- 1Department of Wood and Forest Sciences, Research Centre on Renewable Materials, Université Laval, Quebec City, QC, Canada
- 2Department of Wood and Forest Sciences, FORAC Research Consortium, Université Laval, Quebec City, QC, Canada
- 3Quebec Office of the Chief Forester, Caplan, QC, Canada
Management of boreal forests can increase terrestrial carbon sinks and reduce greenhouse gas (GHG) emissions to the atmosphere. A case study was conducted in the boreal balsam fir forests of Quebec (Canada), a commercially important region for forestry, to identify optimal management and wood production solutions that contribute to reducing GHG emissions to the atmosphere. Scenarios were based on a steady level of harvest and silvicultural activities over time and a stable flow of wood products to markets. Scenarios included: increases and decreases in the volume of harvested timber; the transition of harvesting activities from clearcuts (the most common practice in the region) to partial cuts; and changes in the rate of natural regeneration (the usual mode of regeneration) vs. plantations. All scenarios provided a carbon sink regardless of the time frame. Compared with other scenarios, reducing harvest levels increased the forest carbon sink in the short (10 to 20 years) and medium (20 to 50 years) terms. Also, for a similar harvest level, the increased use of partial cutting and planting increased the forest carbon sink. In the long term (over 50 years), strategies with low harvesting levels resulted in lower ecosystem carbon sequestration, even though they still had the lowest cumulative emissions. Nevertheless, higher harvesting levels could not be justified because the long-term increase in the forest ecosystem carbon sink could not offset higher emissions from wood products, particularly from short-lived paper products. Sensitivity analyses showed that improving sawmill efficiency and thus increasing the proportion of long-lived products was an important factor that can greatly reduce emissions. On the other hand, transportation distances between forest stands and sawmills had a relatively marginal impact on the overall carbon balance of forest management and wood production scenarios.
1 Introduction
In the context of global warming, forests and wood products can play an essential role in reducing greenhouse gas (GHG) emissions to the atmosphere (Nabuurs et al., 2022). Mitigation options for the forestry sector include activities that (i) maintain or increase ecosystem carbon stocks through afforestation/reforestation, forest management, restoration or conservation, (ii) store carbon in long-lived wood products, and (iii) promote the use of wood products that have a low GHG footprint associated with their production and manufacturing to replace non-renewable, GHG-intensive materials such as steel and concrete (Nabuurs et al., 2022).
In Quebec, a province of eastern Canada, the boreal forest under management represents 320,000 km2, for which approximately 90% is under public tenure. Public forests are regulated by the Quebec Sustainable Forest Territory Management Act (Loi sur l’aménagement durable du territoire forestier chapitre A.-18.1). Under this Act, it is required that sustainable forest management meet multiple objectives such as conservation of biodiversity, protection of soils and water, harmonization of forest uses, maintenance or increase of forest productivity, consideration of the socio-economic benefits from the forest, and contribution of the forest to ecological processes. Governmental authorities consider these objectives when calculating the annual allowable cut (AAC) for all forest areas under public tenure. Moreover, in response to societal and political demands for GHG reduction and climate change mitigation, there is an increasing need to quantify impacts on the carbon balance of forest management strategies as determined by the AAC process and consider these impacts in decision-making.
When analyzing the impacts of a management strategy on the carbon balance, it is essential to use a system perspective that includes not only the forest but also the wood products that are generated (Hennigar et al., 2008; Lemprière et al., 2013). For example, management strategies that reduce carbon stocks in the forest, but allow for high carbon storage in long-lived wood products and a high substitution effect of GHG-intensive, non-renewable products on markets, can yield an overall favorable carbon balance (Perez-Garcia et al., 2005). This points out the importance of considering carbon fluxes from the forest ecosystems, the industrial network that processes the wood supply from forests, and the markets that consume wood products.
In the boreal forest of Quebec, clearcut harvesting with the protection of advanced natural regeneration and soils is the regular practice. In most cases, natural regeneration is sufficient and artificial regeneration through planting is unnecessary. However, evidence suggests clearcut harvest can turn the forest ecosystem into a net source of carbon emissions for a decade or more, and it may take several years for the system to become a net carbon sink, even when considering wood products (Paradis et al., 2019). Alternative forest management strategies that can reduce carbon emissions and increase carbon uptake in forests include the use of extended clearcut rotation length, planting, partial cut (as opposed to clearcut), and conservation (Pukkala, 2014; Smyth et al., 2014; Paradis et al., 2019).
Extending the rotation length, i.e., the time between harvest operations, allows for accumulating slow-decomposing downed woody debris (Liski et al., 2001; Paradis et al., 2019). It also lets trees grow for a longer period and reach larger diameters. Such a strategy can thus contribute to producing a lower share of wood chips dedicated to paper products, i.e., short-lived products, and a higher percentage of sawnwood products, which can store carbon for a longer period (Paradis et al., 2019). However, longer rotations can reduce the total harvested volume over a landscape. For its part, partial cut, which removes 1/3 to 2/3 of the stand basal area and therefore creates an irregular vertical structure while limiting soil disturbance and preserving a forest cover, has the potential to reduce emissions associated with harvesting (Ameray et al., 2021). Meanwhile, plantation can shorten regeneration delay, reduce the time before a stand becomes a net carbon sink, and sequester more carbon than a naturally regenerated stand (Colombo et al., 2005). High timber production in plantations can also reduce the harvested area and allow other mitigation activities, such as conservation, to be implemented (Nabuurs et al., 2022).
Forest conservation strategies can have a high mitigation potential in the short term, especially for young, growing forests when the carbon sequestration rate of the conserved forest is high. In Finland, Pukkala (2014) concluded that the carbon balance of unmanaged forests (i.e., in the absence of harvest) was superior to that of managed forests for about 120 to 130 years; however, after this point, the carbon balance of managed forests was higher. Similarly, Harel et al. (2021) found that boreal old-growth balsam fir forests, although large carbon stocks, were neither an active sink nor a carbon source. On the other hand, some studies have shown that some types of old forests can continue to be a carbon sink for centuries (Pan et al., 2011); it is notably the case for boreal black spruce forests of northern Quebec, which can remain a carbon sink for centuries in the absence of wildfire (Andrieux et al., 2018). However, strategies based on forest conservation can be more vulnerable to natural disturbances (wildfires, insect epidemics, windthrows) and thus to the release of carbon; such strategies also provide lower quantities of wood products (Hennigar et al., 2008; Ter-Mikaelian et al., 2008). Therefore, the type of ecosystem, the time horizon and the accounting scope (e.g., ecosystems only, or ecosystems + products) are important variables when analyzing the impact of a management strategy on the carbon balance (Perez-Garcia et al., 2005; Pukkala, 2017).
Forest management strategies applied at the landscape scale may include a mix of the abovementioned practices. Optimal combinations of these practices over time and the landscape will influence the quantity, nature, and spatial and temporal distribution of wood supply. This, in turn, will affect the GHG emissions associated with wood extraction, transportation and manufacturing into wood products. The configuration and efficiency of the industrial wood-processing network to which the wood supply is sent will also influence the carbon balance. Emissions from forest operations, wood transportation and manufacturing are often thought to be marginal relative to the forest ecosystem fluxes and other GHG sources. For example, the ecosystem carbon uptake associated with intensive forestry is expected to more than offset the emissions related to machinery operations (Markewitz, 2006). In the United States, Hudiburg et al. (2019) found that emissions from harvest, transportation and manufacturing accounted for less than 10% of all wood product emissions; on the other hand, the decay of wood products during their lifespan was the main emission contributor.
For a given harvested wood volume, distinct management strategies, e.g., large, concentrated clearcut blocks vs. scattered partial cuts, will influence the distance traveled within the forest area and to the processing facilities. The choice of mills to which timber is destined is usually based on geographic attributes such as mill locations, but also on log characteristics, such as species and size, and economic factors such as processing efficiency and capacity (Rijal et al., 2018). In turn, timber destinations will have an impact on emissions related to transportation and product manufacturing and use. In the context of the boreal forest of Quebec, the extent to which decisions related to management strategies, wood transportation and product manufacturing influence the overall carbon balance of forest management and wood production remains to be quantified.
This study aimed to identify optimal forest management and wood production solutions for the boreal forest that can provide a sustained yield and a steady flow of wood products to markets over time while also contributing to reducing carbon emissions to the atmosphere. Using balsam fir (Abies balsamea (L.) Mill) – white birch (Betula papyrifera Marsh) forests as a case study, which are commercially important and abundant in the boreal area of Quebec and Canadian Atlantic provinces, the objective was to compare and analyse the carbon balance (estimated in units of CO2 equivalent, CO2e) of scenarios of forest management, wood supply and product manufacturing based on strategic planning optimization of AAC. The carbon balance was calculated for the Forest Ecosystem-Harvested Wood Products system using the simple decay approach that reports atmospheric flows (Hashimoto, 2008). The carbon balance was thus defined to include fluxes from forest ecosystems (photosynthesis and heterotrophic and autotrophic respiration) and harvested wood products (including emissions from wood harvesting, transportation and manufacturing, and wood product decay over their service life and in landfills). Scenarios were defined based on the AAC, i.e., allowing a sustained yield and a steady level of harvest and silvicultural activities over time and the configuration of the wood-processing facilities. Scenarios varied according to harvested timber, the proportion of partial cut relative to clearcut, planting levels, and the configuration and efficiency of the wood-processing network. Relative effects of management scenarios on forest structure and ecosystem carbon stocks were assessed, and impacts of forest management, transportation distances and wood processing efficiency on the carbon balance were compared.
2 Materials and methods
2.1 Study area
The study area was the Montmorency Forest (47°19′19.6″N 71°08′49.6″W), the experimental forest research station of Université Laval. This area covers 397 km2 (39,700 ha) and is located in the eastern boreal forest of Quebec in the balsam fir-white birch bioclimatic domain (Figure 1). The average annual daily temperature is 0.5°C, and the yearly precipitations are 1,583 mm (Environment Canada, 2021). The forest structure is primarily shaped by periodic spruce budworm epidemics and windthrow, as wildfires are rare in this area (Leblanc and Bélanger, 2000). Several forest types are found across the area; most stands are composed of balsam fir, accompanied by white birch and, to a lesser extent, by white spruce (Picea glauca (Moench) Voss), black spruce (Picea mariana (Mill.) BSP), and trembling aspen (Populus tremuloïdes Michx).
In 2020, 45% of the Montmorency Forest was in the 30 years age class (21 to 40 years), while 30% was considered as an old forest (90+ year age class, based on the definition of old balsam fir forests of Couillard et al. (2013)). In business-as-usual operations, clearcut harvesting with the protection of advanced regeneration and soils is the primary type of harvest, while partial cuts account for about 10% of all harvests. While most harvested sites are regenerated naturally (following the provincial regulations that require the promotion of natural regeneration), enrichment planting of white and black spruce, at a rate of 500 to 1,500 stems ha−1, is used on sites when natural regeneration is not sufficient after clearcutting (Thiffault et al., 2013).
2.2 Study framework
Our study estimated the net CO2e balance of different forest management strategies in the Montmorency Forest, including: (i) ecosystem fluxes, (ii) emissions from harvesting, transportation and manufacturing of wood products, and (iii) the decay of wood products during their service life and in landfills. We based our definition of mitigation in the forestry sector on the simple decay approach as described in the 2006 IPCC Guidelines for National Greenhouse Gas Inventories (IPCC, 2006). In this approach, all product emissions are reported by the wood-producing jurisdiction, regardless of where they occur, i.e., decay emissions from all wood products sourced from the area are reported, even if they are exported, and the focus is on atmospheric fluxes.
2.3 Modeling
Forest management scenarios were modeled with the Woodstock timber supply software (Remsoft, 2006). All inputs (yield curves, forest characteristics, zoning) required to run the model were provided by the Quebec Office of the Chief Forester. The Woodstock model “grows” forest strata (i.e., aspatial clusters of forest polygons with common attributes) from yield curves for a defined period. Woodstock also simulates senescence, harvesting and several other silvicultural treatments. An objective function is used to maximize total harvested timber. Constraints are added to meet legal obligations such as those included in the Quebec Sustainable Forest Territory Management Act (chapter A.-18.1) and to specify levels of silvicultural treatments associated with a given management strategy. The model is optimized by the MOSEK linear programming solver (Andersen and Andersen, 2000). The result is an optimized intervention schedule that ensures a sustained wood yield over time, which is then used to determine the species-wise annual allowable cut (AAC) for the territory. The model did not include natural disturbances and climate projections, as they have yet to be routinely and explicitly included in Quebec AAC calculations.
