- 1Department of Botany, Kumaun University, Nainital, India
- 2Wildlife Institute of India, Dehradun, India
Introduction: Riverine forests in the Himalaya represent a biodiverse, dynamic, and complex ecosystem that offers numerous ecosystem services to local and downstream communities and also contributes to the regional carbon cycle. However, these forests have not been assessed for their contribution to dry matter dynamics and carbon flux. We studied these parameters along three classes of riverine forests in eastern Uttarakhand, dominated by Macaranga, Alnus, and Quercus-Machilus forest.
Methods: Using volume equations, we assessed tree biomass, carbon storage, and sequestration in the study area.
Results: The total standing tree biomass in Macaranga, Alnus, and Quercus-Machilus forest ranged from 256.6 to 558.1 Mg ha−1, 460.7 to 485.8 Mg ha−1, and 508.6 to 692.1 Mg ha−1, respectively. A total of 77.6–79.6% of vegetation biomass was stored in the aboveground biomass and 20.4–22.4% in belowground plant parts across the riverine forests. The carbon stock in Macaranga forest ranged from 115.5 to 251.1 Mg ha−1, in Alnus forest from 207.3 to 218.6 Mg ha−1, and in Quercus-Machilus forest from 228.9 to 311.4 Mg ha−1. The mean annual litterfall was accounted maximum for Quercus-Machilus forest (5.94 ± 0.54 Mg ha−1 yr.−1), followed by Alnus (5.57 ± 0.31 Mg ha−1 yr.−1) and Macaranga forest (4.67 ± 0.39 Mg ha−1 yr.−1). The highest value of litterfall was recorded during summer (3.40 ± 0.01 Mg ha−1 yr.−1) and the lowest in winter (0.74 ± 0.01 Mg ha−1 yr.−1). The mean value of net primary productivity and carbon sequestration was estimated to be highest in Quercus-Machilus forest (15.8 ± 0.9 Mg ha−1 yr.−1 and 7.1 ± 0.9 Mg C ha−1 yr.−1, respectively) and lowest in Alnus forest (13.9 ± 0.3 Mg ha−1 yr.−1 and 6.1 ± 0.3 Mg C ha−1 yr.−1, respectively).
Discussion: The results highlight that riverine forests play a critical role in providing a large sink for atmospheric CO2. To improve sustainable ecosystem services and climate change mitigation, riverine forests must be effectively managed and conserved in the region.
1. Introduction
Riverine forests play a significant role in ecosystem processes, including energy flow and carbon dynamics (Allan et al., 2021). These forests are also known to serve as important bio-corridors for the movement of a variety of faunal groups during various seasons (Rawat, 2017). They are a productive ecosystem and are hotspots for epiphytic orchids and agricultural output (Marwah et al., 2021). Riverine forests hold discrete ecological traits because of their interaction with the aquatic ecosystem. Thus, their borders can be identified by variations in soil conditions, vegetation, and other elements that represent this aquatic–terrestrial interaction (Naiman et al., 2000). The riverine forests all over the Himalayan region are susceptible to natural (floods and landslides), anthropogenic, and climatic fluctuations (Bookhagen and Burbank, 2010). Despite the importance of these forests, overexploitation, unrestricted grazing, fuel wood collection, agricultural development, boundary disputes, developmental projects, and linear infrastructures pose a serious threat to these ecosystems (Merawi, 2016; Rajpara et al., 2022).
The riverine forests in the Himalayan region are represented by a number of early and mid-seral communities such as Acacia catechu–Dalbergia sissoo along foothills, Drypetes roxburghii–Toona ciliata, Duabanga grandiflora–Terminalia myriocarpa (eastern Himalaya), Macaranga pustulata, Alnus nepalensis, Debregeasia hypoleuca, Hippophae salicifolia, Salix species, and Populus ciliata, etc., at higher elevations (Champion and Seth, 1968; Rawat, 2017). Of these, most dominant forest formations, especially between 900 and 2,200 m above sea level, are formed by Macaranga pustulata and Alnus nepalensis. These species form early successional stages (Dhar et al., 1997), colonizing freshly eroded river banks and landslide areas. In Central and Eastern Himalaya, the following communities of riverine forests become more prevalent: Macaranga forests (below 1,000 m), Alnus forests between 1,000 and 2000 m, and Quercus-Machilus forests between 1,500 and 2,200 m asl. The alder forest frequently forms pure stands in landslide-prone areas, although it also occurs mixed with other late successional species in the Himalayan region (Joshi and Garkoti, 2021). It is primarily found close to moist habitats and can fix atmospheric nitrogen through symbiotic interaction with gram-positive Frankia, allowing it to survive exceptionally hard climatic conditions and hasten the natural succession (Crisafulli et al., 2015; Joshi and Garkoti, 2021). Due to their crucial role in socioeconomic advantages, ecological functioning, and soil conservation, the local communities depend on these forests for their sustenance needs (Bisht et al., 2022a).
