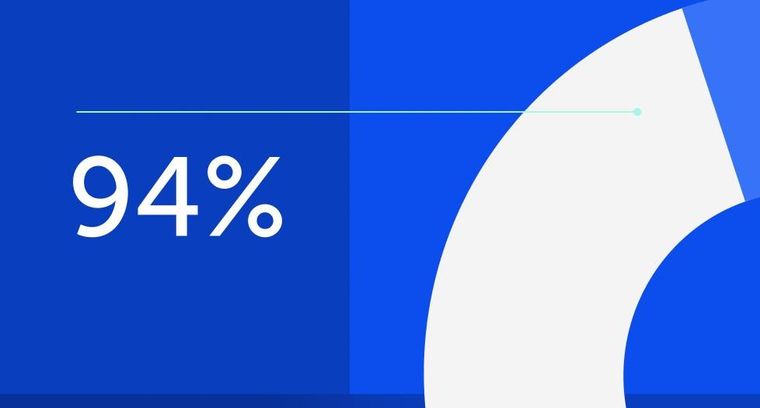
94% of researchers rate our articles as excellent or good
Learn more about the work of our research integrity team to safeguard the quality of each article we publish.
Find out more
ORIGINAL RESEARCH article
Front. For. Glob. Change, 18 October 2023
Sec. Forest Ecophysiology
Volume 6 - 2023 | https://doi.org/10.3389/ffgc.2023.1224838
This article is part of the Research TopicDynamics of Asia's and Australasia's forests in a changing worldView all 22 articles
Radial growth of trees can result in opposite wood (OW) and compression wood (CW) due to the varying impact of stem mechanical stress, such as that caused by gravity or wind. Previous research has identified higher xylem production in CW compared to OW. Yet, it remains unclear whether the difference in the number of xylem cells between OW and CW results from differences in growth rate or the duration of xylem cells. In this study, we collected wood microcores on a weekly basis from March 2019 to January 2020 in Pinus massoniana Lamb. located on a steep slope. Our objective was to compare the dynamic of cambial activity and resulting cellular anatomical parameters between OW and CW in a humid subtropical environment. Our results showed that the xylem phenology of OW and CW was generally consistent with the xylem cell division process beginning in early March and ceasing in November. The last latewood cell completed its differentiation at the end of December. The response of wood formation dynamics to climate was consistent in both OW and CW. Moreover, both wood types exhibited a limited development of the enlargement phase due to the heat and drought during the summer. The rate of cell division was responsible for 90.7% of the variability in the number of xylem cells. The CW xylem obtained a larger number of cells by increasing the rate of cell division and displayed thinner earlywood cells with larger lumens than OW cells. Our findings showed that the xylem of conifer species responds to mechanical stress by accelerating the cell division rate. As a result, we suggest calculating the ratio between OW and CW widths to reconstruct wind stress changes rather than calculating the residuals used in the current study.
Trees form annual rings due to the seasonal changes that occur within a year (Little and Bonga, 1974). The cambium, which is the tissue responsible for the production of new wooden cells, typically remains active from spring to autumn (Campoy et al., 2011; Singh et al., 2017). Environmental conditions before or during the growing season can directly influence the characteristics of the cells produced and the consequent functioning of xylem (Kellomäki and Wang, 2001; Rathgeber et al., 2016; Castagneri et al., 2017). Tree rings can provide insight into past climate conditions, for example, missing rings may indicate very cool summers (George and Anchukaitis, 2015) and intra-annual density fluctuations (IADFs) indicating extreme climate events during the growing season (De Micco et al., 2016; Zhang et al., 2020; Gao et al., 2021). The dynamics of tree growth can reflect past environmental changes and play a significant role in global change (Frank et al., 2022).
However, xylem cells within the same annual rings may exhibit distinct characteristics in different directions. For instance, mechanical stress, such as snow pressure or prevailing winds can cause the generation of specific wood structures, known as “reaction wood” (Sultana and Rahman, 2013). This type of wood induces asymmetric growth patterns (Groover, 2016) and is responsible for controlling the response to mechanical stress in conifers, unlike tension wood in hardwood species (Telewski, 2016). Current research indicates that the formation mechanism of compression wood (CW) is highly intricate and regulated by gravity, plant hormones, and gene expression (Du and Yamamoto, 2007; Yamashita et al., 2007, 2009). However, it is relatively clear that when trees experience mechanical stress that causes tilting, they adapt to the gravitational force, resulting in CW formation (Yamashita et al., 2007; Sultana and Rahman, 2013). The composition and structure of the CW’s cell wall undergo significant changes. Notably, CW exhibits an increase in cellulose content, and the content and composition of lignin also undergo alterations (Peng et al., 2019; Wang et al., 2020). CW is characterized by thicker cells with a gelatinous layer produced in the compressed stem region, leading to stiffer mechanical characteristics (Donaldson, 2001). Moreover, annual rings in the compressed stem region are usually larger and contain more cells than those on the opposite side of the stem (OW, opposite wood) (Donaldson et al., 2004).
The reconstruction of mechanical stress relies on the asymmetric growth of the stem, which typically indicates the timing and strength of the stem eccentricity (Zhang et al., 2017; Fang et al., 2022a). For example, Fang et al. (2022b) have proposed a method to reconstruct wind speed by analyzing shifts in the geometric center of tree rings. Although there is significant interest in comprehending the drivers of wood formation, particularly in response to climate, the formation of reaction wood has received little attention (Palombo et al., 2018). Therefore, quantifying and comprehending the differences between CW and OW or other types of wood could provide valuable insights into wood formation processes. Moreover, it could improve the use of annually datable markers in mechanical events-related reconstruction.
