- 1Department of Geography, University of Bonn, Bonn, Germany
- 2Institute for Environment and Human Security, United Nations University, Bonn, Germany
While healthy forest ecosystems deliver various services that can reduce flood risk, they can also contribute to flooding by providing wood that potentially contributes to the clogging of waterways and associated backwater effects. In this regard, deadwood, as a key aspect of healthy forests, is often in focus of post-flood disaster discourses. This research reflects on this ambiguity in the different forest management goals when it comes to managing forests for flood risk reduction versus forest health. A working definition of forest health will be presented and an overview of the different aspects of how a forest potentially can affect the flood hazard will be provided. This will refer to the ways forests influence (1) the discharge of water from the landscape into channels and (2) the characteristics of the channel and its riparian area and their respective influence on the transport of water, sediment, and debris. Often these two determining factors for the development of the flood peak are discussed separately and by different academic fields. This paper aims to connect the existing knowledge spheres and discusses the synergies and trade-offs. The review shows that the two objectives of forest health and flood risk reduction are largely synergetic. However, in direct proximity to watercourses trade-offs might occur. This is especially due to the ambivalent relation of living vegetation and deadwood to flood hazard. In places without susceptible infrastructures to clogging, deadwood and diverse vegetation structures should be supported due to their beneficial effects on water retention and channel characteristics. In places where susceptible infrastructures exist, trade-offs between the two objectives arise. Here the potential of freshly uprooted vegetation to cause damages should be reduced while maintaining the vegetation’s supportive characteristics, for example, concerning bank and slope stability. Where the risk of clogging is assessed as too high, also the selective removal or shortening of dead in-channel Large Wood can be considered. However, based on the literature review the risk deriving from dead Large Wood is evaluated as comparably low. This is related to its generally lower proportions and its smaller and less stable characteristics compared to freshly uprooted vegetation.
1. Introduction
In mid-July 2021 Western Europe experienced severe floods. More than 220 people died in Germany and Belgium and huge public and private economic damages occurred amounting to €46 billion in Europe of which €33 billion accounted to Germany. This makes it the costliest extreme weather event in Germany and Europe to date (Munich RE, 2022). One particularly affected area is the Ahr valley in the low-ranging mountain area of Eifel, Germany. Within a few hours, the water level rose several meters. At 7 p.m. on the 14th of July, the flood wave exceeded the historic mark of 3.2 m meters in the small city of Altenahr, Rhineland-Palatinate (RLP). Just 1 h later it overflowed the gauge at a water level of more than 5 m. The water level reached its highest level of about 10 m in the early morning of the 15th of July (Landesamt für Umwelt Rheinland-Pfalz, 2022). Ultimately, more than 130 humans lost their lives and more than 42,000 people are affected in the Ahr valley (Deutsches Komitee Katastrophenvorsorge e.V, 2022). Despite recovery and aid initiatives many people in the area continue to suffer from the effects of the flood due to, i.e., property loss, livelihood disruption, and mental health effects (Welsch, 2022).
The flood was triggered by the low-pressure system “Bernd,” which brought regionally pronounced heavy rainfall in large parts of river basins. In West Germany, 60–180 mm of rain fell in only 22 h on the 14th of July. These large quantities of water quickly ran off due to the prevailing orographic conditions and already saturated soils. In the partly narrow valleys, the water got channeled and led to quickly rising streams (Dietze and Ozturk, 2021; Junghänel et al., 2021; Deutsches Komitee Katastrophenvorsorge e.V, 2022). This factor in interaction with mobilized sediment and debris led to not yet commonly observed effects in the region. Bridges were clogged which resulted in extending floods to areas hundreds of meters away from the initial riverbed (Dietze and Ozturk, 2021). One major factor that has been in the focus for this effect is deadwood (Dietze and Ozturk, 2021; tagesschau, 2021; ZDF Frontal, 2021). This discourse was also taken up locally. Similar to the discussions observed by Borga et al. (2019) after a flash flood in the Veneto Region of Italy, statements ranged from “exaggerated” nature conservation that did not allow to clear riparian forests from deadwood (ZDF Frontal, 2021) or to calls to remove trees along the streams (SWR Aktuell, 2022) to a not sufficient protection of ecosystems, as, e.g., healthy forest ecosystems allow rainfall to infiltrate and drain better in contrast to monocultures or heavily managed forests (BÜNDNIS 90/DIE GRÜNEN Ahrweiler, 2022). Indeed, this has been acknowledged in alpine areas for long under the concept of protective forests,1 however, has not received much attention in low and medium-ranging mountain areas (Markart et al., 2021; Welle, 2021). Against this background, the question of the adequate management of forests arises, in particular regarding the treatment of deadwood as a key aspect of healthy forest ecosystems, to strengthen its function in providing flood protection, while taking other interests into account.
This article aims to shed light on this issue and to support future developments in the complex forest-flood nexus by elaborating on the potential effects of forest ecosystem health aspects on flood risk. For this purpose, the research aims to address the following question: What are potential trade-offs and synergies between the forest management objectives of forest ecosystem health and of flood risk reduction?
To answer this question, firstly a theoretical background on forest health and flood risk will be provided. This is followed by an investigation of the ways a forest and its condition can influence the development of the flood wave after a heavy precipitation event occurs (Figure 1). Due to the large public attention on the discourse on dead and freshly uprooted wood, we will reflect on this aspect more in detail. Lastly, we will discuss to what extent synergies between the objectives of forest health and of flood risk reduction exist and where likely trade-offs appear.
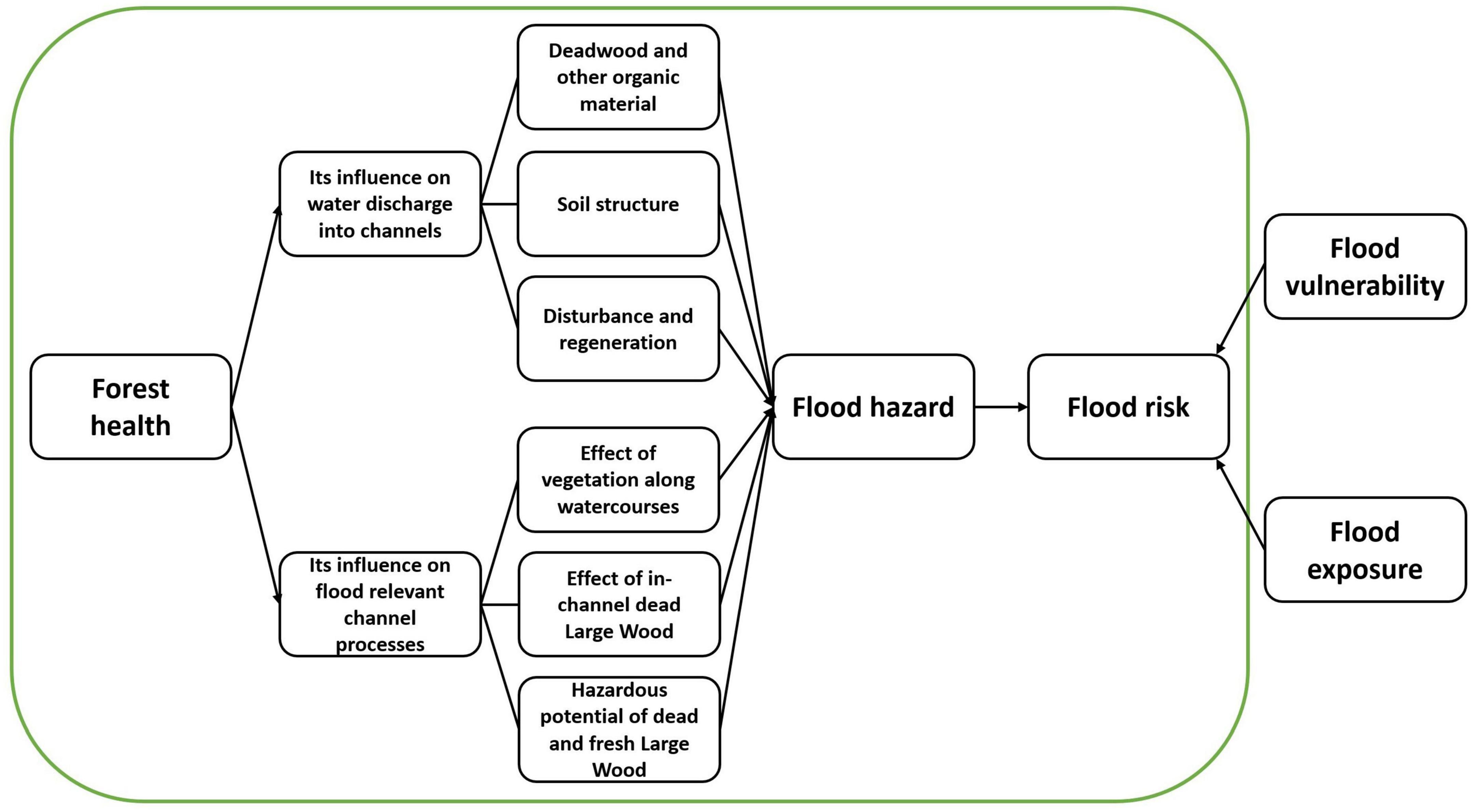
Figure 1. The green frame depicts the thematic foci of the review to identify synergies and trade-offs between the forest management objectives forest health and flood risk reduction. It covers the influences of forest conditions on water discharge into channels and on flood relevant channel processes.
2. Theoretical and analytical background
2.1. Defining forests and forest health
The origin of the term forest health is strongly connected with the concerns over acidic precipitation and the dying-off of forests, the “Waldsterben,” in the 1970s and 1980s in North America and Europe (ICP Forests, 2018; Forest Information System for Europe, 2022). However, there is no consensus on the definition of forest health or of forests to date.
Over time, different definitions of forests have been developed that reflect different management objectives and which shape the development of environmental policies (Chazdon et al., 2016). The most widely applied forest definition is from the Food and Agriculture Organization of the United Nations (FAO) which broadly frames forests as
Land spanning more than 0.5 hectares with trees higher than 5 meters and a canopy cover of more than 10 percent, or trees able to reach these thresholds in situ. It does not include land that is predominantly under agricultural or urban land use (FAO, 2020, p. 10).
Further, it differentiates them into naturally regenerating forests, planted forests, and plantation forests. The FAO’s generic definition is partly heavily criticized for not reflecting on the ecology and complexity of the natural biotope forest (Naturwald Akademie, 2020; Persson, 2020). In this regard, for example, Buettel et al. (2017) propose that deadwood should be an integrated part of the definition of forests.
As there is no agreed-upon definition of forests, also the definition of forest health varies widely. The FAO provides no official definition of forest health. However, its AGROVOC multilingual thesaurus, a tool to classify data homogeneously and facilitate interoperability, defines forest health as “the perceived condition of a forest derived from concerns about such factors as its age, structure, composition, function, vigor, presence of unusual levels of insects or disease, and resilience to disturbance” (FAO, 2022). This highlights the term’s subjectivity and dependence on forest management objectives. Accordingly, definitions of forest health range broadly, from strictly utilitarian to ecological perspectives (Trumbore et al., 2015). For instance, from a utilitarian perspective, factors indicating forest health include diseases, growth rates, and damages but also water quality. Many of these indicators are also included from an ecological perspective. However, it expands to indicators that do not have an immediate use, such as existing deadwood, or which could even be considered unhealthy from a utilitarian perspective, such as the patchiness of forests with different successional stages. For example, patches with a high ratio of old and dying trees would not count as healthy in a strictly utilitarian sense as no immediate use or even loss, e.g., of income would result from it. However, these patches facilitate various ecological processes, such as regeneration or habitat creation on a wider scale (Trumbore et al., 2015). Therefore, according to Trumbore et al. (2015) forest health is characterized by “mosaic(s) of successional patches representing all stages of the natural range of disturbance and recovery” (Trumbore et al., 2015, p. 815). Whereas, the frequency, strength, and spatial extent of disturbances should not exceed the natural variability nor affect the trajectory of recovery at the landscape to regional scale (Trumbore et al., 2015). This characterization of forest health is close to the structures and processes of old-growth forests where in absence of large disturbances, the forest dynamics tend to be small scale and homogenous stand structures rarely reach an extent of more than 0.5–1 ha (Korpel’, 1995). Old-growth forests are characterized by a multi-layered structure with high growing stocks and large deadwood volumes (Burrascano et al., 2013; Commarmot et al., 2013).
For the purpose of the article’s research objective, we adapt a definition of forest health that draws upon the structure of old-growth forests. Therefore, forest health is characterized by (1) mosaics of successional patches that represent all stages of forest dynamics, (2) a multi-layered structure, and (3) high amounts of growing stock and deadwood.
Forest health depends on various factors and is influenced by natural and anthropogenic stressors. Anthropogenic climate change is seen as one of the major challenges for the forestry sector and many different management approaches are discussed and explored to adapt the forest to climate change. These comprise, among others, the use of other or newly bred tree species or more diverse forest structures and deadwood (Bundesministerium für Ernährung und Landwirtschaft, 2021; European Commission, 2021b; Wissenschaftlicher Beirat für Waldpolitik beim Bundesministerium für Ernährung und Landwirtschaft, 2021). However, many of the stressors are related directly to the trade-offs that come along with the forest management objectives. This is particularly the case when the ecosystem management’s target is to maximize the provision of one ecosystem service, such as the provision of timber, which often leads to a considerable decline in the ecosystem’s ability to provide other ecosystem services, such as water retention (Bennett et al., 2009; Cavender-Bares et al., 2015; Watson et al., 2018).
2.2. Ecosystem (dis)services, nature-based solutions, ecosystem-based adaptation, and disaster risk reduction
As touched upon before, intact forest ecosystems provide various services that are beneficial to people (Watson et al., 2018), so-called ecosystem services (ES). The Millennium Ecosystem Assessment Framework categorizes ES into provisioning, regulating, cultural, and supporting services (Millennium Ecosystem Assessment, 2003). These services have the potential to reduce disaster risk by addressing one or several of its three dimensions: hazard, vulnerability, and exposure (Walz et al., 2021a). Their potential in providing a multi-purpose and sustainable approach to Disaster Risk Reduction (DRR) and Climate Change Adaptation (CCA) is widely acknowledged (Sudmeier-Rieux et al., 2021; IPCC, 2022) and also highlighted in the latest Adaptation Strategy of the European Commission (2021a). However, to what extent an ecosystem can support DRR is largely determined by its condition (Walz et al., 2021a).
Approaches that make use of these services to mitigate climate change, adapt to its impacts, and reduce existing disaster risks are clustered under the umbrella term of “Nature-based Solutions” (NbS). Depending on the target of the approach, it is further differentiated into “Ecosystem-based Adaptation” (EbA) and “Ecosystem-based Disaster Risk Reduction” (Eco-DRR). While EbA addresses climate-related hazards with a focus on long-term changes and future uncertainties due to climate change, Eco-DRR addresses all types of natural hazards and, in contrast to EbA, rather focuses on existing risks (European Environment Agency, 2017a; Walz et al., 2021b). In addition, in the sphere of flood risk management terms like “green infrastructure” (European Environment Agency, 2017b) or “bioengineering” (Bischetti et al., 2014) are frequently used.
While functional ecosystems are widely acknowledged as a promising solution to reduce both current and future risk, they may as well contribute to risk by means of “ecosystem disservices” (Sudmeier-Rieux et al., 2021). An example happened during the 2021 Eifel flood with the provision of woody material which contributed to blocking bridges and similar structures staunching the water and leading to backwater effects (Dietze et al., 2022).