The dry matter dynamics is a key indicator from the energy fixation and flux of biological materials point of view (Anderson, 1971). According to the Intergovernmental Panel on Climate Change, carbon stored in a forest ecosystem is divided into three main pools, i.e., biomass, litter, and soil. The carbon pools in a forest ecosystem are influenced by topography, forest structure, tree species composition, species diversity, age structure, edaphic conditions, and human-induced disturbances (Arasa-Gisbert et al., 2018; FSI, 2021). The current total carbon pool in the forested zone of the Indian Himalayan Region is about 103.9 Mg ha−1, of which 23% is in the sub-tropical forests (FSI, 2021). Among the edaphic factors, the nutrient content in the soil may affect parameters such as tree growth and basal area and thus consequently influence the structure of plant communities (Becknell and Powers, 2014) and carbon storage. To formulate viable strategies to increase the soil carbon, it is critical to include soil restoration, forest regeneration, and nutrient management (Sagar and Singh, 2005; Lal, 2015). The majority of organic matter elaborated by plants through photosynthesis is returned to the soil as litter. The litterfall results in seasonal or constant input of organic matter and nutrients to the forest floor and represents one of the most important pathways for the transfer of energy, affecting the growth and development of the vegetation and soil pool (Mfilinge et al., 2002).
There is a paucity of literature on the structure and functioning of these forests from the region. Therefore, the assessment of riverine forests, including their conservation status and potential for carbon flux, standing biomass, productivity, and carbon accumulation, is critical for long-term conservation planning and national accounting of the United Nations convention on climate change. Since forests sequester carbon from the atmosphere as part of the growth process, any increase in the biomass constitutes a sink that reduces the build-up of atmospheric carbon dioxide. This emphasizes the importance of forest ecosystems in the global carbon cycle and the necessity to accurately evaluate the amount of carbon stored in forest ecosystems (Pan et al., 2011). Interestingly, this is the first study on the dynamics of dry matter and carbon flux in riverine forests from the Gori valley, Western Himalaya. There is no prior literature on forest biomass and accumulation of carbon for this region. This stimulated the estimation of productivity and sequestration to understand the functioning of the riverine forest ecosystem. The information obtained from this study will significantly contribute to the dry matter dynamics and carbon cycle across the globe. Furthermore, it will benefit forest officials and ecologists for the conservation and restoration of riverine habitats. Thus, the present study was conducted to address the following questions: (1) How does the altitude affect phytosociological parameters in the region? (2) What is the pattern of biomass and net primary productivity in the riverine forests? (3) What is the magnitude of litterfall? (4) How do the carbon stock and sequestration potential vary in the riverine forests of Kumaun Himalaya, India?
2. Materials and methods
2.1. Study area
The study was carried out in the Gori watershed of the eastern Kumaun region, encompassing an area of 1920 km2 (Figure 1). Out of the 11 micro-watersheds, 6 (Dogrigad, Ghosigad, Painagad, Madkani river, Dhuratoli, and Patmoligad) were selected for the study after a reconnaissance survey. These study sites were located at 29°45′11.03 to 30°20′56.20 N and 80°22′49.90 to 80°11′41.34 E between 900 and 2,200 m asl in Pithoragarh district of Uttarakhand state. All the sampling was done within a 500 m distance of rivers and rivulets. The riverine forests in the study area are represented by two early seral communities (Macaranga and Alnus) and two more stable and climax communities (Toona-Engelhardia and Quercus-Machilus). Around 25.5% (489.2 km2) of the geographical study area consists of forest, with broadleaf deciduous forest (Macaranga, Alnus) and broadleaf evergreen forest (Quercus-Machilus) occupying a 25.1 and 36.3 km2 area, respectively. The area forms a transition zone between eastern (M. pustulata) and western (Pinus roxburghii and Q. semecarpifolia) Himalayan forests and comprises an exceptional diversity of orchid flora (Bisht and Adhikari, 2014). However, Toona-Engelhardia patches along the Gori river have disappeared from lower altitudes due to linear infrastructure and development projects.