In this study, we investigated the intra-annual xylem formation dynamics of Pinus massoniana Lamb. in both OW and CW as well as their responses to climate and mechanical stress using microcore monitoring. We hypothesized that the intra-annual xylem formation dynamics and climate responses would exhibit similarities between OW and CW and that xylem would respond to mechanical stress by accelerating the rate of cell division. Specifically, our tasks were as follows: to (a) obtain the intra-annual xylem formation dynamics of P. massoniana Lamb. in both OW and CW and analyze the response of xylem cell dynamics to climate; (b) compare the anatomical characteristics of xylem cells in OW and CW; and (c) investigate the effect of the duration and rate of cell division on the number of xylem cells. By doing so, we hope to gain a better understanding of the physiological mechanisms underlying differential growth in a tree of different aspects. Results obtained could help to verify or propose a methodology for reconstructing regional wind speed.
This study was conducted at the Geshikao (GSK) nature reserve in Sanming City, Fujian Province (26°9′13″’N, 117°28′19″E, 320 m above sea level), which is a permanent monitoring site in the humid subtropical region of China (Figure 1A). Specifically, the site is located on a steep hill (slope angle of ca. 50°) in a mixed forest of bamboo and P. massoniana Lamb. and is 3 km away from the GSK field station (Figure 1B). The main soil type in the study area is mountain red soil followed by mountain yellow soil and purple soil.
Figure 1. Site location and sampling design. Location of the sampling site of Geshikao station in Sanming City of Fujian Province (A). The plot is located on a 45° slope within a mixed forest of bamboo and Pinus massoniana Lamb. (B). The monthly climate data of Yong’an meteorology station from 1954 to 2019 (C). The daily climate data of the GSK meteorology station during 2019 (D).
The mean annual temperature at the study site between 1954 and 2019 was 19.46°C, with the warmest and coldest monthly mean temperatures of 34.72 and 5.84°C being recorded in July and January, respectively. Annual precipitation at the site is 1,573 mm, roughly 75% of which is received from March to August (data from Yong’an meteorological station) (Figure 1C). Yong’an meteorological station is 25.5 km away from the monitoring site. Daily mean, maximum, and minimum air temperatures, as well as total precipitation, were collected by the micro-automatic meteorological station at the GSK field station (Figure 1D). The daily-scale meteorological data was simultaneously monitored with a 3-m high automated weather station (KME101, LSI-LASTEM, Milan, Italy). A set of probes for measuring air temperature (Ta; KME101, accuracy ± 0.2°C) and precipitation (P; DQA230.1#C, resolution 0.2 mm).
Four healthy P. massoniana Lamb. trees were selected. The monitored trees had an average diameter at breast height (DBH) of 37.7 ± 5.5 cm, height of 13.9 ± 1 m, and tree age of 45 ± 2 years. The sample size and tree parameters met the requirements of a micro-sampling study (Nehrbass-Ahles et al., 2014; Huang et al., 2018). From 11 March 2019 to 10 January 2020, weekly samples were taken at breast height (i.e., 1.3 m above the ground) using a Trephor (Rossi et al., 2006). Microcores were collected from both the CW (the widest tree rings) and the OW (the narrowest tree rings) (as seen in Supplementary Figure 2). Each core had a diameter of 2 mm and a length of 2–3 cm that contained at least two intact xylem and lamellar bands with adjacent phloem (Zheng et al., 2021). To maximize the use of limited sampling locations and minimize influences between adjacent sample areas, samples were taken in a zigzag pattern around the trunk, with a minimum distance of 5 cm between each sample (Supplementary Figure 3). A total of 360 samples were collected and immediately stored in a mixed solution of 70% alcohol and glacial acetic acid (mixing ratio: 9:1). In the laboratory, samples were dehydrated in different concentrations of alcohol (70, 90, 95, and 100%), soaked in limonene, and finally embedded in paraffin. Transverse sections of 12 μm were cut using a rotary microtome and stained with safranin and Astra blue (Li et al., 2019).
The three continuous columns of cell counts showing good quality and absence of disturbances were selected for the measurements of each sample, and the cells were identified as cambium cells, enlargement cells, wall thickening cells, and mature cells (Rathgeber et al., 2016). The average weekly number of cells in each differentiation phase was then expressed as a function of day of the year (DOY) (Rathgeber, 2012). The cambium cells are typically flattened and have very thin walls, and enlargement cells have an irregular radial diameter and are at least two times larger than cambium cells. Wall-thickening cells appear shiny under polarized light compared to enlargement cells, and mature cells are red-stained by safranin and have mature tubular cells (Camarero et al., 2010; Ren et al., 2018; Supplementary Figure 4). The onset of radial stem growth was determined by the appearance of the first enlargement cells, and radial stem growth was considered completed when wall-thickening cells no longer appeared (Rossi et al., 2006).
Using ImageJ (National Institutes of Health, Bethesda, MD, USA), we measured the cell anatomical parameters of the last collected samples after the end of the growing season (i.e., on 10 January 2020; DOY 375). These included the radial parameters: double cell wall thickness (2CWT), radial lumen diameter (LD), lumen area (LA), cell area (CA), and cell number (Num). Cell wall area (CWA) was calculated as the difference between the CA and LAs (Cuny et al., 2019). According to Mork’s criterion (Denne, 1989), we divided mature cells into earlywood cells (2CWT/LD < 0.5) and latewood cells (2CWT/LD ≥ 0.5).
The number of cells can differ largely between aspects due to differences in growth rate. Therefore, standardization of the number of xylem cells was necessary. This can be achieved by using the number of cambium xylem cells of the previous tree ring width as a reference (Rossi et al., 2006; Zhang et al., 2018). The formula used for this standardization is as follows:
where nci is the standardized number of xylem cells, ncmi is the measured number of xylem cells, rwm is the mean width of the previous tree rings for all samples, and rws is the width of the previous tree rings for each sample (Zheng et al., 2021).