Therefore, comprehensive DRR and CCA management schemes need to reflect on potential ecosystem services and disservices (Sudmeier-Rieux et al., 2021). An example of an established Eco-DRR approach to protect against several natural hazards is the concept of protective forests.
2.3. The concept of protective forests
In low-ranging mountains, which usually have absolute heights between 500 and 1,500 m above sea level and overtop their surrounding countryside by only 300–1,000 m, the protective forest concept has not received much attention so far even though low mountain ranges can already cause considerable orographic precipitation (Spektrum Akademischer Verlag, 2001; Welle, 2021).
In alpine areas protective forests have a long tradition to protect against natural hazards. For example, 42% of Switzerland’s area profits from protective forests, whereas one-third of the Swiss protective forests are located in altitudes below 1,000 m (Brändli et al., 2020). Protective forests, as a “Forest-based Solution” (Teich et al., 2021), target to prevent and mitigate natural hazards. They are generally implemented at the slope scale and are comparably inexpensive and feasible to protect against several hazards at the same time (Huber et al., 2015; Teich et al., 2021). Together with spatial planning, they are used to enhance the effectiveness of existing and to reduce the costs of new technical protection structures downstream (Markart et al., 2021; Teich et al., 2021).
Various definitions of protective forests exist, also in the German-speaking alpine area. According to the current Swiss definition from 2013, protective forests protect against the hazard categories of channel process associated with flowing water, avalanche, and rock- and icefall (Losey and Wehrli, 2013; Bundesamt für Umwelt, 2021). However, forests that exclusively reduce runoff but do not mitigate hazardous channel processes, such as debris flow, do not qualify as protection forests (Bundesamt für Umwelt, 2021). The Austrian Forest Act, amended in 2002, distinguishes protection forests into site-protecting forests (Standortschutzwald) (§ 21[1]) focusing on soil eroding processes, object-protecting forests (Objektschutzwald) protecting certain settlements, infrastructures, and other objects (§ 21[2]), and so-called “ban forests” (Bannwald). Here, the economic or other public interest to be protected proves to be more important than the disadvantages associated with the restriction of forest management and therefore declared by official notice a protection forest (§ 27). In comparison to the Swiss definition, the three protection forest types cover a wider range of services, including runoff reduction and also the protection of drinking water (Bundesministerium für Finanzen, 2022). Similarly broad is the Bavarian concept of protective forest which, however, does not distinguish between different types. It was defined as early as 1852 in the first forestry law for Bavaria and has not changed fundamentally until today (Bayerisches Staatsministerium für Ernährung, Landwirtschaft und Forsten, 2016). According to it, protective forests are forests in the high and ridge regions of the Alps and the low mountain ranges, on sites that tend to karstification or are highly susceptible to erosion, and serve to prevent avalanches, rock falls, rockslides, landslides, floods, soil drifts or similar hazards, or to preserve riverbanks (Art. 10 [1]). Further, forests that protect neighboring forest stands from storm damage are also defined as protection forests (Art. 10 [2]) (Bayerische Staatskanzlei, 2022). Also in Bavaria, runoff reduction is an explicitly mentioned task of the protection forest and its increasing importance is highlighted in the context of climate change to reduce flood risk (Bayerisches Staatsministerium für Ernährung, Landwirtschaft und Forsten, 2016).
2.4. Flood risk
Flood risk is, according to the EU directive on the assessment and management of flood risks, “the combination of the probability of a flood event and of the potential adverse consequences for human health, the environment, cultural heritage and economic activity associated with a flood event” (European Parliament and Council of the European Union, 2007, p. 29). The definition is touching upon the general understanding of risk as the product of hazard, exposure, and vulnerability (IPCC, 2014) which is nowadays the agreed understanding in the DRR and CCA communities. Transferring this understanding to the EU directives definition, the flood event is the hazard and the potential adverse consequences for a given subject constitute of its exposure and its vulnerability to being negatively affected by the flood event. Therefore, a hazard alone does not constitute a risk or a disaster (IPCC, 2014). While acknowledging that forests can affect all three factors of risk–e.g., riparian forests could prevent people to settle in flood plains, or could contribute via timber sale to vulnerability reduction of a municipality when used to better equip emergency teams– this review focuses primarily on the flood hazard factor.
3. Methodology
This study is based on a comprehensive review of English and German literature. For this, peer-reviewed journal articles and book chapters were retrieved from the platforms “Google Scholar,” “Web of Science,” and “Scopus.” The initial applied Boolean operators were based on the keywords “forest*” and “flood*” to receive a broad overview. They have then been further concretized by adding keywords reflecting on forest health, i.e., “deadwood,” “drought,” and “health*,” and flood risk aspects, i.e., “erosion,” “runoff,” “driftwood,” “large wood*,” and “debris.” Relevant papers were selected by screening their titles and abstracts. Papers that largely covered the interests of this review during full-text review, e.g., connecting forest management with runoff or with large wood, were inserted in “connectedpapers.com” to identify other related articles and topics that might not have yet been reflected on. The yielded articles were supplemented with relevant gray literature, such as management guidelines and policy documents to capture current developments and practices in the management of forests and flood risk. During the research and writing process, articles have been continuously added to the initial literature bank via cross-references and specific searches for the applied thematic foci. The information for this review has then been extracted from the full articles, chapters, or their sections and summarized, compared, and analyzed according to the presented structure.
4. Results: the influence of the forest on the flood hazard
The severity of the flood hazard depends, besides the duration and intensity of the precipitation event, largely on two major components which determine the flood runoff volume and the development of its peak: (1) The discharge of water from the landscape into channels and (2) the characteristics of the channel and surrounding green and gray infrastructure and their influence on the channel and the transport of water, sediment, and debris.
4.1. The forests’ influence on water discharge into channels
The discharge is to a certain degree influenced by the landscape characteristics. Forests and their soils provide various supporting and regulating ES that influence the potential discharge rate and velocity of surface and sub-surface runoff. In general, forests are characterized by lower run-offs than, for instance, grasslands (e.g., Chen et al., 2021; Scheidl et al., 2021). This is due to their generally higher evapotranspiration [evaporation from soils and water bodies, and vegetation interception and transpiration (Brooks et al., 2013)]) and infiltration rates, higher soil water storage capacities, and higher surface roughness leading to lower runoff velocities (Schüler, 2006; Eisenbies et al., 2007; Hümann et al., 2011; Markart et al., 2021).
While forests may reduce flood peaks for moderate precipitation events, the effect of the forest and its condition decreases with increasing severity (Schüler, 2006; Bathurst et al., 2022; Xiao et al., 2022). These characteristics are, however, strongly influenced by complex interactions of geographical, edaphic, vegetative and climatic factors and the current and historical management (Schüler, 2006; Eisenbies et al., 2007). Optimal are multilayered forests with rich ground vegetation. In well-structured forest stands, up to 6 mm of precipitation in the canopy and depending on the ground vegetation a further 1–4 mm can be retained per rain event (Markart, 2000; Markart et al., 2021). However, it is remarked that this interception capacity is comparatively quickly reached in case of an extreme precipitation event (Markart et al., 2021). While evaporation and transpiration effects during a heavy rain event are neglectable (Markart et al., 2021), the higher evapotranspiration of forests, especially during the growing season in summer, can lead to relatively low antecedent soil moisture contents. In effect, forest soils generally obtain higher degree of available storage volume before a rain event (Brooks et al., 2013; Archer et al., 2016).
To reduce the runoff amount and velocity, the surface roughness of the forest ground plays an essential role. Hereby, the ground vegetation is important, but also structures like dead branches or laying trunks are essential to increase the roughness and eventually help to decrease erosion (Figure 6A; Lachat et al., 2019; Markart et al., 2020).
4.1.1. Deadwood and other organic material
Deadwood and other organic material, such as litter, act as a sponge for water and increase the water absorption capacity. As such, they can create wet microhabitats that obtain an important role for natural regeneration in dry forest types (Frehner et al., 2005). Decaying wood also serves as a seedbed. In some humid mountain forests, more than half of all spruce trees grow on decaying wood (Lachat et al., 2019). Further, deadwood supports soil structuring micro fauna and is therefore an important factor for the development of forest soils characterized by high water infiltration and retention potential (Schüler, 2006; Bundesamt für Naturschutz, 2020).
While high organic contents of forest soils are important for a high water absorption capacity under normal weather conditions, it can lead to stronger water repellency when long dry periods occur. The drying out of organic material leads to hydrophobic conditions increasing the soil water repellency (Mao et al., 2016; Hewelke et al., 2018) and potentially leading to surface runoff (Hümann et al., 2011). Especially dense spruce forests with no ground vegetation (Piceetum nudum) are disadvantageous as they have a pure needle litter which has a strong water-repellent effect (Markart et al., 2020). This repellent “straw roof” (“Strohdach”) effect is also observable in grasslands with a very dense root system, e.g., some Festuca species, leading to a generally higher runoff as compared to forests (Markart et al., 2011). In low-ranging mountains, peat and swamp areas of swamp forests play an important role due to their high water retention capacities. They are able to buffer sudden heavy rainfall after dry periods and mitigate it until their water storage capacity is fully replenished. A hydrophobic phase of organic material, as previously described, is not observed in swamp forests because of permanent moisture penetration (Schüler, 2007).
4.1.2. Soil structure
The forest soil is one of the key factors influencing water retention and stands in strong relation to the forest type and its tree types. While the soil influences the type of forest that is growing on it, it is also shaped by the forest. Besides the provision of organic material, the forest’s root system is a major factor in the development of soils. The tree roots of forests create a complex structure that acts as a drainage system, as water can more easily infiltrate along roots and macro pore structures left by decaying roots (Figure 6B; Wu et al., 2021). In this way, the root system increases preferential flow, partitions, and transports water within catchments (Archer et al., 2016; Luo et al., 2023). The older the forest, the higher the infiltration of water generally is (Lange et al., 2009; Neary et al., 2009; Karl et al., 2012; Archer et al., 2016). Further, forests with deep-rooting tree species, foremost deciduous species, show generally higher water retention rates than shallow-rooted tree species (e.g., Nordmann et al., 2009).
In a study by Archer et al. (2016), the effect of different forest types in the Cairngorm Mountains, Scotland, has been investigated for in situ field-saturated hydraulic conductivity, root fraction, and proportions and connectivity of macropores. The measured values indicate that with increasing tree and forest age the infiltration rates increase due to larger root fractions and higher proportions and connectivity of macropores. Remarkably, an old-growth Caledonian forest had a 7–15 times larger hydraulic conductivity than a 48-year-old pine plantation, while it was evaluated that a 6-year-old pine plantation likely does not allow for heavy precipitation infiltration. The latter is brought into connection with the treatment of the area before the new trees have been planted. The felling of the previous plantation and the preparation of the soil for the new plantation with heavy machinery resulted in amorphous soil structures with a reduced macropore proportion and connectivity (Archer et al., 2016). However, as the 48-year-old plantation has higher values than the young plantation, it is suggested that the pine plantation was able to relatively quickly recover a root system capable of enhancing water infiltration (Archer et al., 2016).
The results of a study by Hümann et al. (2011), however, draw a different picture. They conducted a series of sprinkling experiments on hillslopes in low-mountain ranges of Rhineland-Palatinate (RLP), Germany, in order to investigate the effect of different forest types and soil properties on runoff. In line with Archer et al. (2016), they found that the soils under established forests are porous with relatively high infiltration and water conductivity values. However, in a 40-year-old Douglas fir plantation and in a 30-year-old deciduous afforestation surface runoff has been observed, indicating a limited infiltration. This was associated with the prevalent soil properties. In the Douglas fir stand, the surface runoff has been associated with local soil compaction and dry weather conditions resulting in higher water repellency of the humus layer. In the 30-year-old afforestation, it is related to the compacted soil layer at 20 cm depth that was derived from previous agricultural practices. In effect, the afforestation still reacts similarly to arable land. This indicates that the potential of forests to reduce runoff generation and enhance water retention mainly depends on the physical soil properties and conditions (Lüscher and Zürcher, 2002; Hümann et al., 2011).
The limiting effect of the soil is also acknowledged in the guidance document “Sustainability and Success Control in Protection Forests” [Nachhaltigkeit und Erfolgskontrolle im Schutzwald -NaiS (Frehner et al., 2005)], which describes the official standards and principles of protection forest management in Switzerland. It states that silvicultural management should focus on sites with deep but inhibited permeable soils as here deep-rooting tree species can significantly increase the water storage effect and, therefore, changes in the forest condition have the highest influence. In contrast, the forest condition has only a limited effect on water storage on sites with heavily waterlogged, very shallow, or excessively permeable soils or, on the contrary, on sites with profound and permeable soils that naturally already have a high soil water storage effect (Frehner et al., 2005). For afforestation on soils that are heavily waterlogged, e.g., due to agricultural use, it is suggested to consider the use of special deep-loosening machinery prior to the planting to break up the compacted soil layers to accelerate the establishment of a complex root system (Hümann et al., 2011).
4.1.3. Disturbance and regeneration
As previously touched upon, discharge reduction is impaired by human management practices. This refers to the age and structure of forests, prevalent trees, and existing forest infrastructures. Naturally, in wide parts of central Europe the beech would be dominant (Ellenberg and Leuschner, 2010). The natural forest types were, however, often replaced by plantation forests. This does not solely negatively affect the discharge due to the different afore-described factors but also the resistance of the forest stand to disturbances which is important for the continuous delivery of runoff-reducing services.
In general, forests with a multi-layered, stepped, or routed structure are considered less susceptible to disturbance than single-layered stands (Hanewinkel et al., 2014). Especially, single-layered needle forests own a high risk to be largely destabilized by wind throw or bark beetle infestations resulting in a wide loss of their protective services (Huber et al., 2015; Sebald et al., 2019). In case areas are disturbed by wind throw, lying deadwood initially provides high surface roughness, but as it decomposes, the protective effect may be temporarily reduced if regeneration is slow to emerge (Lachat et al., 2019). According to NaiS natural regeneration should be present on at least 3–6% of the area to guarantee stable protective forest stands (Frehner et al., 2005). Generally, natural regeneration does not present any difficulties in the lower montane, sub-montane, and colline stages. Here beech dominated forests would naturally occur with different ratios of other tree species (Korpel’, 1995; Schwitter et al., 2019). However, the high browsing pressure of ungulate game species is considered a major challenge to natural regeneration (Frehner et al., 2005; Huber et al., 2015; Brändli et al., 2020). Other challenges for natural regeneration pose the quick spreading of blackberry (Rubus silvaticus) on disturbed or cleared sites (Figure 2), and droughts (Frehner et al., 2005).
In case there is missing natural regeneration in undisturbed forest stands, e.g., due to missing light evoked by the dense canopy of one-layered, single-aged forest stands, the cutting out of gaps can help to promote its development. Further, the development of stable trees can be supported by cutting down neighboring trees to give them enough space to develop (Huber et al., 2015). A respective selective thinning approach applied in protective forests in lower altitudes is the “Z-tree care” (Z-Baum Pflege) presented by Schwitter et al. (2019) which targets the support of single trees to transform single-layered forest stands toward multilayered forests.
As drought is one of the main challenges for natural regeneration, it is important to reflect on the risk of excess sun and heat development when opening the forest canopy which could lead to the drying out of the young trees and the organic matter (Schwitter et al., 2019; Ibisch et al., 2021). Similarly, this should be the case when considering the clearing of dead spruce stands triggered by bark beetle infestations. With the dying of the spruces the temperature within the stand increases. It is, however, considerably lower compared to cleared areas which facilitates the upcoming of natural vegetation (Ibisch et al., 2021).
Clearing of dead trees leads to losses of organic matter and reduces the existing structures and thus the surface roughness (Markart et al., 2021). Moreover, for the harvest often large machines are used that compress the soil, as, e.g., mentioned in the study by Hümann et al. (2011). To reduce the impact of machines on forest soils logging trails are established to avoid areal driving. However, the runoff reducing potential of soil in these trails is lost (Figure 3) and therefore they should have minimum density. Further, in the line structures of logging trails and forest roads and respective trenches, it comes to a concentration of water potentially leading to a quick runoff into forest canals (Figure 4; Schüler, 2007).
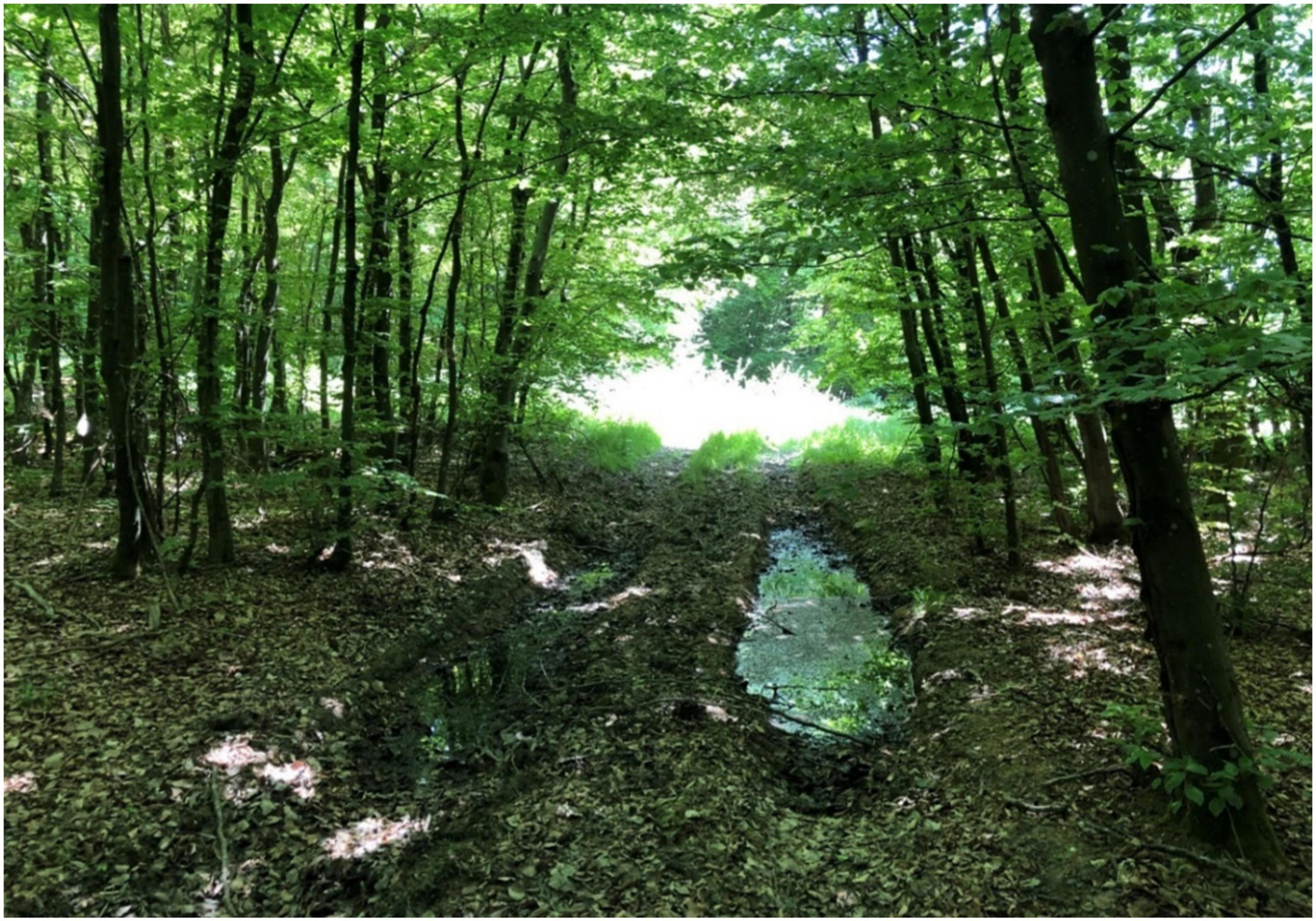
Figure 3. Logging trail with standing water during a hot summer day in 2022 indicating the destroyed water infiltration capacity of the soil due to the compaction of the vehicles, Ahrhütte, Blankenheim, NRW (Fabian Rackelmann).
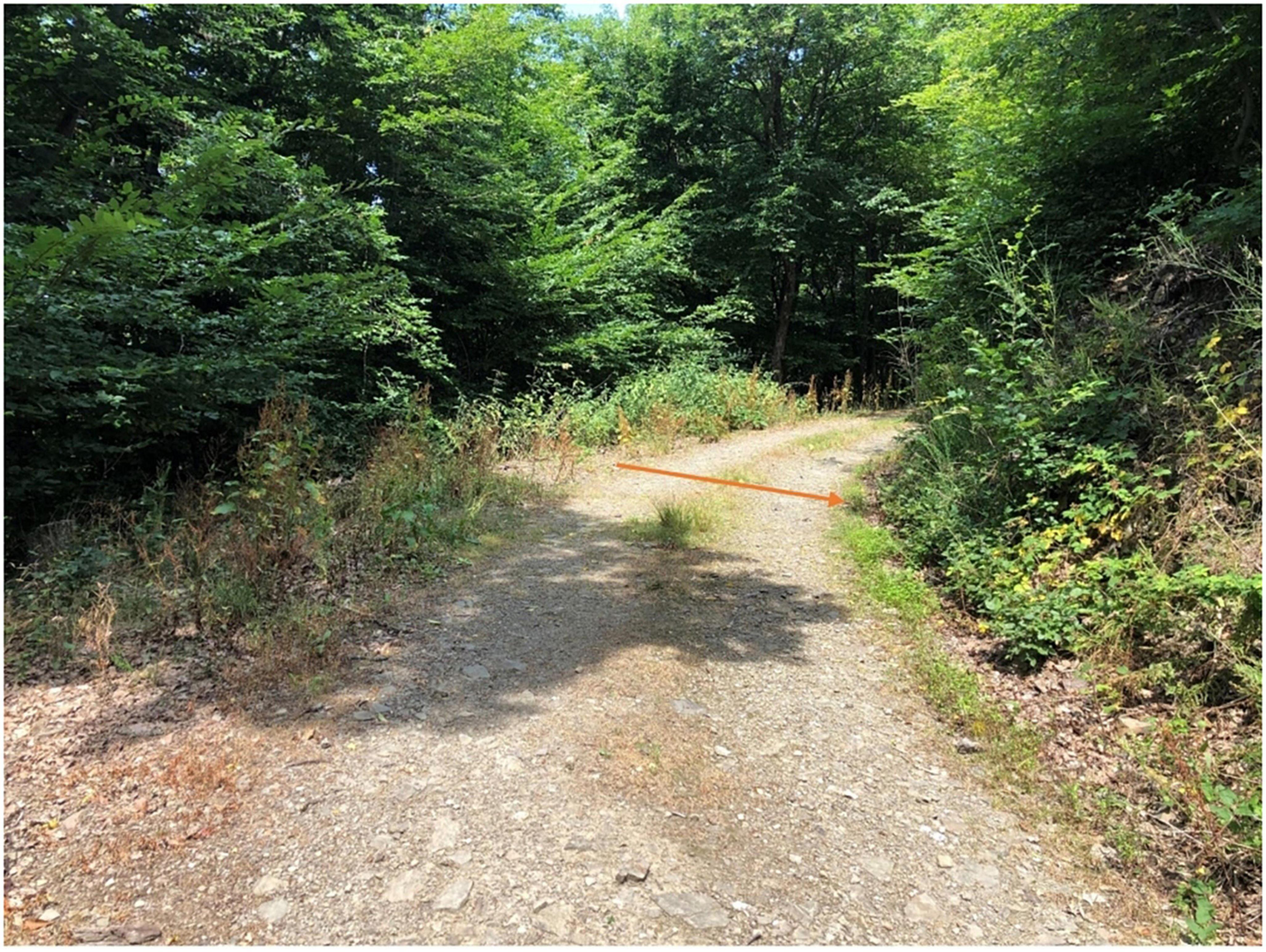
Figure 4. Forest road design tilted toward hillslope favoring the concentration and quick discharge of water, Altenahr, RLP (Fabian Rackelmann).
While the influence of the forest and their conditions in small catchments is largely acknowledged, with increasing catchment size the influence of forest vegetation on flood peaks becomes more contested (Markart et al., 2021; Bathurst et al., 2022). Calder and Aylward (2006) present three possible explanations for it: First, flood peaks from smaller catchments will unlikely come together in the bigger catchment at the same time and therefore will not add up to each other. Second, storms with a spatial scale able to affect a large basin area are potentially also of high severity. As previously described, the higher the severity the smaller the effect of the forest and its condition on the discharge is. And third, the proportional change in water retention by measurements is likely to be higher within a smaller catchment than within a larger catchment.
4.2. The forests’ influence on flood relevant channel processes
The previously described higher roughness of forests also applies to forests in the floodplain and along the channel. Riparian forests increase the flow resistance contributing to the reduction of the flood peak (Bölscher et al., 2010; Reinhardt et al., 2011). They are also a major source of deadwood in channels with a high ecological value (Neuhaus and Mende, 2021) and provide various ES, inter alia, positively affecting channel processes (compare following chapter) (Linstead and Gurnell, 1999; Thomas and Nisbet, 2012). Further, a number of studies have shown the importance of vegetation on streambank stability (Simon and Collison, 2002; Docker and Hubble, 2008; Gasser et al., 2020) and for the reduction of mass movements, such as landslides, by increasing slope stability due to, e.g., root reinforcement and reduced soil’s pore-water pressure (Moos et al., 2016; Vergani et al., 2017; Gasser et al., 2019). Therefore, forests help to reduce the flood peak by decreasing the mobilization of different forms of debris from the riparian stripes, including wood. Further, they also help to retain already mobilized in-channel debris (Figures 5, 6C; Gasser et al., 2019).
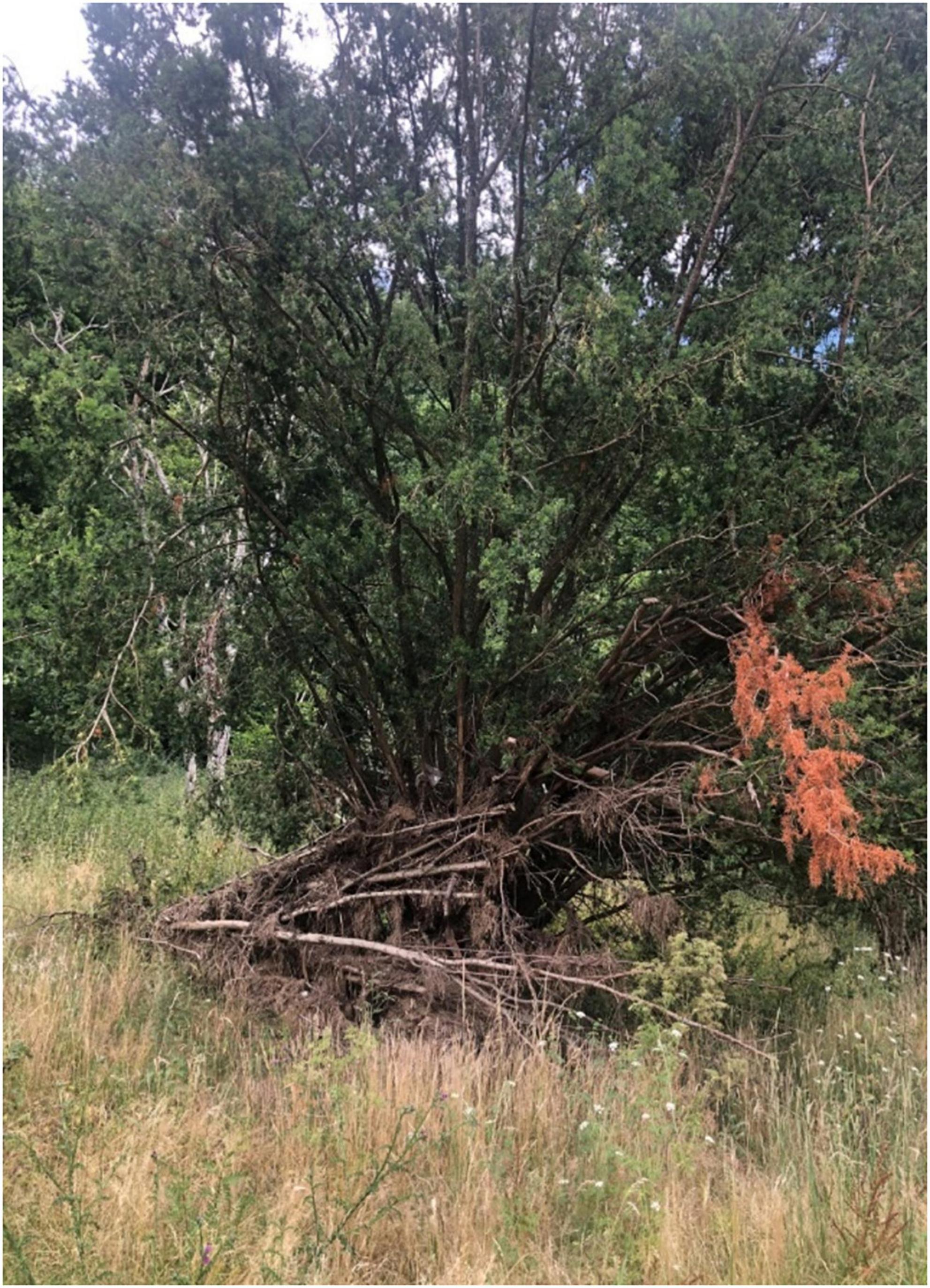
Figure 5. Wood retained by a yew tree (Taxus baccata) close to Brück (Ahr), Altenahr, RLP (Fabian Rackelmann).
