- 1School of the Environment, Washington State University, Pullman, WA, United States
- 2College of Natural Resources, Franklin H. Pitkin Forest Nursery, University of Idaho, Moscow, ID, United States
Introduction: Increasing temperatures associated with climate change can lead to more challenging conditions for tree seedlings, including greater soil surface temperatures and reduced growing season soil moisture. Downed woody debris (DWD) may provide microsite effects, such as shade, that moderate these conditions for seedlings. However, few studies have conducted fine-scale assessments of the strength of the microsite as a function of distance from DWD or how the microsite effect differs between species or across topographic gradients.
Methods: In this study, conducted in the Palouse region of eastern Washington State, we placed three large pieces of DWD (5 m length × 40–50 cm small-end diameter, oriented east–west) on each of three topographic facets (north-facing, flat ridge-top, and south-facing), and planted transects of seedlings of a xerophytic conifer (ponderosa pine, Pinus ponderosa) and a mesophytic conifer (Douglas-fir, Pseudotsuga menziesii) on both sides of the DWD at fixed distances (0, 0.25, 0.5, and 1.5 m). The 1.5 m distance was assumed to serve as a control, with no measurable influence from DWD. Seedling responses (stress rating, survival, basal diameter and height growth, and dark-adapted chlorophyll fluorescence) over two growing seasons were used to interpret the influence of DWD on seedling health and survival, especially during stressful episodes of extreme heat, such as occurred during July 2021, the first growing season of the experiment. Soil surface temperature and soil volumetric water content (10 cm depth) were measured at all seedling locations to understand biophysical contributors to seedling response.
Results: We found that seedlings of both conifers displayed lower stress ratings, higher survival, and greater height growth close to the north side of DWD, with this effect especially pronounced on the flat ridge-top and the south-facing slope. Soil surface temperature decreased greatly in the “shade zone” at 0.0 m and 0.25 m distances on the north side of DWD, and soil volumetric water content declined more quickly outside of the shaded microsite.
Discussion: These findings suggest that creating or retaining DWD on stressful sites may prove an important climate adaptive management strategy in ecosystem restoration or forest management, especially if extreme heat events continue to increase in frequency.
1. Introduction
Achieving successful regeneration of trees is a main objective of forest management and many habitat restoration projects and is essential to maintaining forest cover at stand to landscape scales. Tree populations in many environments are controlled by the “demographic bottleneck” of the seedling stage, where browsing, climate extremes, repeat disturbances, and other stressors may kill substantial proportions of established seedlings (Urza et al., 2019).
This demographic bottleneck is becoming more prominent and problematic due to climate change and its associated disturbances, especially in dryland forests (Petrie et al., 2017). Many forest sites in the Pacific Northwest and other forests with pronounced dry seasons are on the cusp of conversion to non-forest cover types, especially when affected by high severity, large patch disturbances (Stevens-Rumann et al., 2018; Meigs et al., 2022). Additionally, annual moisture deficits are increasing, which will contribute to decreased seedling survival (Tepley et al., 2017; Stevens-Rumann et al., 2018), even at high latitudes (Boucher et al., 2020). Kemp et al. (2019) predict increasing failure of conifer regeneration in the northern Rockies due to increasing summer temperatures, especially during the critical post-disturbance stage when seedling establishment and survival is critical for determining future stand composition. Additional challenges to seedling survival and growth include the increasing frequency of extreme temperature events (Horton et al., 2016; Breshears et al., 2021), such as the 2021 “heat dome” event affecting the Pacific Northwest (Still et al., 2023; White et al., 2023).
Compositional shift in forest ecosystems often occurs during the stand establishment period or early seral period (Oliver, 1980; Kemp et al., 2019; Seidl and Turner, 2022). Therefore, it is crucial to understand factors operating in the early seral pre-forest stage that control the relative success of different plant species, especially trees, in establishment and growth.
Although tree regeneration is controlled at broad scales by top-down factors such as temperature and precipitation patterns, local-scale factors may influence survival through early stages of tree seedling and sapling development (Kuijper et al., 2010), and thus present feedbacks to forest dynamics at larger spatial scales. Down woody debris (DWD) is a common structural component of early seral pre-forest environments (Harmon et al., 1986; Swanson et al., 2011), as it is a biological legacy from the pre-disturbance stand (Franklin et al., 2000). It can provide a number of different functions that support higher seedling survival and growth in forest and woodland environments. In the closed forest environment, DWD can act as an elevated platform that enhances seedling regeneration (Harmon and Franklin, 1989; Takahashi et al., 2000; Heinemann and Kitzberger, 2006; Motta et al., 2006; Bolton and D’Amato, 2011). In arid or xeric environments, DWD can elevate soil moisture, provide shade, or otherwise enhance microsites in favor of successful tree regeneration (Goldin and Hutchinson, 2014, 2015). The importance of DWD-provided microsites may vary depending on factors influential at larger spatial scales, such as the interaction of topographic aspect and slope (Reely and Nelson, 2021). For example, the relative importance of a shaded, cool microsite for seedling survival may be lower on poleward slopes (e.g., north-facing slopes in northern hemisphere), but accentuated on topographic aspects with higher heat load (e.g., south- or southwest-facing slopes in the northern hemisphere).
To enhance survival of planted stock, foresters have long advocated planting in beneficial microsites, such as in the shade of logs, stumps, and snags (Mitchell et al., 1990; Fitzgerald, 2008). In many ecosystems, this practice is still anecdotal. Early literature on planting frequently ignored the importance of microsites provided by DWD (e.g., Goor and Barney, 1968), likely because much of this literature focused on afforestation on intensively managed sites where DWD would be rare. However, a number of studies have examined the role of DWD and other physical structures in creating beneficial microsites for tree regeneration. Flint and Childs (1987) found a positive effect of shade cards on growth of conifer seedlings, although the greatest effects on growth were due to control of competing vegetation and mulching around seedling locations. Seedlings of temperate coniferous tree species in experimental gaps in the Pacific Northwest were facilitated by protective shading from woody debris, showing that structural microsites may be important even in high rainfall areas and with some degree of shade from adjacent closed-canopy forest (Gray and Spies, 1997). Heinemann and Kitzberger (2006) examined Nothofagus seedling survival in Patagonian forests, assessing DWD as a substrate that can retain water through the relatively dry growing season. Bailey et al. (2012), working in post-fire forests of the Tasmanian Midlands, found that close proximity to shading woody debris or rock increased the probability of seedling presence. Marsh et al. (2022) found it difficult to differentiate between shrub-created and woody debris-created microclimate but noted the limited extent of woody debris in the system they examined. Marcolin et al. (2019) contrasted a number of post-disturbance strategies, concluding that retention or recruitment of DWD (by falling standing snags) increased the availability of microsites favorable for seedling establishment and survival. Most recently, Marangon et al. (2022) found that windthrown trees in a site in the southern Italian Alps were important sheltering elements from both climate stressors and browsing ungulates. They employed planted seedlings both in proximity to woody debris and in control sites without woody debris. However, the woody debris was generated by natural disturbance, and orientation and location of the woody debris were determined by the action of disturbance, and not placed in an experimental setting. Similarly, Marzano et al. (2013) found that seedlings were more likely to be found in proximity to woody debris, and that the relationship was anisotropic, with seedlings more likely to be found on the shady side of sheltering elements.
Despite work that has examined DWD as a factor in forest regeneration processes, few studies have quantitatively assessed the microsite-creation role of DWD with a design involving deliberate planting of trees at a range of specified distances from sheltering structures such as DWD. In this experiment, we evaluate the influence of deliberately placed and oriented DWD on soil volumetric water content (VWC) and soil surface temperature (SST) and on the survival and growth responses of two regionally important conifers, ponderosa pine (Pinus ponderosa Douglas ex C. Lawson) and Douglas-fir (Pseudotsuga menziesii (Mirb.) Franco var. glauca (Mayr) Franco), nested within three topographic settings. Ponderosa pine (hereafter PP) is a xerophytic, heat-tolerant conifer that is characteristic of ecotonal sites between forest and arid non-forest communities, while Douglas-fir (hereafter DF) is a relatively mesophytic conifer that is most common in mid-elevation sites in interior western North America (Franklin and Dyrness, 1988). Both are broadly geographically distributed in western North America (Burns and Honkala, 1990) and are important both ecologically and to the regional timber economy. There is concern that tree species like these that comprise ecotonal woodlands or forests in the inland Northwest may be unable to maintain adequate regeneration in the face of climate change and altered disturbance regimes (Meigs et al., 2022). Using planting of seedlings at specified distances from placed DWD, we seek to quantify the spatial domain of protective microsite effects, and furthermore assess species-specific responses to these microsite effects. With this study, we intended to test the following hypotheses:
1. Seedlings closer to protective DWD will display greater survival and growth.
2. Seedlings on north side of DWD will display greater survival and growth.
3. Seedlings on a northern topographic aspect will display greater survival and growth.
4. The mesophytic DF will benefit more from DWD microsite protection than the xerophytic PP.
2. Materials and methods
2.1. Site description
The study site was located on the 18-ha E.H. Steffen Center in Whitman County, WA, USA (46.7083 N, −117.1263 W), which is managed by Washington State University for wildlife and forestry conservation, research, and demonstration. Mean elevation at the Steffen Center is 795 m.a.s.l (meters above sea level) and mean annual precipitation is 517 mm, with the majority falling as winter snow. The climate is a marine modified continental climate with high seasonal temperature variation—July mean high and low temperatures are 28°C and 10°C, respectively; January mean high and low temperatures are 2°C and −5°C, respectively (U.S. Climate Data, 2023). Soils are loess-derived Palouse-Thatuna silt loams, consistent with much of the greater Palouse Prairie ecoregion, and overlie flood basalts of the Columbia River Basalt Group (Donaldson, 1980). Topography is defined by moderate north and south facing slopes, 10 degrees in maximum slope, and a flat ridge (~10 m in width, oriented east–west) through the middle of the site. The planting location had no overstory trees within 10 m (nearest trees, ~10 m in height, were north and west of the planting site and provided no shade to the site). The site had little natural tree regeneration and a very uniform grass/forb stratum dominated by smooth brome (Bromus inermis) and sheep fescue (Festuca ovina). Surrounding areas are dominated by uneven-aged PP forest and woodland, with areas of chiefly non-native grasses and other herbaceous vegetation.
2.2. Experimental design
In May 2021, a John Deere 5,310 tractor was used to translocate nine hand-felled ponderosa pine DWD segments (logs) to the site of the experiment. DWD segments were sourced from ponderosa pine stands belonging to the Steffen Center and were each cut 5 meters in length (a typical log length for timber harvest in the region), had a minimum diameter of 40 cm at the small end of the section, and had been in place for a year prior to planting seedling stock. DWD segments were oriented east–west (90°–270° true compass azimuth) and placed in a 3 × 3 grid; three logs each on the southern, ridge, and northern facing aspects (Figure 1), at positions 5 m apart in three columns: west, center, and east. Planting stock consisted of one-year-old PP and DF seedlings grown in 315B Styroblock containers (root volume 90 mL, top diameter 30 mm, depth 151 mm, with 160 seedlings per Styroblock). Seedlings were planted on the north and south sides of each DWD segment, in three transects- east (1.0 meters from east end of DWD), central (center of the DWD, 2.5 meters) and west (1.0 meters from west end of DWD)- and at four distances- 0 meters (directly on drip-line of the DWD), 0.25 m, 0.5 m, and 1.5 m (Figure 1). No efforts were made to control competing vegetation. Distance is analyzed as a continuous independent variable in all models, instead of as a factor or categorical variable. The 1.5 m distance on each transect is intended to serve as an experimental control, as it is sufficiently distant from the DWD to not experience microclimatic or soil-related influences. A total of 24 seedlings of each species surrounded each DWD segment, 216 seedlings total per species (432 total seedlings for the study). The overall experimental design is a split-split plot design, with topographic aspect and DWD aspect as split factors.
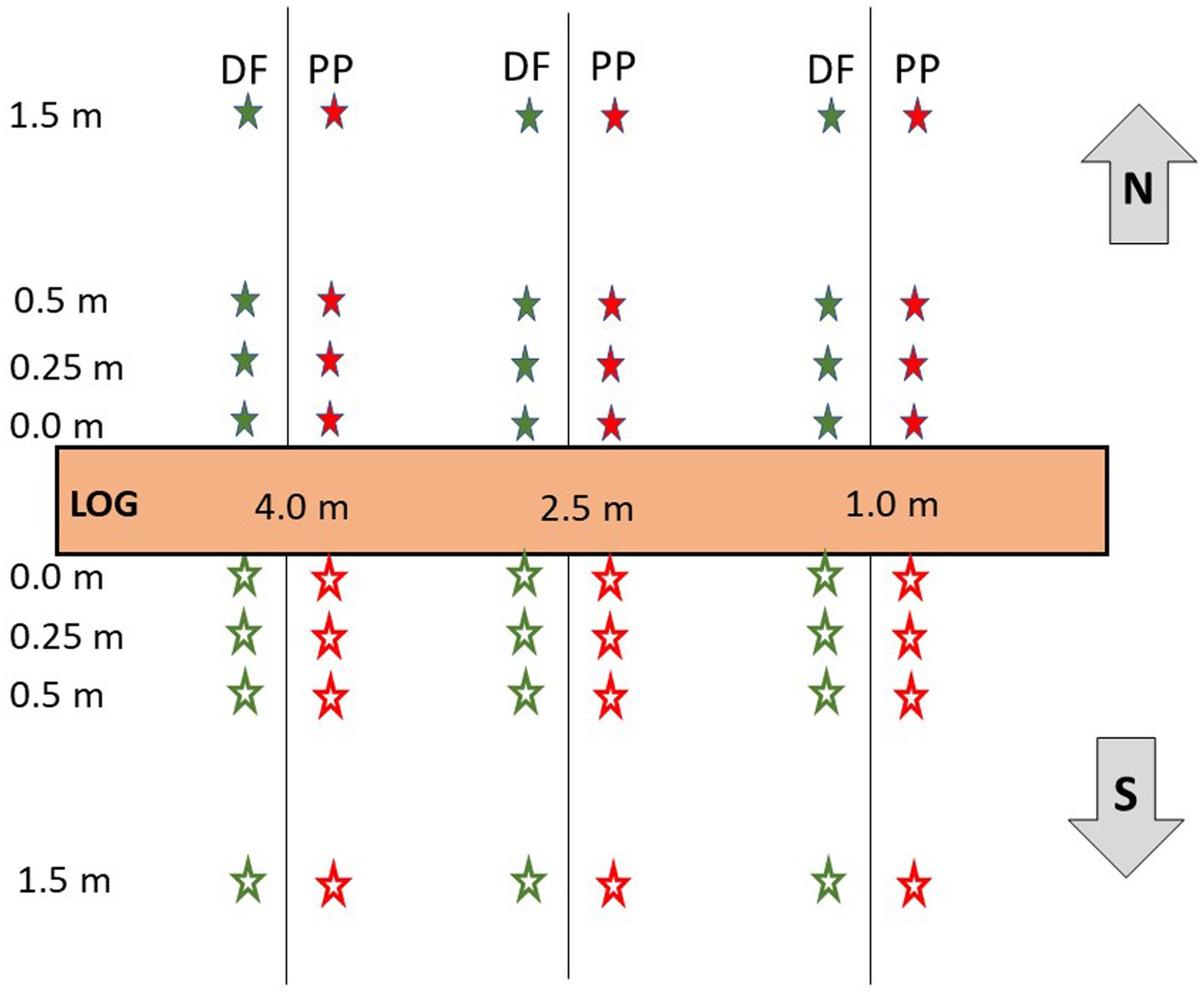
Figure 1. Study layout, with example of transects placed at each DWD showing placement of Douglas-fir (DF) and ponderosa pine (PP) seedlings planted at fixed distances along transects on north and south sides of the DWD, which are placed on three topographic positions: north-facing slope, flat ridgeline, or south-facing slope.