Different from the simple unimodal growth characteristics in the alpine region, the radial growth in the humid subtropical region has a complex growth pattern (Huang et al., 2018; Liu et al., 2019; Zheng et al., 2021). Given that generalized additive models (GAMs) are data-driven, they are better able to describe complex intra-annual wood formation dynamics than traditional Gompertz functions (Cuny et al., 2013). So, we used GAMs to model the growth of cambium xylem cells (enlargement + wall thickening + mature cells) in both the CW and OW of each tree. The model is represented by the equation
In the equation, y is the response variable (the number of cambium xylem cells), x1 is the DOY and f represents the smoothing function. The Poisson link function is used given that the response variable is a count variable. Its derivative was further calculated to obtain the rate of cell division (Huang et al., 2018).
A model is constructed to demonstrate the correlation between the number of cambium xylem cells (Ncell), the mean rate (rm), and the duration (ΔtE) of cell production. The Ncell is the median number of cells remaining in the sample after the cells have stopped dividing. The rm is the average of the rate of cell division, and the ΔtE is calculated from observations, which represents the during of cell division, from the emergence to the disappearance of enlarged cells (Rathgeber et al., 2011; Ren et al., 2019):
Before determining the correlation between the number or rate of cells at different times and climate records, average meteorological variables were calculated over a 7- and 10-day period preceding the sampling date. This is because xylem cell growth is affected by climate with a lag effect (Prislan et al., 2016). The variations in key times of xylem phenology and wood anatomy between CW and OW were identified using ANOVA and Tukey’s test. Normality was confirmed for all critical dates before using ANOVA.
The seasonal dynamic of xylem cell numbers at different phase in both CW and OW were very similar. Cambial cells enlarged in early March and stopped dividing in November. The number of cambium cells fluctuated between three and seven throughout the growing season at both wood types and stabilized at ca. five at the end of the xylem enlargement phase (Figure 2A). At the initial sampling date (March 11, DOY 70), CW samples already presented cells in the enlargement phase, indicating that the cambium had begun to activate before the first date. In contrast, the OW cambium did not activate until the second sampling date (March 16, DOY 75). The seasonal dynamic of the enlarged cells exhibited a bimodal pattern. The enlarged cells reached a first growth peak in early April (DOY 100), and then gradually decreased until a secondary growth peak occurred in early October (DOY 280) (Figure 2B). The bimodal pattern in the wall thickening phase was less evident (Figure 2C), while the intra-annual dynamics of mature cells are characterized by a single “S” shaped change (Figure 2D).
Figure 2. Number of cambium cells (A), enlargement cells (B), wall thickening cells (C), and mature cells (D) of OW (red) and CW (blue) of Pinus massoniana Lamb. during 2019 at GSK field station. The dots and the bars represent the mean cell number and standard errors among the four selected trees, respectively.
The response of xylem cell numbers at different phase to climatic factors (minimum, mean and maximum temperatures, and precipitation) was highly consistent between CW and OW. The effects of temperature and precipitation on xylem cell numbers between CW and OW were independent of time periods. Precipitation had a weak promoting effect on cells in the mature phase and the cumulated cells (P > 0.05). Temperature had an inhibiting effect on cells in the mature phase and the cumulated cells (P > 0.05), while the temperature 10 days before sampling significantly inhibited cells in the mature phase and the cumulated cells (P < 0.05) (Figure 3).
Figure 3. The correlation between the number of mature cells (MC), the number of cumulated cells (CC), and climatic variables at the monitoring site during 2019 of OW and CW. Mean, minimum, and maximum temperature (°C) and total precipitation (mm) on a 7-day (A) and 10-day (B) duration prior to the sampling. Red stands for positive correlation while blue is negative. The number in the box indicates the correlation coefficient (r value). Black numbers indicate P > 0.05, and white numbers indicate P < 0.05.
The phenological timings between OW and CW were insignificantly different, while the rate of xylem cell division was significantly different. The cambium activates at DOY 75 ± 3 (OW) and DOY 73 ± 5 (CW), and cell division ended at DOY 307 ± 5 (OW) and DOY 321 ± 7 (CW), leading to ΔtE of 232 ± 8 days (OW) and 248 ± 12 days (CW), respectively. The first latewood cells appeared at DOY 193 ± 18 (OW) and DOY 179 ± 8 (CW), respectively. The date of first appearance of cell wall thickening cells was on DOY 82 ± 10 (OW) and DOY 79 ± 8 (CW). The cambium entered the dormancy stage at DOY 352 ± 3 (OW) and DOY 359 ± 5 (CW), respectively. There was no significant difference in the growing season length between OW and CW (279 ± 13 and 284 ± 13 days for OW and CW, respectively; Table 1). The GAMs model explained 80.9% (83.9%) of the intra-annual dynamics of xylem cells at the OW (CW) (Figure 4A). The seasonal dynamics of the cell division rate showed a bimodal shape, with the cell division rate reaching a first growth peak in early March and a second growth peak in the middle of September (Figure 4B). The rm for OW was 0.126 cells/day and the rm for CW was 0.154 cells/day. When compared to OW, xylem cell production and rm of CW increased by 22.2 and 24.8%, respectively. However, the ΔtE for CW was only 6% longer than OW.
Table 1. Critical dates of xylem phenology between OW (opposite wood) and CW (compressed wood) of Pinus massoniana Lamb.
Figure 4. The dynamic (A) and the cell growth rate (B) of cumulated cells (enlargement cells + wall thickening cells + mature cells) of OW (red) and CW (blue) of Pinus massoniana Lamb. modeled by the GAM model during 2019.
The response of cell production rates at different periods (earlywood phase or latewood phase) to climatic factors (minimum, mean and maximum temperatures, and precipitation) was highly consistent between CW and OW. During earlywood formation, temperature and precipitation did not significantly affect the xylem cell division rate during earlywood formation, while the precipitation the 10 days before sampling significantly promoted the production rate of earlywood cells (P < 0.05). However, during latewood growth temperature significantly facilitated the rate of cell production. The mean temperature of 7 days before sampling and both the mean and maximum temperature 10 days before sampling significantly promoted the latewood cell production rate (P < 0.05) (Figure 5).