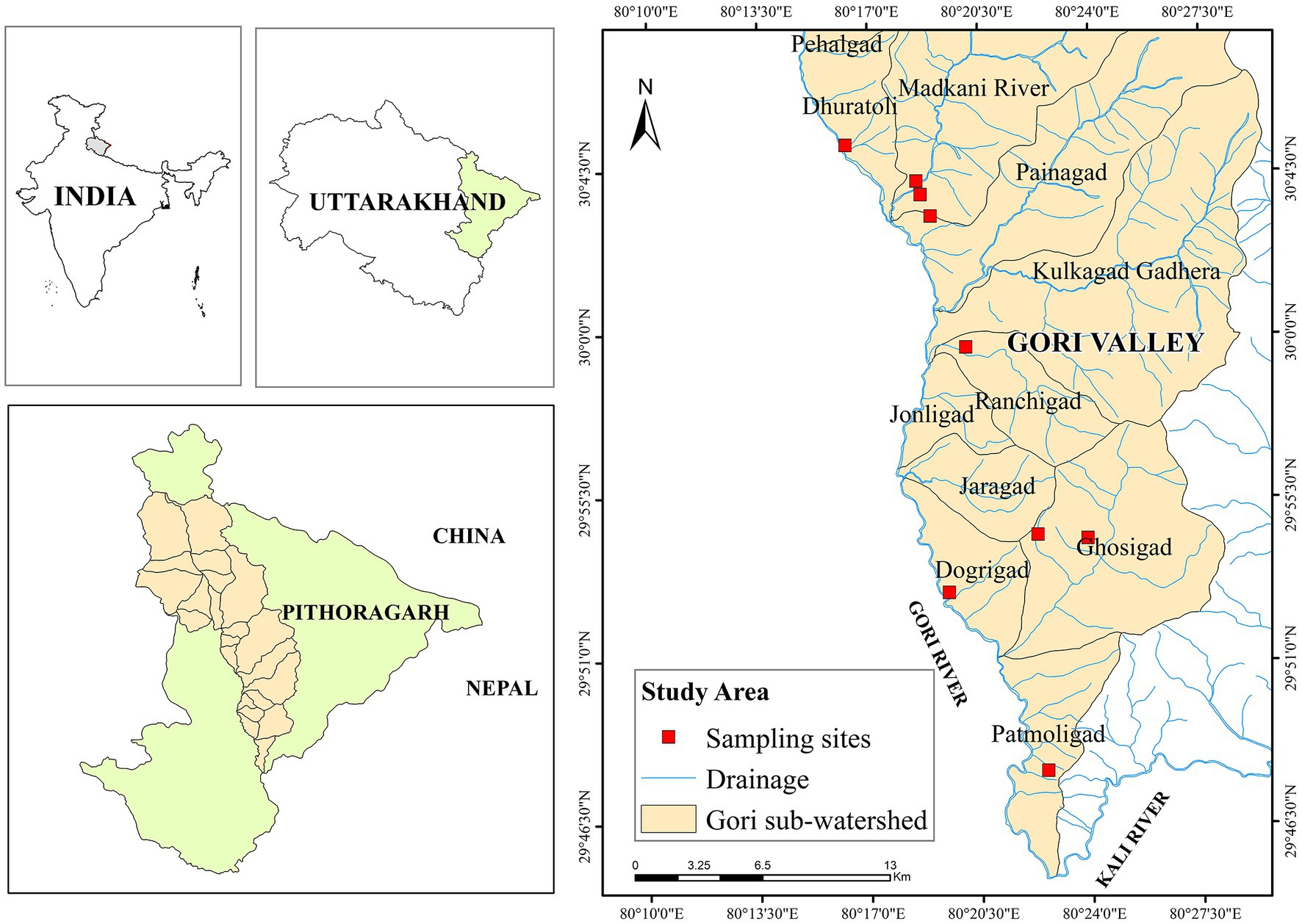
Figure 1. Map of study area showing sampling points and micro-watersheds in Gori valley, Western Himalaya.