Harvest operations included clearcut, i.e., removal of 99% of merchantable timber (Poulin, 2013a), and three types of partial cut: (i) commercial thinning (33% removal of merchantable timber) (Poulin, 2013b), (ii) extended irregular shelterwood (40% removal of merchantable timber) (Raymond et al., 2009), and (iii) continuous cover irregular shelterwood (35% removal of merchantable timber) (Poulin, 2013c), the first two types being eventually followed by a clearcut, while the latter being followed by other partial removals. Sequences of silvicultural treatments (the most common presented in Table 1) applied to strata were based on the dominant species targeted, the desired stand structure and the silvicultural intensity. Strata evolution followed yield curves generated by the Office of the Chief Forester for the AAC calculation in the eastern balsam fir – white birch sub-bioclimatic domain (Bureau du forestier en chef, 2018); curves were generated by the Natura forest growth model (Auger, 2017), according to strata composition and silvicultural treatments. Yield tables from Prégent et al. (1996) and Prégent et al. (2010) were used for black and white spruce plantations. Following commercial thinning, stand increment (m3 ha−1 year−1) was maintained to reflect an increase in the diameter growth of residual trees. Stand increment (m3 ha−1 year−1) following irregular shelterwood harvest assumed no change in the diameter growth rate of residual trees (Bureau du forestier en chef, 2018).
2.4 Forest management strategies
Management strategies were based on different combinations of silvicultural treatments applied across landscapes and time. Seven management scenarios were modeled, including a Business-as-usual scenario (Bau) (Table 2). Generic constraints imposed by the Quebec Sustainable Forest Territory Management Act were applied to all scenarios (Bureau du forestier en chef, 2018) (except Natural Evolution, see below); for example, this includes the prohibition of harvesting in areas identified as habitats for caribou and other vulnerable or endangered species, the maximum proportion of stands with a height of less than 7 m over the landscape, and requirements for the protection and the restoration of old-growth forests. The Bau scenario is representative of common operational practices in the boreal forest areas under public tenure in Quebec; it includes the average amount of planting and proportion of partial cutting generally performed in Quebec balsam fir landscapes and reflects the goal of generating the highest AAC while meeting all constraints imposed by the Quebec Sustainable Forest Territory Management Act. Alternative forest management scenarios were defined relative to the Bau. They included: (i) a decrease or an increase in harvested volumes per year, (ii) an increase in planting and associated tending activities (as opposed to reliance on natural regeneration), and/or (iii) an increase in the use of partial cut (as opposed to clearcut harvest). A Natural Evolution scenario, i.e., a total conservation scenario with no management or harvesting (and no natural disturbances, as these were excluded from all scenarios), was also included for comparison. All scenarios were run over a 150 years planning horizon starting in 2015, but only results up to the 2115 timeframe are presented as results become more uncertain in the more distant future.
2.5 Carbon dynamics of forest ecosystems
Forest ecosystem carbon fluxes for modeled scenarios, expressed in multiples of tonnes of CO2-equivalent (CO2e), were estimated using the Generic Carbon Budget Model (GCBM), a spatially explicit modeling environment (Smyth et al., 2020). GCBM is the latest version of the Carbon Budget Model of the Canadian Forest Sector (CBM-CFS3) (Kurz et al., 2009) and is based on the same parameters, equations and pools. Based on stand yield data, this model tracks the annual evolution of carbon stocks and fluxes in above- and belowground living biomass and dead organic matter (including soil carbon). Disturbances such as harvest are simulated based on matrices that describe how carbon is transferred among ecosystem pools and toward harvested wood products. The model inputs and parameters combine spatial layers such as forest age and tree species and aspatial layers such as yield curves (Smyth et al., 2020). The initialization procedure described in Kurz et al. (2009) was applied, whereby initial carbon stocks are estimated by simulating recurring wildfires until C stocks in slow-cycling dead organic matter pools have stabilized, followed by the more recent disturbances recorded in the provincial forest inventory. The Forest Management Tools (FMT), an open-source C++ library developed by the Office of the Chief Forester, then served to process the initial carbon map information and the optimized intervention schedule from Woodstock into rasters suitable for GCBM (FMT library available at https://github.com/Bureau-du-Forestier-en-chef). While Woodstock provides intervention schedules using 5 years time steps and considers the detailed species composition of stands, FMT translates Woodstock outputs into yearly time steps and combines species into softwood and hardwood groups (assigning to each group the wood density of the most abundant species of the group, i.e., balsam fir and white birch, respectively). The GCBM model was then run for 100 years using 1,000 Monte Carlo iterations and a pixel resolution of 14 ha.
2.6 Carbon dynamics of harvested wood products
Wood supply from harvest was simulated to circulate within the industrial wood-processing network based on common practices in the studied region. Harvested volumes were only defined in terms of species groups (softwood and hardwood), as information about tree/log size distribution and log quality is not tracked by the model. Softwood logs were assumed to be sent to sawmills; sawmill co-products (wood chips and sawdust) were directed to pulp production. The resulting basket of wood products was based on the average sawmilling efficiency for the province of Quebec (Beauregard et al., 2019) (Figure 2). All hardwood logs were sent directly to pulp production, according to the current practice in the study region.
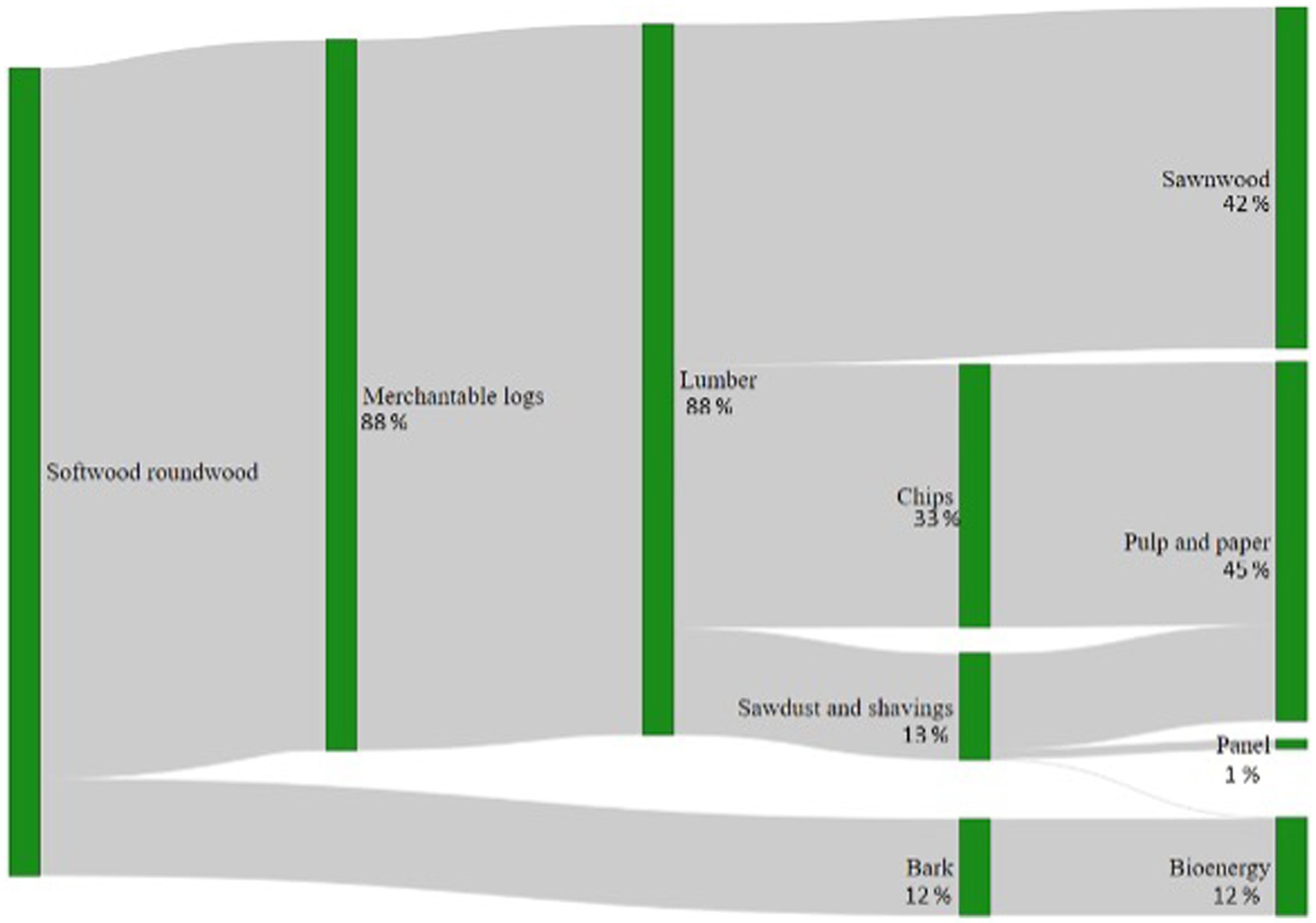
Figure 2. Basket of wood products from softwood species harvested in Montmorency Forest, based on Quebec provincial average (Beauregard et al., 2019).
The carbon in harvested wood products was then tracked over 100 years (2015–2115) with the Carbon Budget Modeling Framework for Harvested Wood Products (CBM-FHWP). This model uses a conceptual flow network to simulate carbon dynamics in products and emissions from decay based on half-life parameters for wood products (Environment and Climate Change Canada, 2023). We used default half-lives of 35 years for sawnwood, 25 years for panels and 2 years for pulp and paper (IPCC, 2016). Bioenergy from tree bark was assumed to be used internally as bio-heat within sawmills; any bioenergy emission was thus included in sawnwood manufacturing emissions (see below).
After the retirement of sawnwood and panels, 99% was assumed to be sent to landfills, while the rest was assumed to be incinerated; in landfills, 77% was assumed not to be degradable, while the other 23% was degradable with a half-life of 29 years (IPCC, 2006). For pulp and paper, the proportions were 93% to landfills and 7% to incineration; 44% of the landfilled material was assumed to be not degradable, while the remaining 56% was assumed to be degradable with a half-life of 14.5 years (IPCC, 2006). Details about landfill management and dynamics of emissions in landfills were based partly on Head et al. (2021), Smyth et al. (2014) and expert opinion. Degradation in landfills was assumed to cause 50% CO2 and 50% CH4 emissions. Products at their end-of-life could be sent to two types of landfills (reflecting the fact that products would end up either in landfills in Quebec or elsewhere). It was assumed that discarded products were sent to the first type of landfill according to the following proportions: 61.4% of sawnwood, 44.3% of pulp and paper products, and 58% of panels; in those landfills, CH4 emissions were either directly released to the atmosphere (66%), captured for flaring (16.8%), or for energy production (17.2%), in both cases with carbon then emitted as 99.7% CO2 and 0.3% CH4. The rest of the discarded products were assumed to be sent to the other type of landfill in which 32.5% of CH4 was released to the atmosphere, 10.62% was flared, and 56.8% was used for energy production.
No evolution or innovation in wood processing, product manufacturing, or end-of-life management that may impact the life cycle of products was included over the simulation period.
Both CO2 and CH4 emissions were compiled and expressed in tonnes of CO2e, with a global warming potential for a 100 years horizon (GWP100) of 25 for CH4 (Forster et al., 2007). To facilitate comparisons of scenarios, the pool of wood products was assumed to be empty at the beginning of the simulation period (2015), i.e., we did not track emissions associated with wood products from previous years as they were assumed to be the same for all scenarios.
We also calculated the carbon emissions from wood extraction, transportation and manufacturing. For extraction and transportation, we used an average emission rate of 9.9 and 8.68 kg CO2e m−3 for all harvested wood; this assumes the same transport distance, i.e., 90 km from forest to the mill, based on an average from Eastern Canada calculated in Athena Sustainable Materials Institute (2018) for all harvested wood. Emissions related to manufacturing were then computed for each product. For sawnwood, 20.48 kgCO2e m−3 was assumed to be emitted (Athena Sustainable Materials Institute, 2018). For panels, emissions associated with manufacturing were estimated at 64.94 kgCO2e m−3 (average of plywood and oriented strand board) (Athena Sustainable Materials Institute, 2018). For pulp and paper manufacturing, we assumed that a tonne of paper contains 0.43 tonne of carbon (Skog, 2008) and used an emission factor of 250 kgCO2e per tonne of paper, based on values calculated for Nordic countries, which have a similar energy portfolio to that of Quebec (Sun et al., 2018).
2.7 Sensitivity analyses
Sensitivity analyses were performed to assess the impact on the carbon balance of (i) sawmilling efficiency and (ii) the transport distance from forest to mills. For these two parameters, different values were simulated (keeping other parameters stable), and results were compared.
2.7.1 Sawmilling efficiency
First, the effect of an improved sawmilling efficiency yielding a higher share of sawnwood from harvested conifer species was tested. Thus, for the “Increased efficiency” scenario, a proportion of 53% of sawnwood was assumed to be generated from softwood logs (based on Paradis et al., 2019) instead of 42%, thereby reducing the share of wood ending up as pulp and paper products from 45 to 34%.
2.7.2 Transportation distances
Second, we tested the impact of transport distances on the carbon balance. For this assessment, instead of using the average transportation emissions described above, we modeled the Montmorency Forest industrial network using Logilab to get more detailed estimates of transport distances and related emissions. Logilab is a spatially explicit linear optimization software developed by the FORAC Research Consortium and described in Boukherroub et al. (2017). Our study used Logilab as a simulation tool rather than an optimization tool by forcing the model with constraints to ensure that logs were sent to the desired sawmills.