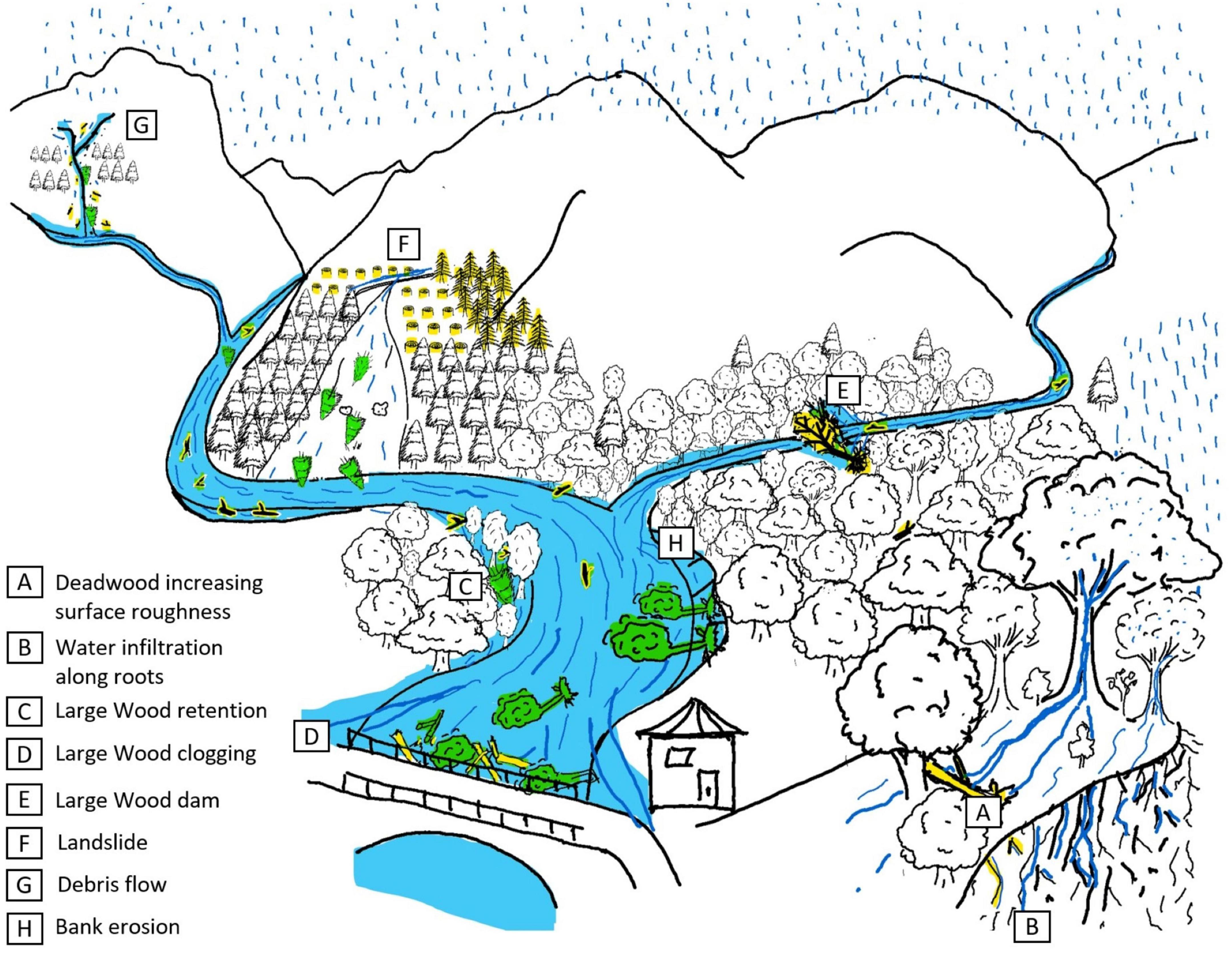
Figure 6. Overview of different forest influences on flood hazard with a particular focus on Large Wood processes. Deadwood is highlighted in yellow. Fresh Large Wood is depicted in green.
However, the services provided by trees and deadwood hold the potential to evolve to flood accelerating disservices during flood events due to mobilized material contributing to blockages of infrastructures, such as bridges.
Dead and fresh woody materials which are located within the floodplain are widely defined as “Large Wood (LW),” “instream wood,” or “(large) woody debris.” The connection with the term “debris” is, however, not frequently used anymore due to its negative connotation (Bundesamt für Umwelt, 2019). The dimensions of LW are generally defined as being at least 1 m in length and 10 cm in diameter. Various LW aspects are increasingly comprehensively researched. The research foci vary, inter alia, from investigating its role for flora and fauna, the origins of the woody pieces that are mobilized during a flood event, to the transport in the watercourse and its interactions with different hydro-geological channel processes, and its potential in contributing to clogging of infrastructures.
As the issue of LW has been very prominent regionally in the discussions on the causes of the Eifel flood disaster of 2021, the following sections will give an overview of what the distribution of dead and fresh LW in previous floods has been and where they originated from to better evaluate the potential risk from different types of LW. Further, the interaction of dead LW with channel processes relevant for the development of floods, will be described. The sections will, moreover, inform on suggested management practices concerning flood risk reduction.
4.2.1. The role of dead and fresh Large Wood during previous flood events
The type of LW, its size and form, the type of vegetation, and whether it is deadwood (woody vegetation, or pieces thereof, that have been dead before the event) or fresh wood (woody vegetation that has been uprooted or broken apart and washed away during the event) determine its potential to contribute to the clogging of bridges and other infrastructure within the floodplain. Long pieces with branches or adjunct root systems pose a higher risk for wood jams than smaller less complex LW forms as further LW and debris can be more easily retained by the more complex structures. Fresh LW is generally longer and more complex than dead LW (Schalko, 2018). For its potential of clogging also the stability of the LW is an important factor. This is determined by the size, especially the diameter, but also by the type and vitality as it determines the stability of the wood. Fresh LW derived from deciduous trees is generally more stable than dead LW originating from coniferous trees and, therefore, potentially owns a larger clogging potential to block bridges (Figure 6D; Rickli et al., 2018; Schalko, 2018).
Besides the type of LW, also its transport form influences its potential to block infrastructure, e.g., single pieces of LW have a lower potential to block bridges than congested LW (Mazzorana et al., 2018). Models to estimate the potential of different types of LW to contribute to clogging are important for an adequate LW management. They have become more complex in recent years. For example, from initially only accounting for straight LW pieces options to including different forms, such as the roots, have been incorporated (Schalko, 2018). For more information on different methods and models to assess LW-related hazards, e.g., Mazzorana et al. (2018) and Friedrich et al. (2022) can be consulted.
Large Wood that has been transported during flood events, has different proportions of deadwood and fresh wood. The proportions are heavily influenced by the management of the watercourse and the floodplain, e.g., the age and management of the forest but will also be influenced by the methodology of the assessment. Even though areas around LW are increasingly investigated, studies on the proportions of dead and fresh LW (Table 1) remain rare. Bänziger (1990) assessed the proportions of dead and fresh LW for the summer 1987 floods in Switzerland. Based on interviews and regional aerial photographs, he estimated that 35% of the total LW has been in-channel deadwood, 19% was derived from eroded alluvial forests, 12% from the streamside vegetation, 17% was mobilized by landslides, and another 17% was derived from lumberyards and timber. Interestingly, the interviewees suggested that the alluvial forests have been responsible for 52% of the total LW mobilization, which was far higher than according to the 19% of his final analysis.
For the 2005 flood in Switzerland, Waldner et al. (2007) assessed the proportion of different LW types with standardized visual assessments of the bark of full piles of LW that either naturally accumulated or artificially were piled in the aftermath of the flood in 4 torrent catchments. The collected data were reevaluated by Rickli et al. (2018) applying some filters to reflect on human influences such as the mechanic piling and cutting of wood (Figure 7). Large variations in the distribution among the different locations were observed. For instance, the fresh LW proportion varied from 35 to 79%. However, as the LW has often completely been debarked during the mobilization process, this may have led to an overestimation of the contribution of deadwood (Waldner et al., 2007; Steeb et al., 2017). Steeb et al. (2017) have modeled, based upon parameters that reflect the recruitment and deposition processes of a catchment, the LW budget during the 2005 flood in Switzerland for four tributaries to the Kander catchment. The proportion of deadwood varied within the 4 rivers from 9.3 to 22% with an average of 12.1%, whereas approx. two-thirds of it, or 8% of the total LW amount, has been located in the channel, and one-third, 4% of the total LW, has been deadwood located in the riparian forests which were then eroded during the flood event.
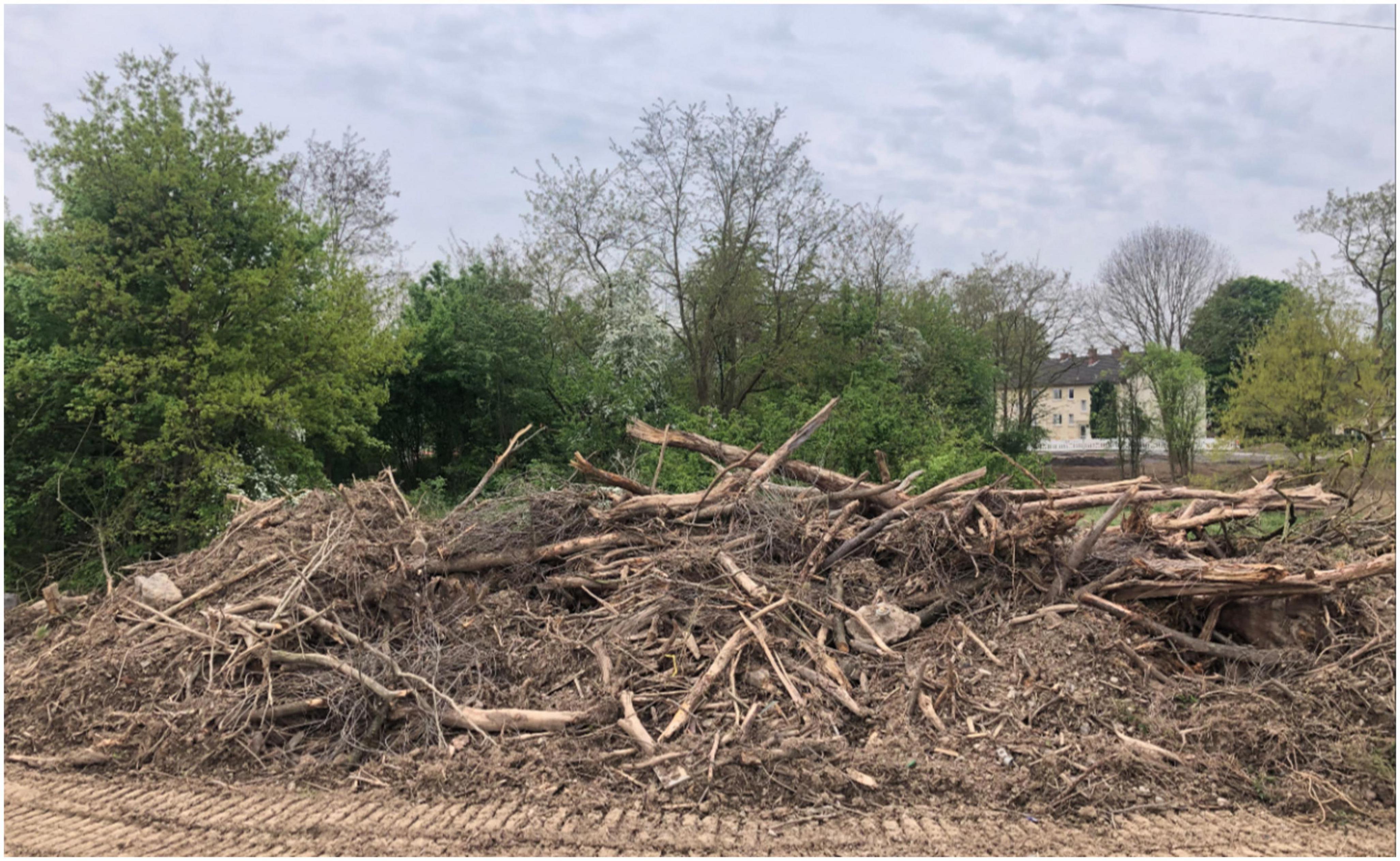
Figure 7. Mechanically pilled Large Wood as one factor exacerbating potential endeavors to determine the source and proportion of Large Wood, Sinzig, RLP (Fabian Rackelmann).
Waldner et al. (2007) particularly paid attention in their investigation on the role of LW to the question of the proportion of storm-damaged wood, since after the event, the assumption was made on various occasions that the large amount of LW had been caused to a substantial extent by storm-damaged areas that had not been cleared. Some of the study regions were severely affected by the storm “Lothar” in 1999. These showed proportions of fresh LW of 53%, while in areas that were barely affected by the storm a fresh LW proportion of 78% was found. In the regions severely affected by the storm, bark beetle traces were found in about 17% of the total volume of LW recorded, in the others it was about 2%. Since bark beetles proliferate especially in spruce stands after storm damage, this is an indication that relatively little LW originated from storm areas. Especially in the areas downstream of the alpine rim lakes, a high proportion of softwood tree species typical of the riparian vegetation of valley rivers was found. This is an indication that a large part of the LW was recruited by lateral erosion during the flood along the larger water courses (Waldner et al., 2007).
As touched upon earlier, the type of LW also depends on the recruitment processes which vary from tree mortality (Figure 6E) and wind-throw to landslides (Figure 6F), debris flows (Figure 6G), and bank erosion (Figure 6H). Depending on the climatic conditions, topography, and geology of the river catchment, and the channel and landscape management the input sources and processes vary. In areas that are not frequently affected by extreme precipitation events, LW recruitment occurs mostly by tree mortality, wind throw, and bank erosion. Conversely, in areas where heavy precipitation events are frequently occurring, LW recruitment is increasingly shaped by mass wasting processes, such as landslides and debris flows (Seo et al., 2010). In mountain catchments, the recruitment processes are quite variable. This is, i.e., related to the varying precipitation distribution which leads to different discharge rates within catchments (Rickli et al., 2018). For example, Rickli et al. (2018) observed no drastic LW transport in four headwater streams during the 2005 flood in Switzerland, which stands in contrast to the high amounts of LW observed in larger rivers further downstream (Steeb et al., 2017; Rickli et al., 2018). Further, the proportions of LW recruitment from the valley bottom by alluvial processes and from the hillslopes by foremost gravitational processes vary considerably, even within small mountain catchments (Comiti et al., 2016a). For the 2005 flood in Switzerland, it has been shown that in the steep headwater torrent catchments few mass wasting events such as landslides and debris flows have been the dominant LW recruitment processes contributing to much of the LW input (Waldner et al., 2007; Rickli et al., 2018). In the lower reaches of the mountains, where catchment areas reached around 100 km2, lateral bank erosion has become the major process for LW recruitment (Steeb et al., 2017). Similar relations have been obtained by other studies, as well (Seo et al., 2010; Lucía et al., 2015). Click or tap here to enter text. However, for example, during the 2011 floods in the Magra River basin in northwestern Italy, severe bank erosion has been already the dominant LW recruitment process for much smaller catchments. For the tributaries and their catchments Gravegnola (34.3 km2) and Pogliaschina (25.1 km2), it has been estimated by Lucía et al. (2015) that 70–80% of the LW were derived from the valley bottom and its flood plains through severe channel widening. The rest of the LW came from a few hillslope processes. While flood plain erosion has been the prevailing process in most of the observed areas, in some upstream sub-basins hillslope processes, foremost landslides, have been majorly responsible for LW recruitment (Lucía et al., 2015).
Based on these observations, it is assumed that with increasing catchment area and decreasing channel slope, the proportion of LW recruited by mass wasting events decreases and the proportion of LW mobilized by erosion increases (Rickli et al., 2018).
As channel widening has been a major contributor to LW recruitment and a direct hazard to infrastructure along the channels during the 2011 flood in Italy, studies by Nardi and Rinaldi (2015), Surian et al. (2016), and Comiti et al. (2016b) helped to better grasp this phenomenon by analyzing geomorphological and hydraulic variables. Despite that, the studies provided a good understanding of the influence of channel slope, channel confinement, upstream sediment supply, and unit stream power, the quantitative prediction of the channel widening during an extreme flood event remains subject to large uncertainties. This in turn limits the capability to precisely evaluate the LW volumes that need to be expected during flood events from vegetated floodplains (Comiti et al., 2016a). However, to estimate LW supply to rivers different models have been developed which follow empirical, deterministic, stochastic, and GIS-based approaches. A review of the different models and their characteristics is provided by Steeb et al. (2023), Preprint who further evaluated the usefulness of two recent GIS-based models for flood hazard assessments.
4.2.2. Suggested multi-goal management of vegetation along watercourses
To meet these uncertainties, water managers and many foresters tend to prevent vegetation growth along the watercourses to avoid the risk of clogging (Gasser et al., 2019). This is also suggested partly by recent practices in which it is recommended to clear the channel along one to one and half times the tree’s height (Pichler and Stöhr, 2018; Markart et al., 2021). This might reduce the input of LW but also reduce the before described benefits of riparian vegetation and thus tends to, inter alia, favor the creation of main LW recruitment processes such as bank erosion (Simon and Collison, 2002; Docker and Hubble, 2008; Gasser et al., 2020) and landslides (Moos et al., 2016; Vergani et al., 2017; Scheidl et al., 2021). According to Markart et al. (2021), the effects of such a clearance management approach became apparent after the 2005 flood in Austria where a lot of debris was mobilized but nearly no LW.
Therefore, considerations between the stabilizing effect of vegetation and the potential recruitment during floods need to be made. To help practitioners in their decision-making, Gasser et al. (2019) provide guidance on this issue for two main LW recruitment processes during heavy precipitation events: Hydraulic bank erosion and landslides. They created respective graphs which evaluate the positive stabilizing effect of the vegetation in high, variable, and low depending on the main characteristics of the slope and channel.
Regarding hydraulic bank erosion, the positive effect of vegetation compared to its potential to act as a LW source tends to decrease with increasing channel width and channel gradient. In areas where the positive effect is variable or low, large trees should be removed and lower-growing vegetation such as different shrubs and grasses should be planted (Gasser et al., 2019).
Regarding landslides, the positive effect of vegetation tends to decrease with increasing slope steepness and soil thickness. Nevertheless, also in areas where the positive effect of vegetation is variable or comparatively low, vegetation is important for overall slope stability. However, here tree heights and densities should be adapted to ensure continuous regeneration to guarantee a continually existing root structure. Further, a mixture of tree species should be adapted to initiate higher root reinforcement (Gasser et al., 2019).
4.2.3. The effect of in-channel dead Large Wood on watercourse processes
The previous section has shown that the risk deriving from dead in-channel LW is likely to be comparatively low. Nevertheless, its widespread removal from the watercourses is widely practiced to reduce flood risk (Thomas and Nisbet, 2012). As with the removal of living vegetation this, however, can have detrimental effects on the development of the flood peak. This section will take a closer look at the ways dead in-channel LW interacts with the watercourse processes and its effect on the flood hazard.
4.2.3.1. Presence, storage, and transport of in-channel dead Large Wood
The presence, storage, and mobilization of in-channel dead LW depend on the characteristics of the LW, and of the stream and its hydro-geomorphic conditions and management. Generally, the lower the channel widths and depths are, the higher the storage of LW in the channel and its interaction with the channel bed and bank. LW pieces that are longer than the channel width are considered largely stable (Figure 8; Lienkaemper and Swanson, 1987; Ruiz-Villanueva and Stoffel, 2017). In this regard, Gurnell et al. (2002) categorize channels in small, medium, and large. Small channels have widths smaller than most of the LW lengths. Medium channels are wider than most of the LW pieces and large channels have widths larger than all of the supplied LW pieces.
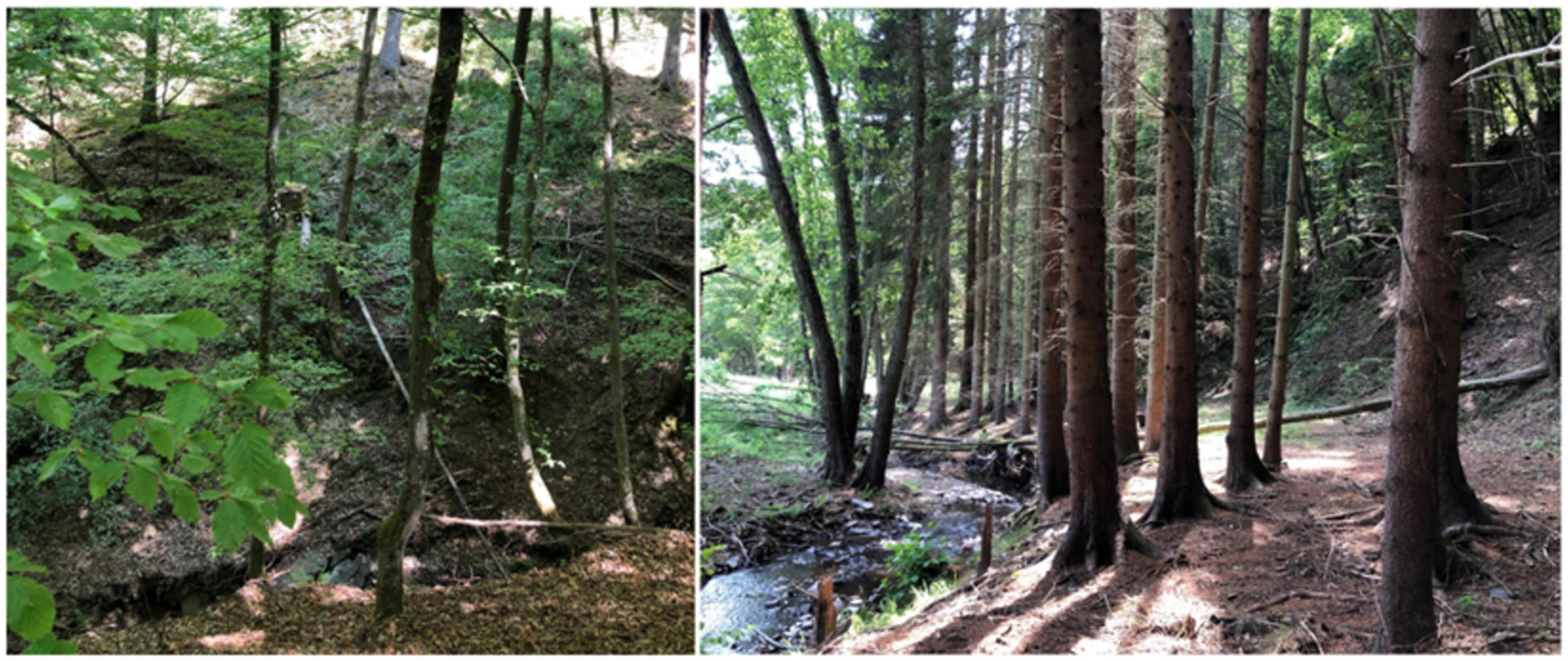
Figure 8. Dead Large Wood with very low potential of being mobilized during a flood event as their lengths are greater than the stream widths. (Left) Stream Aulbach close to Ahrhütte, Blankenheim, NRW. (Right) Stream Vischelbach close to Kreuzberg, RLP (Fabian Rackelmann).
Further, the stream flow power influences the stream’s capacity to transport LW. In small headwater catchments, the mobilized LW generally deposits not far away from its source areas due to narrower valley channels and lower stream power compared to larger rivers. Even during extreme flood events in a larger catchment, the LW in the smaller contributing headwater catchments remains often stable as the precipitation and discharge peaks locally are generally not as severe as compared to the larger catchment (Seo et al., 2010; Rickli et al., 2018). An exception is debris flows as they often transport large amounts of LW, boulders, and sediment into headwater streams and their adjunct watercourses, where, however, the LW recruited generally breaks into smaller pieces (Seo et al., 2010; Steeb et al., 2017). As with the recruitment of fresh LW, in larger streams fluvial processes play the major role in the transportation of LW downstream or on the floodplains (Figure 9). Here, LW has less influence on the channel characteristics than in small streams (Lienkaemper and Swanson, 1987; Seo et al., 2010).
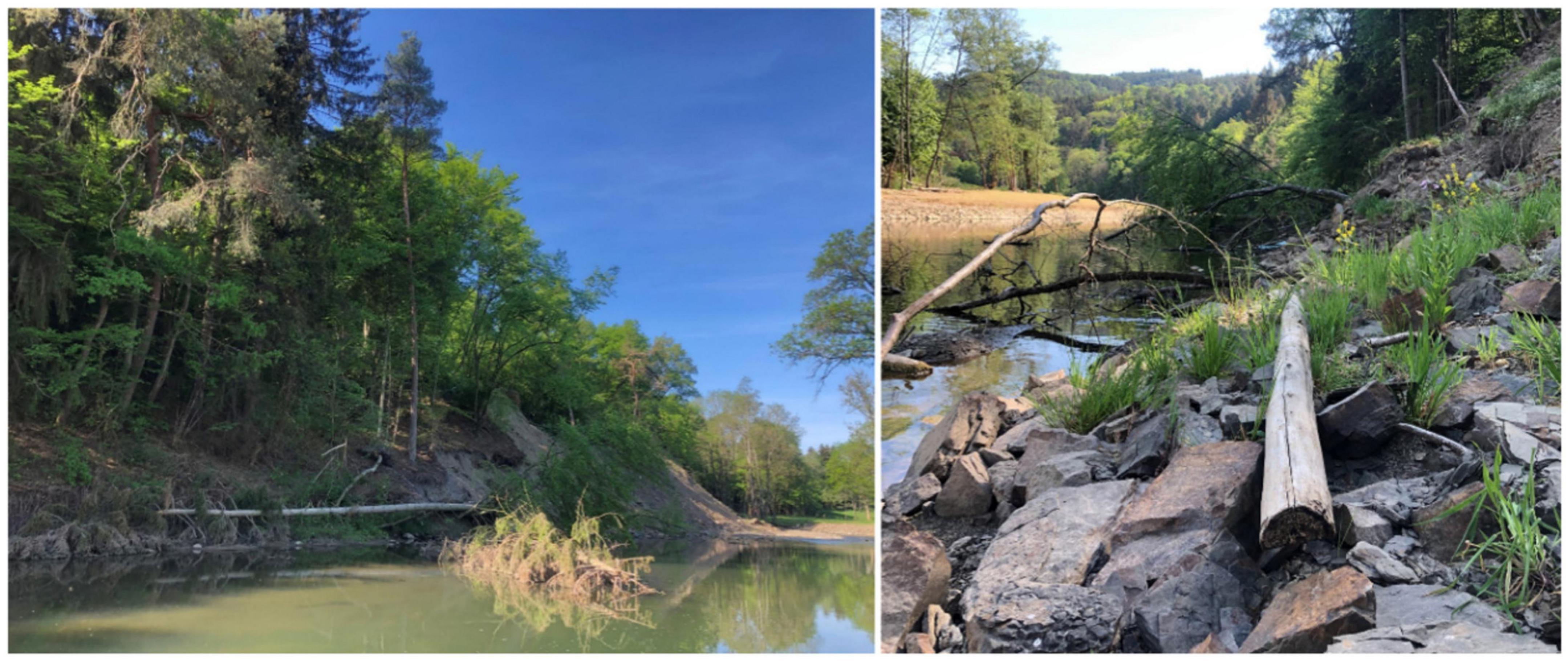
Figure 9. Large Wood with a high potential of being mobilized during a flood event. River Ahr close to Müsch, RLP (Fabian Rackelmann).
The dominant watercourse morphologies in mountainous regions are step-pool channels (Chin, 1989). In mountain streams, the natural accumulation form of LW are dams, also called logjams. Logjams are frequently defined as more than two LW pieces that exert combined effects on hydraulics and sediment storage of a channel (Rickli et al., 2018; Wohl and Scamardo, 2020). A simple and widely applied categorization of LW accumulations is the one from Gregory et al. (1985) in “partial dams,” “complete dams” and “active dams,” which have been further elaborated on by Linstead and Gurnell (1999). Partial dams present only an incomplete barrier to the water flow as it does not cover the full width of the channel. Complete dams extend across the full channel width, however, consisting of a rather loose structure that allows the water flow to continue and not to influence the water profile at regular flows. Active dams also extend across the complete width, however, induce a step in the water profile by (partially) blocking the water flow. Active dams need the longest time in their generation but are also the most stable type, persisting often many years at the same location (Linstead and Gurnell, 1999). The porosity of the LW dams depends on the structure and size of the LW and the accumulating material. A matrix out of small and big wood pieces will create denser dams than big wood pieces alone. The porosity of the dams also depends on the seasons, being the lowest in autumn when leaf litter in the stream accumulates behind the dam (Thomas and Nisbet, 2012). Over time, the characteristics and hydro-geomorphic effect of stable LW dams vary by differing amounts of wood pieces and sediment trapped by the key pieces of the LW dam (Wohl and Iskin, 2022). The size of the dams increases downstream, whereas the frequency of LW accumulations decreases (Swanson et al., 1982; Wohl and Scamardo, 2020).
Under largely unmanaged conditions it was found for British headwater rivers that approximately every 7–10 times of the channel width LW dams occur naturally whereas the forms and characteristics of dams vary (Linstead and Gurnell, 1999). Most of the LW dams are relatively transient. Only a few dams persist for several years and remain at the same site, however, the density over time remains relatively equal under unmanaged conditions. Despite the relative transience of LW dams, persistent effects in channel morphology and backwater storage are observed (Wohl and Scamardo, 2020; Wohl and Iskin, 2022).
4.2.3.2. The effect of in-channel Large Wood on morphology and water retention
Several studies have highlighted the role of LW in dissipating excess energy that otherwise would contribute to slope erosion (Chin, 1989; Chin and Wohl, 2005). This is, inter alia, because LW accumulations cause an increase in the flow resistance, its effect, however, decreases with increasing water discharge rates (Gregory et al., 1985; Linstead and Gurnell, 1999). They exhibit a higher dispersive fraction than streams without LW and it has been shown that the flow resistance is far greater in step-pool channels with LW than without. This, however, is largely influenced by the location of the LW in the watercourse. LW located close to the lip of the step rises the step height and increases the flow resistance more than compared to LW which is located in the pool of the channel. In this way, LW is an essential factor for the stabilization of these channels (Abbe and Montgomery, 1996; Curran and Wohl, 2003; Chin and Wohl, 2005; Wilcox and Wohl, 2006).
Further, LW creates greater variability in flow depth and flow velocity. Different types of pools are created in the channel which plays an integral role in the retention of water and sediments and provides habitats for fauna and flora (Linstead and Gurnell, 1999; Thomas and Nisbet, 2012). In streams in which LW is unmanaged pools can occur approximately every two channel-widths (Linstead and Gurnell, 1999).
When water is facing LW dams (Figure 6D), the flow is concentrated when it flows over, under, or through the dam’s matrix where it gets concentrated leading to higher velocities after the dam (Thomas and Nisbet, 2012). During high flows, dammed pools can act as locations of flow avulsion. Further, if active dams persist for long, they can also lead to a change in the position of the channel itself if the bank is erodible (Linstead and Gurnell, 1999; Thomas and Nisbet, 2012; Wohl, 2013). These effects lead to an increased capacity to hold back water in the channel and in the floodplain, which decreases the total runoff and stretches the runoff time. Thomas and Nisbet (2012) have reintroduced LW in the tributaries of the Welsh river Fenni and have modeled based on the observed changes that each dam has the potential to delay the flood peak by 2–3 min. This effect might be low for a single dam, however, on a catchment scale it may be considerably high (Linstead and Gurnell, 1999; Thomas and Nisbet, 2012). Linstead and Gurnell (1999) argued that this might also help to desynchronize the runoff of the different tributaries leading to a decrease in the flood peak of the downstream river. This, however, could not be proven by Thomas and Nisbet (2012).
Moreover, particularly active dams are important for trapping sediment and LW and keeping it within the headwater system (Linstead and Gurnell, 1999). In turn, the removal of LW dams would trigger the downstream mobilization of sediment and the remaining LW, incise the channel bed, and reduce habitat diversity (Linstead and Gurnell, 1999). In this regard, the downstream accumulation of LW can help restore previously eroded and incised channels by forming a renewed step and therefore contributing to the momentary channel-hillslope stability reducing the probability of landslides (Golly et al., 2017).