2.2. Climate
The annual climate of the area is monsoon type, which can be divided into three prominent seasons, i.e., winter (October to February), summer (March to mid-June), and rainy season (mid-June to September). The mean maximum monthly temperature ranges from 15.1°C (January) to 30.4°C (June), and the minimum temperature ranges from 2.6 (January) to 18.8 (July). The average annual precipitation is 793.6 mm, and the annual mean relative humidity is 51.2% (Figure 2).
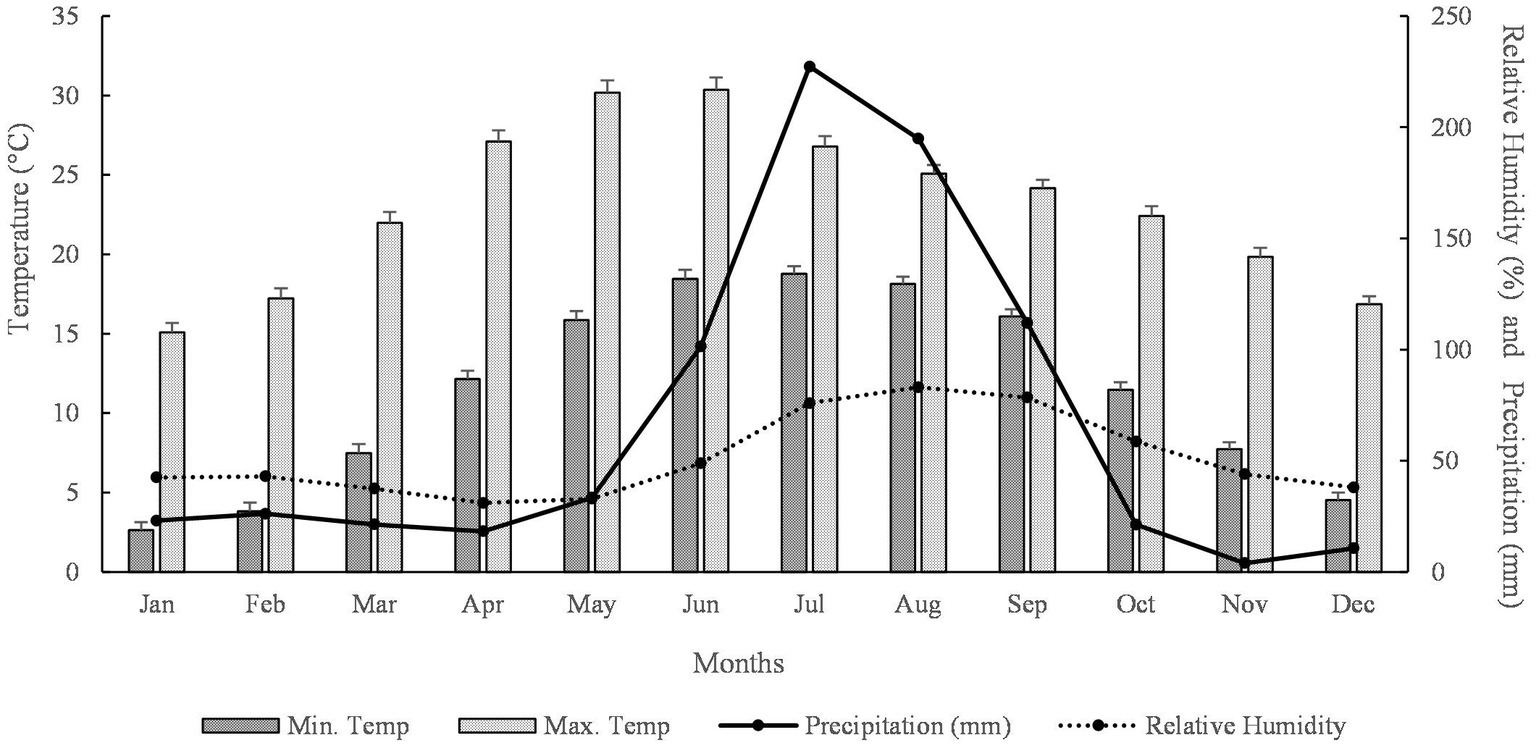
Figure 2. Meteorological data of the study area from 1981 to 2019 (Source: https://power.larc.nasa.gov, accessed on 6 June 2022).