The Woodstock solutions of management scenarios were disaggregated using a simplified version of the methodology presented by Cantegril et al. (2019), to obtain the annual wood supply per species and product. We then modeled two industrial networks based on the current and potential buyers of the wood harvested from the Montmorency Forest (See Figure 1 for sawmills locations). In the Close scenario, all harvested logs were assumed to be sent to the nearest mill (i.e., Sawmill #1). In the Far scenario, sawmill-grade hardwood logs were sent to Sawmill #1, softwood logs were sent to Sawmill #2 and pulp and paper-grade hardwood logs were sent to Sawmill #3, thereby increasing the average transport distances from forest to sawmills. For each industrial network scenario, transport distances were then calculated from the centroid of each of the 14 management units of the Montmorency Forest to each sawmill based on existing forest and public roads.
Emission factors for the transportation of wood from each harvested species, which consider specific wood densities (Table 3), were then used in conjunction with specific transport distances for harvested wood to estimate transportation emissions for each combination of forest management and industrial network scenarios.
3 Results
3.1 Age structure
In 2020 (results not shown), the age structure distribution in the area under forest management, irrespective of the scenario, was 7% in the 10 years age class, 45% in the 30 years age class, 12% in the 50 years age class, 6% in the 70 years age class and 30% in the 90+ year age class. In 2050 (Figure 3A), for all scenarios, the 30 years age class was much less represented, with the 50 and 70 years age classes being the largest, followed by old forests in the 90+ year age class. By 2110, the 90+ year age class accounted for most of the area, followed by the 70 years age class (Figure 3B).
The management strategy had an impact on the age structure of the forest. For instance, the Natural Evolution scenario was only composed of old forests in 2110; scenarios with reduced harvest levels also had a higher proportion of forests in the +90 years age class than other scenarios. Scenarios with increased use of partial cut also had a higher proportion of forests in the 70 years age class. On the other hand, the Business-as-usual and the scenario with increased harvest had slightly higher proportions of young forests (10- and 30 years age classes) than other scenarios.
3.2 Ecosystem carbon stocks
The management strategy influenced ecosystem carbon stocks, but the differences were subtle (Figure 4). Soil carbon stocks remained stable over the simulation period and were similar for all scenarios, at about 87 tonnes of C per hectare (tC ha−1). Also, total ecosystem carbon stocks increased for all scenarios between 2020 and 2110. However, the Bau, Reduced harvest, Increased harvest + increased partial cut + increased planting and Natural Evolution scenarios saw a slight decrease in total carbon stock in the last years of the simulation. The Bau scenario had a total carbon stock of 214 tC ha−1 at the end of the simulation. Only the Increased harvest + increased partial cut + increased planting scenario had a lower C stock at 211 tC ha−1. The Natural Evolution scenario had the highest total C stock at the end of the simulation (244 tC ha−1), which was 29 tonnes higher than the Bau scenario. For its part, the Increased partial cut + increased planting scenario had a total carbon stock of 223 tC ha−1 at the end of the simulation, i.e., 9 tC more than the Bau scenario.
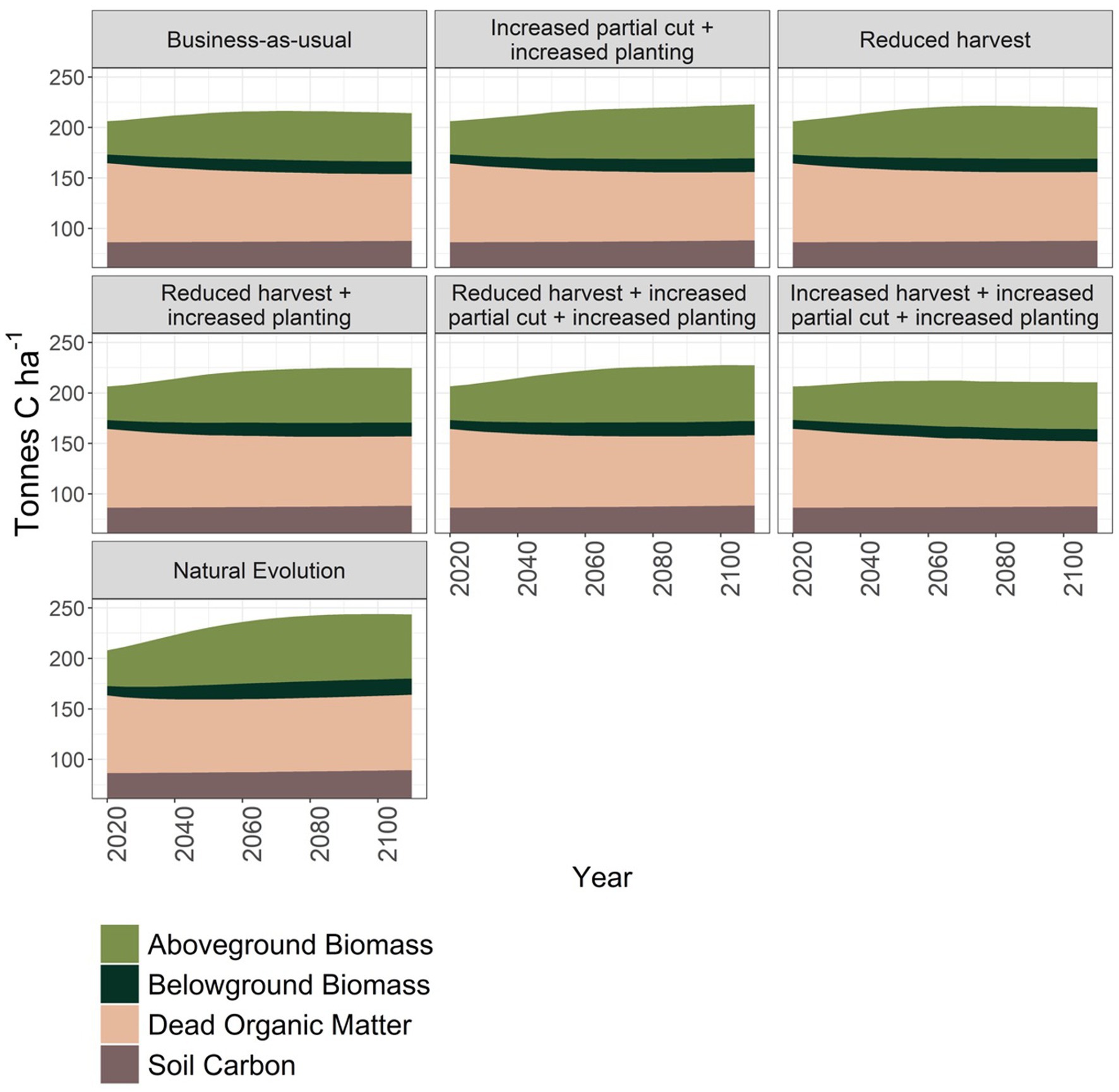
Figure 4. Evolution of ecosystem carbon stocks in tonnes of carbon per hectare (tonnes C ha−1) for the seven scenarios over a 95 years horizon. Deadwood and litter were combined in the dead organic matter pool. Stocks are presented as an annual average per 5 years period.
The three scenarios with similar (but lower than the Bau) harvesting levels (Reduced harvest, Reduced harvest + increased planting, Reduced harvest + increased partial cut + increased planting) had total carbon stocks of 220, 225, and 227 tC ha−1 in 2110, respectively. This result indicates that throughout the simulation, for a similar harvesting level, a higher proportion of partial cut caused an overall increase of total ecosystem carbon stock of 2 tC ha−1. In contrast, greater plantation use caused a rise of 5 tC ha−1. The aboveground biomass pool increased for each scenario during the simulation. The largest increase was in the Natural Evolution scenario (28 tC ha−1), and the smallest was in the Increased harvest + increased partial cut + increased planting scenario (14 tC ha−1). This pool was responsible for most of the variation among scenarios. For its part, the belowground biomass pool averaged only about 5.6% of the total carbon stock. It increased slightly for each scenario over the course of the simulation, with the largest difference again observed in the Natural Evolution scenario. Finally, the dead organic matter (DOM) carbon pool decreased from the beginning to the end of the simulation for all scenarios. The difference was more prominent in high-harvesting scenarios (−14 tC ha−1 from 2020 to 2110 for Increased harvest + increased partial cut + increased planting) than for the Natural Evolution scenario (−2 tC ha−1 from 2020 to 2110).
3.3 Ecosystem carbon fluxes
In terms of annual CO2e fluxes in the forest ecosystems (Figure 5A), all scenarios followed a somewhat similar trend. In the first decade, the carbon sink increased (i.e., net emissions became more negative). After that, emissions increased until about 2080 and then stabilized, except for the Natural Evolution scenario, for which emissions continued to increase until the end of the simulation (Figure 5A). This result suggests that regardless of the management strategy, the strong carbon sink of the early decades could not be maintained.
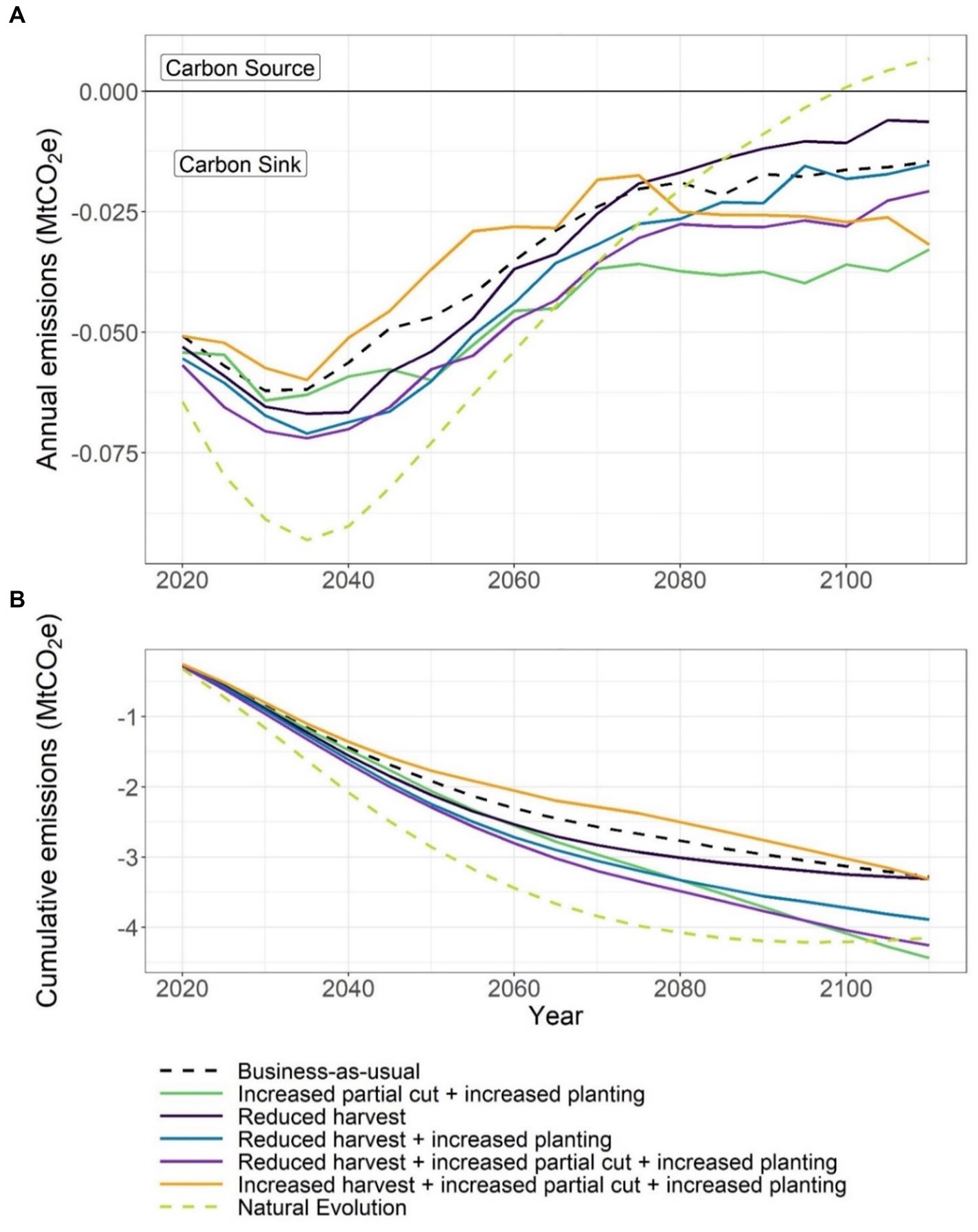
Figure 5. Emissions for the forest ecosystem in megatonnes of CO2e for the seven scenarios over a 95 years horizon. Negative values represent reductions of atmospheric CO2e. (A) Annual emissions presented as an annual average per 5 years period; (B) cumulative emissions.