Figure 5. The correlation between the cell growth rate of earlywood phase (A), latewood phase (B), and climatic variables at the monitoring site during 2019. Mean, minimum, and maximum temperature (°C) and total precipitation (mm) on a 7-day (P7) and 10-day (P10) duration prior to the sampling. Red stand for positive correlation while blue is negative. Black numbers indicate P > 0.05, white numbers indicate P < 0.05, and yellow numbers indicate P < 0.01.
A physical model was constructed to determine whether xylem cell production is controlled by rm or ΔtE. The model, which was based on Ncell, rm, and ΔtE, was found to have a good fit (COD = 70.2%) (Figure 6A). Sensitivity analysis of the model revealed that an increase in rm resulted in a corresponding increase in Ncell, while ΔtE had a minor effect on Ncell (Figure 6B). The model indicated that a majority portion (90.7%) of the variability in Ncell was attributed to rm. When rm remains constant at the mean and ΔtE varies around its mean by twice its standard deviation, Ncell varies only in the range of 36.5–39.2 cells (a range of variation of 2.7 cells). Similarly, when ΔtE was constant at its mean and rm varied around its mean within twice its standard deviation, Ncell varied within a range of 24.6–51.1 cells (a range of variation of 26.5 cells).
Figure 6. The physical simple model between the number of cambium xylem cells (Ncell) and the rate (rm) and duration (ΔtE) of cell division (A). Sensitivity analysis of the physical model (B). The dashed lines represent the means, and the frame delimits the area of the mean ± standard deviation; the black lines indicate the number of cells.
A comparison of microcore sections revealed differences in xylem cell anatomy between CW and OW (Supplementary Figures 2B, C). Compared to OW, CW earlywood cells exhibited distinct anatomical changes. Specifically, compared to OW earlywood cells, the 2CWT of CW was slightly thinner (P > 0.05); the LD of CW earlywood cells was significantly larger (P < 0.05); the LA and CA were larger but insignificant (P > 0.05); and the CWA and Num increased significantly (P < 0.05) (Figures 7A, C, E, G, I, K). Compared to OW, CW had smaller 2CWT, LA, CWA, LD, and CA but a higher number of latewood cells. However, it is worth noting that none of the differences in latewood anatomy were significant (Figures 7B, D, F, H, J, L). When comparing the morphology of earlywood to latewood cells, it was observed that earlywood cells in CW had larger cells with thinner walls and larger lumens, while latewood cells were significantly smaller with thicker walls and smaller lumens.
Figure 7. Comparison of the wood anatomical parameters between opposite wood (OW, red) and compressed wood (CW, blue). Double cell wall thickness (2CWT), radial lumen diameter (LD), lumen area (LA), cell area (CA), cell wall area (CWA), and number (Num) of earlywood (Ew) (A,C,E,G,I,K) and latewood (Lw) (B,D,F,H,J,L). Numerical values are mean ± standard error. Shades of red indicate a significant difference at 0.05 probability.
In this study, xylem cell division of P. massoniana Lamb. began in March and ceased in November. The growth duration was prolonged by 3–4 months compared to coniferous species in cold environments (Rossi et al., 2016) but was consistent with the findings of Huang et al. (2018) and Zheng et al. (2021) who reported that the regional climate plays a significant role in wood formation processes of subtropical conifers. A humid and warm climate is optimal for leaf photosynthesis, which will further promote the radial growth of trees (Ding et al., 2021). When favorable conditions occur in the spring, cambium cells begin to divide and expand (Li et al., 2017). Turgor within the cells is essential to maintain the expansion pressure required for division and expansion, and the availability of water plays a crucial role at this stage (as seen in Supplementary Figure 1; Cabon et al., 2020). At the early and late stages of cell expansion, the temperature in the study area is already high, but the low precipitation levels do not effectively replenish soil moisture, which has an inhibiting effect on the production of expanding cells (Song et al., 2022).
It is worth noting that the seasonal dynamic of xylem cells at the enlargement and wall thickening phase of P. massoniana Lamb. in the study area presented a bimodal pattern, which is in line with previous reports in the Mediterranean region that is driven by seasonal changes in water availability (Pacheco et al., 2016; Campelo et al., 2018). Radial growth of P. massoniana Lamb. in the study area is affected by summer heat and drought (Li et al., 2016). Studies using tree-ring isotopes and dendrometer measurements suggest that tree growth of subtropical conifers is constrained by summer heat and drought (Liu et al., 2019; Bing et al., 2022), leading to the bimodal seasonal growth patterns of trees in humid subtropical China. As the latewood cells develop, the regulation of cell production rates transitions from being solely dependent on precipitation to becoming modulated by temperature. The occurrence of summer drought results in decreased activity of the cambium or even dormancy, which subsequently causes a seasonal alteration in limiting factors that restrict the growth rate of xylem cells (Cartenì et al., 2018; Liu X. et al., 2018).
Several studies have suggested that wood production is correlated with the length of the growing season (Rossi et al., 2012; Liu S. et al., 2018; Camarero et al., 2022). However, others contend that wood production may be more strongly influenced by the rate of cell production rather than growing season length (Ren et al., 2019; Jiang et al., 2021). Our research demonstrated that a faster rate cell production leads to larger wood production on the CW. The tree-ring of P. massoniana Lamb. exhibits evident eccentricity in order to regain their upright position via maintaining balance (Bamber, 2001; Donaldson and Singh, 2013). The tree growth responds to mechanical stress in different aspects by changing the rate of cell production.