2.3. Geology
The dominant rock types include phyllites, schists, gneisses, siltstone, and basic intrusive rocks belonging to the Vaikrita Group, especially in the Gori valley (Dumka, 2011; Ali et al., 2013). Between Baram and Baikot, a steeply dipping NNWSSE (western syncline) trending fault coincides with the thrust, defining the base of the Chhiplakot Klippe. Significantly, a subvertical slab of coarse-grained, unmetamorphosed granite with accompanying quartz porphyry and felsite is emplaced along the fault zone, bringing about contact metamorphism in the Precambrian to Lower Paleozoic dolomite and carbonaceous slate of the Tejam zone. Its mylonitized and greatly shared border along the wide valley bears testimony to the post-emplacement movement along the fault. There is yet another similar granitic body right within the same sedimentary, between dolomites and carbonaceous slates, discernible in the tract further downstream in the valley between Toli and Chiphaltara (Valdiya, 1976).
2.4. Soil sampling
2.4.1. Data collection
The soil samples from each forest site were collected from three depths (0–10, 10–20, and 20–30 cm) in triplicate with the help of a soil corer of known volume. The collected soil samples were systematically mixed and packed separately in zip lock bags and instantly transferred to the laboratory. Manual removal of coarse items such as stones, roots, and litter were carried out. The soil balls were crushed to separate the soil particles, and then, field damp soil samples were sieved with a 2 mm mesh and air dried to test the soil physico-chemical characteristics. The soil moisture (Mo) was determined gravimetrically by drying the soil samples in an oven to constant weight. The weight of the fresh soil and the oven dry weight of the same soil sample was expressed as a percentage of the difference of the moisture content (Jackson, 1958). The water-holding capacity (WHC) of the soil samples was measured by using the formula following Piper (1950). The bulk density (BD) was estimated with the help of a special mental core cylinder of known volume.
The soil pH was measured using a digital soil pH meter (Systronic-μ pH system 361) with ±0.05 accuracy. The soil water suspension was conducted in the ratio of 1:2. The instrument was calibrated with a standard buffer solution of pH 4, 7, and 9.2 before measurement. The soil organic carbon (OC) was determined using the Walkley and Black (1934) titration method following Jackson (1958). Nitrogen (N) was estimated using Kjeltec-2300 following the micro-Kjeldahl application of Peach and Tracey (1956) and Misra (1968). Available phosphorus (P) was estimated following the Olsen et al. (1954) method and potassium (K) by Flame Photometer after proper digestion of the samples. The factor of 1.724 was used to convert the OC into soil organic matter (SOM; Jackson, 1958; Misra, 1968). The value of organic carbon and SOM were determined by multiplying the values of carbon (%) with factors of 1.3 and 1.724, respectively.
2.5. Growing stock and biomass estimation
Nine forest sites were assessed for biomass and carbon stock based on altitudinal gradient. Within each site, 10 quadrats of a 10×10 m size were laid randomly with a replicate of three. All individual trees were measured for circumference at breast height (cbh) at 1.37 m from the ground (Singh et al., 1997). Following Curtis and McIntosh (1950), Misra (1968), and Mueller-Dombois and Ellenberg (1974), the data were analyzed for density and the basal area of the tree species for distinct forest types. The species diversity index was computed using the Shannon–Weiner diversity Index (Shannon and Weaver, 1949), and richness was considered as the total number of species in the sampled site (Wani and Pant, 2023). The forest types were identified using TWINSPAN.
The growing stock volume (GSV) equations (FSI, 1996, Table 1) were used to estimate aboveground biomass (Sharma et al., 2010; Dimri et al., 2017; Bisht et al., 2023) since allometric equations were not available for each species. The estimated GSV (m3 ha−1) was multiplied by the appropriate biomass expansion factor (Brown et al., 1999) to convert into aboveground biomass (AGB), i.e., stems, branches, twigs, and leaves. In addition, the equation of Cairns et al. (1997) was used to estimate belowground biomass (BGB), which reflects the biomass of root components. The sum of AGB and BGB yielded the total biomass for trees (TB, Mg ha−1).