All scenarios except Natural Evolution were carbon sinks over the 95 years simulation period. Indeed, the Natural Evolution scenario (total conservation strategy) was a larger carbon sink than all harvesting scenarios for the first 80 years; however, in the long term, this scenario generated more emissions than all others and became a carbon source around 2100.
The Business-as-usual scenario resulted in more emissions than most scenarios in the short to medium terms (20 to 50 years), with only the Increased harvest + increased partial cut + increased planting scenario having higher annual emissions. However, the intensive management strategy of the latter scenario maintained high productivity and, thus, long-term sequestration, which appeared to strengthen the sink toward the end of the simulation. Its annual emissions were lower than those of the Bau scenario from around 2080 onward. Conversely, the Reduced harvest scenario had lower emissions than the Bau scenario until 2075 due to a lower harvested area and, thus, lower post-harvest emissions. Still, this strategy resulted in higher emissions by the end of the simulation.
The Increased partial cut + increased planting scenario had similar emissions to the Bau scenario at the beginning but quickly generated a much larger sink. In fact, this scenario (a combination of a relatively high harvest level and increased use of partial cut) generated the largest sink at the end of the simulation among all the scenarios modeled. The role of partial cut was also noticed in the Reduced harvest + increased partial cut + increased planting scenario, which performed better than Reduced harvest + increased planting for most of the simulation (on average −3,903 tCO2e year−1) with the same harvesting and planting levels. Both scenarios performed better than the Bau scenario, especially in the short and medium terms. For a similar harvest level, an increase in planting level (Reduced harvest + increased planting vs Reduced harvest) generated a larger sink (+6,084 tCO2e year−1) because artificially regenerated stands were simulated as having higher productivity than naturally regenerated stands.
In terms of cumulative emissions, i.e., the addition of annual emissions (Figure 5B), the Bau scenario presented the smallest cumulative sink in the forest ecosystem at year 95 (cumulative value of −3.28 MtCO2e). The Natural Evolution scenario (cumulative value of −4.15 MtCO2e) was the largest cumulative sink (−0.87 MtCO2e less than Bau) for most of the simulation due to the higher sequestration early in the simulation. However, the Increased partial cut + increased planting scenario (cumulative value of −4.44 MtCO2e) was the largest cumulative sink at year 95, exceeding the Bau scenario by −1.16 MtCO2e; this scenario had high emissions in the beginning but was the best scenario in terms of annual emissions from 2060. The Increased harvest + increased partial cut + increased planting (cumulative value of −3.31 MtCO2e) had higher emissions than the Bau scenario for most of the simulation. Still, the large sink in the last years resulted in slightly lower cumulative emissions at year 95. The Reduced harvest scenario (cumulative of −3.31 MtCO2e) had the same cumulative emissions as the Increased harvest + increased partial cut + increased planting scenario. However, in this case, emissions were lower than the Bau scenario for most of the simulation; the low sequestration in the last years reduced the gap at year 95. A decrease in harvested timber (Reduced harvest + increased partial cut + increased planting), combined with an increase in partial cut and plantation, generated a large sink in the forest ecosystem (cumulative value of −4.26 MtCO2e). Increased use of partial cut (Reduced harvest + increased partial cut + increased planting vs. Reduced harvest + increased planting) reduced cumulative emissions by −0.37 MtCO2e; for their part, higher planting levels (Reduced harvest + increased planting vs. Reduced harvest) reduced cumulative emissions by −0.58 MtCO2e.
3.4 Harvested wood product carbon fluxes
Emissions from HWP included two main components: emissions associated with extraction, transportation and manufacturing (Figure 6A) and emissions from product decay over their service life and in landfills (Figure 6B). No emissions were reported for the Natural Evolution scenario since no harvesting was done. For other scenarios, HWP emissions were proportional to the amount of wood harvested and, therefore, relatively constant throughout the simulation since harvested timber was kept stable over the years. HWP emissions were mainly due to product decay and pulp and paper manufacturing. Overall, emissions from decay, pulp and paper manufacturing, sawnwood and panel manufacturing, extraction and transportation accounted for 88.5, 8.1, 1.2, 1.1, and 1.0% of all emissions, respectively (average for the six scenarios in Figure 6).
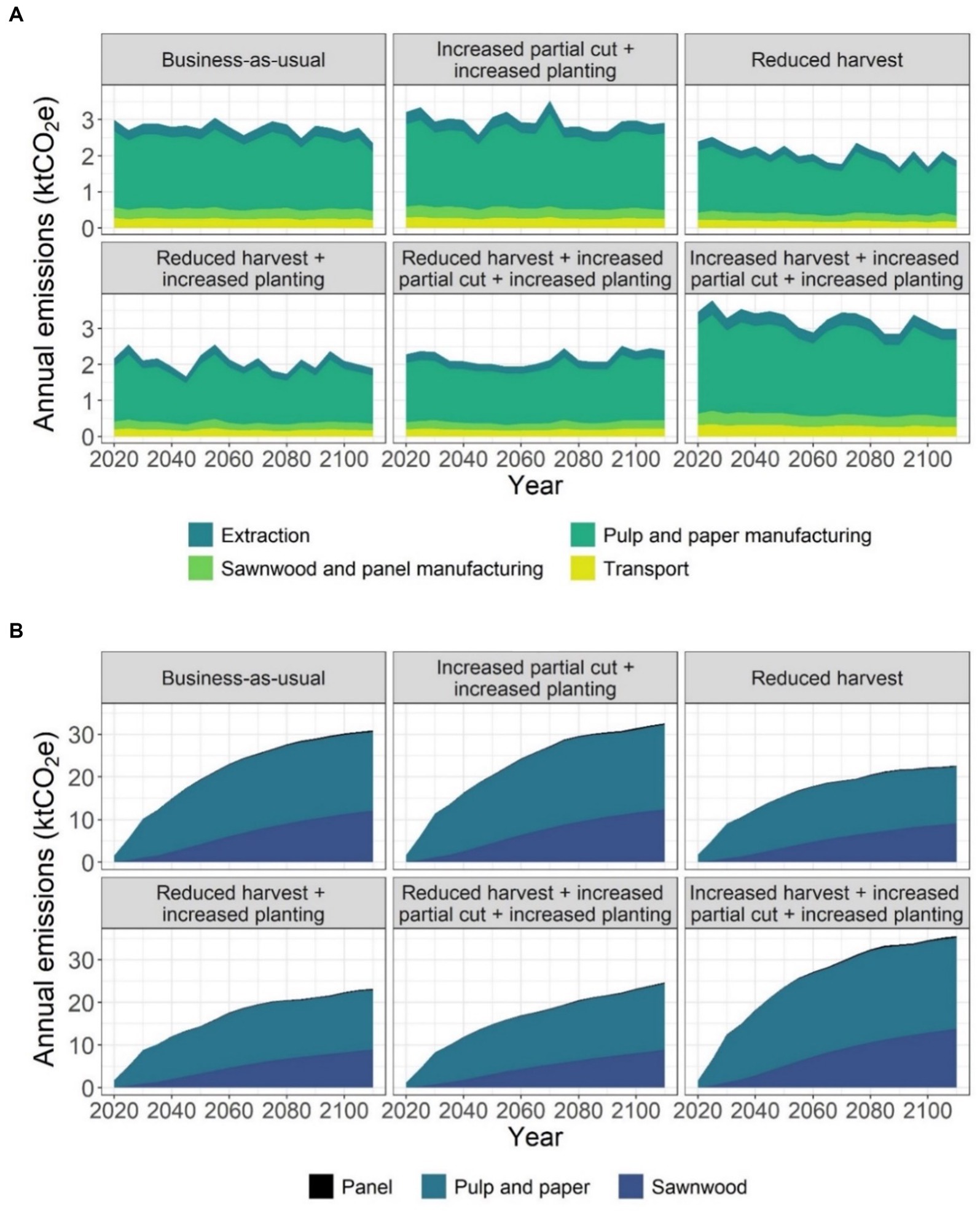
Figure 6. Emissions from harvested wood products in kilotonnes of CO2e for the six harvesting scenarios over a 95 years horizon. Emissions are presented as an annual average per 5 years period. (A) Emissions from extraction, transportation from the forest to the processing site, pulp and paper manufacturing, and sawnwood and panel manufacturing; (B) emissions from product decay during their service life and in landfills.
In terms of cumulative emissions (Figure 7), the Increased harvest + increased partial cut + increased planting scenario had the highest emissions (cumulative value of 2.71 MtCO2e at the end of the horizon), 0.40 MtCO2e more than the Bau scenario (cumulative value of 2.31 MtCO2e). The Increased partial cut + increased planting scenario (cumulative value of 2.45 MtCO2e) produced more emissions than the Bau scenario for a similar volume of harvested timber because it generated more (short-lived) pulp and paper products. A reduction in harvested timber significantly reduced total HWP emissions, with all three Reduced harvest scenarios resulting in lower emissions than the other three scenarios.
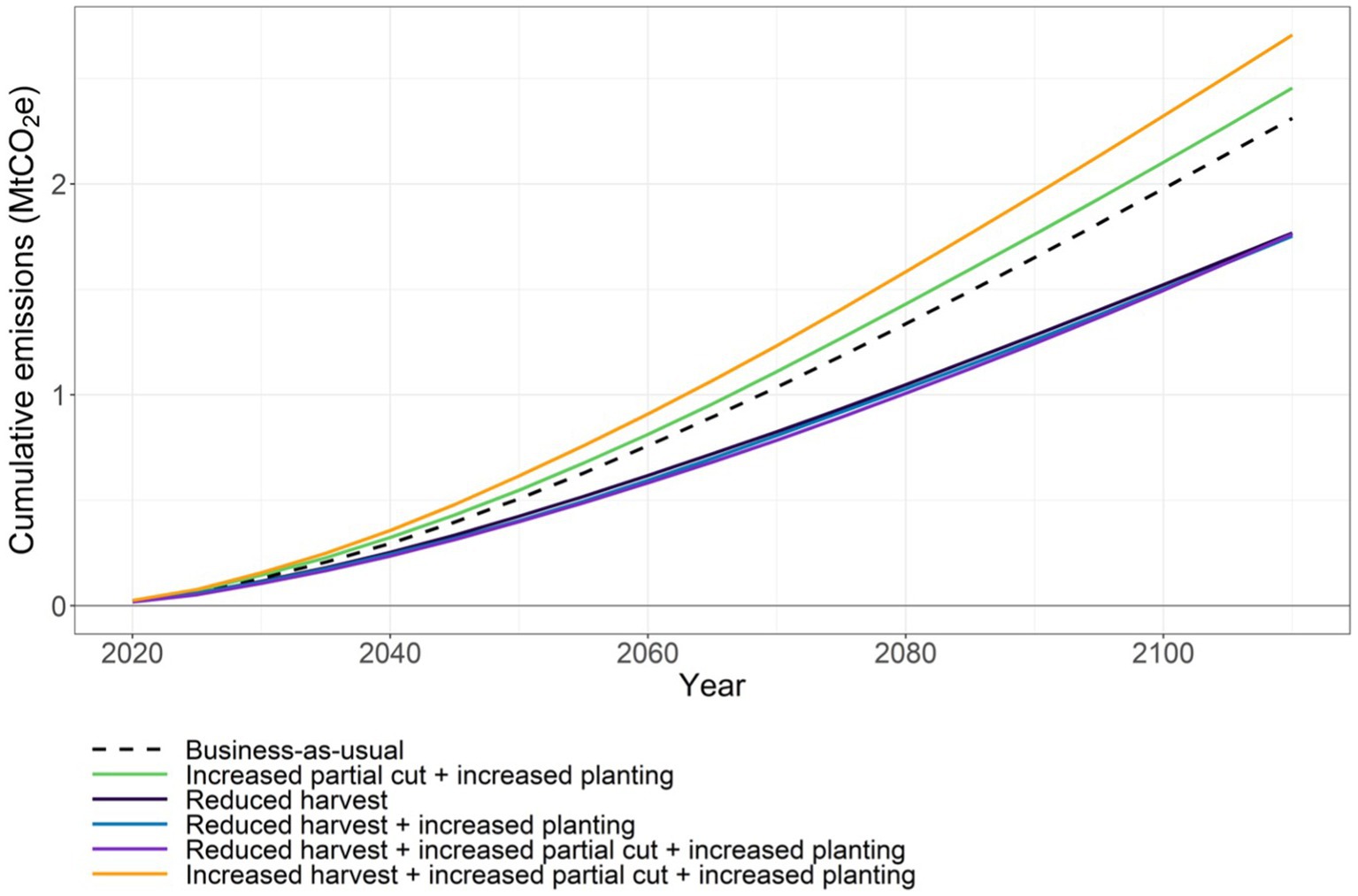
Figure 7. Cumulative emissions from harvested wood products in megatonnes of CO2e for the six harvesting scenarios over a 95 years horizon. Emissions include extraction, transportation, manufacturing and product decay during their service life and in landfills.