2.3. Plant specific measurements
After planting in May 2021, we took baseline measurements of stress rating, height, and basal diameter for all 432 seedlings. We measured seedling height (cm) from soil-stem interface to shoot apical meristem, and basal diameter (mm) 3 cm above ground level to avoid soil and root bulges. Initial seedling height and basal diameter were used in calculating seedling growth over the first and second growing seasons, by subtracting the initial height and basal diameter of each seedling upon planting from the height and basal diameter at the end of the 2021 and 2022 growing seasons. We classified stress rating according to an ordinal scale where stress rating 1 = healthy seedling, little to no damage or red needles; stress rating 2 = slightly unhealthy, evidence of needle damage but a majority of green needles; stress rating 3 = further evidence of damage, seedling alive but nearing death; stress rating 4 = dead seedling (Figure 2). Seedling measurements were repeated monthly over the first growing season (May–September 2021) and twice during the second growing season (July and September 2022). Dead seedlings were visually assessed to identify a likely cause of mortality. Mortality due to girdling by voles (Microtus spp.) was assigned to those dead seedlings that had 50% or greater girdling of the main stem as a binary variable (0 = alive or dead, but not killed by vole, and 1 = killed by vole herbivory).
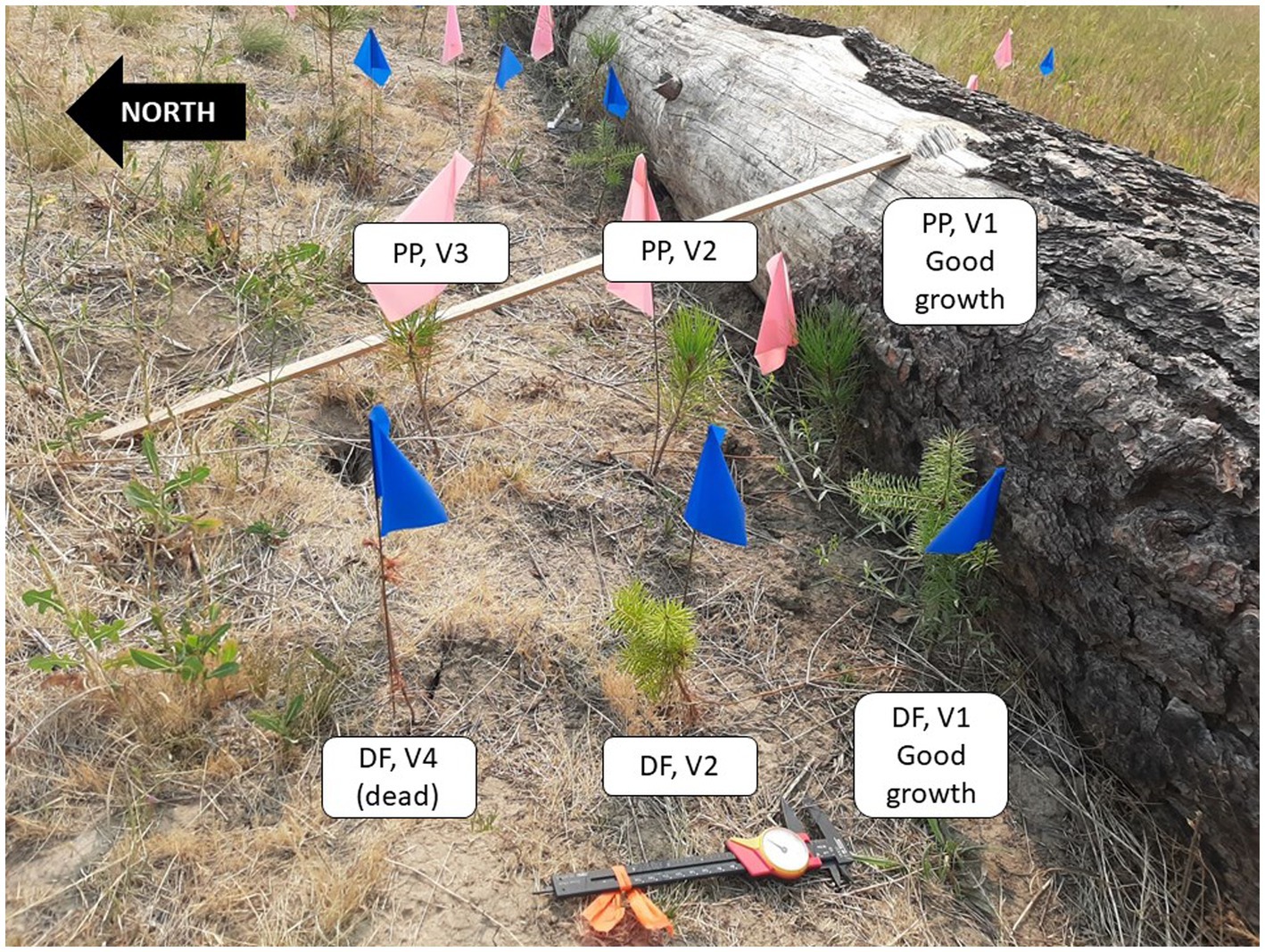
Figure 2. Photo showing typical results on north side of DWD (in this case, on flat ridgetop) for ponderosa pine (PP) and Douglas-fir (DF) seedlings. Codes indicate stress rating: V1 = stress rating 1, V2 = stress rating 2, V3 = stress rating 3, V4 = stress rating 4.
We also measured dark-adapted chlorophyll fluorescence of seedlings twice during the first growing season (June and September 2021). Specifically, we measured Fv/Fm, the ratio of maximum to minimum fluorescence, which quantifies the maximum quantum efficiency of Photosystem II (Kitajima and Butler, 1975), using an OS-30p + chlorophyll fluorometer (Opti-Sciences, Hudson, NH). Measurements were taken at least one hour after sunset to ensure dark adaptation of each seedling and avoid influence of actinic light on Fv/Fm values. We applied a one-second, saturating light pulse to an arbitrarily selected cluster of fascicles on each seedling to excite photosystem II. The corresponding Fv/Fm value represented overall photosynthetic capacity of the seedling. Fv/Fm values between 0.790 and 0.840 indicated relatively high photosynthetic capacity (Cavender-Bares and Bazzaz, 2004), and values below 0.250 can indicate dead or dying plants, including conifers (Guadagno et al., 2017). While all these plant measurements were taken repeatedly throughout the growing seasons, analyses were conducted only on the final measures of each growing season as these were most informative to capture seedling health and survival and to calculate growth.
2.4. Abiotic site measurements
We measured soil volumetric water content (VWC) and soil surface temperature (SST) during the first growing season (May 12, 2021 and September 15, 2021 respectively). To avoid shading from seedlings, we measured these variables at a point 5 cm west of each seedling. We recorded VWC to the nearest 0.1% with a HydroSense hand-held soil moisture sensor (Campbell Scientific, Logan, UT) and SST to the nearest 0.1°C with a hand-held infrared (IR) thermometer 1,080 (Etekcity, Vesync Company, Anaheim, CA). To capture the daily temperature maximum, we measured SST between 14:00 and 16:00 PST.
During the first growing season of the experiment, extreme heat conditions occurred, with near-surface air temperatures approximately 12–14°C above normal in late June 2021 in the area of the experiment [extrapolated from map data presented in White et al. (2023)]. Figure 3 presents local weather station data for monthly mean temperatures, including separate traces for high temperature averages, for 1990–2020 and for 2021 and 2022 individually.1
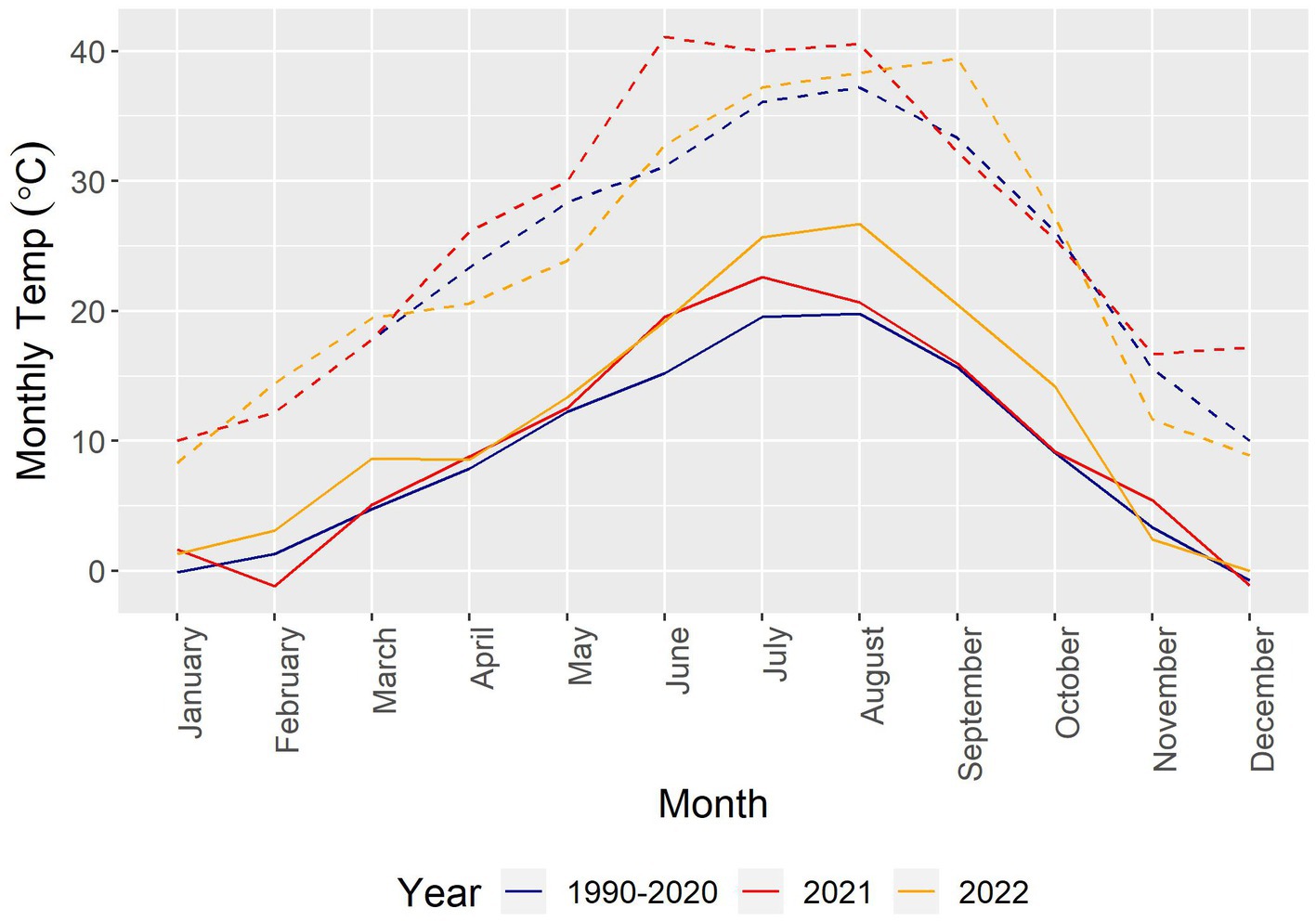
Figure 3. Comparison of monthly temperature patterns over the periods 1990–2020, 2021, and 2022. Temperature data taken from the National Weather Service with source Pullman 2 NW, co-op station 456,789. Solid lines represent monthly averages, and dashed lines represent maximum values.
2.5. Data analysis
All data analyses were performed in the R statistical programming environment, version 4.2.2 (R Core Team, 2022). We used linear mixed-effect models (Neter et al., 1996) (R-package ‘lme4’) with a split-plot design (Quinn and Keough, 2002) to evaluate the response of SST and VWC to topographic aspect, DWD aspect, and distance from DWD. DWD aspect was considered a split-plot factor, with three replicate transects within each side of the log. Fixed effects variables included distance from DWD, DWD aspect (north vs. south side of a log), topographic aspect (south-facing, flat ridge-top, and north-facing), and an interaction between distance and DWD aspect, with response variables SST and VWC. We did not incorporate ‘year’ (2021 vs. 2022) as a variable in the models, but modeled each year separately (Table 1).
The influence of topographic aspect, DWD aspect, distance from DWD, and seedling species (PP vs. DF) on seedling height growth, basal diameter growth, and photosystem II fluorescence were assessed using linear mixed-effects models (lmer) with seedling species, distance from DWD, DWD aspect, topographic aspect, and an interaction term between distance from DWD and DWD aspect included as predictor variables. We ran two lmer models each for height growth that occurred during the first and second growing seasons, and also for basal diameter growth that occurred during the first and second growing seasons. Seedlings that were dead at the end of each growing season were removed from the height and basal growth analyses (n = 209 for 2021 growing season; n = 159 for the 2022 growing season). Additionally, we fit two linear mixed-effects models with log-transformed fluorescence values taken mid-season and end of season. Seedlings dead at the time of measurement were removed from the fluorescence analysis as they have a fluorescence value of zero that would skew the model (n = 293 for mid-season 2021; n = 215 for end of season 2021).
To assess the influence of microsite on the stress rating of the PP and DF, we applied a cumulative link mixed model (CLMM) with the R package ‘Ordinal’ (Christensen, 2022). The cumulative link mixed model is a special case of the generalized linear mixed model that accepts an ordinal response variable. In this case the response variable was stress rating (1–4), converted to an ordered factor for use with the ‘Ordinal’ package. Predictor variables included species (DF or PP), DWD aspect (north or south side of a piece of DWD), distance from DWD (0, 0.25, 0.5, 1.5) and topographic aspect (north, ridge, south), and the interaction between DWD aspect and distance from DWD. Overall survival and vole mortality were analyzed with a generalized linear mixed model (R package ‘lme4’) specifying a binomial distribution and logit link. Seedlings that died because of damage from voles were removed from the dataset prior to survival and stress rating analysis in order to isolate mortalities caused by physical conditions (n = 414).
In all linear mixed-effects models and generalized linear mixed models, we estimated a random effect for ‘location’ (east, center, and west blocks, or N-S columns within our 3×3 experimental layout) to explore variance among these positions within the study layouts as a block effect. This random effect was removed if inclusion led to singular model fits or the variance was estimated as zero, as occurred in several models. Significance of random effects were evaluated with the package ‘lmerTest’ (Kuznetsova et al., 2017). Due to the limitations of the ‘clmm’ function in the Ordinal package, we could not replicate the same random effects structure as in the other models. For this model, individual DWD and location were implemented as separate random effects.
We attempted to include a random effect for transects within one DWD aspect (north side or south side of an individual piece of DWD) to explore possible autocorrelation of observations within transects. This random effect, however, could not be incorporated, as inclusion of this effect led to singular model fits.
In all models, interactions between all predictor variables were tested but were removed if they were not statistically significant. Coefficients, standard errors, and value of ps were reported for all main effects, whether significant at the α = 0.05 level or not (Table 1).
3. Results
3.1. Stress rating
At the end of the first growing season, 209 of 432 seedlings were alive (48.4% overall survival), 58 DF (26.9% survival of that species) and 151 PP (70% survival of that species). Of the 209 surviving seedlings, 61 were stress rating 1, 116 were stress rating 2, and 32 were stress rating 3. Stress rating 1 seedlings of both species were primarily located at the 0.0 and 0.25 m distances (Figure 2 illustrates typical results), with stress rating 1 DF occurring mostly on the north side at these locations, and stress rating 1 PP on both DWD aspects. DF seedlings had largely separated into stress rating 1 and stress rating 4 (dead), with few individuals of stress rating 2 and stress rating 3. PP seedlings were more evenly distributed across the four stress ratings, exhibiting lower overall mortality at all locations (Figure 4).