During the sampling process, it was observed that on steep slopes, the branches of P. massoniana Lamb. were primarily located on the downhill side, and the young leaves and shoots of the canopy were the main sites of phytohormone production (Huang et al., 2014; Ding et al., 2021), indicating that phytohormones play a significant role in the xylem formation of coniferous species (Guo et al., 2022). Studies have shown that the concentration of phytohormones in the trunk affects the rate of division and differentiation, size, and lignin accumulation in xylem cells (Perrot-Rechenmann, 2010; Sorce et al., 2013; Rathgeber et al., 2016; Buttò et al., 2020). At the start of xylem activity, before the plant begins to sprout, there is no difference in phytohormone concentration between OW and CW, and the timing of xylem initiation is also similar. As leaf extension and maturation occur, phytohormones are enriched at CW and xylem cells are rapidly produced and expanded (Rossi et al., 2009; Guo et al., 2022), influencing tubular cell lumen and CWA (Majda and Robert, 2018). As a result, earlywood cells at CW are significantly larger in lumen and CWA than at OW. The transition from earlywood to latewood in xylem cells occurs after leaf bursting and extension is completed (Fajstavr et al., 2019), when phytohormone concentrations in the stem are at low levels and a slight reduction in cell size at CW occurs due to gravity (Tarmian and Azadfallah, 2009; Palombo et al., 2018). This leads to a significant increase in the size and number of xylem earlywood cells at CW.
Previous studies have used the difference of tree ring width between the opposite and compression sides (CW-OW) to quantify the effect of wind stress on trees (Fang et al., 2022a). This method extracts a composite signal that encompasses a prolonged growing season and increased growth rate, operating under the premise that xylem production is co-regulated by the duration of the growing season and the growth rate (Rossi et al., 2012; Camarero et al., 2022). In our study, we found that the greater accumulation of xylem cells on the CW side was primarily due to a higher rate of cell production rather than the duration of xylem cell production. The xylem formation process was found to be consistent between OW and CW in response to climate, with the CW side responding to mechanical stress by accelerating its cell division rate. Therefore, we suggest that the impact of external stress on trees can be studied by considering the ratio between the two sides simultaneously. When reconstructing regional wind speeds, using the ratio chronology will provide a more accurate reflection of wind speed changes than a difference chronology.
However, the physiological response of trees to prevailing winds is complex, as wind not only generates mechanical stresses in different directions compared to the steady mechanical stresses generated by gravity but also accelerates related physiological processes such as leaf transpiration (Telewski, 2012; Mitchell, 2013). Therefore, we recommend that future studies to accurately understand the effects of strong winds on wood formation in different directions within trees should include monitoring of multiple tree species in strong wind conditions to further clarify the effects of wind speed on tree growth and cambium activity.
This study investigates the dynamics of xylem formation in P. massoniana Lamb. and compares the phenological and anatomical characteristics of trees growing on steep slopes facing opposite directions (OW and CW) in the humid subtropics. Our findings demonstrate that xylem formation responds similarly to climate in both OW and CW. Moreover, the number of cells in the enlargement phase was limited by summer drought in P. massoniana Lamb. in both directions. We observed that the cell division rate of xylem cells in CW was accelerated, due to the effect of gravity, although there was no significant difference in cell division time. The earlywood cells in CW had larger cell lumens and thinner cell walls, while the latewood cells had smaller cell lumens and thicker cell walls. These differences in cellular anatomical parameters suggest that phytohormones may influence xylem cell morphology. The results of our study imply that trees growing in locations with different mechanical stress primarily modify the rate of cell division. So, we suggest calculating the ratio between OW and CW widths to reconstruct wind stress changes rather than calculating the residuals used in the current study.
The raw data supporting the conclusions of this article will be made available by the authors, without undue reservation.
KF, ZZ, and FZ designed the experiment. CW and ZZ performed the material preparation, data collection, and analysis. CW wrote the first draft of the manuscript. XL, PF, and JG commented on previous versions of the manuscript. All authors contributed to the preparation of the manuscript, and read and approved the final manuscript.
We acknowledged support from the National Natural Science Foundation of China (41971022, 42101082, 41888101, and 41772180), the Strategic Priority Research Program of the Chinese Academy of Sciences (XDB26020000), the fellowship for Youth Talent Support Program of Fujian Province, and the innovation team project (IRTL1705).
We thank Meiqing Yang and Ping Chen for their help during field sampling.
The authors declare that the research was conducted in the absence of any commercial or financial relationships that could be construed as a potential conflict of interest.
All claims expressed in this article are solely those of the authors and do not necessarily represent those of their affiliated organizations, or those of the publisher, the editors and the reviewers. Any product that may be evaluated in this article, or claim that may be made by its manufacturer, is not guaranteed or endorsed by the publisher.