3.5 Total carbon fluxes
Carbon sequestration in the forest ecosystems was large enough to offset the source of emissions from HWP, so each scenario was a net sink over the 95 years simulation when considering the total system (Figure 8). The Natural Evolution scenario had the strongest sink over the entire simulation (cumulative value of −4.15 MtCO2e) due to the strong sequestration in the forest ecosystem in the first half of the simulation (Figure 5A) and the absence of HWP emissions. The emissions of this scenario were 3.18 MtCO2e lower than those of the Bau scenario (cumulative value of −0.97 MtCO2e at the end of the simulation). In fact, only the Increased harvest + increased partial cut + increased planting (cumulative value of −0.61 MtCO2e) had higher emissions compared to the Bau scenario with a difference of 0.36 MtCO2e; these scenarios had very similar ecosystem emissions, but the increased harvested timber resulted in more HWP emissions.
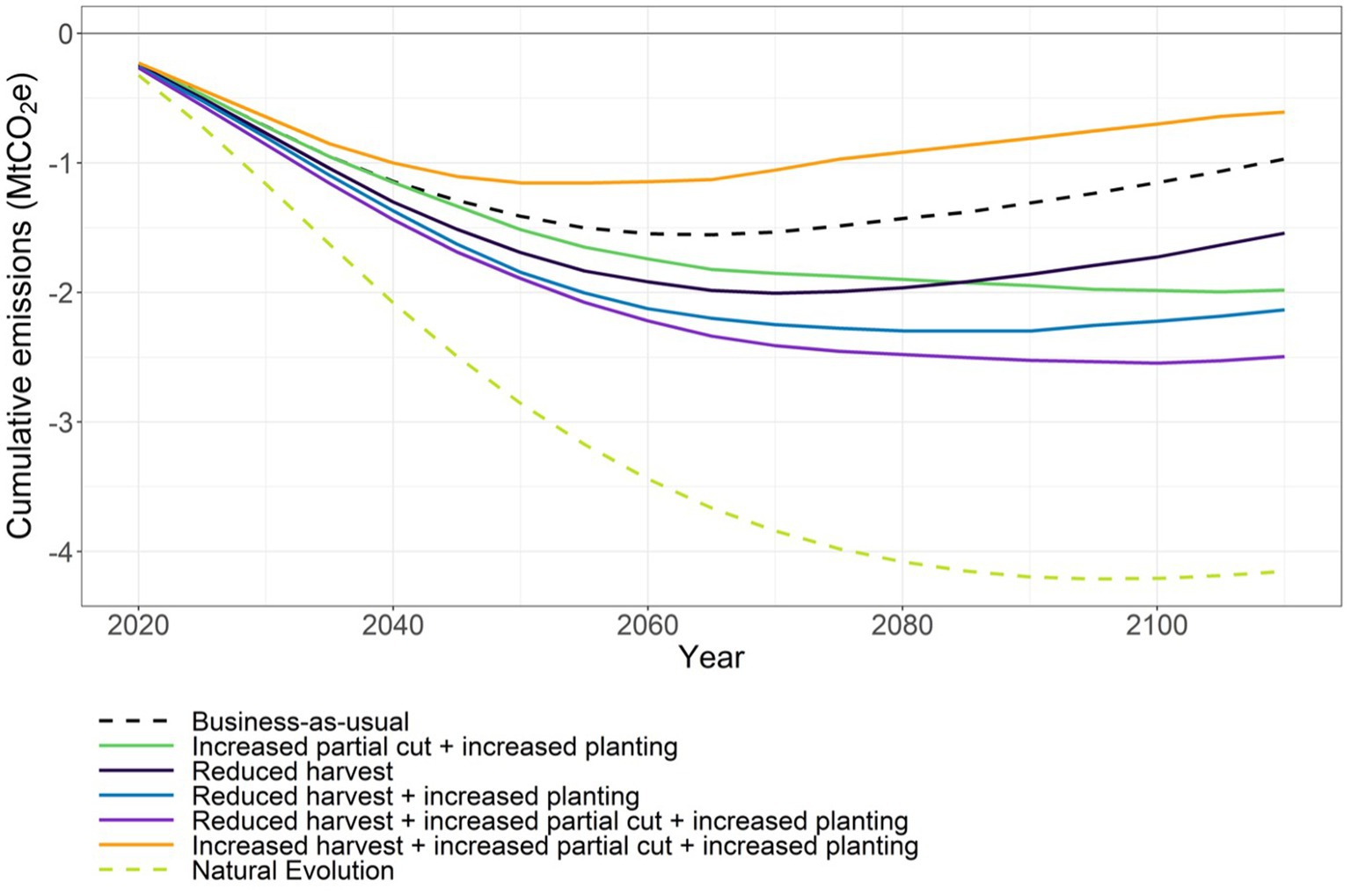
Figure 8. Cumulative emissions for the Forest + Products system in megatonnes of CO2e for the seven scenarios over a 95 years horizon. Negative values represent reductions of atmospheric CO2e.
The Reduced harvest scenario (cumulative value of −1.54 MtCO2e at the end of the simulation) also had similar ecosystem emissions compared to Bau, but the reduced harvested timber reduced HWP emissions for a total difference of −0.57 MtCO2e. At the end of the simulation period, the Reduced harvest + increased planting scenario had a cumulative sink of −2.13 MtCO2e, representing an additional sequestration of −1.16 MtCO2e relative to Bau. The Reduced harvest + increased partial cut + increased planting generated the greatest overall carbon sink (−2.50 MtCO2e), representing an additional sequestration of −1.52 MtCO2e relative to Bau. The increase in planting (Reduced harvest + increased planting vs. Reduced harvest) generated a sink of −0.59 MtCO2e, while an increase in partial cut (Reduced harvest + increased partial cut + increased planting vs. Reduced harvest + increased planting) resulted in an additional sink of −0.36 MtCO2e. The Increased partial cut + increased planting (cumulative value of −1.98 MtCO2e) had the largest forest ecosystem sink at year 95 but was ranked fourth in total emissions due to its high HWP emissions.
3.6 Sensitivity analyses
3.6.1 Sawmilling efficiency
The effect of a higher proportion of sawnwood products generated from wood by sawmills on HWP emissions was tested. Results for scenarios with contrasted harvest levels (Bau, Reduced harvest and Increased harvest + increased partial cut + increased planting) are presented in Table 4. Increasing the sawnwood yield from 42 to 52% reduced the cumulative emissions from HWP by an average of 12% for all scenarios for 2030, 11% for 2050 and 7% for the 2100 horizon.
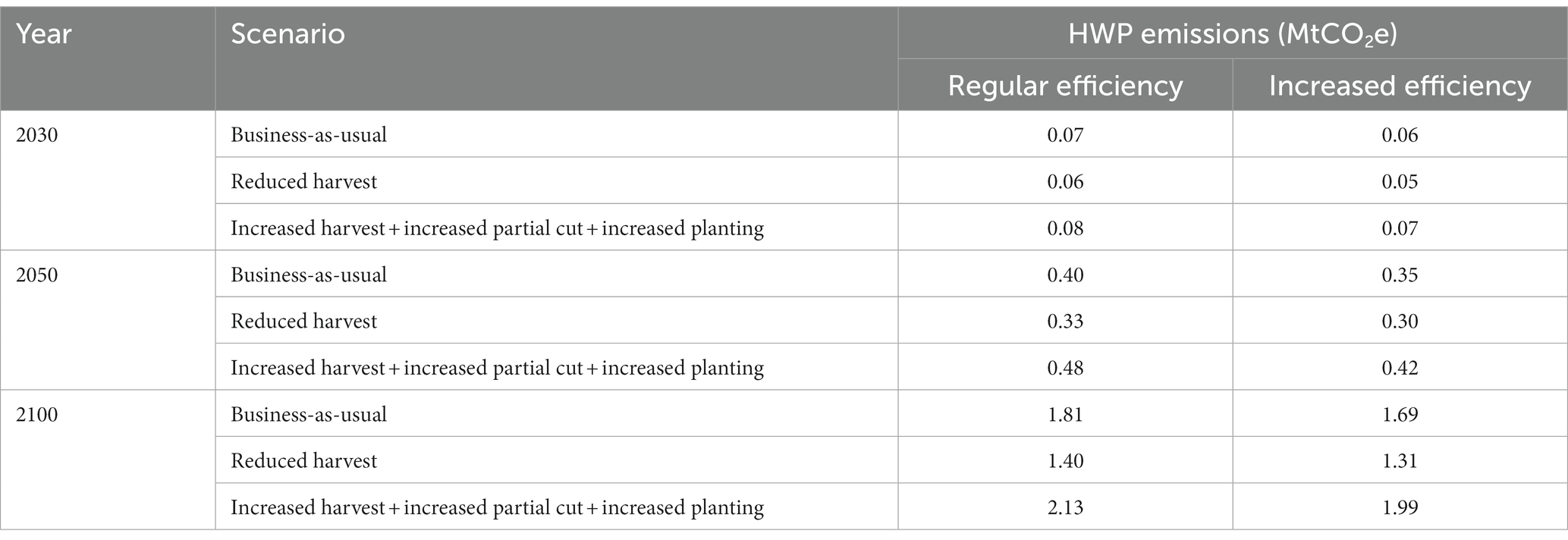
Table 4. Cumulative emissions from harvested wood products for 2030, 2050, and 2100 for the Business-as-usual, Reduced harvest and Increased harvest + increased partial cut + increased planting scenarios with a regular and an increased sawmilling efficiency.
3.6.2 Transport distance from forest to mills
The impact on the carbon balance of increasing transport distances from forest to sawmills was also tested by comparing (i) distinct management strategies and (ii) distinct industrial structures for wood destinations, both of which would influence distances. First, results from two scenarios with similar harvested volume but contrasting levels of partial cut are presented (Bau and Increased partial cut + increased planting) since a partial cut strategy should result in more dispersed harvest operations over the landscape relative to a clearcut strategy. The Increased partial cut + increased planting scenario resulted in slightly higher average transport distances because of the partial cut-based management strategy and thus produced more emissions than the Bau scenario per unit of wood harvested (Table 5). However, this increase caused by the management strategy did not lead to any meaningful difference in total cumulative emissions, as they remained similar between management scenarios.
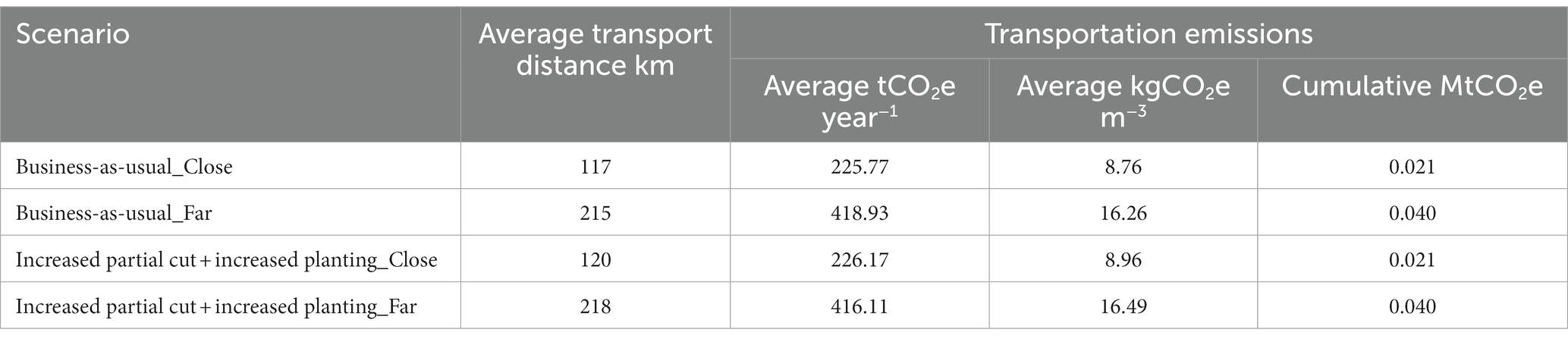
Table 5. Emissions from transportation for the Business-as-usual and the Increased partial cut + increased planting scenarios with two wood destinations.
Second, the average transport distance was almost double for the Far scenario for wood industrial destinations for which wood volumes of different species and qualities were sent to different industrial facilities, relative to the Close scenario for which all harvested wood was sent to the closest mill. The Far scenario nearly doubled emissions related to transportation for both management scenarios (Table 5) and caused an increase in cumulative emissions of 0.018 MtCO2e at the end of the simulation period.
4 Discussion
This study evaluated the carbon balance of forest management and industrial wood processing of scenarios stemming from strategic planning optimization of annual allowable cut (AAC). As such, our forest management scenarios reflected realistic solutions that consider the need to maintain a steady wood supply and level of silvicultural activities over the years. Our simulation results suggest that for the boreal balsam fir-white birch landscapes of Quebec that contain a large share of young stands, a reduction in harvest rates combined with an increase in the use of partial cut (instead of clearcut) and planting (instead of natural regeneration) can significantly reduce GHG emissions to the atmosphere even on the long term. The higher transport distances that result from the more dispersed harvest operations associated with partial cut only had a negligible effect on total emissions.
High harvest rates and intensive management can potentially increase the long-term carbon sink of the forest ecosystem. However, this sink may not be sufficient to offset the increase in decay emissions from a larger pool of harvested wood products if the latter contains an important share of short-lived pulp and paper products. Aiming for a high proportion of long-lived wood products such as sawnwood should be a priority. Moreover, variations in transport distances from forest to processing facilities, while crucial for the profitability of wood production, only have a marginal effect on the overall carbon balance of forest management and wood production, at least for the range of distances that we tested (117 km–218 km). In the context of climate change mitigation, the efficiency of wood processing (sawnwood yield), and not the location of (and transport distances to) mills per se, appears as a greater priority.
4.1 Harvesting and wood production
Scenarios with lower harvest levels, or no harvest at all (Natural Evolution), had the lowest cumulative carbon emissions (including fluxes in both the forest ecosystems and wood products). However, in the longer term, reduced harvest/conservation strategies were not optimal for maintaining a sizeable annual C sink in forest ecosystems, a result similar to the one observed by Dong et al. (2023). The time horizon considered is an important variable affecting the mitigation potential of management strategies, especially when comparing total conservation to intensification scenarios (Pukkala, 2017; Skytt et al., 2021). We could hypothesize that a total conservation strategy would have a declining mitigation potential relative to the Bau scenario if the time horizon were increased to 150 or 200 years, for example. Conservation strategies can lead to lower sequestration due to a lower proportion of young, actively growing stands and the absence of silvicultural practices that increase productivity. In our case, at the beginning of the simulation, most forest stands (45% of the territory under management) were premature (around 30 years old), meaning that they were a significant carbon sink and were at the peak of their carbon sequestration (Senez-Gagnon et al., 2018; Uri et al., 2022). As the simulation progressed, the proportion of stands in this age class decreased in favor of the 50, 70, and 90+ year age classes, reducing forest carbon uptake. Boreal balsam fir stands at the later stages of the succession (70+ years), while containing important carbon stocks, have been shown to be more or less carbon-neutral (i.e., neither a sink nor a source) (Harel et al., 2021). The proportion of stands in the 10 years age class that are a source of emissions in this type of ecosystem (Paradis et al., 2019) remained relatively constant throughout the simulation for harvesting scenarios and thus did not have a substantial impact. In Quebec, the ecosystem-based forest management principles at the heart of the Forest Act include requirements to maintain a maximum proportion of regenerating forest (maximum of 30%) and a minimum proportion of old-growth forest at the landscape scale (corresponding to at least 30% of the historical average, or at least 25.8% of the forest area in this case study), for the purpose of restoring or maintaining biodiversity (Ministère des Forêts de la Faune et des Parcs, 2016); these regulatory constraints were applied in all our scenarios. Based on our results, these requirements likely have an important impact on the carbon balance of forest ecosystems; they constrain the overall harvest levels and influence the proportions of stands that are net carbon sources vs. carbon sinks vs. carbon-neutral at any given time.
Studies in North America (Chen et al., 2018; Smyth et al., 2018) and Europe (Lundmark et al., 2014; Gustavsson et al., 2017) suggest that intensification strategies are superior to conservation strategies to mitigate climate change. For example, a study in Ontario, Canada (Chen et al., 2018) found that increased harvesting could have a positive long-term mitigation potential if the harvested wood is used for long-lived products in the construction sector. While this effect is partially due to the longer carbon storage in those products, it is also a result of the assumed high substitution effects of such products on markets, i.e., the displacement of GHG-intensive materials by wood. In many instances, increased harvesting levels can only provide a mitigation potential if combined with high substitution benefits. Indeed, in most studies where an increase in harvest rates or management intensification led to an increased mitigation potential, the estimated substitution effect (treated as a carbon sequestration) was considerable. Meta-analyses by Leskinen et al. (2018) and Sathre and O’Connor (2010) calculated average displacement factors (DF) of 1.2 and 2.1 tonnes of C avoided per tonne of harvested C transferred to wood products. In Canada, the average DF are much lower: Smyth et al. (2017) calculated a DF of 0.54 tonne of C avoided per tonne of C in wood products for sawnwood, which is therefore estimated to have a much more limited positive impact on the mitigation potential of intensive management strategies.
Yet, uncertainties reside in the potential climate benefits provided by substitution. First, calculations of the substitution effect rely on displacement factors that are likely to change over time as technological advances and emission reductions are made not only in the forestry sector but also in other sectors such as steel and concrete. This may lead to an overestimation of the expected benefits of substitution (Harmon, 2019). There is also the underlying assumption that a change in harvest rates will affect the consumption of wood and its substitutable materials (Howard et al., 2021). The Montmorency Forest is only a minor contributor of wood products relative to the rest of the province (20,000–30,000 harvested m3 per year vs. 29 millions m3 for the province). While it would be different if simulations were made at the scale of a province or a country, as was done for, e.g., Quebec (Moreau et al., 2023), Canada (Smyth et al., 2014) or Mexico (Olguin et al., 2018), an increase in harvest rates in Montmorency Forest would only marginally impact markets and emissions from other sectors such as energy and materials (Leturcq, 2020). The simple decay approach to carbon accounting used in our study reports the removals/emissions from forest ecosystem processes and the emissions from the decay of wood products sourced from the producing area (Montmorency Forest) (Hashimoto, 2008; Brunet-Navarro et al., 2016). Detailed material flow projections at the regional or national level would be needed to quantify the larger GHG impacts (both in the forestry and other sectors) of an increase/decrease in wood supply from Montmorency Forest.
4.2 Silvicultural strategies
Results of our study suggest that for a given harvested volume, it is possible to reduce carbon emissions with increased use of partial cut and/or planting. Indeed, both planting and partial cut contributed strongly to the forest C sink and were more efficient in the long term than simply reducing overall harvest levels.
In Montmorency Forest, it has been estimated that it takes 26 years following a clearcut for the stand to become a net carbon sink (Paradis et al., 2019). It is therefore essential to develop strategies that reduce the period during which harvested areas are carbon sources. Strukelj et al. (2015) reported that the growth and carbon sequestration of residual stems after a partial cut can quickly allow the stand to become a net carbon sink. Also, partial cut in the form of continuous-cover management or high thinnings (i.e., half of the removed basal area from the largest diameter classes and the other half from all diameter classes) can produce larger logs and therefore a larger share of long-lived products that reduce emissions from decay and manufacturing (Pukkala, 2014). In contrast, in Norway, Nilsen and Strand (2013) found that over a period of 5 to 8 decades, even-aged management is superior to uneven-aged management for carbon sequestration in the ecosystem at the stand scale (i.e., without considering carbon storage in wood products), due to overall higher wood production in even-aged stands. Nevertheless, our study suggests that it is possible to attain similar overall harvest levels at the landscape scale with a management strategy that includes a larger share of partial cuts.
Compared with plantation, natural regeneration of harvested areas is cheaper (Greene et al., 2002) and contributes to creating stands with composition, structure, genetic pool, and dead wood stocks closer to natural unmanaged forests (Lieffers et al., 2003). However, plantation and related practices such as brushing and commercial thinning, as used in our simulations, can generate much higher yields than naturally regenerated stands (Paquette and Messier, 2010) and, therefore, sequester more carbon. Carefully established and managed plantations could also increase the proportion of long-lived products that would be manufactured from the harvested wood [e.g., due to stems with larger diameters (Morris et al., 2014)], therefore contributing to reduced carbon emissions from forest management and wood production.
4.3 Wood industrial network
Our results indicated that the transportation distances did not account for a large portion of total emissions. For instance, the cumulative emissions at the end of the simulation for the Increased partial cut + increased planting scenario with wood of different species and qualities sent to different wood destinations (Far scenario) were increased by 1.78% (0.019 MtCO2e) with a near doubling of average transportation distances in comparison to the Bau scenario for which all harvested wood was sent to the closest mill (Close scenario) (218 vs. 117 km). In contrast, when we assumed an improved sawmilling efficiency yielding a more important share of long-lived products (a proportion of 53% of sawnwood from softwood logs instead of 42%) (but no change in transportation distance), this caused a reduction in cumulative emissions of 13.75% (or −0.139 MtCO2e).
Therefore, in our study, the sawmilling efficiency appears to be a more important factor in the carbon balance than the distance to processing facilities over the range of values we tested here. A 1% increase in sawmilling efficiency yielded a cumulative reduction of 0.0126 MtCO2e over the simulation period; the same emission reduction would necessitate a decrease in average transportation distances of about 67 km. This suggests that, from a carbon balance standpoint, it is advantageous to seek wood processing facilities that can guarantee a higher material yield, even if they are located further (notwithstanding effects on costs). Such higher material yields could be obtained by better planning of wood-cutting patterns (Dumetz et al., 2021) and better tracing and sorting of logs (Björk et al., 2011). However, advances in reducing fossil fuel emissions in wood supply chains can also contribute to an overall reduction in emissions; efforts in this matter should not be overlooked (Yan et al., 2021). Based on our results, emissions from transportation would need to be reduced by an average of 0.03 to 0.05 kgCO2e m−3 km−1 to cause the equivalent carbon effect of a 1% increase in sawmilling efficiency. Nevertheless, for a given forest management system, the strategic, technical or financial feasibility of modifying transport distances vs. transport fuel efficiency vs. sawmilling efficiency might differ.
The importance of increasing the share of long-lived products by improvements in manufacturing processes and/or management practices that increase the size and/or fibre quality of trees has often been mentioned in the literature (Pukkala, 2014; Gustavsson et al., 2017; Chen et al., 2018). In our study, we assumed no change in wood processing and manufacturing practices. However, technological advancements in these areas will likely lead over time to the production of new wood products, products with extended service life and/or increased recycling rates, thereby increasing carbon storage and reducing emissions (Li et al., 2022). Changes toward an increased share of long-lived products can also be driven by increased market demand for wood for home applications and constructions (Zhao et al., 2022). Likewise, improvements in landfill management (such as an increased recovery and use of CH4) would also reduce expected emissions from the end-of-life of wood products (Moreau et al., 2023). Our basket of products also did not vary according to forest management scenarios. It would, however, be possible to modulate the basket to reflect a greater proportion of larger and/or higher quality stems that might emerge from silvicultural practices, which would influence emissions from wood products. Our analysis of the impact of transport distances could also be refined in future work, as we did not disaggregate Woodstock solutions into diametrical classes or log quality and did not include financial aspects. Data on costs and log size and quality could (i) give an indication of the difference caused by the management strategy on the proportion of long-lived products in wood supply and (ii) assess the profitability and mitigation costs of alternative strategies.
4.4 Natural disturbances and climate change
Natural disturbances were not included in our simulations. Their impact would most certainly affect the results as the Montmorency Forest is subject to frequent (30–40 years) spruce budworm epidemics and windthrows. In eastern Canada, simulations by Dymond et al. (2010) and Liu et al. (2019) suggest that large forest areas that are currently carbon sinks could become carbon sources during the current outbreak because of reduced growth of trees suffering from defoliation and mortality. Our results showed that conservation strategies lead to a higher proportion of mature and old forests, which are more vulnerable to spruce budworm outbreaks (MacLean, 1980); these strategies could, therefore, be more susceptible to carbon loss following an outbreak. Windthrow can also reduce the effectiveness of partial cut to mitigate CO2 emissions if residual stems fall soon after harvest (Moreau et al., 2023). Since the mitigation potential of partial cut is strongly dependent on the regeneration success and the capacity to increase the growth of residual trees (Kellomäki et al., 2019), windthrow could counteract these benefits. Moreover, in Russia, Knohl et al. (2002) found that windthrow had a large impact on carbon ecosystem exchanges for an extended period as deadwood decomposition is slow.
Finally, we did not account for the effect of climate change on forest landscapes. As it is unlikely that the climate conditions will be identical for the 95 years simulation, there are larger uncertainties for the long-term results of this study. Boreal forests of eastern Canada are particularly vulnerable to anthropogenic climate forcing and will likely undergo important changes in composition and a decrease in productivity (Boulanger and Pascual Puigdevall, 2021). It remains to be seen if specific management practices (including conservation) can either reduce or amplify these impacts. For example, Landry et al. (2021) found that harvesting increased the overall ecosystem C sink of forests at the boreal-temperate ecotone of Quebec, but rendered the landscapes more vulnerable to climate change effects than conservation.