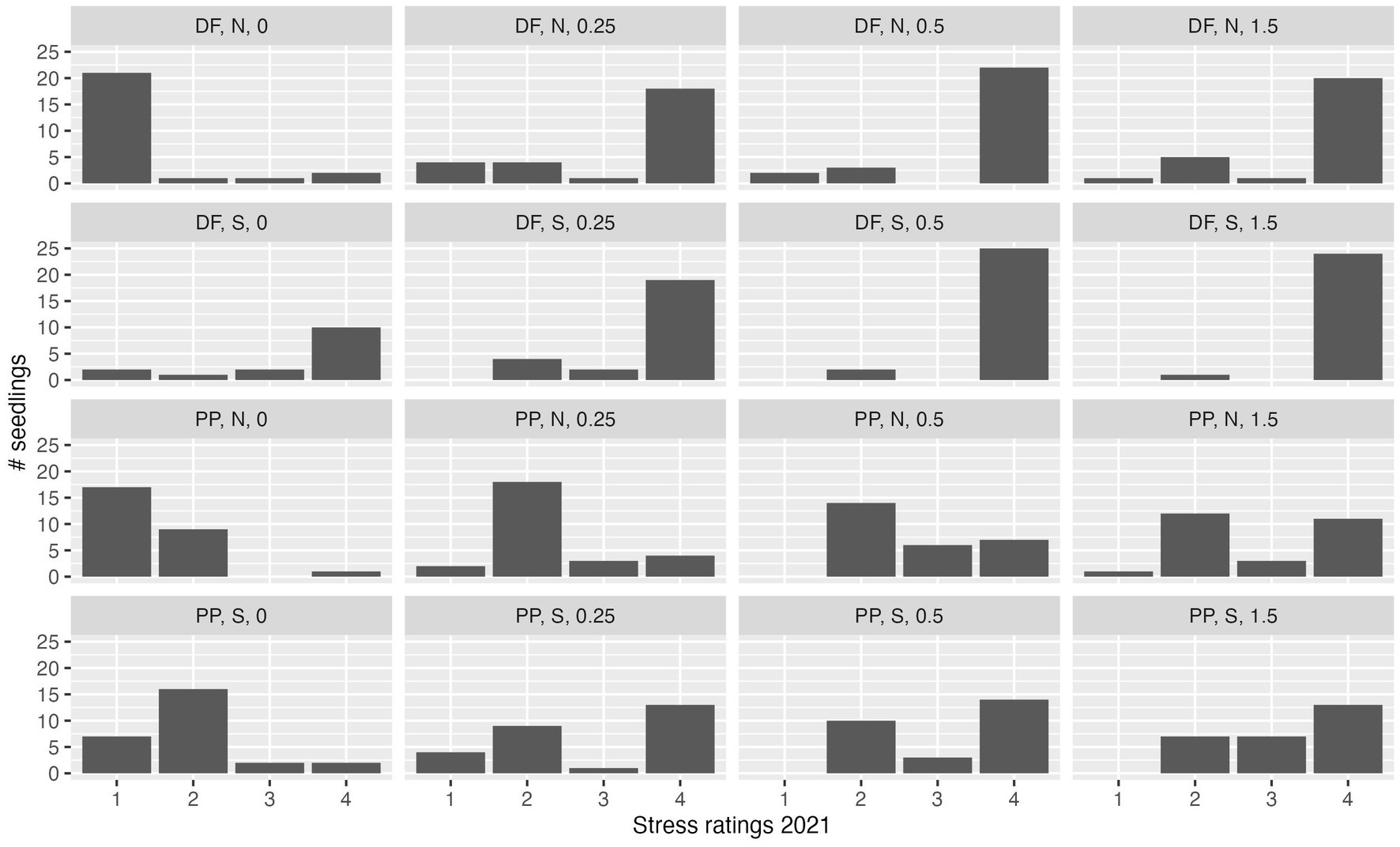
Figure 4. Stress rating distribution at the end of the first growing season. Data are shown for Douglas-fir (DF) and ponderosa pine (PP) seedlings planted at 0, 0.25, 0.5, and 1.5 m distance from DWD on the north (N) or south (S) sides of the DWD. Data are pooled among DWD on north-facing, ridgeline, or south-facing positions (i.e., topographic aspect not represented in this graph).
The mixed-effects model results indicate that species, DWD aspect, distance to DWD, and topographic aspect were significant predictors of stress rating for both growing seasons (Table 2). Independent of location, PP had overall lower stress ratings compared to DF (p < 0.0001). Stress rating values were greater on the south side of the DWD compared to the north side (p < 0.0001). Stress ratings increased with increasing distance from the log (p < 0.0001). Across the three slope aspects (south-facing, flat ridgetop, and north-facing), stress ratings were lowest on the north slope, with significant increases in stress rating associated with seedlings on the ridgetop (p = 0.0005) but not the south aspect (p = 0.1039). The interaction between DWD aspect and distance from DWD was not significant.
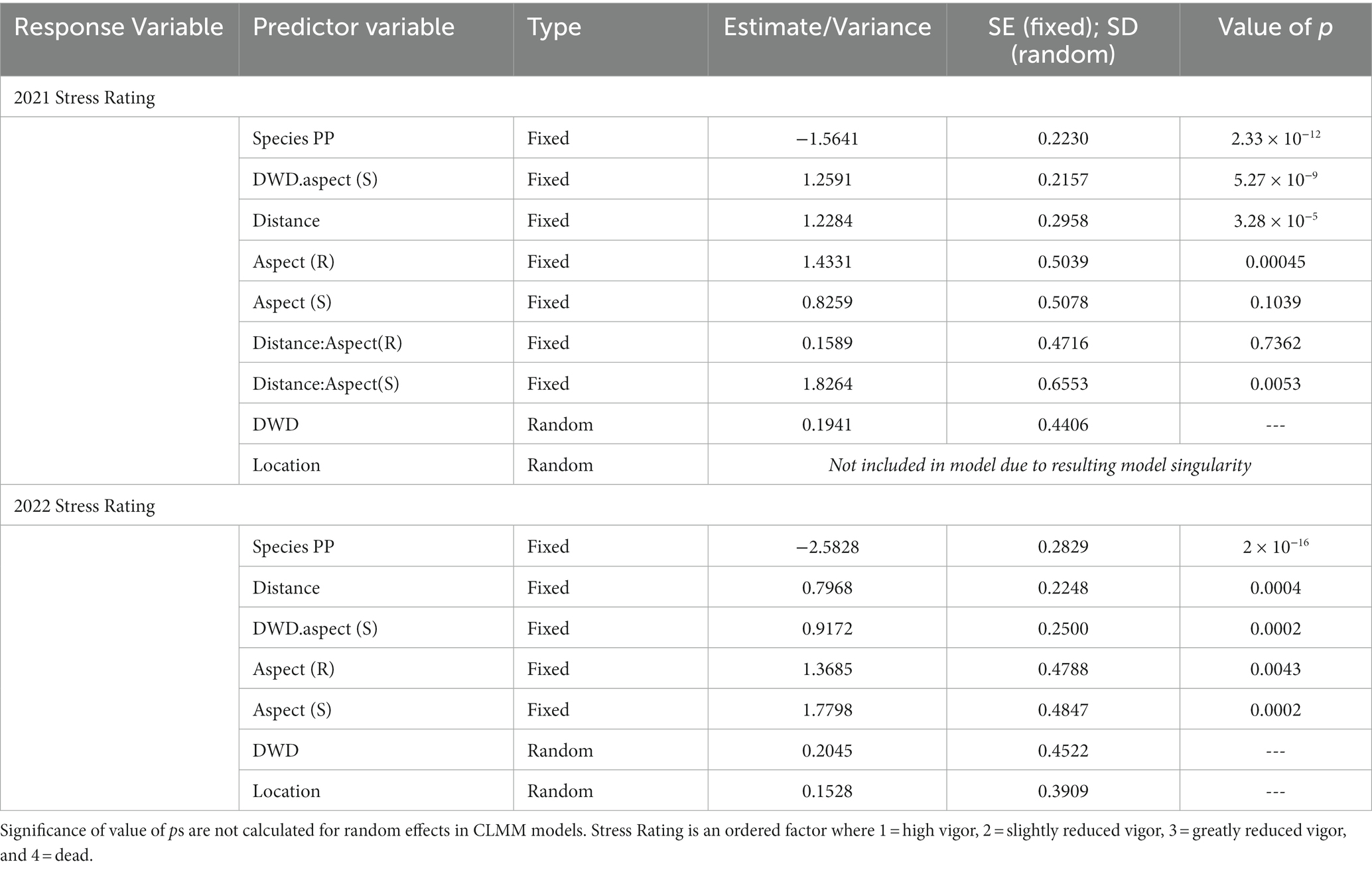
Table 2. Cumulative link mixed model output with end of growing season stress rating in 2021 and end of growing season stress rating 2022 as dependent variables.
At the end of the second growing season, only 159 of 432 seedlings remained alive (37% overall survival), 30 DF (13.8% survival of that species) and 129 PP (59.7% survival of that species). The distribution of stress ratings shifted from the end of the first growing season, with 135 of the remaining seedlings rated as stress rating 1, 22 rated as stress rating 2, and only two seedlings rated as stress rating 3 (Figure 5). PP seedlings experienced lowest stress ratings at the end of two growing seasons on the north side of DWD at the 0.0 and 0.25 m distances. PP seedlings experienced moderate success at other distances, having separated into low stress and dead individuals, with few individuals of stress ratings 2 and 3. Few DF seedlings with stress rating 1 remained in locations other than the 0.0 m distance on the north side of DWD.
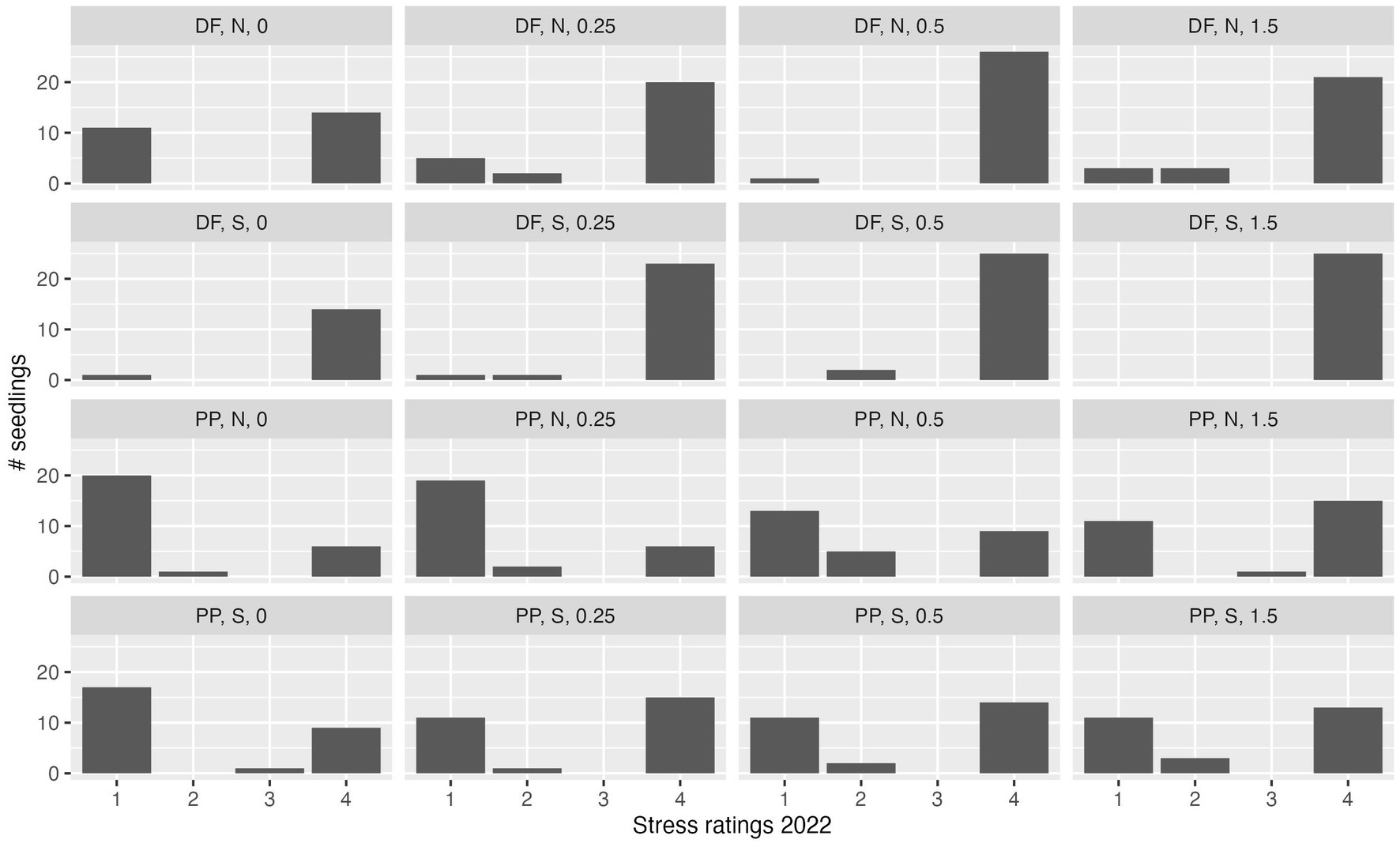
Figure 5. Stress rating distribution at the end of the second growing season. Data are shown for Douglas-fir (DF) and ponderosa pine (PP) seedlings planted at 0, 0.25, 0.5, and 1.5 m distance from DWD on the north (N) or south (S) sides of the DWD. Data are pooled among DWD on north-facing, ridgeline, or south-facing positions (i.e., topographic aspect not represented in this graph).
Independent of location, PP had overall lower stress ratings compared to DF (p < 0.0001). Stress ratings were greater on the south side of the woody debris compared to the north side (p = 0.0002) and increased with increasing distance from the DWD (p = 0.0004). Stress ratings were lowest on the north slope and increased significantly on the ridge (p = 0.0043) and south facing slopes (p = 0.0002). Interaction between DWD aspect and distance from DWD was not significant (Table 2). The estimates of variance associated with random effect were small compared to coefficients for fixed effects, suggesting that variation in seedling vigor response among logs was low.
3.2. Survival
DF experienced greatest survival on the north side of DWD, and at the 0.0 and 0.25 m distances (Figure 6). Both PP and DF experienced a greater range of outcomes on the south-facing slope, with the greatest distinction between the protected shade zone of the immediate north side of the DWD and relatively unprotected positions. DF fared poorly on the flat ridge-top but experienced its highest survival rates at the ridge-top location at the 0.0 m distance on the north side of the DWD (Figure 6).