4.2.3.3. The influence of water and forest management on Large Wood and suggested approaches for Large Wood management
As previously described, the presence and mobilization of in-channel LW are dependent on the characteristics of the stream which is strongly influenced by existing gray infrastructures, such as bridges, and green infrastructures, such as living vegetation and existing LW dams. These are largely shaped by river and forest management (Seo et al., 2010; Lucía et al., 2015; Comiti et al., 2016a).
Forest management substantially affects the input of the species and sizes of the wood. The removal of trees from the riparian forest impairs the development of stable dams as they generally form the basis of them (Linstead and Gurnell, 1999). Along afforested riverbanks, LW densities (number of pieces per km of shoreline) are much higher than in open landscapes such as fields (Angradi et al., 2004). Also, river management largely affects the presence of LW. It often interferes with the flow regime and targets an increased flow conveyance. This includes the direct removal of LW but also targets to reduce the LW retention capacity of the channel. At stabilized riverbanks considerably less LW is found than on natural riverbanks consisting of sand or silt. Also, the normally high effect of riparian forests on LW density is diminished by stabilized riverbanks (Angradi et al., 2004). Combined this leads to a reduced possibility that stable LW dams can form which in turn means that potentially more LW and sediment are transported downstream and the blockage of infrastructure becomes more likely (Linstead and Gurnell, 1999). Therefore, Linstead and Gurnell (1999) come to the same conclusion as Benke et al. (1985):
Although there are certain situations that may require wood removal to eliminate stream blockage, the wisest management practice is usually no “management” other than protection of the adjacent flood plain (Benke et al., 1985, p. 12).
However, while indiscriminate removal of LW should be avoided, a (partly) removal of LW should be considered if the channel conveyance receives a high priority, or when extensive amounts of LW have entered the channel, e.g., by forest operations. Excess input of small materials into rivers might lead to fully closing the matrix of dams and thus hinder the migration of fish and other organisms. If the riparian forest is not managed, this problem is unlikely to occur (Linstead and Gurnell, 1999). Further, as it is with living vegetation, dead LW should be removed when less stable LW accumulations are close to infrastructure susceptible to clogging. However, in case the estimated flooding is of a lower extent, the partial removal of looser LW should be considered to reduce negative ecological and morphological impacts, such as the incising of riverbanks. The most stable LW pieces should remain at their place, therefore primarily LW should be removed that is not longer than the channel width, unstable and not fixed within the stream bed on one or two ends, by either being buried, anchored, or braced by riparian trees, boulders, bedrock outcrops, or by LW that does not have just listed characteristics. The removal should only happen at a certain length of the watercourse and not include the removal of all dams in the river, as especially active dams hold back LW in the upper stream system (Gurnell et al., 1995; Linstead and Gurnell, 1999). Besides the removal of LW, the systematic management of LW should also be supplemented by engineering approaches, such as bridge design and retention structures, to protect areas susceptible to LW (Schmocker and Weitbrecht, 2013; Mazzorana et al., 2018).
The various benefits of LW are increasingly acknowledged. However, often the watercourses and the floodplains are heavily managed, especially in Europe, and managed forested areas along rivers can often only insufficiently provide LW. Therefore, the reintroduction of LW into streams is increasingly considered for river restorations after years of active removal of LW from streams (Neuhaus and Mende, 2021; Swanson et al., 2021; Wohl and Iskin, 2022) and even described as state of the art for river restoration projects in Switzerland (Neuhaus and Mende, 2021). A further approach to river restoration is covered under the rewilding approach which for example includes the support of beaver populations. A study from Bavaria indicates that their dams have a similar effects on the flood hazard as active dams (Neumayer et al., 2020). Further insights on the role of beavers on, for example, river morphology are presented by, e.g., Levine and Meyer (2019) and Larsen et al. (2021). Besides beavers also the management of riparian forests influences the LW supply into streams (Linstead and Gurnell, 1999; Vaz et al., 2011; Neuhaus and Mende, 2021). Missing forests and the clearance of trees along the stream have the consequence that LW dams are more difficult to sustain. Therefore, forest management should include buffer strips along the watercourses including trees of different ages and sizes that can act as LW sources, where the risk for infrastructures is evaluated as low. The buffer stripe should optimally be 20 meters, as this reflects on the natural height of mature native tree species in forests, which defines the range in which wood can reach the watercourse, e.g., through wind throw. If a riparian forest only consists of a single-age structure that is not able to act as a source of LW the input will consequently be low and a (continuous) supply of LW to the watercourse might be necessary to sustain LW dams. Therefore, the development of a more structurally diverse forest should be supported, if the development of LW dams is envisaged (Gurnell et al., 1995; Linstead and Gurnell, 1999).
5. Discussion: synergies and trade-offs between forest ecosystem health and flood risk reduction
In general, the objectives of forest ecosystem health and flood risk reduction are largely synergetic. A forest management that is focused on forest health by developing or allowing the development of successional patches and a multilayered structure and increasing growing and deadwood volumes stands in line with forest structures that support water retention in the landscape. High volumes of growing stock, with a multilayered structure, and deadwood support soil structures that have high water holding and infiltration rates. Further, it increases surface roughness reducing runoff. The presence of small successional patches enhances a continuous regeneration which in combination with the lower risk of healthy forests for large-scale disturbances from, e.g., wind throw or bark beetle calamities supports the constant provision of its flood hazard-reducing services. Existing forest disturbances reduce the forest’s ability to retain water. In accordance with the forest health objective for high deadwood volumes, the disturbed areas, however, should not be cleared as this would go along with a reduced soil roughness and, depending on the clearing operation, with a high and long-lasting loss of the soil’s capacity to retain water due to mechanical compaction. Further, deadwood supports the upcoming of new seedlings by acting as a seedbed with high water storage which gains special relevance on soils with low soil thickness or soils with a low water holding capacity. Further, disturbed forests with standing deadwood have lower temperatures compared to cleared areas which are also important for the upcoming and survival of new tree seedlings. Its importance is likely to increase with progressing global warming. Therefore, the restructuring of monocultures and single layered forest stands toward the objectives of forest health benefits in general also the reduction of the flood hazard. Due to its short-term effects, e.g., by not clearing dead spruce stands, and its long term effects by developing diverse and resilient forest structures, the objectives of forest health contribute to both, Eco-DRR and EbA.
Further, removing or adapting man-made infrastructures in the forest, like logging trails, will reduce the water runoff and benefit both the vitality of drought-affected forest stands and the reduction of the flood hazard. Moreover, these infrastructures might trigger landslides during heavy precipitation events that potentially mobilize large amounts of LW into channels (Figure 6E; Seo et al., 2010).
Along water streams, the relationship between forest health and flood risk is more complex. While the afore-discussed benefits of healthy forest structures also hold true along the streams, the close interactions with the water dynamics lead to more aspects that need to be accounted for in the management of riparian forests. This is particularly the case due to the ambivalent role of existing in-channel LW and vegetation that could become LW during a flood event. As described, dead in-channel LW and vegetation in the floodplain or on aligning slopes have the potential to deliver valuable services for the reduction of flood hazards. The objectives of forest health stand in line with it as the diverse structures and high volumes of deadwood and growing stock increase the flow resistance. Further, the multilayered structures and different successional patches enhance the root penetration which positively affects streambank (Simon and Collison, 2002; Docker and Hubble, 2008; Gasser et al., 2020) and slope stability (Moos et al., 2016; Vergani et al., 2017; Gasser et al., 2019). Moreover, these structures, translating in more and thicker stems, are likely to better retain already mobilized in-channel debris and to better keep deadwood than forest stands with fewer volumes of growing stock and less complex structures on the ground that could benefit the locking.
Further, a forest management that follows the objectives of forest health benefits the continuous LW supply. The different layers and successional patches support the regular supply of LW with different size distributions. In contrast to timber-focused forest management, this management would also facilitate the supply of long and stable LW, such as full tree stems. These can act especially in small and medium channels as key pieces for the development of persistent LW dams with their beneficial effects on water retention.
However, the in-channel LW and riparian vegetation might exacerbate the flood hazard in case they are mobilized during the flood event. Therefore, if located close to infrastructure susceptible to LW, the forest management objective of forest health might collide with the interest in flood risk reduction. Here, however, deadwood plays only a subordinate role for hazard creation. This is, as described, related to its lower proportions and its shorter and less stable characteristics compared to fresh LW (Rickli et al., 2018; Schalko, 2018). Higher volumes of deadwood in healthy forests might increase the proportions but as the overall contribution of deadwood from riparian forests is low (Steeb et al., 2017), this effect is likely to be negligible.
Another concern that potentially affects the management toward forest health objectives, is that disturbed forest areas could largely contribute to the mobilization of LW and thus should be cleared, as it has been discussed for example for the 2005 floods in Switzerland over wind throw forests. While the investigations of Waldner et al. (2007) in this regard found that storm-damaged wood was present, its overall contribution to the LW amounts was relatively small. The LW amounts were shaped majorly by the downstream erosion of deciduous trees. Similarly, it is likely the case for forest areas affected by bark beetle calamities and wildfires. Bark beetle calamities, which are widely present in many German low mountain ranges, result in large areas with standing deadwood. Here the potential to exacerbate the clogging is likely even lower compared to storm-hit areas, as once the dead tree stems fall, they will show lower stabilities and sizes due to different decomposition processes. Likewise, this is potentially the case for forest areas that were affected by wildfires. It was found that fire-affected LW is less complex (Vaz et al., 2011) and, thus, has a lower clogging potential. Different forest disturbances, such as wildfires,2 will increasingly come along with climate change and therefore also influence LW dynamics. This will further exacerbate the predictability of how and from where most of the LW is mobilized (Swanson et al., 2021). However, so far there are no indications that deadwood from disturbed areas, and consequently the objectives of forest health, contributes over proportionally to the overall LW volumes mobilized during a flood event and, therefore, should be cleared. On the contrary, a clearance could lead to higher erosion rates and trigger mass movements potentially transporting LW.
The major risk of healthy forests in areas that are susceptible to LW is therefore not stemming from their contribution to deadwood supply but from the mobilization of high volumes of uprooted vegetation during a flood event, especially in medium to large channels. Here particularly late successional patches with outgrown trees pose a risk due to their high potential in contributing to clogging of bridges. As such, in line with the guiding framework by Gasser et al. (2019), this work suggests to remove big trees with a high LW potential in riparian areas close to susceptible infrastructure. While Gasser et al. (2019) provide guidance under which conditions of the channel and adjunct slope the positive services of forests could be outweighed by the potential of being transformed to hazardous LW and suggestions on how vegetation should be (re)structured, no information on deadwood management within these areas is provided. The findings of this work suggest that deadwood from riparian forests play only a negligible role in the severity of the flood hazard. Therefore, in line with the objectives of forest health, we argue that deadwood in riparian forests and adjunct slopes should remain. The existence of high deadwood volumes might also partly compensate for the preventive removal of big trees to reduce the LW potential as suggested by Gasser et al. (2019). The preventive removal will negatively affect the inner forest climate and therefore the success of natural regeneration that is essential for continuous root penetration. The existence of deadwood will support the upcoming of natural regeneration due to its water-storing properties. Further, during flood events, it can provide higher flow resistance and direct the water into the forest stand. In case the risk of large deadwood sizes is considered too high due to potential high erosion rates or the probability of landslides engineering approaches should be considered, especially, downstream of areas that are not accessible for LW removal, e.g., due to difficult terrain or environmental regulations. Further, the concision of deadwood might be an approach to decrease the risk of clogging, as already suggested by Bänziger (1990) three decades ago, while maintaining most of its beneficial properties inside the riparian forest.
6. Conclusion
This study has elaborated on the relations between the two forest management objectives of forest health and flood risk reduction. For this, we have provided a theoretical background on related concepts and have presented a working-definition for forest health. The different aspects of how a forest potentially can affect the flood hazard have been reviewed. This included the forests’ influences (1) on the discharge of water from the landscape into channels, as well as (2) its influences on the characteristics of the channel and its riparian area and their respective influence on the transport of water, sediment, and debris. The review’s results and discussion suggest that the two objectives are largely synergetic. However, in direct proximity to watercourses trade-offs might occur. This is especially due to the ambivalent relation of living vegetation and deadwood to flood hazard. In places where no susceptible infrastructures to clogging exist, diversely structured vegetation and deadwood should be supported due to its beneficial effects on water retention and channel characteristics. Here, the objectives of forest health and flood risk reduction are aligned. In places where susceptible infrastructures exist, trade-offs between the two objectives arise. Here the potential of freshly uprooted vegetation to cause damages should be reduced while maintaining the vegetation’s supportive characteristics, e.g., concerning bank and slope stability. For this, primarily the adjustment of existing vegetation structures toward a better root penetration should be targeted while reducing the prevalence of high growing stocks that could result in large and stable LW sizes. Where the risk of clogging is perceived as too high, also the selective removal or shortening of dead in-channel wood can be considered. However, based on the literature review the risk deriving from dead LW is evaluated as comparability low. This is related to its generally lower proportions and its smaller and less stable characteristics compared to freshly uprooted vegetation. In this regard more research needs to be conducted. More insights on the proportions and sources of dead and fresh LW after flood events are necessary to better evaluate the respective roles and, therefore, to deliver a better foundation for the connections and suggestions made in this review. Furthermore, more studies are needed focusing on low-ranging mountains as well as floods spanning several orders of magnitudes (ranging up to 500–1,000 years events like the flood in the Ahr valley). Lastly, we want to acknowledge that forest health and flood risk are only two of the various objectives shaping forest management. Therefore, the findings of this review should be seen as a contribution toward a comprehensive forest management taking different interests into account.
Author contributions
FR, ZS, and RB contributed to the conception and design of the study. FR wrote the first draft of the manuscript. All authors contributed to the manuscript revision and read and approved the submitted version.
Funding
This work was supported by the Open Access Publication Fund of the University of Bonn. Further, FR has received funding through the United Nations University’s Climate Resilience Initiative Project, financed by the German Federal Ministry of Education and Research (BMBF).
Conflict of interest
The authors declare that the research was conducted in the absence of any commercial or financial relationships that could be construed as a potential conflict of interest.
Publisher’s note
All claims expressed in this article are solely those of the authors and do not necessarily represent those of their affiliated organizations, or those of the publisher, the editors and the reviewers. Any product that may be evaluated in this article, or claim that may be made by its manufacturer, is not guaranteed or endorsed by the publisher.
Abbreviations
CCA, climate change adaptation; DRR, disaster risk reduction; EbA, ecosystem-based adaptation; Eco-DRR, ecosystem-based disaster risk reduction; ES, ecosystem service; LW, Large Wood.
Footnotes
- ^ Often also described as “protection forests.” However, in the literature increasingly termed as “protective forests” to avoid confusion with forest protection (Dorren and Moos, 2022). Various definitions exist which will be elaborated on in Section “2.3. The concept of protective forests.”
- ^ The aspect of forest fires and burned LW and its ES was investigated by, e.g., Vaz et al. (2021) and Iskin and Wohl (2021), however, without linking it to flood hazard.
References
Abbe, T. B., and Montgomery, D. R. (1996). Large wooody debris jams, channel hydraulics and habitat formation in large rivers. Regul. Rivers Res. Mgmt. 12, 201–221.