5 Conclusion
This study evaluated the carbon balance of forest management strategies as defined by the determination of the annual allowable cut (AAC) calculation process, using a boreal forest of Quebec in eastern Canada as a case study. All management scenarios provided a carbon sink over the whole period analysed in this study (i.e., 95 years). However, a reduction in harvested timber, an increase in partial cuts, and an increase in plantations all contributed to an increase in the forest ecosystem sink compared with the business-as-usual (Bau) scenario. Higher levels of harvesting (such as the Increased harvest + increased partial cut + increased planting) compared to the business-as-usual could not be justified per se because the long-term (i.e., >50 years) increase in the forest ecosystem carbon sink could not offset the growth in emissions from wood products, mainly from short-lived products such as paper.
Some trade-offs need to be made between optimization of the carbon balance and ecosystem-based management objectives to promote biodiversity, such as increasing the proportion of old-growth forests (low carbon uptake) and relying on natural regeneration (lower carbon uptake than plantations). Partial cut appears to be a solution that allows synergies between ecosystem-based management and short (<20 years) to long-term emission (>50 years) reduction objectives. The next step should be the inclusion of natural disturbances in the simulations, as they play an important role in Québec’s boreal forests and would certainly influence the results; analysis of the interaction with climate change projections would also be needed.
Moreover, our analysis of the transport distances on the carbon balance suggests that these emissions are marginal when compared to the total fluxes of the Forest-Products system. Aiming for a higher yield of long-lived wood products such as sawnwood should be an overarching goal through an improvement of sawmilling processes and/or silvicultural practices that increase the quality of wood supply. Nevertheless, optimizing transportation routes from forest to mills, and improving fuel/energy efficiency of trucks and other machinery, can also contribute to improving the carbon balance of forest management and wood production. The costs of various potential actions must be assessed to identify the most cost-effective portfolio of climate change mitigation measures.
Data availability statement
The datasets presented in this study can be found in online repositories. The names of the repository/repositories and accession number(s) can be found at: https://figshare.com/articles/dataset/Giasson_et_al_Carbon_balance_of_forest_management/21197215.
Author contributions
L-AG and ET: conceptualization. L-AG: formal analysis and writing (original draft). ET: funding acquisition and project administration. L-AG, ET, LL, and J-FC: methodology and writing (review and editing).
Funding
The author(s) declare financial support was received for the research, authorship, and/or publication of this article. This work was supported by a research contract from the Quebec Ministry of Forests, Wildlife and Parks (PI: ET; research project 142332174-E).
Acknowledgments
This article is adapted from the MSc thesis of L-AG at Université Laval. The authors wish to thank the team from the Quebec Office of the Chief Forester for their support in providing a Woodstock model and parameterizing and troubleshooting simulations in GCBM, as well as Alexandre Morneau and Edith Brotherton of the FORAC Consortium for their help with Logilab. Warm thanks to Jean-François Lamarre, forest manager of Montmorency Forest, for his help with management scenarios.
Conflict of interest
The authors declare that the research was conducted in the absence of any commercial or financial relationships that could be construed as a potential conflict of interest.
Publisher’s note
All claims expressed in this article are solely those of the authors and do not necessarily represent those of their affiliated organizations, or those of the publisher, the editors and the reviewers. Any product that may be evaluated in this article, or claim that may be made by its manufacturer, is not guaranteed or endorsed by the publisher.
References
Ameray, A., Bergeron, Y., Valeria, O., Montoro Girona, M., and Cavard, X. (2021). Forest carbon management: a review of Silvicultural practices and management strategies across boreal, temperate and tropical forests. Curr. Forestr. Rep. 7, 245–266. doi: 10.1007/s40725-021-00151-w
Andersen, E.D., and Andersen, K.D., (2000) The Mosek interior point optimizer for linear programming: an implementation of the homogeneous algorithm. High performance optimization. H. Frenk, K. Roos, T. Terlaky, and S. Zhang (Eds.), Springer US, Boston, MA, 197–232
Andrieux, B., Beguin, J., Bergeron, Y., Grondin, P., and Paré, D. (2018). Drivers of postfire soil organic carbon accumulation in the boreal forest. Glob. Chang. Biol. 24, 4797–4815. doi: 10.1111/gcb.14365
Athena Sustainable Materials Institute (2018). A cradle-to-gate life cycle assessment of Canadian surfaced dry softwood lumber, 43.
Athena Sustainable Materials Institute (2018). A cradle-to-gate life cycle assessment of Canadian plywood, 33.
Athena Sustainable Materials Institute (2018). A cradle-to-gate life cycle assessment of Canadian oriented Strand board – OSB, 33.
Auger, I. (2017), Natura-2014: Mise à jour et évaluation du modèle de croissance forestière à l'échelle du peuplement. Note de recherche forestière No 147. Direction de la recherche forestière, Ministère des Forêts, de la Faune et des Parcs: Sainte-Foy, Qc, 32
Beauregard, R., Lavoie, P., Thiffault, E., Ménard, I., Moreau, L., Boucher, J.-F., et al. (2019). Report of the working group on forests and climate change (in French: Rapport du groupe de travail Sur la forêt et les changements climatiques) Ministère des Forêts, de la Faune et des Parcs du Québec, Ministère de l’Environnement et de la Lutte contre les changements climatiques et Conseil de l’industrie forestière du Québec, Québec, 53.
Björk, A., Erlandsson, M., Häkli, J., Jaakkola, K., Nilsson, Å., Nummila, K., et al. (2011). Monitoring environmental performance of the forestry supply chain using RFID. Comput. Ind. 62, 830–841. doi: 10.1016/j.compind.2011.08.001
Boukherroub, T., LeBel, L., and Lemieux, S. (2017). An integrated wood pellet supply chain development: selecting among feedstock sources and a range of operating scales. Appl. Energy 198, 385–400. doi: 10.1016/j.apenergy.2016.12.013
Boulanger, Y., and Pascual Puigdevall, J. (2021). Boreal forests will be more severely affected by projected anthropogenic climate forcing than mixedwood and northern hardwood forests in eastern Canada. Landsc. Ecol. 36, 1725–1740. doi: 10.1007/s10980-021-01241-7
Brunet-Navarro, P., Jochheim, H., and Muys, B. (2016). Modelling carbon stocks and fluxes in the wood product sector: a comparative review. Glob. Chang. Biol. 22, 2555–2569. doi: 10.1111/gcb.13235
Bureau du forestier en chef . (2018), Évolution des strates Fascicule 2.4. Manuel de détermination des possibilités forestières 2018–2023, Bureau du forestier en chef, Gouvernement du Québec, Roberval, Qc, 7
Bureau du forestier en chef . (2018), Calcul et détermination des possibilités forestières. Fascicule 1.2. Manuel de détermination des possibilités forestières, Bureau du forestier en chef, Gouvernement du Québec, Roberval, Qc, 5
Cantegril, P., Paradis, G., LeBel, L., and Raulier, F. (2019). Bioenergy production to improve value-creation potential of strategic forest management plans in mixed-wood forests of eastern Canada. Appl. Energy 247, 171–181. doi: 10.1016/j.apenergy.2019.04.022
Chen, J., Ter-Mikaelian, M. T., Ng, P. Q., and Colombo, S. J. (2018). Ontario’s managed forests and harvested wood products contribute to greenhouse gas mitigation from 2020 to 2100. For. Chron. 94, 269–282. doi: 10.5558/tfc2018-040
Chen, J., Ter-Mikaelian, M. T., Yang, H., and Colombo, S. J. (2018). Assessing the greenhouse gas effects of harvested wood products manufactured from managed forests in Canada. Forestry Int. J. Forest Res. 91, 193–205. doi: 10.1093/forestry/cpx056
Colombo, S.J., Parker, W., Luckai, N., Dang, Q., and Cai, T., (2005), The effects of forest management on carbon storage in Ontario’s forests. Climate Change Research Report-Ontario Forest Research Institute. Ontario Ministry of Natural Resources, Peterborough, Ont.. 126
Couillard, P.-L., Payette, S., and Grondin, P. (2013). Long-term impact of fire on high-altitude balsam fir (Abies balsamea) forests in south-Central Quebec deduced from soil charcoal. Can. J. For. Res. 43, 188–199. doi: 10.1139/cjfr-2012-0414
Dong, L., Bettinger, P., and Liu, Z. (2023). Periodic harvests, rather than passive conservation, increase the carbon balance of boreal forests much more in Northeast China. For. Ecol. Manag. 530:120777. doi: 10.1016/j.foreco.2023.120777
Dumetz, L., Gaudreault, J., Bril El-Haouzi, H., Thomas, A., Lehoux, N., and Marier, P. (2021). Tactical-operational coordination of a divergent production system with coproduction: the sawmilling challenge. INFOR 59, 377–399. doi: 10.1080/03155986.2021.1906057
Dymond, C. C., Neilson, E. T., Stinson, G., Porter, K., Mac Lean, D. A., Gray, D. R., et al. (2010). Future spruce budworm outbreak may create a carbon source in eastern Canadian forests. Ecosystems 13, 917–931. doi: 10.1007/s10021-010-9364-z
Environment Canada . (2021), Canadian climate normals 1981–2010. Available at: https://climate.weather.gc.ca/climate_normals/index_e.html (Accessed on October 2021).
Environment and Climate Change Canada . (2023). National inventory report: greenhouse gas sources and sinks in Canada, part 2. (Ottawa, Ont: Government of Canada). 324.
Forster, P., Ramaswamy, V., Artaxo, P., Berntsen, T., Betts, R., Fahey, D.W., et al., (2007), Changes in atmospheric constituents and in radiative forcing. Climate change 2007: The physical science basis. Contribution of working group I to the 4th assessment report of the intergovernmental panel on climate change. S. Solomon, D. Qin, M. Manning, Z. Chen, M. Marquis, and K.B. Averyt, et al. (Eds.), Cambridge University Press, Cambridge, United Kingdom and New York, USA, 129–264
Greene, D. F., Kneeshaw, D. D., Messier, C., Lieffers, V., Cormier, D., Doucet, R., et al. (2002). Modelling silvicultural alternatives for conifer regeneration in boreal mixedwood stands (aspen/white spruce/balsam fir). For. Chron. 78, 281–295. doi: 10.5558/tfc78281-2
Gustavsson, L., Haus, S., Lundblad, M., Lundström, A., Ortiz, C. A., Sathre, R., et al. (2017). Climate change effects of forestry and substitution of carbon-intensive materials and fossil fuels. Renew. Sust. Energ. Rev. 67, 612–624. doi: 10.1016/j.rser.2016.09.056
Harel, A., Thiffault, E., and Paré, D. (2021). Ageing forests and carbon storage: a case study in boreal balsam fir stands. Forestry Int. J. Forest Res. 94, 651–663. doi: 10.1093/forestry/cpab021
Harmon, M. E. (2019). Have product substitution carbon benefits been overestimated? A sensitivity analysis of key assumptions. Environ. Res. Lett. 14:065008. doi: 10.1088/1748-9326/ab1e95
Hashimoto, S. (2008). Different accounting approaches to harvested wood products in national greenhouse gas inventories: their incentives to achievement of major policy goals. Environ. Sci. Pol. 11, 756–771. doi: 10.1016/j.envsci.2008.08.002
Head, M., Magnan, M., Kurz, W. A., Levasseur, A., Beauregard, R., and Margni, M. (2021). Temporally-differentiated biogenic carbon accounting of wood building product life cycles. Appl. Sci. 3:62. doi: 10.1007/s42452-020-03979-2
Hennigar, C. R., Mac Lean, D. A., and Amos-Binks, L. J. (2008). A novel approach to optimize management strategies for carbon stored in both forests and wood products. For. Ecol. Manag. 256, 786–797. doi: 10.1016/j.foreco.2008.05.037
Howard, C., Dymond, C. C., Griess, V. C., Tolkien-Spurr, D., and van Kooten, G. C. (2021). Wood product carbon substitution benefits: a critical review of assumptions. Carbon Balance Manag. 16:9. doi: 10.1186/s13021-021-00171-w
Hudiburg, T. W., Law, B. E., Moomaw, W. R., Harmon, M. E., and Stenzel, J. E. (2019). Meeting GHG reduction targets requires accounting for all forest sector emissions. Environ. Res. Lett. 14:095005. doi: 10.1088/1748-9326/ab28bb
IPCC . (2006) 2006 IPCC guidelines for National Greenhouse gas Inventories, prepared by the National Greenhouse gas Inventories Programme. Institute for Global Environmental Strategies: Kanagawa, Japan
Kellomäki, S., Strandman, H., and Peltola, H. (2019). Effects of even-aged and uneven-aged management on carbon dynamics and timber yield in boreal Norway spruce stands: a forest ecosystem model approach. Forestry Int. J. Forest Res. 92, 635–647. doi: 10.1093/forestry/cpz040
Knohl, A., Kolle, O., Minayeva, T. Y., Milyukova, I. M., Vygodskaya, N. N., Foken, T., et al. (2002). Carbon dioxide exchange of a Russian boreal forest after disturbance by wind throw. Glob. Chang. Biol. 8, 231–246. doi: 10.1046/j.1365-2486.2002.00475.x
Kurz, W. A., Dymond, C. C., White, T. M., Stinson, G., Shaw, C. H., Rampley, G. J., et al. (2009). CBM-CFS3: a model of carbon-dynamics in forestry and land-use change implementing IPCC standards. Ecol. Model. 220, 480–504. doi: 10.1016/j.ecolmodel.2008.10.018
Landry, G., Thiffault, E., Cyr, D., Moreau, L., Boulanger, Y., and Dymond, C. (2021). Mitigation potential of ecosystem-based forest management under climate change: a case study in the boreal-temperate forest ecotone. Forests 12:667. doi: 10.3390/f12121667
Leblanc, M., and Bélanger, L. (2000) La sapinière vierge de la forêt boréale et de sa région, une forêt boréale distincte. Mémoire de recherche forestière no 136. Gouvernement du Québec, Ministère des Ressources naturelles, Forêt Québec, Direction de la recherche forestière: Québec, QC, 91
Lemprière, T. C., Kurz, W. A., Hogg, E. H., Schmoll, C., Rampley, G. J., Yemshanov, D., et al. (2013). Canadian boreal forests and climate change mitigation. Environ. Rev. 21, 293–321. doi: 10.1139/er-2013-0039
Leskinen, L., Cardellini, G., González-García, S., Hurmekoski, E., Sathre, R., Seppälä, J., et al. (2018). Substitution effects of wood-based products in climate change mitigation. From science to policy 7 European Forest Institute, 27.