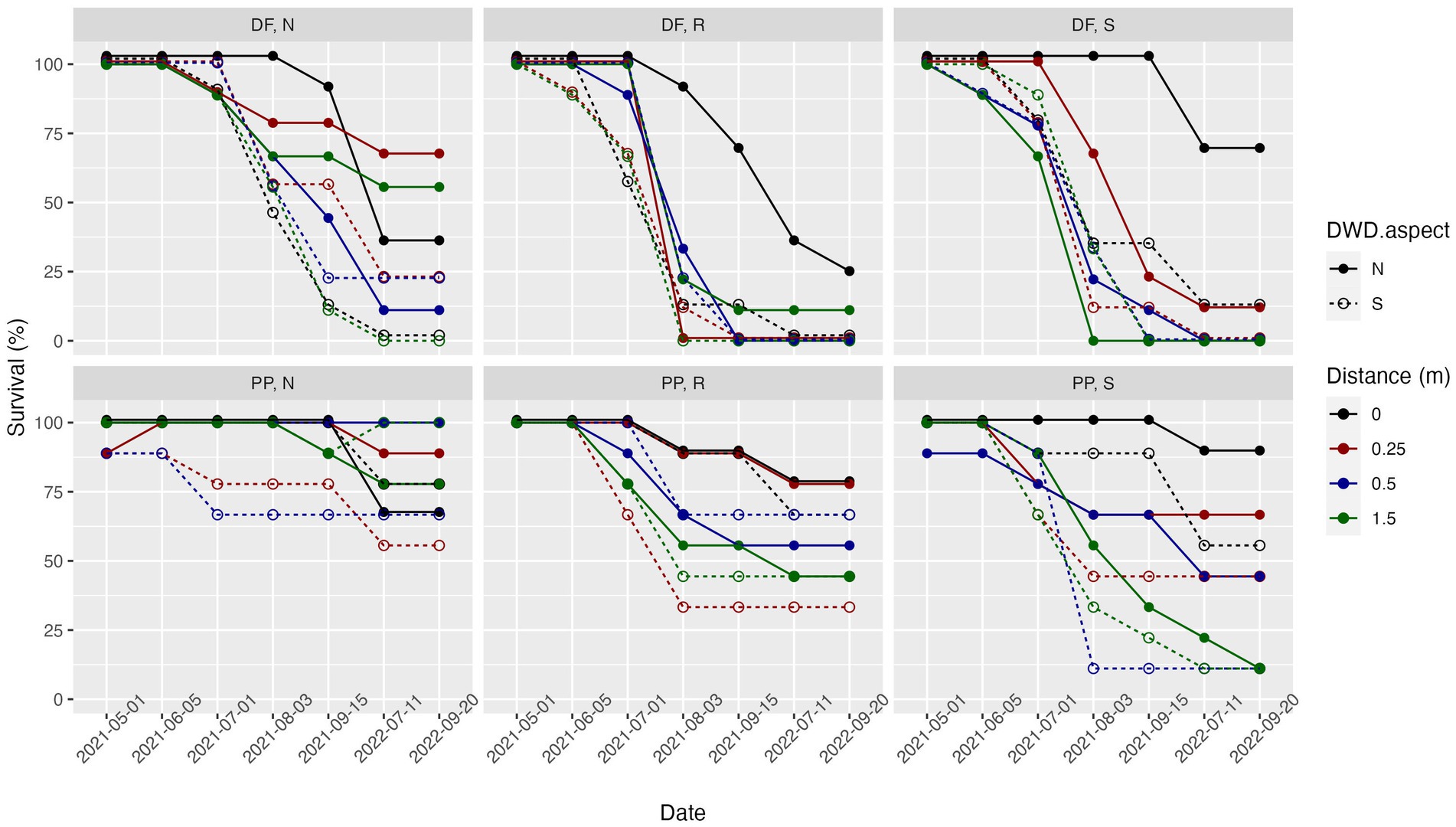
Figure 6. Percent survival over two growing seasons, with individual traces representing combinations of side of DWD (north vs. south) and distance from the DWD. Dashed lines with open points represent the south DWD aspect while solid lines with colored in points represent the north DWD aspect. Each color is representative of a distance: black = 0.0 m, red = 0.25 m, blue = 0.5 m, green = 1.5 m. Each panel represents a combination of species (DF, PP) and topographic setting (north-facing slope, flat ridge-top, south-facing slope).
Survival at the end of both growing seasons was clearly conditioned by species, DWD aspect, distance from DWD, and topographic position (Table 3). At the end of the first growing season, survival was higher for PP compared to DF (p < 0.0001). Overall survival was lower on the south DWD aspect (p < 0.0001) and decreased as distance from DWD increased (p = 0.0338). In addition, the interaction term between distance from DWD and location on the south aspect was significant (p = 0.0044). Survival was significantly lower on the south topographic aspect (p = 0.0485) and the ridge (p = 0.0018) compared to the north topographic aspect. Interaction between DWD aspect and distance from DWD was not significant.
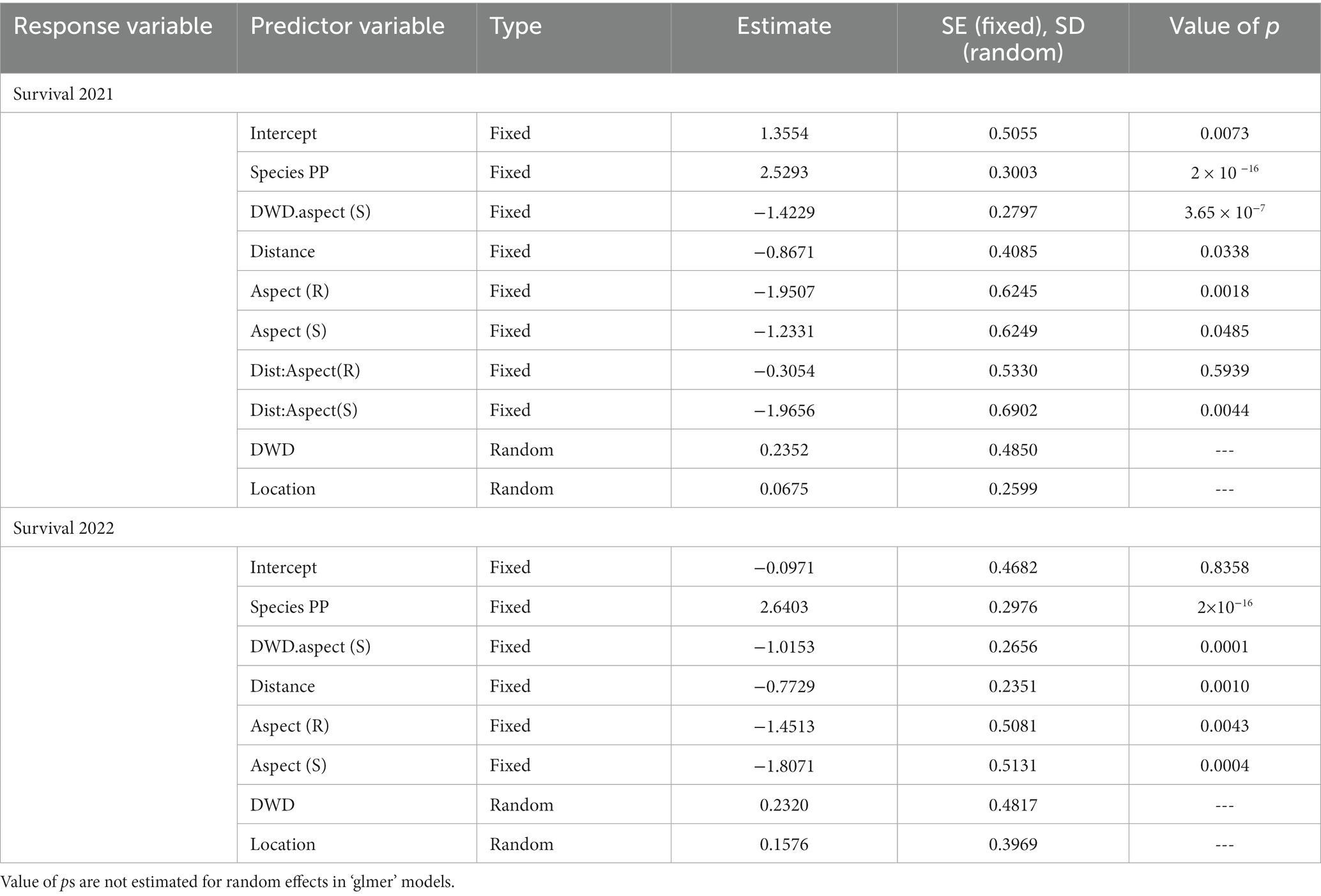
Table 3. Generalized linear mixed model (binomial dependent variable) output for models with 2021 survival and 2022 survival as dependent variables (0 = dead, 1 = alive).
Survival was greater for PP than for DF (p < 0.0001) during the second growing season (Table 3). For both species, survival was lower on the south DWD aspect compared to the north DWD aspect (p = 0.0001) and survival decreased as distance from DWD increased (p = 0.0010). Survival was significantly lower on the ridge (p = 0.0043) and south topographic aspects (p = 0.0004) compared to the north topographic aspect. Interaction between DWD aspect and distance from DWD was not significant. Random effects associated with individual DWD, DWD aspect within individual DWD, and location were small compared to coefficients for fixed effects, suggesting that variability of response among replicate logs was not considerable.
3.3. Basal diameter growth
At the end of the first growing season, basal diameter exhibited no significant changes with seedling species, distance from DWD, DWD aspect, topographic aspect, or the interaction between DWD aspect and distance from DWD (Table 4). By the end of the second growing season, PP exhibited significantly higher basal diameter growth compared to DF (p < 0.0001), and basal diameter growth decreased with distance from DWD (p = 0.0153) There is marginal evidence (p = 0.0927) for lower basal diameter growth on the south topographic aspect compared to the north. Otherwise, basal diameter growth was not significantly different as a function of DWD aspect or the interaction between DWD aspect and distance from DWD (Table 4). Random effects were not significant, suggesting that basal diameter growth did not vary as a function of location within the experiment.
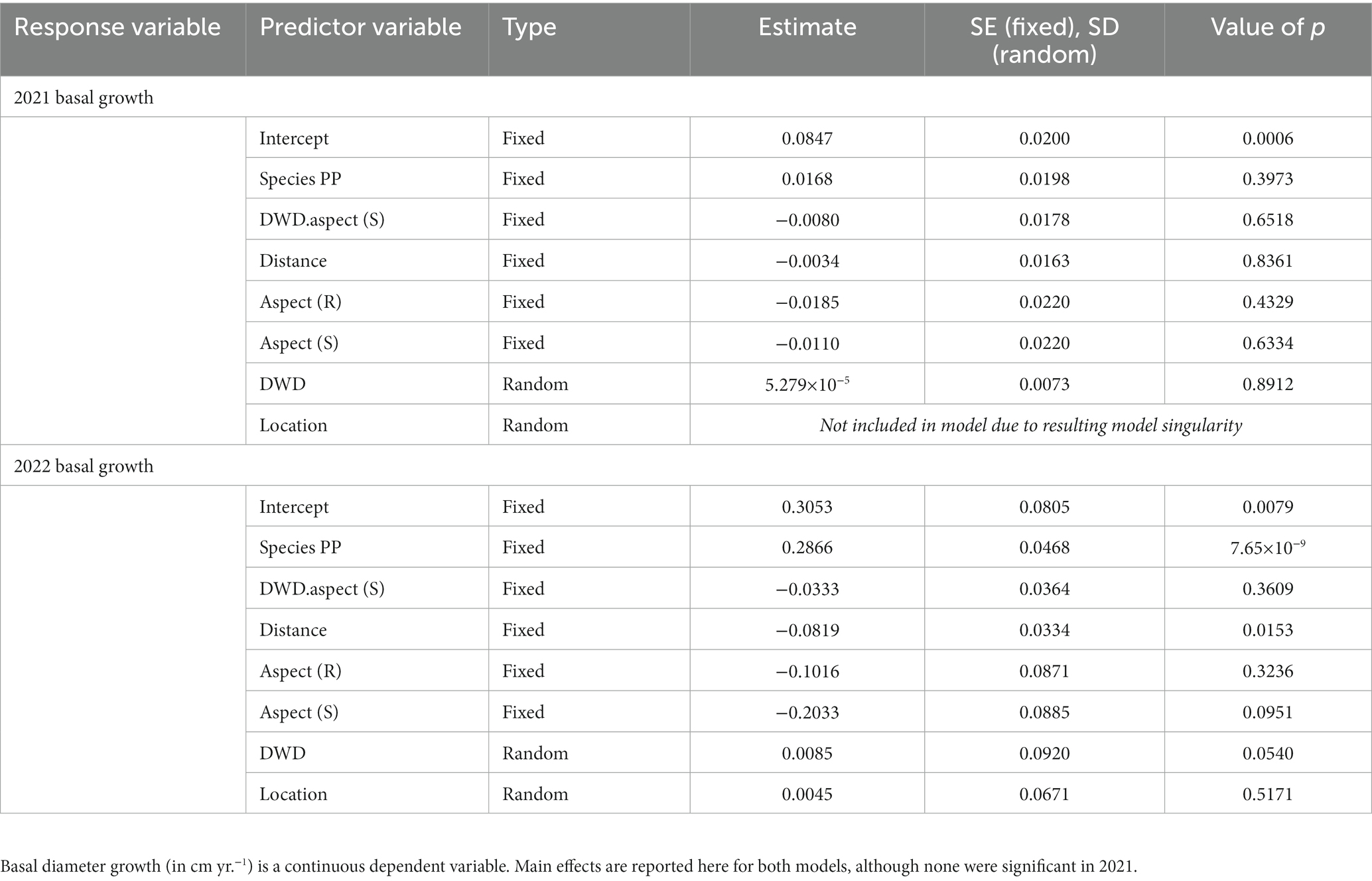
Table 4. Linear mixed model output for models with basal diameter growth 2021 and basal diameter growth 2022 as dependent variables.
3.4. Height growth
During the first growing season, PP exhibited greater height growth than did DF (p < 0.0001), but end of growing season heights were not significantly different between species (Student’s t-test, p = 0.545). Height growth for both species decreased with distance from DWD (p = 0.0029) (Table 5). Seedlings exhibited highest height growth on the north side of DWD and on the north topographic aspect, compared to DWD south and the ridge and south topographic aspects respectively, although these differences were not significant. The interaction between DWD aspect and distance from DWD was not significant. Random effects for individual DWD and location were not significant, suggesting fairly uniform response over the extent of the experiment.
At the end of the second growing season, PP still exhibited greater height growth than did DF (p < 0.0001), and PP seedlings were taller on average compared to DF with a mean height of 31.9 cm compared to 28.5 cm (Student’s t-test, p = 0.0084). For seedlings of both species, height growth declined as distance from DWD increased (p = 0.0005). Seedlings exhibited marginally lower height growth on the south DWD aspect (p = 0.0515). Height growth in the second growing season did not appear to be affected by topographic aspect. The interaction between DWD aspect and distance from DWD was not significant (Table 5). The random effect for individual DWD was significant (p = 0.0182), suggesting some variability of height response between pieces of woody debris, but not for the location variable.