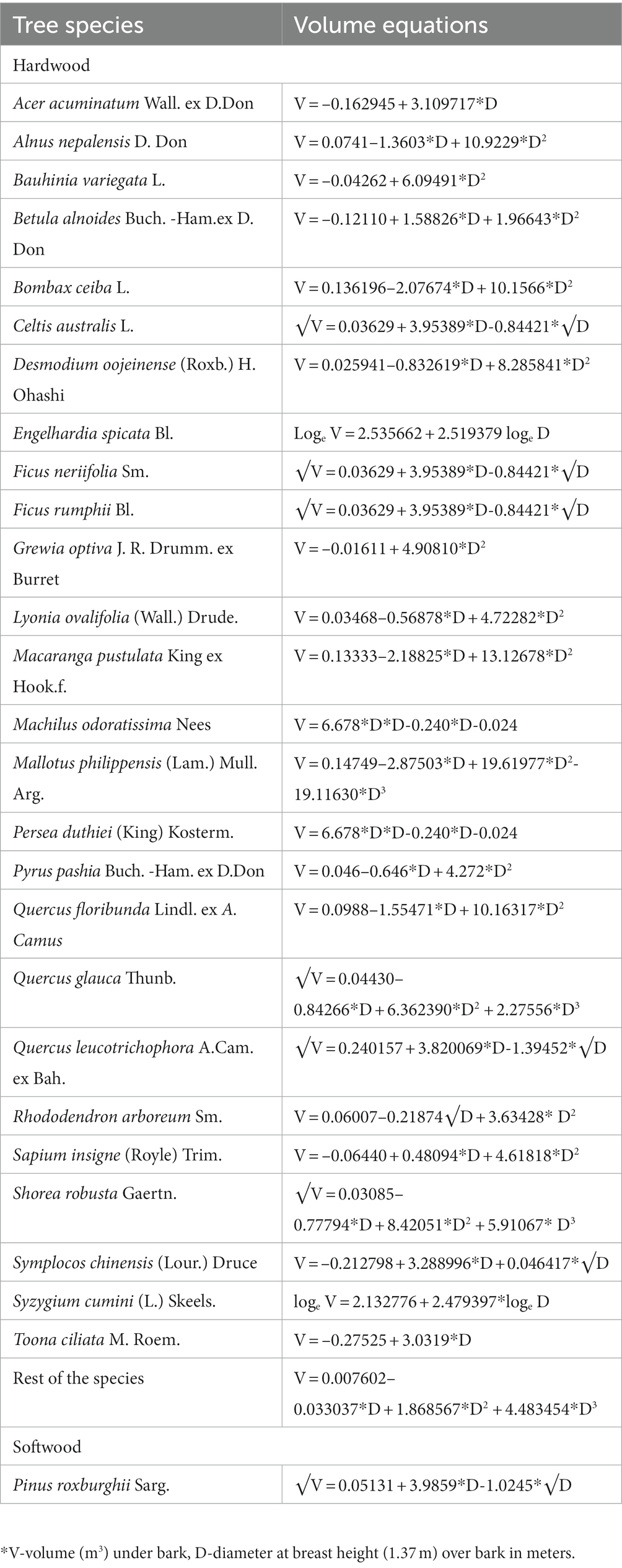
Table 1. List of volume equations used for different tree species in the study sites based on Forest Research Institute and Forest Survey of India.
2.6. Estimation of litterfall and net primary productivity
The litterfall was estimated annually by placing five litter traps at different locations at each forest site. Each wooden trap was 0.5×0.5×0.15 m in size and fitted with a nylon net at the bottom. The litter from each trap was collected in a zip-lock bag season wise, i.e., winter (October to February), summer (March to May), and rainy season (June to September). The samples were then brought to the laboratory and oven-dried at 80°C. Half of the oven-dried weight of the collected litter from each site was considered its carbon content (Verma and Jain, 2017).
For the estimation of productivity in each forest, 1/4th of the trees were marked in the year 2017, and their cbh was remeasured in each sampling plot in the subsequent year, i.e., 2018. The net change in biomass (ΔB = B2-B1) yielded annual biomass accumulation. Annual litterfall was added to the aboveground biomass accumulation, and one-fifth of the leaf litter was added to the belowground biomass accumulation as mortality in fine roots (Orlov, 1968; Ogino, 1977). The latter value equaled net primary productivity. The biomass accumulation ratio (BAR), a measure of biomass to net primary productivity, was used to characterize the production condition in the forest communities (Whittaker, 1975).
2.7. Estimation of carbon stock and sequestration
For the total carbon stock (TC, Mg C ha−1), we multiplied TB with species-specific carbon factor, where the C factor of 46% was used for forest types in which all conifers collectively constituted more than half of the forest composition. The C was taken as 45% for forest types where conifers and broadleaf species coexisted or where broadleaf species constituted more than half of the total (Manhas et al., 2006). Net change in carbon stock (ΔC) was calculated by subtracting the carbon stock of two consecutive years. Annual accumulation of carbon in the litter was added to ΔC to obtain the carbon sequestration potential (CSP) at each site (Awasthi et al., 2022).
3. Results
3.1. Phytosociological attributes
All riverine forests occurred within a 15–50° slope along the river or stream. A total of 43 tree species belonging to 37 genera and 27 families were recorded along the altitudinal gradient. Among these, most were angiosperms (97.7%). The three forest types identified near the riverine habitat were Macaranga (890–1,500 m), Alnus (1355–1,645 m), and Quercus-Machilus (1865–2,195 m) forest. The tree density was at the maximum in Quercus-Machilus, ranging from 510 to 790 individuals ha−1, followed by Alnus (480,630 individuals ha−1) and Macaranga forest (470–640 individuals ha−1). The total basal area was highest in Quercus-Machilus forest, ranging from 31.4 to 51.4 m2 ha−1, followed by Alnus forest (26.8–39.5 m2 ha−1) and Macaranga forest (11.7–27.2 m2 ha−1). The tree diversity was highest in Macaranga forest site 2 (2.09), and it was lowest in Alnus forest site 1 (0.32). In terms of tree richness, Macaranga forest site 2 had the maximum (14 spp.) and Alnus forest site 1 and 3 (4 spp. each) had the minimum number of species (Table 2).
3.2. Soil properties
Across the sampled sites, soil was slightly acidic in nature, and pH varied between 5.3 ± 0.1 (Alnus site 3) and 6.4 ± 0.1 (Quercus-Machilus site 1). The soil moisture ranged from 17.7 ± 1.9% (Quercus-Machilus site 2) to 31.4 ± 1.1% (Macaranga site 2). The water-holding capacity varied between 28.3 ± 1.6% (Quercus-Machilus site 2) and 33.9 ± 1.5% (Macaranga site 2). The bulk density ranged from 0.39 ± 0.05 to 0.72 ± 0.04 g cm−3 at Macaranga site 3 and Quercus-Machilus site 1, respectively (Table 3).
The organic carbon, total nitrogen, available phosphorus, and available potassium ranged from 1.98 ± 0.11% (Macaranga site 1) to 3.66 ± 0.17% (Quercus-Machilus site 2), 0.16 ± 0.02% (Macaranga site 1 and Quercus-Machilus site 1) to 0.24 ± 0.02% (Alnus site 3), 11.0 ± 1.5 Kg ha−1 (B1) to 22.6 ± 1.2 Kg ha−1 (Macaranga site 2), and 105.4 ± 5.0 Kg ha−1 (Macaranga site 1) to 176.4 ± 13.7 Kg ha−1 (Quercus-Machilus site 3), respectively (Table 3). The soil organic matter and soil carbon stock ranged from 3.4 ± 0.2% (Macaranga site 1) to 6.3 ± 0.3% (Quercus-Machilus site 2) and 8.7 ± 0.7 Mg C ha−1 (Macaranga site 3) to 22.9 ± Mg C ha−1 (Quercus-Machilus site 2), respectively.
3.3. Biomass, litterfall, and net primary productivity
The tree biomass ranged from 256.6–558.1 Mg ha−1 in Macaranga forest to 460.7–485.8 Mg ha−1 in Alnus forest and 508.6–692.1 Mg ha−1 in Quercus-Machilus forest (Table 4). Of the total biomass, the aboveground biomass (544.2 Mg ha−1) and belowground biomass (147.9 Mg ha−1) contributed the maximum in Quercus-Machilus forest site 1. However, aboveground biomass (198.1 Mg ha−1) and belowground biomass (58.6 Mg ha−1) recorded the minimum for Macaranga forest site 1. The dominant tree species in Alnus forest contributed 62–88% to total biomass. However, Macaranga and Quercus-Machilus forest contributed only 23–45% to total biomass. The total annual litterfall ranged from 4.01–5.35 Mg ha−1 yr.−1 in Macaranga forest to 4.99–6.07 Mg ha−1 yr.−1 in Alnus forest and 4.91–6.72 Mg ha−1 yr.−1 in Quercus-Machilus forest (Table 5). