editing. All authors contributed to the article and approved the submitted version.
Funding
This work was partially supported by the National Institute of Food and Agriculture, U.S. Department of Agriculture, McIntire-Stennis Research Program (Accession Number 1005547). Additional funding was provided by the U.S. Forest Service Northern Research Station (Award # 09-JV-11242307-111).
Acknowledgments
The authors gratefully acknowledge the many technicians, undergraduate students, and graduate students who conducted CWD surveys over the years, including Merril Flannery, Nic Eiche, Ryan Quite, Forrest Miller, Felicity Shirkey, Julia Maugans, John Barton, and William Wittenbraker. The authors also thank David Collett for help collecting streamflow data over the years. Wesley Staats and Marco Contreras contributed figures and analysis from a 2013 LiDAR study. KS thanks his wife Susanna Sena, whose unpaid domestic labor made his contributions to this project possible.
Conflict of interest
The authors declare that the research was conducted in the absence of any commercial or financial relationships that could be construed as a potential conflict of interest.
Publisher’s note
All claims expressed in this article are solely those of the authors and do not necessarily represent those of their affiliated organizations, or those of the publisher, the editors and the reviewers. Any product that may be evaluated in this article, or claim that may be made by its manufacturer, is not guaranteed or endorsed by the publisher.
Footnotes
1. ^To convert cubic feet per second (cfs) to cubic meters per second (cms), multiple by 0.0283.
References
Arthur, M. A., Coltharp, G. B., and Brown, D. L. (1998). Effects of best management practices on forest streamwater quality in eastern Kentucky. J. Am. Water Resour. Assoc. 34, 481–495. doi: 10.1111/j.1752-1688.1998.tb00948.x
Aust, W. M., and Blinn, C. R. (2004). Forestry best management practices for timber harvesting and site preparation in the eastern United States: an overview of water quality and productivity research during the past 20 years (1982–2002). Water Air Soil Pollut. 4, 5–36. doi: 10.1023/B:WAFO.0000012828.33069.f6
Bowker, D., Stringer, J., and Barton, C. (2020). Influence of timber harvesting operations and streamside management zone effectiveness on sediment delivery to headwater streams in Appalachia. Forests 11:623. doi: 10.3390/f11060623
Burton, J. I., Olson, D. H., and Puettmann, K. J. (2016). Effects of riparian buffer width on wood loading in headwater streams after repeated forest thinning. For. Ecol. Manag. 372, 247–257. doi: 10.1016/j.foreco.2016.03.053
Everett, R. A., and Ruiz, G. M. (1993). Coarse woody debris as a refuge from predation in aquatic communities: an experimental test. Oecologia 93, 475–486. doi: 10.1007/BF00328954
Fausch, K. D., and Northcote, T. G. (1992). Large woody debris and salmonid habitat in a small coastal British Columbia stream. Can. J. Fish. Aquat. Sci. 49, 682–693. doi: 10.1139/f92-077
Forrester, J. A., Mladenoff, D. J., Gower, S. T., and Stoffel, J. L. (2012). Interactions of temperature and moisture with respiration from coarse woody debris in experimental forest canopy gaps. For. Ecol. Manag. 265, 124–132. doi: 10.1016/j.foreco.2011.10.038
Fraver, S., Milo, A. M., Bradford, J. B., D’Amato, A. W., Kenefic, L., Palik, B. J., et al. (2013). Woody debris volume depletion through decay: implications for biomass and carbon accounting. Ecosystems 16, 1262–1272. doi: 10.1007/s10021-013-9682-z
Fritz, K. M., Pond, G. J., Johnson, B. R., and Barton, C. D. (2019). Coarse particulate organic matter dynamics in ephemeral tributaries of a Central Appalachian stream network. Ecosphere 10:e02654. doi: 10.1002/ecs2.2654
Gangloff, M. M., Perkins, M., Blum, P. W., and Walker, C. (2015). Effects of coal mining, forestry, and road construction on southern Appalachian stream invertebrates and habitats. Environ. Manag. 55, 702–714. doi: 10.1007/s00267-014-0429-1
Gurnell, A. M., Gregory, K. J., and Petts, G. E. (1995). The role of coarse woody debris in forest aquatic habitats: implications for management. Aquat. Conserv. 5, 143–166. doi: 10.1002/aqc.3270050206
Harmon, M. E., Franklin, J. F., Swanson, F. J., Sollins, P., Gregory, S. V., Lattin, J. D., et al. (1986). Ecology of coarse woody debris in temperate ecosystems. Adv. Ecol. Res. 15, 133–302. doi: 10.1016/S0065-2504(08)60121-X
Holmes, K. L., Goebel, P. C., and Morris, A. E. L. (2010). Characteristics of downed wood across headwater riparian ecotones: integrating the stream with the riparian area. Can. J. For. Res. 40, 1604–1614. doi: 10.1139/X10-106
Idol, T. W., Figler, R. A., Pope, P. E., and Ponder, F. Jr. (2001). Characterization of coarse woody debris across a 100 year chronosequence of upland oak–hickory forests. For. Ecol. Manag. 149, 153–161. doi: 10.1016/S0378-1127(00)00536-3
Jenkins, M. A., Webster, C. R., Parker, G. R., and Spetich, M. A. (2004). Coarse woody debris in managed central hardwood forests of Indiana, USA. For. Sci. 50, 781–792. doi: 10.1093/forestscience/50.6.781
Jia-Bing, W., De-Xin, G., Shi-Jie, H., Mi, Z., and Chang-Jie, J. (2005). Ecological functions of coarse woody debris in forest ecosystem. J. For. Res. 16, 247–252. doi: 10.1007/BF02856826
Jones, J. M., Heath, K. D., Ferrer, A., Brown, S. P., Canam, T., and Dalling, J. W. (2019). Wood decomposition in aquatic and terrestrial ecosystems in the tropics: contrasting biotic and abiotic processes. FEMS Microbiol. Ecol. 95:fiy223. doi: 10.1093/femsec/fiy223
Loeb, S. C. (1996). “The role of coarse woody debris in the ecology of southeastern mammals” in General technical report (Southern Research Station, USDA Forest Service), 108–118.
McCarthy, B. C., and Bailey, R. R. (1994). Distribution and abundance of coarse woody debris in a managed forest landscape of the Central Appalachians. Can. J. For. Res. 24, 1317–1329. doi: 10.1139/x94-172
McClure, J. M., Kolka, R. K., and White, A. (2004). Effect of forest harvesting best management practices on coarse woody debris distribution in stream and riparian zones in three Appalachian watersheds. Water Air Soil Pollut. 4, 245–261. doi: 10.1023/B:WAFO.0000012815.30596.97
McDade, B., Martin, D. J., Van De Gevel, S. L., and Mitchell, J. (2020). Impacts of the hemlock woolly Adelgid (Adelges tsugae) on headwater stream large woody debris loads in the southern Appalachian Mountains. Southeast. Geogr. 60, 65–86. doi: 10.1353/sgo.2020.0005
McMinn, J. W., and Crossley, D. A., (1993). Biodiversity and coarse woody debris in southern forests. Proceedings of the Workshop on Coarse Woody Debris in Southern Forests: Effects on Biodiversity. Athens, GA, October 18–20, 1993.
Merten, E. C., Vaz, P. G., Decker-Fritz, J. A., Finlay, J. C., and Stefan, H. G. (2013). Relative importance of breakage and decay as processes depleting large wood from streams. Geomorphology 190, 40–47. doi: 10.1016/j.geomorph.2013.02.006
Morrison, D. L., Nicholas, N. S., and Creed, I. F. (2004). Is coarse woody debris a net sink or source of nitrogen in the red spruce—Fraser fir forest of the southern Appalachians, U.S.A.? Can. J. For. Res. 34, 716–727. doi: 10.1139/X03-211
Muller, R. N., and Liu, Y. (1991). Coarse woody debris in an old-growth deciduous forest on the Cumberland Plateau, southeastern Kentucky. Can. J. For. Res. 21, 1567–1572. doi: 10.1139/x91-218
Nakamura, F., and Swanson, F. J. (1993). Effects of coarse woody debris on morphology and sediment storage of a mountain stream system in western Oregon. Earth Surf. Process. Landf. 18, 43–61. doi: 10.1002/esp.3290180104
Norden, B., Ryberg, M., Gotmark, F., and Olausson, B. (2004). Relative importance of coarse and fine woody debris for the diversity of wood-inhabiting fungi in temperate broadleaf forests. Biol. Conserv. 117, 1–10. doi: 10.1016/S0006-3207(03)00235-0
Oberle, B., Lee, M. R., Myers, J. A., Osazuwa-Peters, O. L., Spasojevic, M. J., Walton, M. L., et al. (2020). Accurate forest projections require long-term wood decay experiments because plant trait effects change through time. Glob. Change Biol. 26, 864–875. doi: 10.1111/gcb.14873
Otto, C. R. V., Kroll, A. K., and McKenny, H. C. (2013). Amphibian response to downed wood retention in managed forests: a prospectus for future biomass harvest in North America. For. Ecol. Manag. 304, 275–285. doi: 10.1016/j.foreco.2013.04.023
Overstreet, J. C. (1984). Robinson Forest inventory, 1980–1982 Department of Forestry, College of Agriculture, University of Kentucky, 52.
Parker, R. P., and Hart, J. L. (2014). Patterns of riparian and in-stream large woody debris across a chronosequence of southern Appalachian hardwood stands. Nat. Areas J. 34, 65–78. doi: 10.3375/043.034.0108
Phillips, E. C., and Kilambi, R. V. (1994a). Use of coarse woody debris by Diptera in Ozark streams, Arkansas. J. N. Am. Benthol. Soc. 13, 151–159. doi: 10.2307/1467234
Phillips, E. C., and Kilambi, R. V. (1994b). Utilization of coarse woody debris by Ephemeroptera in three Ozark streams of Arkansas. Southwest. Nat. 39, 58–62. doi: 10.2307/3672193
R Core Team (2021). R: a language and environment for statistical computing. R Foundation for Statistical Computing, Vienna, Austria. Available at: https://www.R-project.org/.
Rubino, D. L., and McCarthy, B. C. (2003). Evaluation of coarse woody debris and forest vegetation across topographic gradients in a southern Ohio forest. For. Ecol. Manag. 183, 221–238. doi: 10.1016/S0378-1127(03)00108-7
Sena, K. L., Williamson, T. N., and Barton, C. D. (2021). The Robinson Forest environmental monitoring network: long-term evaluation of streamflow and precipitation quantity and stream-water and bulk deposition chemistry in eastern Kentucky watersheds. Hydrol. Process. 35:e14133. doi: 10.1002/hyp.14133
Siderhurst, L. A., Griscom, H. P., Hudy, M., and Bortolot, Z. J. (2010). Changes in light levels and stream temperatures with loss of eastern hemlock (Tsuga canadensis) at a southern Appalachian stream: implications for brook trout. For. Ecol. Manag. 260, 1677–1688. doi: 10.1016/j.foreco.2010.08.007
Sweka, J. A., and Hartman, K. J. (2006). Effects of large woody debris addition on stream habitat and brook trout populations in Appalachian streams. Hydrobiologia 559, 363–378. doi: 10.1007/s10750-005-9117-8
Tirpak, J. M., Giuliano, W. M., Miller, C. A., Allen, T. J., Bittiner, S., Edwards, J. W., et al. (2006). Ruffed grouse nest success and habitat selection in the central and southern Appalachians. J. Wildl. Manag. 70, 138–144. doi: 10.2193/0022-541X(2006)70[138:RGNSAH]2.0.CO;2
Villines, J. A., Agouridis, C. T., Warner, R. C., and Barton, C. D. (2015). Using GIS to delineate headwater stream origins in the Appalachian coalfields of Kentucky. J. Am. Water Resour. Assoc. 51, 1667–1687. doi: 10.1111/1752-1688.12350
Wang, J. X., LeDoux, C. B., Edwards, P., and Jones, M. (2005). Soil bulk density changes caused by mechanized harvesting: a case study in central Appalachia. For. Prod. J. 55, 37–40.
Webster, C. R., and Jenkins, M. A. (2005). Coarse woody debris dynamics in the southern Appalachians as affected by topographic position and anthropogenic disturbance history. For. Ecol. Manag. 217, 319–330. doi: 10.1016/j.foreco.2005.06.011
Williamson, T. N., and Barton, C. D. (2020). Hydrologic modeling to examine the influence of the forestry reclamation approach and climate change on mineland hydrology. Sci. Total Environ. 743:140605. doi: 10.1016/j.scitotenv.2020.140605
Keywords: timber harvest, hydrology, forest management, streamside management zone, BMPs
Citation: Sena KL, Flynn JK, Leuenberger W, Kolka R and Barton CD (2023) Long-term changes in coarse woody debris abundance in three Appalachian headwater streams with differing best management practices. Front. For. Glob. Change. 6:1242878. doi: 10.3389/ffgc.2023.1242878
Edited by:
Barry Alan Gardiner, Institut Européen De La Forêt Cultivée (IEFC), FranceReviewed by:
Salli F. Dymond, Northern Arizona University, United StatesMark Ford, United States Department of the Interior, United States
Copyright © 2023 Sena, Flynn, Leuenberger, Kolka and Barton. This is an open-access article distributed under the terms of the Creative Commons Attribution License (CC BY). The use, distribution or reproduction in other forums is permitted, provided the original author(s) and the copyright owner(s) are credited and that the original publication in this journal is cited, in accordance with accepted academic practice. No use, distribution or reproduction is permitted which does not comply with these terms.
*Correspondence: Kenton L. Sena, a2VudG9uLnNlbmFAdWt5LmVkdQ==