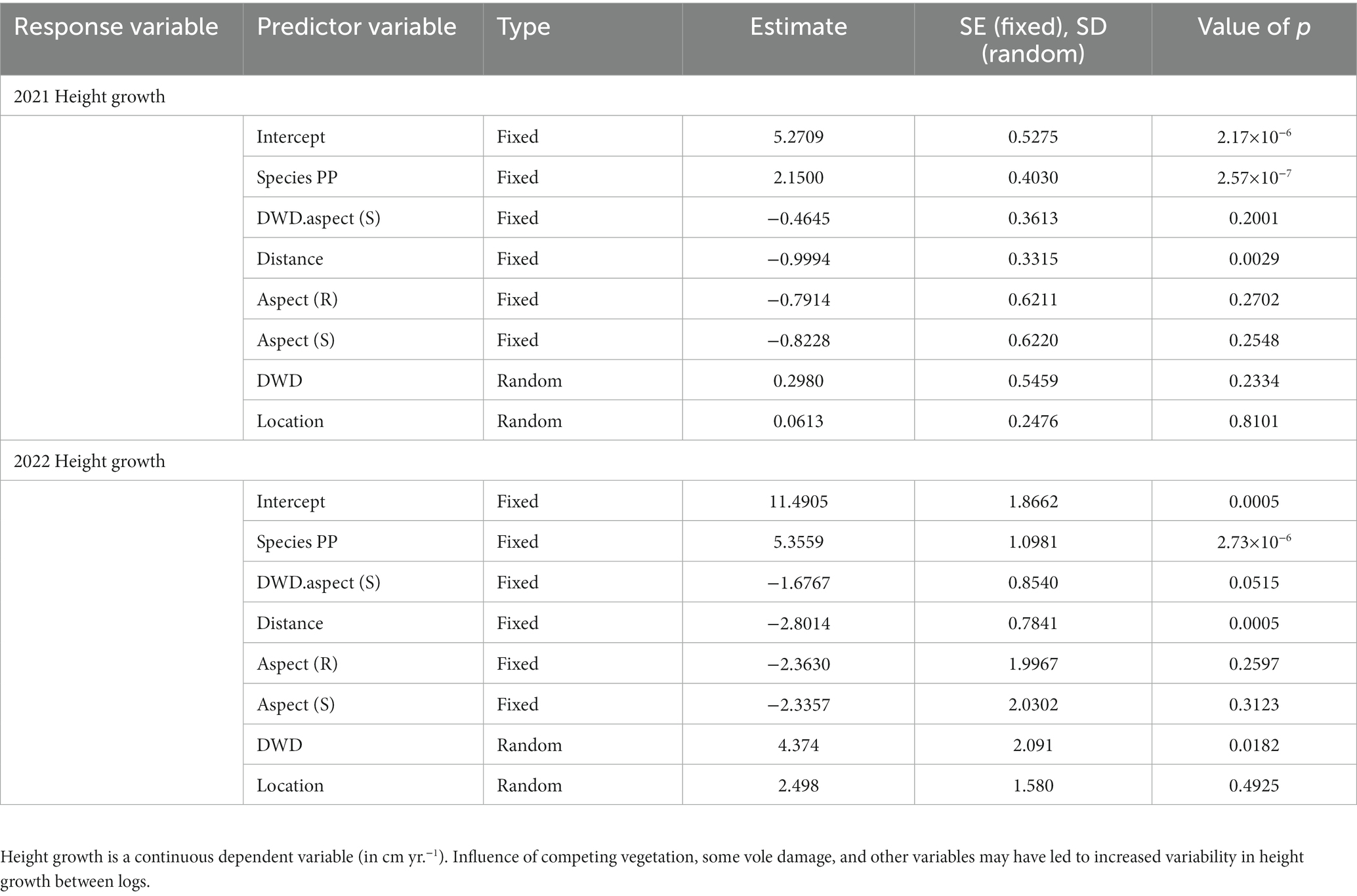
Table 5. Linear mixed model output with 2021 height growth and 2022 height growth as dependent variables.
3.5. Chlorophyll fluorescence
The average mid-season measure of Fv/Fm was 0.581. Independent of planting location, Fv/Fm values were significantly higher for PP seedlings than for DF seedlings (p < 0.0001). Fv/Fm values were greater on the north DWD aspect compared to the south DWD aspect (p < 0.0001) and decreased with increasing distance from DWD (p < 0.0001). Fv/Fm values were greatest on the north facing slope, exhibiting significant declines on the ridge (p = 0.0367) and on the south-facing slope (p = 0.0177; Figure 7; Table 6).
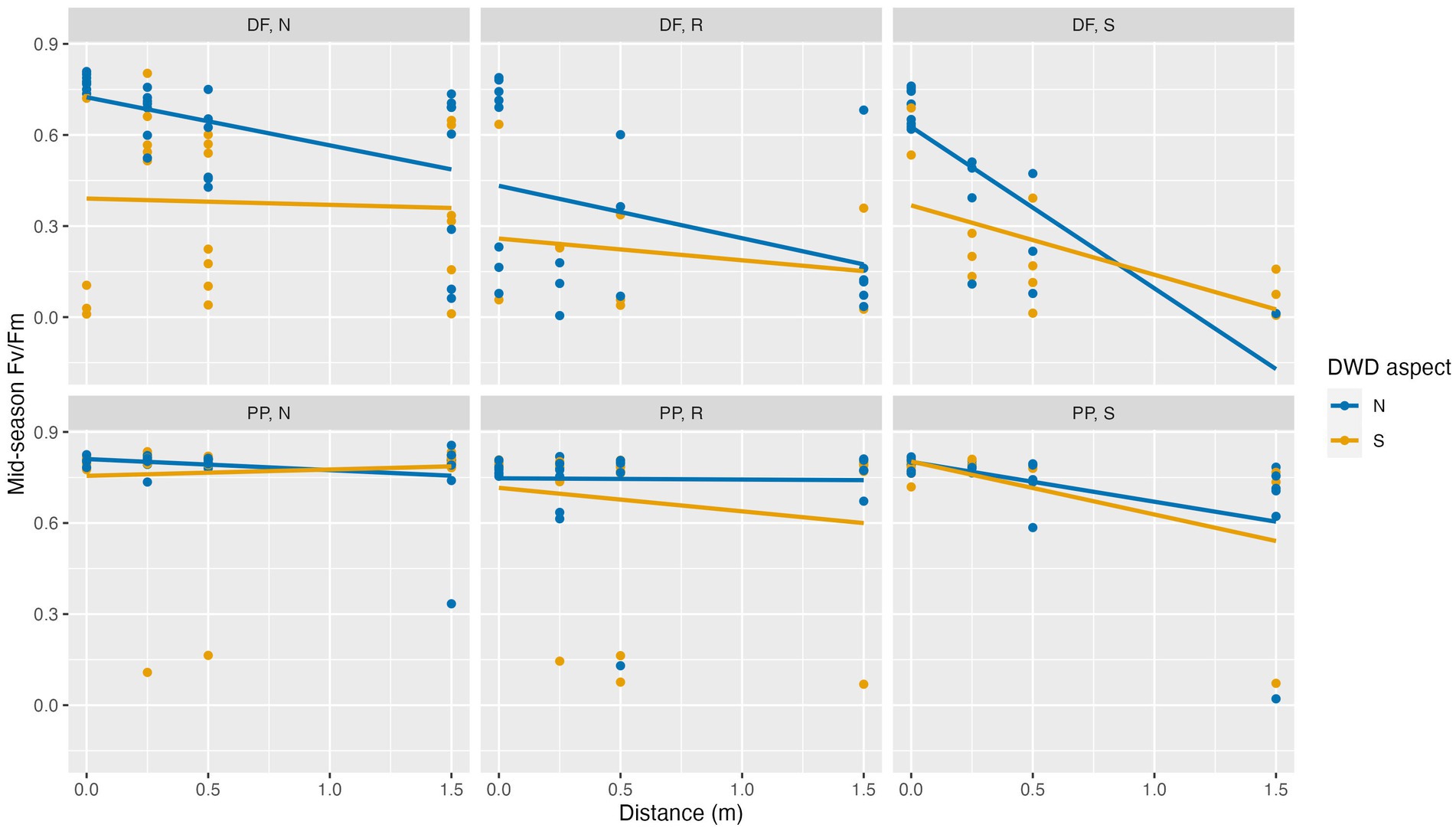
Figure 7. Dark-adapted chlorophyll fluorescence (Fv/Fm) for Douglas-fir (DF) and ponderosa pine (PP) seedlings planted at 0, 0.25, 0.5, and 1.5 m distance from DWD on the north (N) or south (S) sides of DWD on a north-facing slope (N), flat ridgeline (R), or south-facing slope (S). Measurements were made in June 2021, during the middle of the first growing season (n = 275). Note that dead seedlings (Fv/Fm value = 0) are excluded. Lines indicate linear trends estimated from the data.
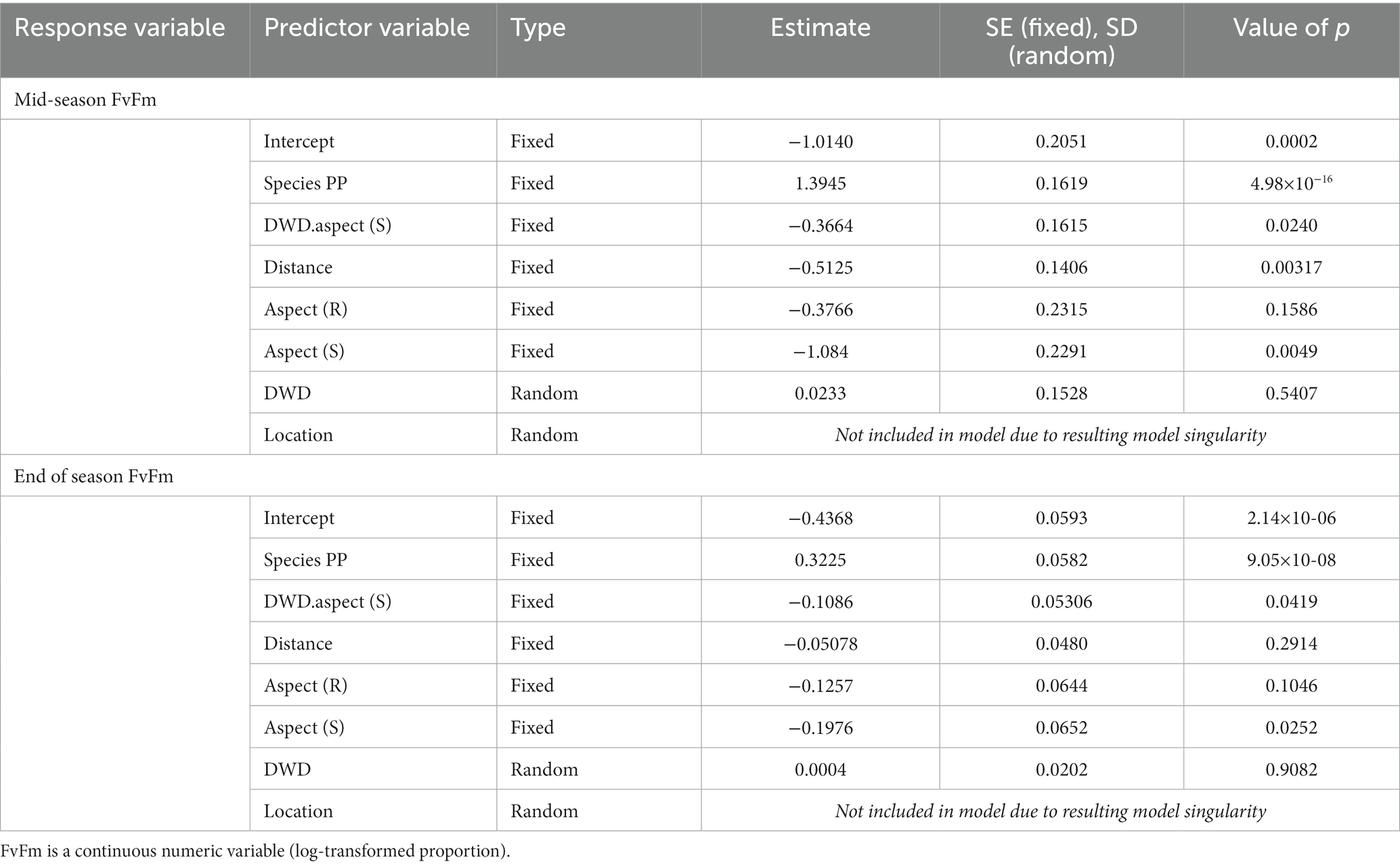
Table 6. Linear mixed model output for mid-season and end of season fluorescence (2021 growing season).
The average Fv/Fm value increased to 0.739 by the end of the first growing season. Fv/Fm values remained greater for PP compared to DF (p < 0.0001), and were significantly greater on the north DWD aspect compared to the south DWD aspect (p = 0.0419). The relationship between Fv/Fm values and distance to DWD was no longer statistically significant (p = 0.2914). Additionally, slope aspect influenced Fv/Fm values, with highest values observed on the north facing slope and significantly lower values on the south slope (p = 0.0252). The ridge top did not differ from the north slope (p = 0.1046). The interaction between DWD aspect and distance from DWD was not significant in models of either mid-season fluorescence or end of season fluorescence (Table 6). The random effects associated with specific log for models of both growing seasons were not significant, and the size of the coefficients was small, suggesting low variability in response between logs.
3.6. Mechanisms- soil surface temperature and volumetric water content
SST observations were lowest on the north facing slope, increasing on the ridge (p = 0.0021), and highest on the south facing slope (p < 0.0001) (Figure 8). SST increased as distance from DWD increased, peaking at the 1.5 m distance (p < 0.0001). Additionally, SST was greater on the south DWD aspect compared to the north DWD aspect (p < 0.0001). The interaction term between DWD aspect and distance was significant (p < 0.0001). On the south side of DWD, SST does not increase at the same rate with distance from DWD as it would on the north side of DWD (Table 7). However, at the 1.5 m distance there is little to no difference between SST on the north and south DWD aspects (Figure 8). The random effects for replicate log and location were small and not statistically significant, indicating little variation in response across the experiment.
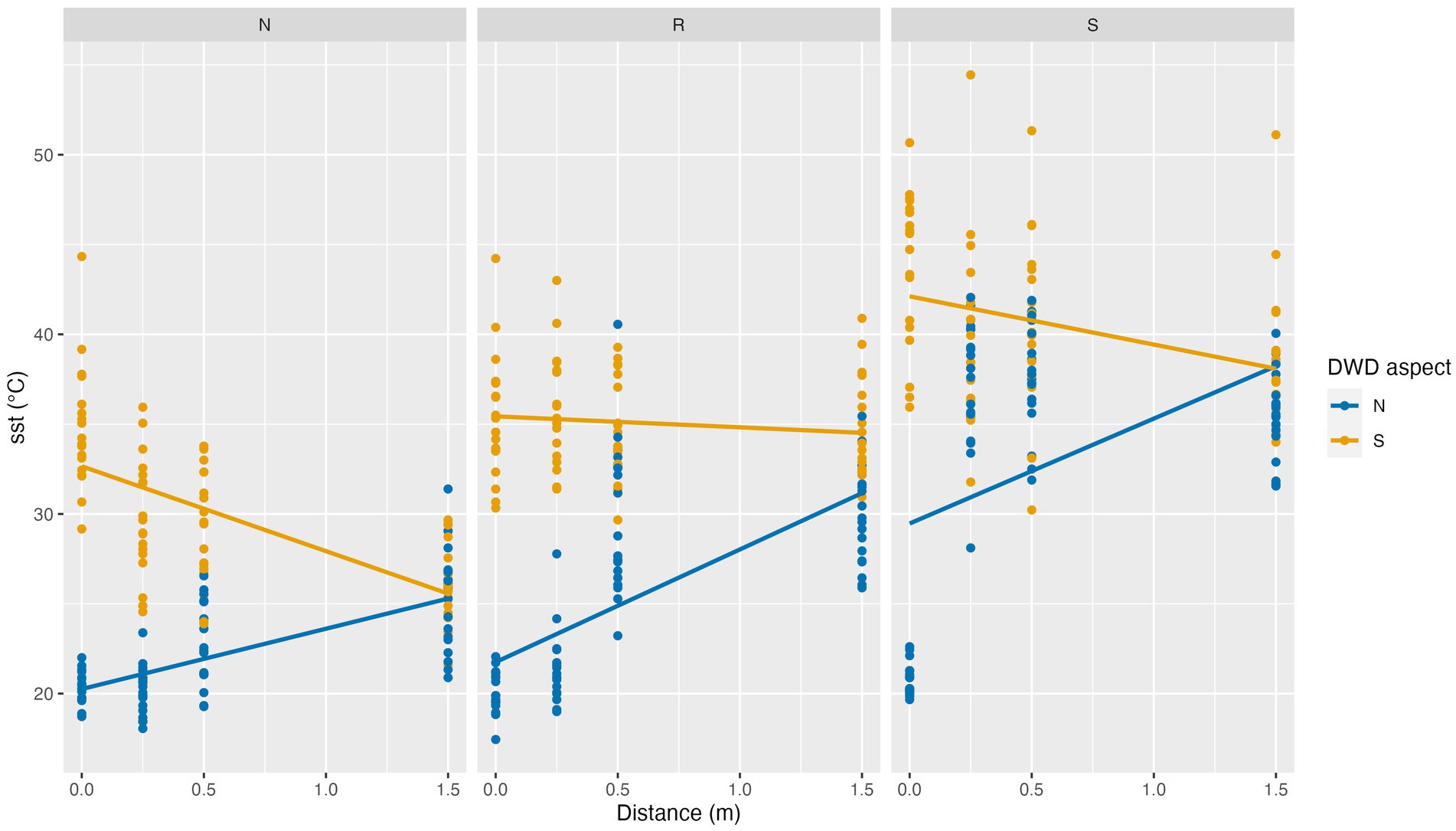
Figure 8. Soil surface temperature (SST) as a function of topographic aspect, distance to DWD, and DWD aspect (blue vs. orange symbols), September 25, 2021. Lines indicate linear trends estimated from the data.
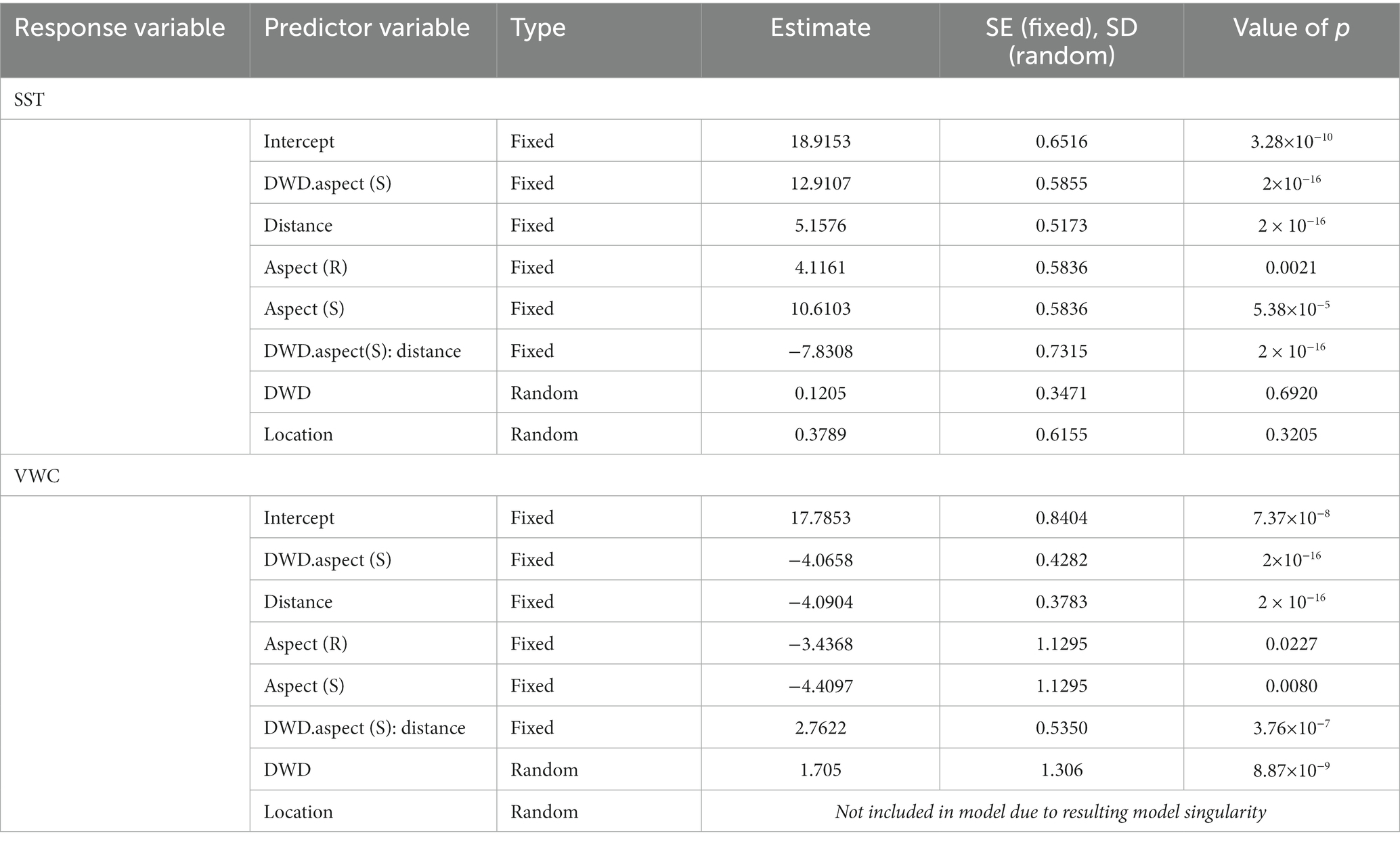
Table 7. Linear mixed model output for soil surface temperature (SST) and volumetric water content (VWC), both continuous numeric dependent variables.
VWC exhibited inverse trends to that of SST. On the site scale, VWC was greatest on the north facing slope, decreasing on the ridge (p = 0.0227) and reaching the lowest values on the south facing slope (p = 0.0080). VWC decreased with distance from DWD (p < 0.0001) and was higher on the north side of DWD compared to the south side (p < 0.001). VWC exhibited a smaller decline with increasing distance from DWD on the south DWD aspect than it does on the north (p < 0.0001) (Table 7). At the 1.5 m distance the difference in VWC between the north and south aspects of the DWD becomes negligible (Figure 9). The random effect for location could not be fit, as it resulted in model singularity. The random effect for replicate log was statistically significant, but small in magnitude, indicating some correlation in VWC within the neighborhood of the log.
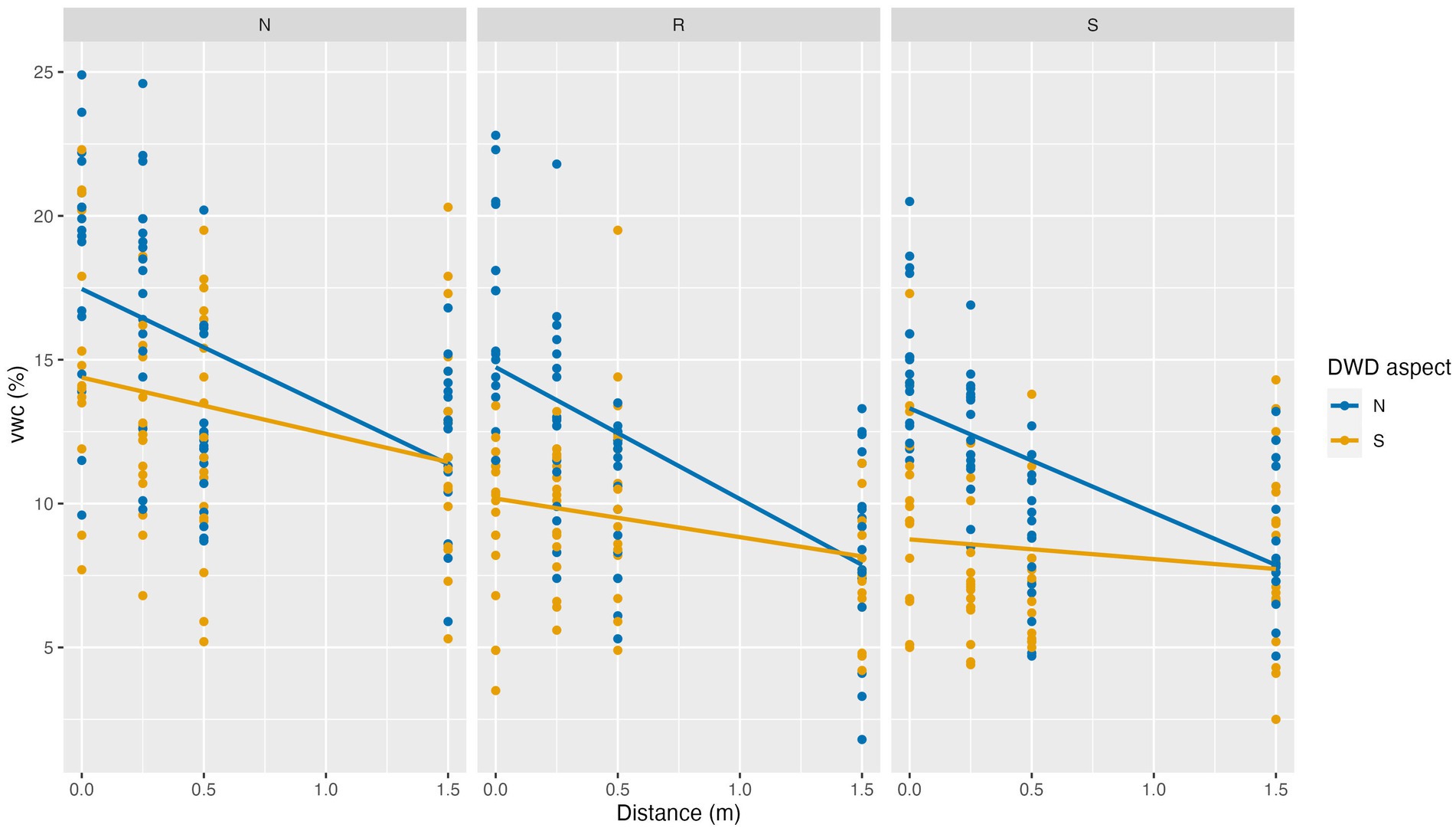
Figure 9. Soil volumetric water content (VWC) as a function of topographic aspect, distance to DWD, and DWD aspect (blue vs. orange symbols), May 12th, 2021. Lines indicate linear trends estimated from the data.
3.7. Vole mortality
Girdling by voles was one of few other sources of recognizable mortality. Vole girdling mortality was largely restricted to the zone proximate to DWD (Figure 10), with strong declines in vole caused mortality as distance from DWD increased (p < 0.0001, Table 8). Vole girdling mortality was also higher on the south side of logs (p = 0.0374). This mortality, however, was insufficient to change the general results of the models relating seedling survival and stress ratings to distance to DWD and DWD aspect. Vole damage did not significantly change with species or topographic aspect (Table 8).
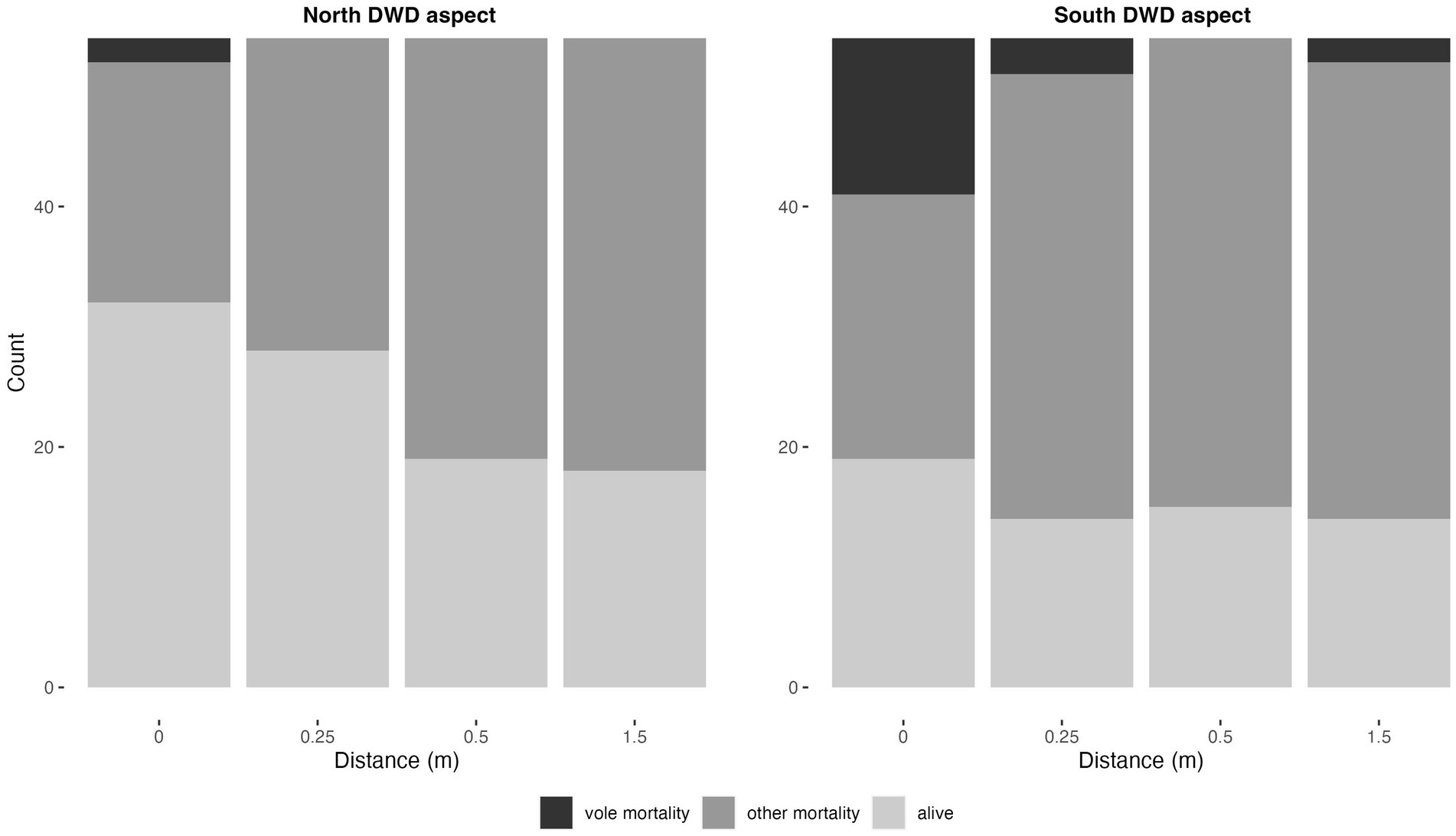
Figure 10. Total vole mortality for both the 2021 and 2022 growing seasons, pooled among species for the north and south sides of the DWD. Y-axis represents total number of seedlings with dark gray representing vole mortality, lighter gray representing mortality from other causes, and lightest gray representing alive seedlings.
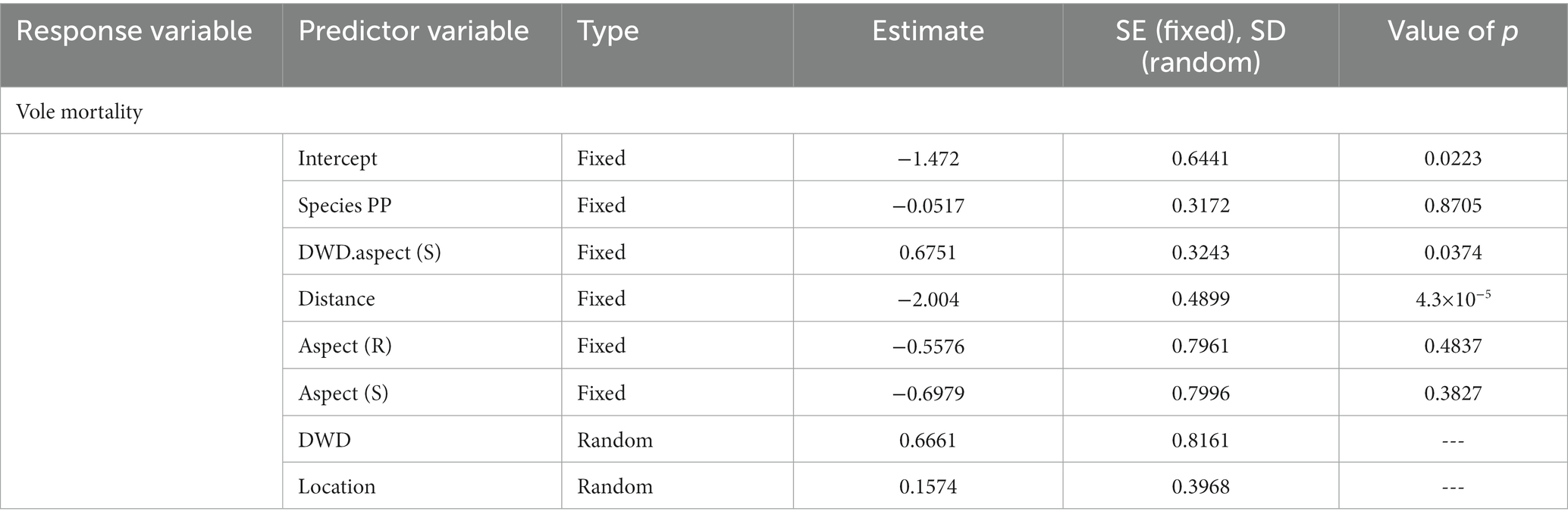
Table 8. Generalized linear mixed model (binomial dependent variable) output for vole mortality (0 = killed by voles, 1 = not killed by voles).
4. Discussion
4.1. Seedling responses to microsite effect and topography
The mesophyte DF was far more reliant on DWD-created microsites and the cooler, moister conditions found within the influence of the microsite on the north side of logs within 0.25 m of the log. The xerophyte PP seemed to respond to the higher soil moisture level found adjacent to the DWD on both north and south sides. This suggests that moisture availability, not temperature, was limiting to PP, while the mesophytic DF was sensitive to both temperature and moisture availability. Snow tended to drift against the DWD on both south and north sides during the winter months (unpublished data). This would then translate to increased soil moisture adjacent to the DWD in the spring months, an effect which would persist into the growing season. Rainwater may also be shed from the superior surfaces of woody debris onto the area beneath the dripline, or outer margin of the woody debris, also increasing available moisture within a short distance of the log. Further research is needed to investigate the role of DWD on redistribution of both snow and rain forms of precipitation.
At low elevations in the Palouse and foothills of adjacent mountains, first year survival of planted ponderosa pine and Douglas-fir seedlings may be expected to be 30–50% and 20–30%, respectively, without control of competing vegetation (R. Schaefer IV, Alpha Services, pers. comm.). Survival of our planted stock on the immediate north side of DWD exceeded these general survival probabilities, emphasizing the facilitative role of reduced SST and increased soil VWC.
A number of individuals surviving to season 2 of the experiment “recovered” to a lower stress rating, following an increase in stress during the first growing season. This reflects the stressful initial phase of seedling establishment, which is crucial as a filter on tree species persistence in marginal environments (Clark-Wolf et al., 2022).
Both SST and VWC were strongly modified by DWD, despite the pieces of DWD having been placed just one year prior to the planting of the monitored seedlings. These variables have been shown to be of primary importance in tree seedling survival and growth (Hill and Ex, 2020; Wooten et al., 2022). This work demonstrates that even recently placed DWD can have a relatively immediate effect on biophysical conditions on a site. These moderating effects can operate even during extremes of climate, such as the “Heat Dome” event of the summer of 2021. As such events increase in frequency (Horton et al., 2016), the presence of DWD may become even more important to maintain conifer regeneration on dry sites or sites with high solar heat load (e.g., south-facing slopes). VWC, which we measured at 10 cm depth in this study, may have decreased in importance as a predictor of survival or vigor, since 2nd year seedlings will have increased the depth of their taproots, and are accessing deeper soil moisture persisting later into the growing season (Daubenmire, 1968). Future work should examine the effect of DWD on deeper soil moisture, if any. SST, however, was still a significant predictor of stress in the second growing season, as above-ground portions of seedlings are still subjected to temperature extremes.
Topographic aspect was a significant independent variable for the majority of either biophysical or plant-level responses, suggesting topographic setting is a crucial top-down constraint on the importance of DWD-associated microsites. The nearly exclusive association of conifer stress ratings with the immediate north side of the DWD seen on the south-facing and ridge-top settings was relaxed considerably on the relatively mesic north-facing slope. This suggests that DWD-associated microsite protection is less critical as a component of reforestation or restoration efforts on north or northeast aspects, but much more important on flat surfaces or warm slopes (e.g., south or southwest aspects). This, of course, may become less important with increasing elevation, or in more mesic plant communities, since the importance of microsite effects may vary with elevation (Callaway, 1998; García-Camacho et al., 2010). The effects of topographic aspect, and the relative influence of woody debris-created microsites, may therefore be even stronger on steeper slopes than those studied in this work, since heat load is a function of both aspect and slope (McCune and Keon, 2002).
4.2. Discussion of methods
Herbivory by rodents (primarily voles, Microtus spp.) was tightly associated with the placed DWD. This suggests the potential for a countervailing influence of DWD presence that may reduce, or act in opposition to, the benefit of DWD to tree seedlings survival. In our study, observed mortality from voles was not sufficient to obscure the primary patterns of survival and growth. The vole mortality observed in this study did not change regression model coefficients, whether included in model generation or removed from the data set. However, rodent-associated mortality has the potential to substantially alter conifer seedling survival, especially during peaks of rodent population cycles (Sullivan and Sullivan, 2018). Due to rodent use of DWD as a protective element against predation, girdling mortality may reach higher levels in the immediate vicinity of DWD, potentially counteracting the beneficial functions of microsite protection.
Although not reported in this paper, competing vegetation increased dramatically next to the logs. Tall forbs such as prickly lettuce (Lactuca serriola) and tall annual willowherb (Epilobium brachycarpum, syn. E. paniculatum) appeared in profusion on both sides of the logs, regardless of topographic aspect, and were absent 0.5 m from the logs. These doubtless competed with seedlings for nutrients and moisture, but there seemed to be no negative effect on the conifer seedlings, even those overtopped by these vigorous forbs. It may be that the additional shading from the crowns of these forbs even adds to the benefit of the log-created microsite.
Another effect that became apparent during the course of research, but did not proceed to a level where it affected outcomes of growth or survival, was the sloughing of bark from sections of several DWD pieces. Decorticating bark has the potential to displace, crush, or shade seedlings and may heighten the variability of benefit obtained by seedlings within the optimal microsite zone under natural post-disturbance conditions (Harmon, 1989).
Size, species, compass orientation, and decay class of DWD (Sollins, 1982) was held constant in this research. However, future research might address how larger or more decayed DWD might influence the results observed here, with changes in color (and thus reflectance/absorption of light, and dependent heat load), moisture content, or physical stability possibly providing different outcomes for seedlings. One effect that seems important for further work is the zone underneath the overhang of the DWD, where shade is maximal, but moisture input might be reduced. Our measurements at the 0.0 m distance were taken at the “dripline” or outer edge of the DWD, and thus did not necessarily reflect conditions under the DWD.
Seedling stock type is an important consideration in forest regeneration operations. We used containerized seedlings for this research, which have been shown to have greater survival and growth in dry or low elevation sites than bare-root seedlings (Stein and Owston, 1977; McDonald, 1991). Future research should investigate the response of different stock types to microsite facilitation by DWD.
4.3. Implications for management and restoration
Davis et al. (2019) clearly demonstrate increasing challenges to successful tree regeneration in western North America, highlighting the role of increasing soil temperature, increasing vapor pressure deficit, and decreasing soil moisture. This work, in combination with our findings, suggests that amelioration of growing conditions at the microsite scale may be a key adaptive strategy for management of tree regeneration in post-disturbance ecosystems. Relatively warm dry settings could benefit from either retention or importation of woody elements (Marcolin et al., 2019). DWD may or may not be abundant in early seral pre-forest (Harmon et al., 1986; Roccaforte et al., 2012) lending stochasticity to the availability of microsites that favor tree regeneration. In addition, salvage logging, when conducted exhaustively, can reduce DWD available for influencing ecological processes (Lindenmayer et al., 2012). Our work agrees with that of Marangon et al. (2022), in that we found that north-facing microsites are characterized by significantly lower soil temperatures and higher volumetric water content. Our work differs from theirs in that we analyzed a greater number of distances of planted stock to explore the dimensions of the microsite effect to a greater degree; in addition, our work involved the experimental placement of DWD, while their investigation utilized naturally windthrown elements.
Disturbed sites may benefit from the presence of DWD with respect to enhanced conifer regeneration, especially permitting mesophytic conifer species to survive on a site. Managers frequently decide to remove large DWD due to perceived contribution to fire hazard, for economic reasons, or to expose more surface area for planting species that require root contact with mineral soil (Spies and Cline, 1988). This research suggests that retention of at least some percentage of post-harvest or post-disturbance DWD may be advisable to provide protective microsites, in addition to the other recognized functions of DWD such as provision of wildlife habitat (Maser et al., 1979; Harmon et al., 1986). Given our finding of greater survival of seedlings in all planting positions relative to DWD on the north-facing slope, the need for planting to occur on the north side of DWD may be less critical in cooler topographic aspects or settings.
In fire-prone environments, trees that began as seedlings in a DWD-created microsite may be at greater risk of mortality from fire effects (Dr. Robert Keefe, University of Idaho Experimental Forest, personal communication; see also Brown et al., 2003). Monsanto and Agee (2008), working in post-fire conifer forests of the east Washington Cascades, determined that fire-caused lethal temperatures occur in close proximity to woody debris both above- and belowground. These factors may limit the utility of DWD-created microsites if prescribed fire (particularly fall burns, when woody debris has minimum fuel moisture) is anticipated as a part of early stand management. Further research to understand the magnitude of this issue is warranted. In situations where mesophytic conifer regeneration is not desired by managers, who may wish to reduce future stand-level fuel loading or tailor stand composition to anticipated drier, warmer conditions, it may be advisable to avoid the creation of additional woody debris-associated microsites.
Alternatives to DWD for provision of shading and other microsite effects do exist, where DWD is inaccessible, economically infeasible to import, or where financial or fuel bed-related concerns preclude its use. Shrubs generally are regarded as competitors for soil moisture (e.g., Plamboeck et al., 2008), but may also act as a ‘nurse plant’, decreasing direct solar radiation and temperature, and increasing relative humidity around seedlings (Conard and Radosevich, 1982; Castro et al., 2004; Legras et al., 2010). Traditional silvicultural approaches such as shelterwood harvesting can decrease direct radiation and soil temperatures on droughty or warm sites, enhancing regeneration (Childs and Flint, 1987; Tesch and Mann, 1991). Shade cards, although costly, may reduce soil surface temperature and temperatures at the base of seedling stems, but they do not provide reductions in soil temperatures throughout the depth of the soil profile, as do shelterwood treatments (Childs and Flint, 1987). However, on many sites, DWD will be a reasonable option already present on site.
Allowing some trees within a stand to achieve greater age and larger diameter may increase their eventual value as DWD, since a larger tree will likely create a larger shaded microsite. While we held DWD diameter and length relatively constant to ensure a uniform experiment, we recommend that future research investigate the role of DWD dimensions in creating beneficial microsite conditions.
4.4. Conclusion
Studies such as this one should be repeated to understand the functioning of DWD more fully, especially with respect to variables such as species and diameter of the DWD and response of volunteer non-target vegetation and across the major forest or woodland types of North America. It is clear that DWD can exert a powerful influence on physical characteristics of a site and associated effects on tree demography. However, deeper understanding of such phenomena, and their spatial and temporal behaviors, could prove useful to successful incorporation of DWD into useful strategies for climate-adapted tree regeneration.
Data availability statement
The raw data supporting the conclusions of this article will be made available by the authors, without undue reservation.
Author contributions
MS and AN conceived the research idea and experimental design, obtained permission for site use, led installation, and were involved in data collection, data analysis, writing, and interpretation. AN provided seedling stock, grown at the University of Idaho’s Pitkin Forest Nursery. MM participated in data collection, played a pivotal role in data analysis, interpretation, and writing. HA participated in data collection, writing, interpretation, and initiated chlorophyll fluorescence measurements. RE provided critical data collection support and was involved in experimental design and installation. All authors contributed to the article and approved the submitted version.
Funding
MS and HA are supported in part by the USDA National Institute of Food and Agriculture, McIntire-Stennis project #1019284. MM was supported by the Emerging Research Issues in Agriculture program, grant #PG00019576, College of Agricultural, Human, and Natural Resource Sciences at Washington State University. Conifer seedlings were generously provided by the Franklin H. Pitkin Forest Nursery at the University of Idaho, while site permissions were provided by the School of the Environment, Washington State University.
Acknowledgments
MS thanks the Washington State University Forestry Club/Society of American Foresters Student Chapter for their volunteer work in harvesting and placing the logs, particularly highlighting the help of Jonathon Turner, Sean Alexander, Cole Shirley, and Elijah Loftis. John Fluegel, site manager at the E.H. Steffen Center, and Lisa A. Shipley, director of the Steffen Center, provided encouragement and support. The authors are grateful to Rob Smith of WSU Institutional Research, and Trey DeJong and David Rice of the Center for Interdisciplinary Statistical Education and Research at Washington State University for extremely helpful comments and advice regarding data analysis. Thanks to Gabrielle Mickelson for assistance with early field work, and to her friends Colin Kirk-Patterson and Austin Cozad for cheerful and energetic volunteer work with planting of the conifer seedlings. All authors are grateful for the incisive and thorough comments provided by two reviewers, which we believe have resulted in appreciable improvements to the article.
Conflict of interest
The authors declare that the research was conducted in the absence of any commercial or financial relationships that could be construed as a potential conflict of interest.
Publisher’s note
All claims expressed in this article are solely those of the authors and do not necessarily represent those of their affiliated organizations, or those of the publisher, the editors and the reviewers. Any product that may be evaluated in this article, or claim that may be made by its manufacturer, is not guaranteed or endorsed by the publisher.
Footnotes
1. ^National Weather Service, www.weather.gov.
References
Bailey, T. G., Davidson, N. J., and Close, D. C. (2012). Understanding the regeneration niche: microsite attributes and recruitment of eucalypts in dry forests. For. Ecol. Manag. 269, 229–238. doi: 10.1016/j.foreco.2011.12.021
Bolton, N. W., and D’Amato, A. W. (2011). Regeneration responses to gap size and coarse woody debris within natural disturbance-based silvicultural systems in northeastern Minnesota, USA. For. Ecol. Manag. 262, 1215–1222. doi: 10.1016/j.foreco.2011.06.019
Boucher, D., Gauthier, S., Thiffault, N., Marchand, W., Girardin, M., and Urli, M. (2020). How climate change might affect tree regeneration following fire at northern latitudes: a review. New For. 51, 543–571. doi: 10.1007/s11056-019-09745-6
Breshears, D. D., Fontaine, J. B., Ruthrof, K. X., Field, J. P., Feng, X., Burger, J. R., et al. (2021). Underappreciated plant vulnerabilities to heat waves. New Phytol. 231, 32–39. doi: 10.1111/nph.17348
Brown, J. K., Reinhardt, E. D., and Kramer, K. A. (2003). Coarse woody debris: managing benefits and fire hazard in the recovering forest. General technical report RMRS GTR-105. USDA Forest Service, Rocky Mountain Research Station: Ogden, UT.
Burns, R. M., and Honkala, B. H. (1990). “Silvics of North America, Volume 1, Conifers” in U.S.D.A. Forest Service Agriculture 654 (Washington, DC: USDA).
Callaway, R. M. (1998). Competition and facilitation on elevation gradients in subalpine forests of the northern Rocky Mountains, USA. Oikos 82, 561–573. doi: 10.2307/3546376
Castro, J., Zamora, R., Hodar, J. A., Gomez, J. M., and Gómez-Aparicio, L. (2004). Benefits of using shrubs as nurse plants for reforestation in Mediterranean mountains: a 4-year study. Restor. Ecol. 12, 352–358. doi: 10.1111/j.1061-2971.2004.0316.x
Cavender-Bares, J., and Bazzaz, A. F. (2004). From leaves to ecosystems: using chlorophyll fluorescence to assess photosynthesis and plant function in ecological studies. Chlorophyll a Fluorescence 19, 737–755. doi: 10.1007/978-1-4020-3218-9_29
Childs, S. W., and Flint, L. E. (1987). Effects of shadecards, shelterwoods, and clearcuts on temperature and moisture environments. For. Ecol. Manag. 18, 205–217. doi: 10.1016/0378-1127(87)90161-7
Christensen, R. H. B. (2022). “Ordinal—regression models for ordinal data.” R package version 2022.11-16. Available at: https://CRAN.R-project.org/package=ordinal (Accessed August, 2023).
Clark-Wolf, K., Higuera, P. E., and Davis, K. T. (2022). Conifer seedling demography reveals mechanisms of initial forest resilience to wildfires in the northern Rocky Mountains. For. Ecol. Manag. 523:120487. doi: 10.1016/j.foreco.2022.120487
Conard, S. G., and Radosevich, S. R. (1982). Post-fire succession in White fir (Abies Concolor) vegetation of the northern Sierra Nevada. Madrono 29, 42–56.
Daubenmire, R. (1968). Soil moisture in relation to vegetation distribution in the mountains of northern Idaho. Ecology 49, 431–438. doi: 10.2307/1934109
Davis, K. T., Dobrowski, S. Z., Higuera, P. E., Holden, Z. A., Veblen, T. T., Rother, M. T., et al. (2019). Wildfires and climate change push low-elevation forests across a critical climate threshold for tree regeneration. Proc. Natl. Acad. Sci. 116, 6193–6198. doi: 10.1073/pnas.1815107116
Donaldson, N. C. (1980). Soil survey of Whitman County, Washington. Pullman, PA: Soil Conservation Service and Washington State University.
Fitzgerald, S. A. (2008). Successful reforestation: An overview. Oregon State University Extension Service: Corvallis, OR, 1498.
Flint, L. E., and Childs, S. W. (1987). Effect of shading, mulching and vegetation control on Douglas-fir seedling growth and soil water supply. For. Ecol. Manag. 18, 189–203. doi: 10.1016/0378-1127(87)90160-5
Franklin, J. F., and Dyrness, C. T. (1988). Natural vegetation of Oregon and Washington. Corvallis, OR: Oregon State University Press.
Franklin, J. F., Lindenmayer, D., MacMahon, J. A., McKee, A., Magnuson, J., Perry, D. A., et al. (2000). Threads of continuity. Conserv. Biol. Pract. 1, 8–17. doi: 10.1111/j.1526-4629.2000.tb00155.x
García-Camacho, R., Iriondo, J. M., and Escudero, A. (2010). Seedling dynamics at elevation limits: complex interactions beyond seed and microsite limitations. Am. J. Bot. 97, 1791–1797. doi: 10.3732/ajb.1000248
Goldin, S. R., and Hutchinson, M. F. (2014). Coarse woody debris reduces the rate of moisture loss from surface soils of cleared temperate Australian woodlands. Soil Res. 52, 637–644. doi: 10.1071/SR13337
Goldin, S. R., and Hutchinson, M. F. (2015). Thermal refugia in cleared temperate Australian woodlands: coarse woody debris moderate extreme surface soil temperatures. Agric. For. Meteorol. 214-215, 39–47. doi: 10.1016/j.agrformet.2015.07.011
Goor, A. Y., and Barney, C. W. (1968). Forest tree planting in arid zones. New York: The Ronald Press Co.
Gray, A. N., and Spies, T. A. (1997). Microsite controls on tree seedling establishment in conifer forest canopy gaps. Ecology 78, 2458–2473. doi: 10.1890/0012-9658(1997)078[2458:MCOTSE]2.0.CO;2
Guadagno, C. R., Ewers, B. E., Speckman, H. N., Aston, T. L., Huhn, B. J., DeVore, S. B., et al. (2017). Dead or alive? Using membrane failure and chlorophyll a fluorescence to predict plant mortality from drought. Plant Physiol. 175, 223–234. doi: 10.1104/pp.16.00581
Harmon, M. E. (1989). Effects of bark fragmentation on plant succession on conifer logs in the Picea-Tsuga forests of Olympic National Park, Washington. Am. Midland Naturalist 121, 112–124. doi: 10.2307/2425662
Harmon, M. E., and Franklin, J. F. (1989). Tree seedlings on logs in Picea- Tsuga forests of Oregon and Washington. Ecology 70, 48–59. doi: 10.2307/1938411
Harmon, M. E., Franklin, J. F., Swanson, F. J., Sollins, P., Gregory, S. V., Lattin, J. D., et al. (1986). Ecology of coarse woody debris in temperate ecosystems. Adv. Ecol. Res. 15, 133–302. doi: 10.1016/S0065-2504(08)60121-X
Heinemann, K., and Kitzberger, T. (2006). Effects of position, understory vegetation and coarse woody debris on tree regeneration in two environmentally contrasting forests of North-Western Patagonia: a manipulative approach. J. Biogeogr. 33, 1357–1367. doi: 10.1111/j.1365-2699.2006.01511.x
Hill, E. M., and Ex, S. (2020). Microsite conditions in a low-elevation Engelmann spruce forest favor ponderosa pine establishment during drought conditions. For. Ecol. Manag. 463:118037. doi: 10.1016/j.foreco.2020.118037
Horton, R. M., Mankin, J. S., Lesk, C., Coffel, E., and Raymond, C. (2016). A review of recent advances in research on extreme heat events. Curr. Clim. Change Rep. 2, 242–259. doi: 10.1007/s40641-016-0042-x
Kemp, K. B., Higuera, P. E., Morgan, P., and Abatzoglou, J. T. (2019). Climate will increasingly determine post-fire tree regeneration success in low-elevation forests, northern Rockies, USA. Ecosphere 10:e02568. doi: 10.1002/ecs2.2568
Kitajima, M., and Butler, W. L. (1975). Quenching of chlorophyll fluorescence and primary photochemistry in chloroplasts by dibromothymoquinone. BBA-Bioenergetics 376, 105–115. doi: 10.1016/0005-2728(75)90209-1
Kuijper, D. P., Cromsigt, J. P., Jędrzejewska, B., Miścicki, S., Churski, M., Jędrzejewski, W., et al. (2010). Bottom-up versus top-down control of tree regeneration in the Białowieża primeval Forest, Poland. J. Ecol. 98, 888–899. doi: 10.1111/j.1365-2745.2010.01656.x
Kuznetsova, A., Brockhoff, P. B., and Christensen, R. H. B. (2017). lmerTest package: tests in linear mixed effects models. J. Stat. Softw. 82, 1–26. doi: 10.18637/jss.v082.i13
Legras, E. C., Wall, S. B. V., and Board, D. I. (2010). The role of germination microsite in the establishemtn of sugar pine and Jeffrey pine. For. Ecol. Manag. 260, 806–813. doi: 10.1016/j.foreco.2010.05.039
Lindenmayer, D. B., Burton, P. J., and Franklin, J. F. (2012). Salvage logging and its ecological consequences. Washington, DC: Island Press.
Marangon, D., Marchi, N., and Lingua, E. (2022). Windthrown elements: a key point improving microsite amelioration and browsing protection to transplanted seedlings. For. Ecol. Manag. 508:120050. doi: 10.1016/j.foreco.2022.120050
Marcolin, E., Marzano, R., Vitali, A., Garbarino, M., and Lingua, E. (2019). Post-fire management impact on natural forest regeneration through altered microsite conditions. Forests 10:1014. doi: 10.3390/f10111014
Marsh, C., Krofcheck, D., and Hurteau, M. D. (2022). Identifying microclimate tree seedling refugia in post-wildfire landscapes. Agric. For. Meteorol. 313:108741. doi: 10.1016/j.agrformet.2021.108741
Marzano, R., Garbarino, M., Marcolin, E., Pividori, M., and Lingua, E. (2013). Deadwood anisotropic facilitation on seedling establishment after a stand-replacing wildfire in Aosta Valley (NW Italy). Ecol. Eng. 51, 117–122. doi: 10.1016/j.ecoleng.2012.12.030
Maser, C., Anderson, R. G., Cromack, K. J., Williams, J. T., and Martin, R. E. (1979). “Dead and down woody material” in Wildlife habitats in managed forests: The Blue Mountains of Oregon and Washington. ed. J. W. Thomas (Washington, DC: Wildlife Management Institute), 78–95.
McCune, B., and Keon, D. (2002). Equations for potential annual direct incident radiation and heat load. J. Veg. Sci. 13, 603–606. doi: 10.1111/j.1654-1103.2002.tb02087.x
McDonald, P. M. (1991). Container seedlings outperform barefoot stock: survival and growth after 10 years. New For. 5, 147–156. doi: 10.1007/BF00029305
Meigs, G. W., Case, M. J., Churchill, D. J., Hersey, C. M., Jeronimo, S. M., and Smith, L. A. C. (2022). Drought, wildfire and forest transformation: Characterizing trailing edge forests in the eastern Cascade Range, Washington, USA. Forestry 96, 340–354. doi: 10.1093/forestry/cpac046
Mitchell, W. K., Dunsworth, G., Simpson, D. G., and Vyse, A. (1990). “Planting and seeding. Ch. 18” in Regenerating British Columbia’s forests. eds. D. P. Lavender, R. Parish, C. M. Johnson, G. Montgomery, A. Vyse, and R. A. Willis, et al. (Vancouver, BC: University of British Columbia Press)
Monsanto, P. G., and Agee, J. K. (2008). Long-term post-wildfire dynamics of coarse woody debris after salvage logging and implications for soil heating in dry forests of the eastern cascades, Washington. Forest Ecol. Manag. 255, 3952–3961. doi: 10.1016/j.foreco.2008.03.048
Motta, R., Berretti, R., Lingua, E., and Piussi, P. (2006). Coarse woody debris, forest structure, and regeneration in the Valbona Forest reserve, Paneveggio, Italian Alps. For. Ecol. Manag. 235, 155–163. doi: 10.1016/j.foreco.2006.08.007
Neter, J., Kutner, M. H., Nachtsheim, C. J., and Wasserman, W. (1996). Applied linear statistical models. Homewood, IL: Richard D. Irwin.
Oliver, C. D. (1980). Forest development in North America following major disturbances. For. Ecol. Manag. 3, 153–168. doi: 10.1016/0378-1127(80)90013-4
Petrie, M. D., Bradford, J. B., Hubbard, R. M., Lauenroth, W. K., Andrews, C. M., and Schlaepfer, D. R. (2017). Climate change may restrict dryland forest regeneration in the 21st century. Ecology 98, 1548–1559. doi: 10.1002/ecy.1791
Plamboeck, A. H., North, M., and Dawson, T. E. (2008). Conifer seedling survival under closed-canopy and Manzanita patches in the Sierra Nevada. Madrono 55, 191–201. doi: 10.3120/0024-9637-55.3.191
Quinn, G. P., and Keough, M. J. (2002). Experimental design and data analysis for biologists. Cambridge university press.
R Core Team. (2022). R: A language and environment for statistical computing. R Foundation for Statistical Computing, Vienna, Austria. Available at: https://www.R-project.org/ (Accessed August, 2023).
Reely, J. A., and Nelson, A. S. (2021). Root growth potential and microsite effects on conifer seedling establishment in northern Idaho. Forests 12:597. doi: 10.3390/f12050597
Roccaforte, J. P., Fulé, P. Z., Chancellor, W. W., and Laughlin, D. C. (2012). Woody debris and tree regeneration dynamics following severe wildfires in Arizona ponderosa pine forests. Can. J. For. Res. 42, 593–604. doi: 10.1139/x2012-010
Seidl, R., and Turner, M. G. (2022). Post-disturbance reorganization of forest ecosystems in a changing world. Proc. Natl. Acad. Sci. 119:e2202190119. doi: 10.1073/pnas.2202190119
Sollins, P. (1982). Input and decay of coarse woody debris in coniferous stands in western Oregon and Washington. Can. J. For. Res. 12, 18–28. doi: 10.1139/x82-003
Spies, T. A., and Cline, S. P. (1988). Coarse woody debris in forests and plantations of coastal Oregon. From the forest to the sea: a story of fallen trees. Gen. Tech. Rep. PNW-GTR-229. Portland, OR: Pacific Northwest Research Station, Forest Service, US Department of Agriculture, pp.5–23.
Stein, W. I., and Owston, P. W. (1977). Containerized seedlings in western reforestation. J. For. 75, 575–578.
Stevens-Rumann, C. S., Kemp, K. B., Higuera, P. E., Harvey, B. J., Rother, M. T., Donato, D. C., et al. (2018). Evidence for declining forest resilience to wildfires under climate change. Ecol. Lett. 21, 243–252. doi: 10.1111/ele.12889
Still, C. J., Sibley, A., DePinte, D., Busby, P. E., Harrington, C. A., Schulze, M., et al. (2023). Causes of widespread foliar damage from the June 2021 Pacific northwest heat dome: more heat than drought. Tree Physiol. 43, 203–209. doi: 10.1093/treephys/tpac143
Sullivan, T. P., and Sullivan, D. S. (2018). Long-tailed vole (Microtus longicaudus) population outbreaks and refugia after clearcutting of coniferous forests: the search for fluctuations and hotspots. Crop Prot. 112, 49–55. doi: 10.1016/j.cropro.2018.05.008
Swanson, M. E., Franklin, J. F., Beschta, R. L., Crisafulli, C. M., DellaSala, D. A., Hutto, R. L., et al. (2011). The forgotten stage of forest succession: early-successional ecosystems on forest sites. Front. Ecol. Environ. 9, 117–125. doi: 10.1890/090157
Takahashi, M., Sakai, Y., Ootomo, R., and Shiozaki, M. (2000). Establishment of tree seedlings and water-soluble nutrients in coarse woody debris in an old-growth Picea-Abies forest in Hokkaido, northern Japan. Can. J. For. Res. 30, 1148–1155. doi: 10.1139/x00-042
Tepley, A. J., Thompson, J. R., Epstein, H. E., and Anderson-Teixeira, K. J. (2017). Vulnerability to forest loss through altered postfire recovery dynamics in a warming climate in the Klamath Mountains. Glob. Chang. Biol. 23, 4117–4132. doi: 10.1111/gcb.13704
Tesch, S. D., and Mann, J. W. (1991). Clearcut and shelterwood reproduction methods for regenerating Southwest Oregon forests [Technical Report]. Research Bulletin 72, Forest Research Lab, College of Forestry, Oregon State University.
U.S. Climate Data. (2023). U.S. Climate data. Washington: Weather Service. https://www.usclimatedata.com/
Urza, A. K., Weisberg, P. J., Chambers, J. C., and Sullivan, B. W. (2019). Shrub facilitation of tree establishment varies with ontogenetic stage across environmental gradients. New Phytol. 223, 1795–1808. doi: 10.1111/nph.15957
White, R. H., Anderson, S., Booth, J. F., Braich, G., Draeger, C., Fei, C., et al. (2023). The unprecedented Pacific northwest heatwave of June 2021. Nat. Commun. 14:727. doi: 10.1038/s41467-023-36289-3
Keywords: downed woody debris, microsite, seedling survival, heat, topography, climate adaptive reforestation
Citation: Swanson ME, Magee MI, Nelson AS, Engstrom R and Adams HD (2023) Experimental downed woody debris-created microsites enhance tree survival and growth in extreme summer heat. Front. For. Glob. Change. 6:1224624. doi: 10.3389/ffgc.2023.1224624
Edited by:
Di Yang, University of Wyoming, United StatesReviewed by:
Don C. Bragg, Forest Service (USDA), United StatesBianca Eskelson, University of British Columbia, Canada
Copyright © 2023 Swanson, Magee, Nelson, Engstrom and Adams. This is an open-access article distributed under the terms of the Creative Commons Attribution License (CC BY). The use, distribution or reproduction in other forums is permitted, provided the original author(s) and the copyright owner(s) are credited and that the original publication in this journal is cited, in accordance with accepted academic practice. No use, distribution or reproduction is permitted which does not comply with these terms.
*Correspondence: Mark E. Swanson, bWFya3N3YW5zb25Ad3N1LmVkdQ==; Margaret I. Magee, bWFyZ2FyZXQubWFnZWVAd3N1LmVkdQ==