Leturcq, P. (2020). GHG displacement factors of harvested wood products: the myth of substitution. Sci. Rep. 10:e8. doi: 10.1038/s41598-020-77527-8
Li, L., Wei, X., Zhao, J., Hayes, D., Daigneault, A., Weiskittel, A., et al. (2022). Technological advancement expands carbon storage in harvested wood products in Maine, USA. Biomass Bioenergy 161:106457. doi: 10.1016/j.biombioe.2022.106457
Lieffers, V.J., Messier, C., Burton, P.J., Ruel, J.-C., and Grover, B.E.. (2003), Chapter 13. Nature-based silviculture for sustaining a variety of boreal forest values. Towards sustainable Management of the Boreal Forest. P.J. Burton, C. Messier, D.W. Smith, and W.L. Adamowicz (Eds.), NRC Research Press, Ottawa, Ontario, Canada, 481–530
Liski, J., Pussinen, A., Pingoud, K., Mäkipää, R., and Karjalainen, T. (2001). Which rotation length is favourable to carbon sequestration? Can. J. For. Res. 31, 2004–2013. doi: 10.1139/x01-140
Liu, Z., Peng, C., De Grandpré, L., Candau, J.-N., Work, T., Huang, C., et al. (2019). Simulation and analysis of the effect of a spruce budworm outbreak on carbon dynamics in boreal forests of Quebec. Ecosystems 22, 1838–1851. doi: 10.1007/s10021-019-00377-7
Lundmark, T., Bergh, J., Hofer, P., Lundström, A., Nordin, A., Poudel, B. C., et al. (2014). Potential roles of Swedish forestry in the context of climate change mitigation. Forests 5, 557–578. doi: 10.3390/f5040557
MacLean, D. A. (1980). Vulnerability of fir-spruce stands during uncontrolled spruce budworm outbreaks: a review and discussion. For. Chron. 56, 213–221. doi: 10.5558/tfc56213-5
Markewitz, D. (2006). Fossil fuel carbon emissions from silviculture: impacts on net carbon sequestration in forests. For. Ecol. Manag. 236, 153–161. doi: 10.1016/j.foreco.2006.08.343
Ministère des Forêts de la Faune et des Parcs . (2016), Intégration des enjeux écologiques dans les plans d’aménagement forestier intégré de 2018–2023, Cahier 2.1 – Enjeux liés à la structure d’âge des forêts. Direction de l’aménagement et de l’environnement forestiers, Ministère des Forêts de la Faune et des Parcs Québec, QC, 67
Moreau, L., Thiffault, E., and Beauregard, R. (2023), Assessing the effects of different harvesting practices on the forestry sector’s climate benefits potential: A stand level theoretical study in an eastern Canadian boreal forest
Moreau, L., Thiffault, E., Kurz, W. A., and Beauregard, R. (2023). Under what circumstances can the forest sector contribute to 2050 climate change mitigation targets? A study from forest ecosystems to landfill methane emissions for the province of Quebec, Canada. GCB Bioenergy 15, 1119–1139. doi: 10.1111/gcbb.13081
Morris, D. M., Reid, D. E. B., Kwiaton, M., Hunt, S. L., and Gordon, A. M. (2014). Comparing growth patterns of jack pine and black spruce in mixed natural stands and plantations. Écoscience 21, 1–10. doi: 10.2980/21-1-3646
Nabuurs, G., Mrabet, R., Abu Hatab, A., Bustamante, M., Clark, H., Havlík, P., et al., (2022) Agriculture, forestry and other land uses (chapter 7).
Nilsen, P., and Strand, L. T. (2013). Carbon stores and fluxes in even- and uneven-aged Norway spruce stands. Silva Fennica 47, 1–15. doi: 10.14214/sf.1024
Olguin, M., Wayson, C., Fellows, M., Birdsey, R., Smyth, C. E., Magnan, M., et al. (2018). Applying a systems approach to assess carbon emission reductions from climate change mitigation in Mexico’s forest sector. Environ. Res. Lett. 13:035003. doi: 10.1088/1748-9326/aaaa03
Pan, Y., Birdsey, R. A., Fang, J., Houghton, R., Kauppi, P. E., Kurz, W. A., et al. (2011). A large and persistent carbon sink in the world’s forests. Science 333, 988–993. doi: 10.1126/science.1201609
Paquette, A., and Messier, C. (2010). The role of plantations in managing the world's forests in the Anthropocene. Front. Ecol. Environ. 8, 27–34. doi: 10.1890/080116
Paradis, L., Thiffault, E., and Achim, A. (2019). Comparison of carbon balance and climate change mitigation potential of forest management strategies in the boreal forest of Quebec (Canada). Forestry Int. J. Forest Res. 92, 264–277. doi: 10.1093/forestry/cpz004
Perez-Garcia, J., Lippke, B., Comnick, J., and Manriquez, C. (2005). An assessment of carbon pools, storage, and wood products market substitution using life-cycle analysis results. Wood Fiber Sci. 37, 140–148.
Poulin, J. (2013a), Coupes totales. Fascicule 3.3. In Manuel de détermination des possibilités forestières 2013–2018, Bureau du forestier en chef, Gouvernement du Québec, Roberval, QC, 87–90
Poulin, J. (2013b), Éclaircie commerciale. Fascicule 3.5. Manuel de détermination des possibilités forestières 2013–2018. Bureau du forestier en chef (ed.), Gouvernement du Québec, Roberval, Qc, 95–98
Poulin, J. (2013c), Coupe progressive irrégulière. Fascicule 3.7. Manuel de détermination des possibilités forestières 2013–2018, Bureau du forestier en chef, Gouvernement du Québec, Roberval, Qc, 103–107
Prégent, G., Bertrand, V., and Charette, L. (1996), Tables préliminaires de rendement pour les plantations d'Épinette noire au Québec. Direction de la recherche forestière, Ministère des Ressources naturelles du Québec, 70
Prégent, G., Picher, G., and Auger, I. (2010), Tarif de cubage, tables de rendement et modèles de croissance pour les plantations d'épinette blanche au Québec. Ministère des ressources naturelles et de la faune, Direction de la recherche forestière: Québec
Pukkala, T. (2014). Does biofuel harvesting and continuous cover management increase carbon sequestration? Forest Policy Econ. 43, 41–50. doi: 10.1016/j.forpol.2014.03.004
Pukkala, T. (2017). Does management improve the carbon balance of forestry? Forestry Int. J. Forest Res. 90, 125–135. doi: 10.1093/forestry/cpw043
Raymond, P., Bédard, S., Roy, V., Larouche, C., and Tremblay, S. (2009). The irregular Shelterwood system: review, classification, and potential application to forests affected by partial disturbances. J. For. 107, 405–413. doi: 10.1093/jof/107.8.405
Rijal, B., LeBel, L., Martell, D. L., Gauthier, S., Lussier, J. M., and Raulier, F. (2018). Value-added forest management planning: a new perspective on old-growth forest conservation in the fire-prone boreal landscape of Canada. For. Ecol. Manag. 429, 44–56. doi: 10.1016/j.foreco.2018.06.045
Sathre, R., and O’Connor, J. (2010). Meta-analysis of greenhouse gas displacement factors of wood product substitution. Environ. Sci. Pol. 13, 104–114. doi: 10.1016/j.envsci.2009.12.005
Senez-Gagnon, F., Thiffault, E., Paré, D., Achim, A., and Bergeron, Y. (2018). Dynamics of detrital carbon pools following harvesting of a humid eastern Canadian balsam fir boreal forest. For. Ecol. Manag. 430, 33–42. doi: 10.1016/j.foreco.2018.07.044
Skog, K. E. (2008). Sequestration of carbon in harvested wood products for the United States. For. Prod. J. 58, 56–72.
Skytt, T., Englund, G., and Jonsson, B. (2021). Climate mitigation forestry – temporal trade-offs. Environ. Res. Lett. 16:114037. doi: 10.1088/1748-9326/ac30fa
Smyth, C., Rampley, G., Lemprière, T. C., Schwab, O., and Kurz, W. A. (2017). Estimating product and energy substitution benefits in national-scale mitigation analyses for Canada. GCB Bioenergy 9, 1071–1084. doi: 10.1111/gcbb.12389
Smyth, C. E., Smiley, B. P., Magnan, M., Birdsey, R., Dugan, A. J., Olguin, M., et al. (2018). Climate change mitigation in Canada’s forest sector: a spatially explicit case study for two regions. Carbon Balance Manag. 13:11. doi: 10.1186/s13021-018-0099-z
Smyth, C., Stinson, G., Neilson, E., Lemprière, T., Hafer, M., Rampley, G., et al. (2014). Quantifying the biophysical climate change mitigation potential of Canada's forest sector. Biogeosciences 11, 3515–3529. doi: 10.5194/bg-11-3515-2014
Smyth, C. E., Xu, Z., Lemprière, T. C., and Kurz, W. A. (2020). Climate change mitigation in British Columbia's forest sector: GHG reductions, costs, and environmental impacts. Carbon Balance Manag. 15, 1–22. doi: 10.1186/s13021-020-00155-2
Strukelj, M., Brais, S., and Paré, D. (2015). Nine-year changes in carbon dynamics following different intensities of harvesting in boreal aspen stands. Eur. J. For. Res. 134, 737–754. doi: 10.1007/s10342-015-0880-4
Sun, M., Wang, Y., Shi, L., and Klemeš, J. J. (2018). Uncovering energy use, carbon emissions and environmental burdens of pulp and paper industry: a systematic review and meta-analysis. Renew. Sust. Energ. Rev. 92, 823–833. doi: 10.1016/j.rser.2018.04.036
Ter-Mikaelian, M. T., Colombo, S. J., and Chen, J. (2008). Fact and fantasy about forest carbon. For. Chron. 84, 166–171. doi: 10.5558/tfc84166-2
Thiffault, N., Chalifour, D., and Bélanger, L. (2013). Enrichment planting of Picea glauca in boreal mixedwoods: can localized site preparation enhance early seedling survival and growth? New For. 44, 533–546. doi: 10.1007/s11056-012-9361-5
Uri, V., Kukumägi, M., Aosaar, J., Varik, M., Becker, H., Aun, K., et al. (2022). The dynamics of the carbon storage and fluxes in scots pine (Pinus sylvestris) chronosequence. Sci. Total Environ. 817:152973. doi: 10.1016/j.scitotenv.2022.152973
Yan, S., de Bruin, K., Dennehy, E., and Curtis, J. (2021). Climate policies for freight transport: energy and emission projections through 2050. Transp. Policy 107, 11–23. doi: 10.1016/j.tranpol.2021.04.005
Keywords: forest carbon, ecosystems, wood products, sawmilling efficiency, transportation, partial cuts, plantation
Citation: Giasson L-A, Thiffault E, Lebel L and Carle J-F (2023) Carbon balance of forest management and wood production in the boreal forest of Quebec (Canada). Front. For. Glob. Change. 6:1242218. doi: 10.3389/ffgc.2023.1242218
Edited by:
Anna Walkiewicz, Polish Academy of Sciences, PolandReviewed by:
Xinyuan Wei, University of Maine, United StatesLingbo Dong, Northeast Forestry University, China
Copyright © 2023 Giasson, Thiffault, Lebel and Carle. This is an open-access article distributed under the terms of the Creative Commons Attribution License (CC BY). The use, distribution or reproduction in other forums is permitted, provided the original author(s) and the copyright owner(s) are credited and that the original publication in this journal is cited, in accordance with accepted academic practice. No use, distribution or reproduction is permitted which does not comply with these terms.
*Correspondence: Evelyne Thiffault, RXZlbHluZS50aGlmZmF1bHRAc2JmLnVsYXZhbC5jYQ==