Angradi, T. R., Schweiger, E. W., Bolgrien, D. W., Ismert, P., and Selle, T. (2004). Bank stabilization, riparian land use and the distribution of large woody debris in a regulated reach of the upper Missouri River, North Dakota, USA. River Res. Applic. 20, 829–846. doi: 10.1002/rra.797
Archer, N. A. L., Otten, W., Schmidt, S., Bengough, A. G., Shah, N., and Bonell, M. (2016). Rainfall infiltration and soil hydrological characteristics below ancient forest, planted forest and grassland in a temperate northern climate. Ecohydrology 9, 585–600. doi: 10.1002/eco.1658
Bathurst, J. C., Hagon, H., Hambly Barton, F., Iroumé, A., Kilbride, A., and Kilsby, C. (2022). Partial afforestation has uncertain effect on flood frequency and peak discharge at large catchment scales (100–1000 km 2), south-central Chile. Hydrol. Process. 36:14585. doi: 10.1002/hyp.14585
Bayerisches Staatsministerium für Ernährung, Landwirtschaft und Forsten (2016). Der Berg- und Schutzwald in den Bayerischen Alpen. Moreno Valley, CA: Bayerisches Staatsministerium für Ernährung.
Benke, A. C., Henry, R. L., Gillespie, D. M., and Hunter, R. J. (1985). Importance of Snag Habitat for Animal Production in Southeastern Streams. Fisheries 10, 8–13.
Bennett, E. M., Peterson, G. D., and Gordon, L. J. (2009). Understanding relationships among multiple ecosystem services. Ecol. Lett. 12, 1394–1404. doi: 10.1111/j.1461-0248.2009.01387.x
Bischetti, G. B., Di Fi Dio, M., and Florineth, F. (2014). On the Origin of Soil Bioengineering. Landsc. Res. 39, 583–595. doi: 10.1080/01426397.2012.730139
Bölscher, J., Schulte, A., and Huppmann, O. (2010). “Long-term flow field monitoring at the Upper Rhine floodplains,” in Proceedings of the international conference on fluvial hydraulics, Braunschweig.
Borga, M., Comiti, F., Ruin, I., and Marra, F. (2019). Forensic analysis of flash flood response. WIREs Water 6:1338. doi: 10.1002/wat2.1338
Brändli, U.-B., Abegg, M., and Allgaier Leuch, B. (2020). Schweizerisches Landesforstinventar. Ergebnisse der vierten Erhebung 2009–2017. Birmensdorf, Eidgenössische Forschungsanstalt für Wald, Schnee und Landschaft. Bern: Bundesamt für Umwelt.
Brooks, K. N., Ffolliott, P. F., and Magner, J. A. (eds) (2013). Hydrology and the management of watersheds. Ames: Wiley-Blackwell.
Buettel, J. C., Ondei, S., and Brook, B. W. (2017). Missing the wood for the trees? New ideas on defining forests and forest degradation. ReEco 1, 15–24. doi: 10.3897/rethinkingecology.1.13296
Bundesamt für Naturschutz (2020). Wälder im Klimawandel: Steigerung von Anpassungsfähigkeit und Resilienz durch mehr Vielfalt und Heterogenität. Bonn: Bad Godesberg.
Bundesamt für Umwelt (2019). Schwemmholz in Fliessgewässern: Ein praxisorientiertes Forschungsprojekt. Bern: Bundesamt für Umwelt.
Bundesministerium für Ernährung and Landwirtschaft (2021). Waldstrategie 2050. Rochusstr: Bundesministerium für Ernährung.
Bundesministerium für Finanzen (2022). Rechtsinformationssystem des Bundes. Wien: Bundesministerium für Finanzen.
BÜNDNIS 90/DIE GRÜNEN Ahrweiler (2022). Verantwortung in der Katastrophe – ein schwieriges Thema: Pressemitteilung des Ortsverbandes Bad Breisig. Niederdürenbach: BÜNDNIS 90/DIE GRÜNEN Ahrweiler.
Burrascano, S., Keeton, W. S., Sabatini, F. M., and Blasi, C. (2013). Commonality and variability in the structural attributes of moist temperate old-growth forests: A global review. For. Ecol. Manage. 291, 458–479. doi: 10.1016/j.foreco.2012.11.020
Calder, I. R., and Aylward, B. (2006). Forest and Floods. Water Int. 31, 87–99. doi: 10.1080/02508060608691918
Cavender-Bares, J., Balvanera, P., King, E., and Polasky, S. (2015). Ecosystem service trade-offs across global contexts and scales. Ecol. Soc. 20:22. doi: 10.5751/ES-07137-200122
Chazdon, R. L., Brancalion, P. H. S., Laestadius, L., Bennett-Curry, A., Buckingham, K., Kumar, C., et al. (2016). When is a forest a forest? Forest concepts and definitions in the era of forest and landscape restoration. Ambio 45, 538–550. doi: 10.1007/s13280-016-0772-y
Chen, Z., Wang, W., Woods, R. A., and Shao, Q. (2021). Hydrological effects of change in vegetation components across global catchments. J. Hydrol. 595:125775. doi: 10.1016/j.jhydrol.2020.125775
Chin, A. (1989). Step pools in stream channels. Progress Phys. Geogr. 13, 391–407. doi: 10.1177/030913338901300304
Chin, A., and Wohl, E. (2005). Toward a theory for step pools in stream channels. Progress Phys. Geogr. 29, 275–296. doi: 10.1191/0309133305pp449ra
Comiti, F., Lucía, A., and Rickenmann, D. (2016a). Large wood recruitment and transport during large floods: A review. Geomorphology 269, 23–39. doi: 10.1016/j.geomorph.2016.06.016
Comiti, F., Righini, M., Nardi, L., Lucía, A., Amponsah, W., Cavalli, M., et al. (2016b). “Channel widening during extreme floods: how to integrate it within river corridor planning?,” in Proceedings of the 13th Interpraevent Congress, Switzerland.
Commarmot, B., Abegg, M., Brändli, U.-B., Hobi, M. L., Korol, M., and Lanz, A. (2013). “Main results,” in Inventory of the largest primeval beech forest in Europe: A Swiss-Ukrainian Scientific Adventure, eds B. Commarmot, U.-B. Brändli, F. Hamor, and V. Lavnyy (Birmensdorf: Swiss Federal Inst. for Forest Snow and Landscape Research).
Curran, J. H., and Wohl, E. E. (2003). Large woody debris and flow resistance in step-pool channels, Cascade Range, Washington. Geomorphology 51, 141–157. doi: 10.1016/S0169-555X(02)00333-1
Deutsches Komitee Katastrophenvorsorge e.V (2022). Die Flutkatastrophe im Juli 2021 in Deutschland: Ein Jahr danach: Aufarbeitung und erste Lehren für die Zukunft. Bonn: Deutsches Komitee Katastrophenvorsorge e.V.
Dietze, M., Bell, R., Ozturk, U., Cook, K. L., Andermann, C., Beer, A. R., et al. (2022). More than heavy rain turning into fast-flowing water – a landscape perspective on the 2021 Eifel floods. EGUsphere doi: 10.5194/egusphere-2022-7 [Epub ahead of print].
Dietze, M., and Ozturk, U. (2021). A flood of disaster response challenges. Science 373, 1317–1318. doi: 10.1126/science.abm0617
Docker, B. B., and Hubble, T. (2008). Quantifying root-reinforcement of river bank soils by four Australian tree species. Geomorphology 100, 401–418. doi: 10.1016/j.geomorph.2008.01.009
Dorren, L., and Moos, C. (2022). Towards quantitative evidence of Eco-DRR in mountains: A concise review. Ecol. Eng. 175:106485. doi: 10.1016/j.ecoleng.2021.106485
Eisenbies, M. H., Aust, W. M., Burger, J. A., and Adams, M. B. (2007). Forest operations, extreme flooding events, and considerations for hydrologic modeling in the Appalachians—A review. For. Ecol. Manage. 242, 77–98. doi: 10.1016/j.foreco.2007.01.051
Ellenberg, H., and Leuschner, C. (2010). Vegetation Mitteleuropas mit den Alpen: In ökologischer, dynamischer und historischer Sicht; 203 Tabellen. Stuttgart: Ulmer.
European Commission (2021b). New EU Forest Strategy for 2030: Communication from the commission to the European Parliament, the Council, the European Economic and Social Comittee and the Committee of the Regions. Brussels: European Commission.
European Commission (2021a). Forging a climate-resilient Europe - the new EU Strategy on Adaptation to Climate: Communication from the commission to the European Parliament, the Council, the European Economic and Social Committee and the Committee of the Regions. Brussels: European Commission.
European Environment Agency (2017a). Climate change adaptation and disaster risk reduction in Europe: Enhancing coherence of the knowledge base, policies and practices. Luxembourg: Publications Office of the European Union.
European Environment Agency (2017b). Green infrastructure and flood management: Promoting cost-efficient flood risk reduction via green infrastructure solutions. Luxembourg: Publications Office of the European Union.
European Parliament and Council of the European Union (2007). DIRECTIVE 2007/60/EC on the assessment and management of flood risks. Luxembourg: European Parliament and Council of the European Union.
Forest Information System for Europe (2022). Forest Health. Moscow: Forest Information System for Europe.
Frehner, M., Wasser, B., and Schwitter, R. (2005). Nachhaltigkeit und Erfolgskontrolle im Schutzwald: Wegleitung für Pflegemassnahmen in Wäldern mit Schutzfunktion. Switzerland: Bundesamt für Umwelt BAFU.
Friedrich, H., Ravazzolo, D., Ruiz-Villanueva, V., Schalko, I., Spreitzer, G., Tunnicliffe, J., et al. (2022). Physical modelling of large wood (LW) processes relevant for river management: Perspectives from New Zealand and Switzerland. Earth Surf. Process. Landforms 47, 32–57. doi: 10.1002/esp.5181
Gasser, E., Perona, P., Dorren, L., Phillips, C., Hübl, J., and Schwarz, M. (2020). A New Framework to Model Hydraulic Bank Erosion Considering the Effects of Roots. Water 12:893. doi: 10.3390/w12030893
Gasser, E., Schwarz, M., Simon, A., Perona, P., Phillips, C., Hübl, J., et al. (2019). A review of modeling the effects of vegetation on large wood recruitment processes in mountain catchments. Earth-Sci. Rev. 194, 350–373. doi: 10.1016/j.earscirev.2019.04.013
Golly, A., Turowski, J. M., Badoux, A., and Hovius, N. (2017). Controls and feedbacks in the coupling of mountain channels and hillslopes. Geology 45, 307–310. doi: 10.1130/G38831.1
Gregory, K. J., Gurnell, A. M., and Hill, C. T. (1985). The permanence of debris dams related to river channel processes. Milton Park: Taylor & Francis.
Gurnell, A. M., Gregory, K. J., and Petts, G. E. (1995). The role of coarse woody debris in forest aquatic habitats: Implications for management. Aquatic Conserv. 5, 143–166. doi: 10.1002/aqc.3270050206
Gurnell, A. M., Piégay, H., Swanson, F. J., and Gregory, S. V. (2002). Large wood and fluvial processes. Freshw. Biol. 47, 601–619. doi: 10.1046/j.1365-2427.2002.00916.x
Hanewinkel, M., Kuhn, T., Bugmann, H., Lanz, A., and Brang, P. (2014). Vulnerability of uneven-aged forests to storm damage. Forestry 87, 525–534. doi: 10.1093/forestry/cpu008
Hewelke, E., Oktaba, L., Gozdowski, D., Kondras, M., Olejniczak, I., and Górska, E. (2018). Intensity and Persistence of Soil Water Repellency in Pine Forest Soil in a Temperate Continental Climate under Drought Conditions. Water 10:1121. doi: 10.3390/w10091121
Huber, M., Brang, P., and Sandri, A. (2015). “Protection against natural hazards,” in Forest report 2015. Condition and use of Swiss forests, ed. Federal Office for the Environment FOEN and Swiss Federal Institute for Forest (Swiss: Snow and Landscape Reseach WSL), 94–97.
Hümann, M., Schüler, G., Müller, C., Schneider, R., Johst, M., and Caspari, T. (2011). Identification of runoff processes – The impact of different forest types and soil properties on runoff formation and floods. J. Hydrol. 409, 637–649. doi: 10.1016/j.jhydrol.2011.08.067
Ibisch, P. L., Gohr, C., Mann, D., and Blumröder, J. S. (2021). Der Wald in Deutschland auf dem Weg in die Heißzeit: Vitalität und Schädigung in den Extremsommern 2018-2020. Schicklerstraße: Hochschule für nachhaltige Entwicklung Eberswalde.
IPCC (2014). “Climate Change 2014: Impacts, Adaptation, and Vulnerability. Part A: Global and Sectoral Aspects,” in Proceedings of the Working Group II to the Fifth Assessment Report of the Intergovernmental Panel on Climate Change, New York, NY.
IPCC (2022). “Summary for Policymakers,” in Climate Change 2022: Impacts, Adaptation, and Vulnerability. Contribution of Working Group II to the Sixth Assessment Report of the Intergovernmental Panel on Climate Change, eds H.-O. Pörtner, D. C. Roberts, M. Tignor, E. S. Poloczanska, K. Mintenbeck, A. Alegría, et al. (New York, NY: IPCC).
Iskin, E. P., and Wohl, E. (2021). Wildfire and the patterns of floodplain large wood on the Merced River, Yosemite National Park, California, USA. Geomorphology 389:107805. doi: 10.1016/j.geomorph.2021.107805
Junghänel, T., Bissolli, P., Daßler, J., Fleckenstein, R., Imbery, F., Janssen, W., et al. (2021). Hydro-klimatologische Einordnung der Stark- und Dauerniederschläge in Teilen Deutschlands im Zusammenhang mit dem Tiefdruckgebiet,, Bernd“ vom 12. bis 19. Juli 2021. Mumbai: DWD.
Karl, S., Block, J., Schüler, G., Schultze, B., and Scherzer, J. (2012). Wasserhaushaltsuntersuchungen im Rahmen des forstlichen Umweltmonitorings und bei Waldbaulichen versuchen in Rheinland-Pfalz. Germany: Trippstadt.
Lachat, T., Brang, P., Bolliger, M., Bollmann, K., Brändli, U.-B., Bütler, R., et al. (2019). Totholz im Wald: Entstehung, Bedeutung und Förderung. Merkbl. Für Die Prax. 51, 1–12.
Landesamt für Umwelt Rheinland-Pfalz (2022). Bericht: Hochwasser im Juli 2021. Mainz: Landesamt für Umwelt Rheinland-Pfalz.
Lange, B., Lüescher, P., and Germann, P. F. (2009). Significance of tree roots for preferential infiltration in stagnic soils. Hydrol. Earth Syst. Sci. 13, 1809–1821. doi: 10.5194/hess-13-1809-2009
Larsen, A., Larsen, J. R., and Lane, S. N. (2021). Dam builders and their works: Beaver influences on the structure and function of river corridor hydrology, geomorphology, biogeochemistry and ecosystems. Earth-Sci. Rev. 218:103623. doi: 10.1016/j.earscirev.2021.103623
Levine, R., and Meyer, G. A. (2019). Beaver-generated disturbance extends beyond active dam sites to enhance stream morphodynamics and riparian plant recruitment. Sci. Rep. 9:8124. doi: 10.1038/s41598-019-44381-2
Lienkaemper, G. W., and Swanson, F. J. (1987). Dynamics of large woody debris in streams in old-growth Douglas-fir forests. Can. J. For. Res. 17, 150–156.
Linstead, C., and Gurnell, A. M. (1999). Large Wood Debris in British Headwater Rivers: Physical Habitat Role and Management Guidelines. London: United Kingdom Government.
Losey, S., and Wehrli, A. (2013). Schutzwald in der Schweiz - Vom Projekt SilvaProtect-CH zum harmonisierten Schutzwald. Bern: Bundesamt für Umwelt.
Lucía, A., Comiti, F., Borga, M., Cavalli, M., and Marchi, L. (2015). Dynamics of large wood during a flash flood in two mountain catchments. Nat. Hazards Earth Syst. Sci. 15, 1741–1755. doi: 10.5194/nhess-15-1741-2015
Luo, Z., Niu, J., He, S., Zhang, L., Chen, X., Tan, B., et al. (2023). Linking roots, preferential flow, and soil moisture redistribution in deciduous and coniferous forest soils. J. Soils Sed. 23, 1524–1538. doi: 10.1007/s11368-022-03375-w
Lüscher, P., and Zürcher, K. (2002). Wald schützt nicht immer vor Hochwasser. Wasserabfluss hängt wesentlich von Bodeneigenschaften ab. Switzerland: Informationsblatt Wald.
Mao, J., Nierop, K. G. J., Rietkerk, M., Sinninghe Damsté, J. S., and Dekker, S. C. (2016). The influence of vegetation on soil water repellency-markers and soil hydrophobicity. The Science of the total environment 566, 608–620. doi: 10.1016/j.scitotenv.2016.05.077
Markart, G. (2000). Zum Wasserhaushalt von Hochlagenaufforstungen – am Beispiel der Aufforstung von Haggen bei St. Sigmund im Sellrain. Vienna: FBVA-Berichte.
Markart, G., Kohl, B., Sotier, B., Klebinder, K., Schauer, T., Bunza, G., et al. (2011). A Simple Code of Practice for the Assessment of Surface Runoff Coefficients for Alpine Soil-/Vegetation Units in Torrential Rain (Version 2.0). Geneva: Department of Natural Hazards.
Markart, G., Perzl, F., Lechner, V., Kohl, B., Hauser, P., Geitner, C., et al. (2020). Handlungsanleitung -Optimierung der hydrologischen Wirkung von Schutzwäldern Projekt ITAT4041-BLÖSSEN Auswirkungen verzögerter Wiederbewaldung im Schutzwald auf die Sicherheit vor Naturgefahren (insbesondere Abflussbildung). Innsbruck: Land Tirol.
Markart, G., Teich, M., Scheidl, C., and Kohl, B. (2021). “Flood Protection by Forests in Alpine Watersheds: Lessons Learned from Austrian Case Studies,” in Protective forests as Ecosystem-based solution for Disaster Risk Reduction (ECO-DRR) [Working Title], eds M. Teich, C. Accastello, F. Perzl, and K. Kleemayr (London: IntechOpen).
Mazzorana, B., Ruiz-Villanueva, V., Marchi, L., Cavalli, M., Gems, B., Gschnitzer, T., et al. (2018). Assessing and mitigating large wood-related hazards in mountain streams: recent approaches. J. Flood Risk Manage 11, 207–222. doi: 10.1111/jfr3.12316
Millennium Ecosystem Assessment (2003). Ecosystems and Human Well-being: A Framework for Assessment. London: Millennium Ecosystem Assessment.
Moos, C., Bebi, P., Graf, F., Mattli, J., Rickli, C., and Schwarz, M. (2016). How does forest structure affect root reinforcement and susceptibility to shallow landslides? Earth Surf. Process. Landforms 41, 951–960. doi: 10.1002/esp.3887
Munich RE (2022). Weather disasters in USA dominate natural disaster losses in 2021: Media Release. Munich: Munich RE.
Nardi, L., and Rinaldi, M. (2015). Spatio-temporal patterns of channel changes in response to a major flood event: the case of the Magra River (central-northern Italy). Earth Surf. Process. Landforms 40, 326–339. doi: 10.1002/esp.3636
Neary, D. G., Ice, G. G., and Jackson, C. R. (2009). Linkages between forest soils and water quality and quantity. For. Ecol. Manage. 258, 2269–2281. doi: 10.1016/j.foreco.2009.05.027
Neuhaus, V., and Mende, M. (2021). Engineered Large Wood Structures in Stream Restoration Projects in Switzerland: Practice-Based Experiences. Water 13:2520. doi: 10.3390/w13182520
Neumayer, M., Teschemacher, S., Schloemer, S., Zahner, V., and Rieger, W. (2020). Hydraulic Modeling of Beaver Dams and Evaluation of Their Impacts on Flood Events. Water 12:300. doi: 10.3390/w12010300
Nordmann, B., Goettlein, A., and Binder, F. (2009). Einfluss unterschiedlicher Waldbestockung auf die Abflussbildung – ein Beispiel aus einem Wassereinzugsgebiet im Frankenwald. Berlin: Hydrologie und Wasserbewirtschaftung, 80–95.
Persson, R. (2020). The global forest and tree-cover situation in 2020 – Facts. Myths: Lies & White Lies.
Pichler, A., and Stöhr, D. (2018). Gewässerbegleitwälder – 10 Jahre Wildbachbetreuung Tirol. Stand der Technik im Naturgefahren-Ingenieurwesen. Schriftrenreihe Department Bautechnik und Naturgefahren. Vienna: Universität für Bodenkultur, 29–30.
Reinhardt, C., Bölscher, J., Schulte, A., and Wenzel, R. (2011). Decentralised water retention along the river channels in a mesoscale catchment in south-eastern Germany. Phys. Chem. Earth 36, 309–318. doi: 10.1016/j.pce.2011.01.012
Rickli, C., Badoux, A., Rickenmann, D., Steeb, N., and Waldner, P. (2018). Large wood potential, piece characteristics, and flood effects in Swiss mountain streams. Phys. Geogr. doi: 10.1080/02723646.2018.1456310 [Epub ahead of print].
Ruiz-Villanueva, V., and Stoffel, M. (2017). Frederick J. Swanson’s 1976–1979 papers on the effects of instream wood on fluvial processes and instream wood management. Progress Phys. Geogr. 41, 124–133. doi: 10.1177/0309133317692411
Scheidl, C., Heiser, M., Kamper, S., Thaler, T., Rammer, W., Seidl, R., et al. (2021). “Influence of Canopy Disturbances on Runoff and Landslide Disposition after Heavy Rainfall Events,” in Protective forests as Ecosystem-based solution for Disaster Risk Reduction (ECO-DRR), eds M. Teich, C. Accastello, F. Perzl, and K. Kleemayr (London: IntechOpen).
Schmocker, L., and Weitbrecht, V. (2013). Driftwood: Risk analysis and engineering measures. J. Hydraul. Eng. 139, 683–695. doi: 10.1061/(ASCE)HY.1943-7900.0000728
Schüler, G. (2006). Identification of flood-generating forest areas and forestry measures for water retention. For. Snow Landsc. Res. 99–114.
Schüler, G. (2007). “Wasserrückhalt im Wald – Ein Beitrag zum vorbeugenden Hochwasserschutz,” in Dezentraler Wasserrückhalt in der Landschaft durch vorbeugende Maßnahmen der Waldwirtschaft, der Landwirtschaft und im Siedlungswesen: Das INTERREG IIIB NWE Projekt WaReLa – Ergebnisse aus vier Jahren Umsetzung und Forschung für einen nachhaltigen Hochwasserschutz in der Fläche, eds S. Süd and FAWF (Trippstadt: FAWF), 7–20.
Schwitter, R., Glanzmann, L., and Zürcher, S. (2019). Jungwaldpflege im Gebirgs- und Schutzwald. Zurich: ETH Zurich.
Sebald, J., Senf, C., Heiser, M., Scheidl, C., Pflugmacher, D., and Seidl, R. (2019). The effects of forest cover and disturbance on torrential hazards: large-scale evidence from the Eastern Alps. Environ. Res. Lett. 14:114032. doi: 10.1088/1748-9326/ab4937
Seo, J. I., Nakamura, F., and Chun, K. W. (2010). Dynamics of large wood at the watershed scale: a perspective on current research limits and future directions. Landsc. Ecol. Eng. 6, 271–287. doi: 10.1007/s11355-010-0106-3
Simon, A., and Collison, A. J. C. (2002). Quantifying the mechanical and hydrologic effects of riparian vegetation on streambank stability. Earth Surf. Process. Landforms 27, 527–546. doi: 10.1002/esp.325
Steeb, N., Rickenmann, D., Badoux, A., Rickli, C., and Waldner, P. (2017). Large wood recruitment processes and transported volumes in Swiss mountain streams during the extreme flood of August 2005. Geomorphology 279, 112–127. doi: 10.1016/j.geomorph.2016.10.011
Steeb, N., Ruiz-Villanueva, V., Badoux, A., Rickli, C., Mini, A., Stoffel, M., et al. (2023). Geospatial modelling of large wood supply to rivers: a state-of-the-art model comparison in Swiss mountain river catchments. Earth Surf. Dynam. 11, 487–509. doi: 10.5194/esurf-11-487-2023
Sudmeier-Rieux, K., Arce-Mojica, T., Boehmer, H. J., Doswald, N., Emerton, L., Friess, D. A., et al. (2021). Scientific evidence for ecosystem-based disaster risk reduction. Nat. Sustain. 4, 803–810. doi: 10.1038/s41893-021-00732-4
Surian, N., Righini, M., Lucía, A., Nardi, L., Amponsah, W., Benvenuti, M., et al. (2016). Channel response to extreme floods: Insights on controlling factors from six mountain rivers in northern Apennines, Italy. Geomorphology 272, 78–91. doi: 10.1016/j.geomorph.2016.02.002
Swanson, F. J., Gregory, S. V., Iroumé, A., Ruiz-Villanueva, V., and Wohl, E. (2021). Reflections on the history of research on large wood in rivers. Earth Surf. Process. Landforms 46, 55–66. doi: 10.1002/esp.4814
Swanson, F. J., Gregory, S. V., Sedell, J. R., and Campbell, A. G. (1982). “Land-water interactions: the riparian zone,” in Analysis of coniferous forest ecosystems in the Western United States, ed. R. L. Edmonds (Stroudsberg, PA: Hutchinson Ross), 267–291.
Teich, M., Accastello, C., Perzl, F., and Berger, F. (2021). Protective Forests for Ecosystem-based Disaster Risk Reduction (Eco-DRR) in the Alpine Space. London: IntechOpen.
Thomas, H., and Nisbet, T. (2012). Modelling the hydraulic impact of reintroducing large woody debris into watercourses. J. Flood Risk Manage 5, 164–174. doi: 10.1111/j.1753-318X.2012.01137.x
Trumbore, S., Brando, P., and Hartmann, H. (2015). Forest health and global change. Science 349, 814–818. doi: 10.1126/science.aac6759
Vaz, P. G., Merten, E. C., Robinson, C. T., and Pinto, P. (2021). Severely burned wood from wildfires has low functional potential in streams. J. Appl. Ecol. 58, 1346–1356. doi: 10.1111/1365-2664.13872
Vaz, P. G., Warren, D. R., Pinto, P., Merten, E. C., Robinson, C. T., and Rego, F. C. (2011). Tree type and forest management effects on the structure of stream wood following wildfires. For. Ecol. Manage. 262, 561–570. doi: 10.1016/j.foreco.2011.04.026
Vergani, C., Giadrossich, F., Buckley, P., Conedera, M., Pividori, M., Salbitano, F., et al. (2017). Root reinforcement dynamics of European coppice woodlands and their effect on shallow landslides: A review. Earth-Sci. Rev. 167, 88–102. doi: 10.1016/j.earscirev.2017.02.002
Waldner, P., Rickli, C., Köchli, D., Usbeck, T., Schmocker, L., Sutter, et al. (2007). “Schwemmholz,” in Ereignisanalyse Hochwasser 2005: Teil 1: Prozesse, Schäden und erste Einordnungen, eds G. R. Bezzola and C. Hegg (Santa Monica: Bundesamt für Umwelt BAFU and Eidgenössische Forschungsanstalt WSL).
Walz, Y., Janzen, S., Narvaez, L., Ortiz-Vargas, A., Woelki, J., Doswald, N., et al. (2021a). Disaster-related losses of ecosystems and their services. Why and how do losses matter for disaster risk reduction? Int. J. Disaster Risk Reduct. 63:102425. doi: 10.1016/j.ijdrr.2021.102425
Walz, Y., Nick, F., Higuera Roa, O., Nehren, U., and Sebesvari, Z. (2021b). Coherence and Alignment among Sustainable Land Management, Ecosystem-based Adaptation, Ecosystem-based Disaster Risk Reduction and Nature-based Solutions. Bonn: Institute for Environment and Human Security.
Watson, J. E. M., Evans, T., Venter, O., Williams, B., Tulloch, A., Stewart, C., et al. (2018). The exceptional value of intact forest ecosystems. Nat. Ecol. Evol. 2, 599–610. doi: 10.1038/s41559-018-0490-x
Welle, T. (2021). Die unterschätzte Bedeutung von Schutzwäldern in Mittelgebirgen: Erkenntnisse aus dem Hochwasser im Ahrtal. Minneapolis, MN: Erkenntnisse aus dem Hochwasser im Ahrtal.
Wilcox, A. C., and Wohl, E. E. (2006). Flow resistance dynamics in step-pool stream channels: 1. Large woody debris and controls on total resistance. Water Resour. Res. 42:4277. doi: 10.1029/2005WR004277
Wissenschaftlicher Beirat für Waldpolitik beim Bundesministerium für Ernährung und Landwirtschaft (2021). Die Anpassung von Wäldern und Waldwirtschaft an den Klimawandel. Berlin: Bundesministerium für Ernährung und Landwirtschaft.
Wohl, E. (2013). Floodplains and wood. Earth-Sci. Rev. 123, 194–212. doi: 10.1016/j.earscirev.2013.04.009
Wohl, E., and Iskin, E. P. (2022). The Transience of Channel-Spanning Logjams in Mountain Streams. Water Resour. Res. 58:31556. doi: 10.1029/2021WR031556
Wohl, E., and Scamardo, J. E. (2020). The resilience of logjams to floods. Hydrol. Process. doi: 10.1002/hyp.13970 [Epub ahead of print].
Wu, G.-L., Cui, Z., and Huang, Z. (2021). Contribution of root decay process on soil infiltration capacity and soil water replenishment of planted forestland in semi-arid regions. Geoderma 404:115289. doi: 10.1016/j.geoderma.2021.115289
Xiao, L., Robinson, M., and O’Connor, M. (2022). Woodland’s role in natural flood management: Evidence from catchment studies in Britain and Ireland. Sci. Total Environ. 813:151877. doi: 10.1016/j.scitotenv.2021.151877
Keywords: forest management, protective forests, Large Wood, deadwood, ecosystem services, ecosystem disservices, nature-based solutions, ecosystem-based solutions
Citation: Rackelmann F, Sebesvari Z and Bell R (2023) Synergies and trade-offs in the management objectives forest health and flood risk reduction. Front. For. Glob. Change 6:1208032. doi: 10.3389/ffgc.2023.1208032
Received: 18 April 2023; Accepted: 26 June 2023;
Published: 12 July 2023.
Edited by:
Ana Stritih, Technical University of Munich, GermanyReviewed by:
Nicolas Steeb, Swiss Federal Institute for Forest, Snow and Landscape Research (WSL), SwitzerlandMilan Kobal, University of Ljubljana, Slovenia
Copyright © 2023 Rackelmann, Sebesvari and Bell. This is an open-access article distributed under the terms of the Creative Commons Attribution License (CC BY). The use, distribution or reproduction in other forums is permitted, provided the original author(s) and the copyright owner(s) are credited and that the original publication in this journal is cited, in accordance with accepted academic practice. No use, distribution or reproduction is permitted which does not comply with these terms.
*Correspondence: Fabian Rackelmann, ZmFiaWFuLnJhY2tlbG1hbm5AdW5pLWJvbm4uZGU=