The Supplementary Material for this article can be found online at: https://www.frontiersin.org/articles/10.3389/ffgc.2023.1224838/full#supplementary-material
Bamber, R. K. (2001). A general theory for the origin of growth stresses in reaction wood: How trees stay upright. IAWA J. 22, 205–212. doi: 10.1163/22941932-90000279
Bing, X., Fang, K., Gong, X., Wang, W., Xu, C., Li, M., et al. (2022). The intra-annual intrinsic water use efficiency dynamics based on an improved model. Clim. Change 172:16. doi: 10.1007/s10584-022-03368-1
Buttò, V., Deslauriers, A., Rossi, S., Rozenberg, P., Shishov, V., and Morin, H. (2020). The role of plant hormones in tree-ring formation. Trees 34, 315–335. doi: 10.1007/s00468-019-01940-4
Cabon, A., Fernández-de-Uña, L., Gea-Izquierdo, G., Meinzer, F. C., Woodruff, D. R., Martínez-Vilalta, J., et al. (2020). Water potential control of turgor-driven tracheid enlargement in Scots pine at its xeric distribution edge. N. Phytol. 225, 209–221. doi: 10.1111/nph.16146
Camarero, J. J., Campelo, F., Colangelo, M., Valeriano, C., Knorre, A., Solé, G., et al. (2022). Decoupled leaf-wood phenology in two pine species from contrasting climates: Longer growing seasons do not mean more radial growth. Agric. For. Meteorol. 327:109223. doi: 10.1016/j.agrformet.2022.109223
Camarero, J. J., Olano, J. M., and Parras, A. (2010). Plastic bimodal xylogenesis in conifers from continental Mediterranean climates. N. Phytol. 185, 471–480. doi: 10.1111/j.1469-8137.2009.03073.x
Campelo, F., Gutiérrez, E., Ribas, M., Sánchez-Salguero, R., Nabais, C., and Camarero, J. J. (2018). The facultative bimodal growth pattern in Quercus ilex–a simple model to predict sub-seasonal and inter-annual growth. Dendrochronologia 49, 77–88. doi: 10.1016/j.dendro.2018.03.001
Campoy, J. A., Ruiz, D., and Egea, J. (2011). Dormancy in temperate fruit trees in a global warming context: A review. Sci. Hortic. 130, 357–372. doi: 10.1016/j.scienta.2011.07.011
Cartenì, F., Deslauriers, A., Rossi, S., Morin, H., De Micco, V., Mazzoleni, S., et al. (2018). The physiological mechanisms behind the earlywood-to-latewood transition: A process-based modeling approach. Front. Plant Sci. 9:1053. doi: 10.3389/fpls.2018.01053
Castagneri, D., Fonti, P., von Arx, G., and Carrer, M. (2017). How does climate influence xylem morphogenesis over the growing season? Insights from long-term intra-ring anatomy in Picea abies. Ann. Bot. 119, 1011–1020. doi: 10.1093/aob/mcw274
Cuny, H. E., Fonti, P., Rathgeber, C. B., von Arx, G., Peters, R. L., and Frank, D. C. (2019). Couplings in cell differentiation kinetics mitigate air temperature influence on conifer wood anatomy. Plant Cell Environ. 42, 1222–1232. doi: 10.1111/pce.13464
Cuny, H. E., Rathgeber, C. B., Kiessé, T. S., Hartmann, F. P., Barbeito, I., and Fournier, M. (2013). Generalized additive models reveal the intrinsic complexity of wood formation dynamics. J. Exp. Bot. 64, 1983–1994. doi: 10.1093/jxb/ert057
De Micco, V., Campelo, F., De Luis, M., Bräuning, A., Grabner, M., Battipaglia, G., et al. (2016). Intra-annual density fluctuations in tree rings: How, when, where, and why? IAWA J. 37, 232–259. doi: 10.1163/22941932-20160132
Denne, M. P. (1989). Definition of latewood according to Mork (1928). IAWA J. 10, 59–62. doi: 10.1163/22941932-90001112
Ding, X., Jiang, Y., Xue, F., Zhang, Y., Wang, M., Kang, M., et al. (2021). Intra-annual growth dynamics of Picea meyeri needles, shoots, and stems on Luya Mountain, North-central China. Trees 35, 637–648. doi: 10.1007/s00468-020-02065-9
Donaldson, L. A. (2001). Lignification and lignin topochemistry—an ultrastructural view. Phytochemistry 57, 859–873. doi: 10.1016/S0031-9422(01)00049-8
Donaldson, L. A., Grace, J., and Downes, G. M. (2004). Within-tree variation in anatomical properties of compression wood in radiata pine. IAWA J. 25, 253–271. doi: 10.1163/22941932-90000364
Donaldson, L. A., and Singh, A. P. (2013). “Formation and structure of compression wood,” in Cellular aspects of wood formation, ed. J. Fromm (Berlin: Springer Berlin Heidelberg), 225–256. doi: 10.1007/978-3-642-36491-4_9
Du, S., and Yamamoto, F. (2007). An overview of the biology of reaction wood formation. J. Integr. Plant Biol. 49, 131–143. doi: 10.1111/j.1744-7909.2007.00427.x
Fajstavr, M., Bednářová, E., Nezval, O., Giagli, K., Gryc, V., Vavrčík, H., et al. (2019). How needle phenology indicates the changes of xylem cell formation during drought stress in Pinus sylvestris L. Dendrochronologia 56:125600. doi: 10.1016/j.dendro.2019.05.004
Fang, K., Bai, M., Azorin-Molina, C., Dong, Z., Camarero, J. J., Zheng, Z., et al. (2022a). Wind speed reconstruction from a tree-ring difference index in northeastern Inner Mongolia. Dendrochronologia 72:125938. doi: 10.1016/j.dendro.2022.125938
Fang, K., He, M., Bai, M., Dong, Z., Linderholm, H. W., Azorin-Molina, C., et al. (2022b). The potential to use variations in tree-ring geometric center to estimate past wind speed change. Nat. Hazards Res. 2, 132–137. doi: 10.1016/j.nhres.2022.04.004
Frank, D., Fang, K., and Fonti, P. (2022). “Dendrochronology: Fundamentals and innovations,” in Stable isotopes in tree rings. Tree physiology, Vol. 8, eds R. T. W. Siegwolf, J. R. Brooks, J. Roden, and M. Saurer (Cham: Springer), doi: 10.1007/978-3-030-92698-4_2
Gao, J., Rossi, S., and Yang, B. (2021). Origin of intra-annual density fluctuations in a semi-arid area of Northwestern China. Front. Plant Sci. 12:777753. doi: 10.3389/fpls.2021.777753
George, S. S., and Anchukaitis, K. J. (2015). On the AD 1815 tambora eruption and the matter of misplaced tree rings. Past Glob. Changes Magaz. 23, 60–61. doi: 10.1002/jgrd.50692
Groover, A. (2016). Gravitropisms and reaction woods of forest trees–evolution, functions and mechanisms. N. Phytol. 211, 790–802. doi: 10.1111/nph.13968
Guo, X., Huang, J. G., Buttò, V., Luo, D., Shen, C., Li, J., et al. (2022). Auxin concentration and xylem production of Pinus massoniana in a subtropical forest in south China. Tree Physiol. 42, 317–324. doi: 10.1093/treephys/tpab110
Huang, J. G., Deslauriers, A., and Rossi, S. (2014). Xylem formation can be modeled statistically as a function of primary growth and cambium activity. N. Phytol. 203, 831–841. doi: 10.1111/nph.12859
Huang, J. G., Guo, X., Rossi, S., Zhai, L., Yu, B., Zhang, S., et al. (2018). Intra-annual wood formation of subtropical Chinese red pine shows better growth in dry season than wet season. Tree Physiol. 38, 1225–1236. doi: 10.1093/treephys/tpy046
Jiang, Y., Zhang, X., Chhin, S., and Zhang, J. (2021). A bimodal pattern and age-related growth of intra-annual wood cell development of Chinese fir in subtropical China. Front. Plant Sci. 12:757438. doi: 10.3389/fpls.2021.757438
Kellomäki, S., and Wang, K. Y. (2001). Growth and resource use of birch seedlings under elevated carbon dioxide and temperature. Ann. Bot. 87, 669–682. doi: 10.1006/anbo.2001.1393
Li, X., Liang, E., Gričar, J., Rossi, S., Čufar, K., and Ellison, A. M. (2017). Critical minimum temperature limits xylogenesis and maintains treelines on the southeastern Tibetan Plateau. Sci. Bull. 62, 804–812. doi: 10.1016/j.scib.2017.04.025
Li, X., Rossi, S., and Liang, E. (2019). The onset of xylogenesis in Smith fir is not related to outer bark thickness. Am. J. Bot. 106, 1386–1391.
Li, Y., Fang, K., Cao, C., Li, D., Zhou, F., Dong, Z., et al. (2016). A tree-ring chronology spanning 210 years in the coastal area of southeastern China, and its relationship with climate change. Clim. Res. 67, 209–220. doi: 10.3354/cr01376
Little, C. H. A., and Bonga, J. M. (1974). Rest in the cambium of Abies balsamea. Can. J. Bot. 52, 1723–1730. doi: 10.1139/b74-224
Liu, S., Li, X., Rossi, S., Wang, L., Li, W., Liang, E., et al. (2018). Differences in xylogenesis between dominant and suppressed trees. Am. J. Bot. 105, 950–956. doi: 10.1002/ajb2.1089
Liu, X., Nie, Y., and Wen, F. (2018). Seasonal dynamics of stem radial increment of Pinus taiwanensis Hayata and its response to environmental factors in the Lushan Mountains, Southeastern China. Forests 9:387. doi: 10.3390/f9070387
Liu, X., Wang, C., and Zhao, J. (2019). Seasonal drought effects on intra-annual stem growth of Taiwan Pine along an elevational gradient in subtropical China. Forests 10:1128. doi: 10.3390/f10121128
Majda, M., and Robert, S. (2018). The role of auxin in cell wall expansion. Int. J. Mol. Sci. 19:951. doi: 10.3390/ijms19040951
Mitchell, S. J. (2013). Wind as a natural disturbance agent in forests: A synthesis. For. Int. For. Res. 86, 147–157. doi: 10.1093/forestry/cps058
Nehrbass-Ahles, C., Babst, F., Klesse, S., Nötzli, M., Bouriaud, O., Neukom, R., et al. (2014). The influence of sampling design on tree ring-based quantification of forest growth. Glob. Change Biol. 20, 2867–2885. doi: 10.1111/gcb.12599
Pacheco, A., Camarero, J. J., and Carrer, M. (2016). Linking wood anatomy and xylogenesis allows pinpointing of climate and drought influences on growth of coexisting conifers in continental Mediterranean climate. Tree Physiol. 36, 502–512. doi: 10.1093/treephys/tpv125
Palombo, C., Fonti, P., Lasserre, B., Cherubini, P., Marchetti, M., and Tognetti, R. (2018). Xylogenesis of compression and opposite wood in mountain pine at a Mediterranean treeline. Ann. For. Sci. 75:93. doi: 10.1007/s13595-018-0773-z
Peng, H., Salmén, L., Stevanic, J. S., and Lu, J. (2019). Structural organization of the cell wall polymers in compression wood as revealed by FTIR microspectroscopy. Planta 250, 163–171. doi: 10.1007/s00425-019-03158-7
Perrot-Rechenmann, C. (2010). Cellular responses to auxin: Division versus expansion. Cold Spring Harb. Perspect. Biol. 2:a001446. doi: 10.1101/cshperspect.a001446
Prislan, P., Gričar, J., De Luis, M., Novak, K., Martinez del Castillo, E., Schmitt, U., et al. (2016). Annual cambial rhythm in Pinus halepensis and Pinus sylvestris as indicator for climate adaptation. Front. Plant Sci. 7:1923. doi: 10.3389/fpls.2016.01923
Rathgeber, C. B. K. (2012). Cambial activity and wood formation: Data manipulation, visualisation and analysis using R. R package version 1.4-1.
Rathgeber, C. B., Cuny, H. E., and Fonti, P. (2016). Biological basis of tree-ring formation: A crash course. Front. Plant Sci. 7:734. doi: 10.3389/fpls.2016.00734
Rathgeber, C. B., Rossi, S., and Bontemps, J. D. (2011). Cambial activity related to tree size in a mature silver-fir plantation. Ann. Bot. 108, 429–438. doi: 10.1093/aob/mcr168
Ren, P., Rossi, S., Camarero, J. J., Ellison, A. M., Liang, E., and Peñuelas, J. (2018). Critical temperature and precipitation thresholds for the onset of xylogenesis of Juniperus przewalskii in a semi-arid area of the north-eastern Tibetan Plateau. Ann. Bot. 121, 617–624. doi: 10.1093/aob/mcx188
Ren, P., Ziaco, E., Rossi, S., Biondi, F., Prislan, P., and Liang, E. (2019). Growth rate rather than growing season length determines wood biomass in dry environments. Agric. For. Meteorol. 271, 46–53. doi: 10.1016/j.agrformet.2019.02.031
Rossi, S., Anfodillo, T., and Menardi, R. (2006). Trephor: A new tool for sampling microcores from tree stems. IAWA J. 27, 89–97. doi: 10.1163/22941932-90000139
Rossi, S., Anfodillo, T., Čufar, K., Cuny, H. E., Deslauriers, A., Fonti, P., et al. (2016). Pattern of xylem phenology in conifers of cold ecosystems at the Northern Hemisphere. Glob. Change Biol. 22, 3804–3813. doi: 10.1111/gcb.13317
Rossi, S., Morin, H., and Deslauriers, A. (2012). Causes and correlations in cambium phenology: Towards an integrated framework of xylogenesis. J. Exp. Bot. 63, 2117–2126. doi: 10.1093/jxb/err423
Rossi, S., Rathgeber, C. B., and Deslauriers, A. (2009). Comparing needle and shoot phenology with xylem development on three conifer species in Italy. Ann. For. Sci. 66:206. doi: 10.1051/forest/2008088
Singh, R. K., Svystun, T., AlDahmash, B., Jönsson, A. M., and Bhalerao, R. P. (2017). Photoperiod-and temperature-mediated control of phenology in trees–a molecular perspective. N. Phytol. 213, 511–524. doi: 10.1111/nph.14346
Song, W., Zhao, B., Mu, C., Ballikaya, P., Cherubini, P., and Wang, X. (2022). Moisture availability influences the formation and characteristics of earlywood of Pinus tabuliformis more than latewood in northern China. Agric. For. Meteorol. 327:109219. doi: 10.1016/j.agrformet.2022.109219
Sorce, C., Giovannelli, A., Sebastiani, L., and Anfodillo, T. (2013). Hormonal signals involved in the regulation of cambial activity, xylogenesis and vessel patterning in trees. Plant Cell Rep. 32, 885–898. doi: 10.1007/s00299-013-1431-4
Sultana, R. S., and Rahman, M. (2013). A review on structures of secondary wall in reaction wood fiber of hardwood species. Plant 1, 54–59. doi: 10.11648/j.plant.20130105.12
Tarmian, A., and Azadfallah, M. (2009). Variation of cell features and chemical composition in spruce consisting of opposite, normal, and compression wood. Bioresources 4, 194–204. doi: 10.1515/HF.2009.036
Telewski, F. W. (2012). Is windswept tree growth negative thigmotropism? Plant Sci. 184, 20–28. doi: 10.1016/j.plantsci.2011.12.001
Telewski, F. W. (2016). “Flexure wood: Mechanical stress induced secondary xylem formation,” in Secondary xylem biology, (Cambridge, MA: Academic Press), 73–91. doi: 10.1016/B978-0-12-802185-9.00005-X
Wang, D., Lin, L., and Fu, F. (2020). Deformation mechanisms of wood cell walls under tensile loading: A comparative study of compression wood (CW) and normal wood (NW). Cellulose 27, 4161–4172. doi: 10.1007/s10570-020-03095-9
Yamashita, S., Yoshida, M., and Yamamoto, H. (2009). Relationship between development of compression wood and gene expression. Plant Sci. 176, 729–735. doi: 10.1016/j.plantsci.2009.02.017
Yamashita, S., Yoshida, M., Takayama, S., and Okuyama, T. (2007). Stem-righting mechanism in gymnosperm trees deduced from limitations in compression wood development. Ann. Bot. 99, 487–493. doi: 10.1093/aob/mcl270
Zhang, J., Alexander, M. R., Gou, X., Deslauriers, A., Fonti, P., Zhang, F., et al. (2020). Extended xylogenesis and stem biomass production in Juniperus przewalskii Kom. during extreme late-season climatic events. Ann. For. Sci. 77:99. doi: 10.1007/s13595-020-01008-1
Zhang, J., Gou, X., Pederson, N., Zhang, F., Niu, H., Zhao, S., et al. (2018). Cambial phenology in Juniperus przewalskii along different altitudinal gradients in a cold and arid region. Tree Physiol. 38, 840–852. doi: 10.1093/treephys/tpx160
Zhang, Y., Fang, K., Zhou, F., Dong, Z., Li, Y., and Zhang, P. (2017). Reconstruction of soil erosion rates from exposed roots in southeast China. Asian Geogr. 34, 91–105. doi: 10.1080/10225706.2017.1351380
Keywords: microcore, compression wood, tree rings, wood anatomy, xylogenesis, subtropical China
Citation: Wang C, Zheng Z, Zhou F, Liu X, Fonti P, Gao J and Fang K (2023) Intra-annual dynamic of opposite and compression wood formation of Pinus massoniana Lamb. in humid subtropical China. Front. For. Glob. Change 6:1224838. doi: 10.3389/ffgc.2023.1224838
Received: 18 May 2023; Accepted: 25 September 2023;
Published: 18 October 2023.
Edited by:
Ling Zhang, Jiangxi Agricultural University, ChinaReviewed by:
Junzhou Zhang, Lanzhou University, ChinaCopyright © 2023 Wang, Zheng, Zhou, Liu, Fonti, Gao and Fang. This is an open-access article distributed under the terms of the Creative Commons Attribution License (CC BY). The use, distribution or reproduction in other forums is permitted, provided the original author(s) and the copyright owner(s) are credited and that the original publication in this journal is cited, in accordance with accepted academic practice. No use, distribution or reproduction is permitted which does not comply with these terms.
*Correspondence: Keyan Fang, a2ZhbmdAZmpudS5lZHUuY24=
†These authors have contributed equally to this work
Disclaimer: All claims expressed in this article are solely those of the authors and do not necessarily represent those of their affiliated organizations, or those of the publisher, the editors and the reviewers. Any product that may be evaluated in this article or claim that may be made by its manufacturer is not guaranteed or endorsed by the publisher.
Research integrity at Frontiers
Learn more about the work of our research integrity team to safeguard the quality of each article we publish.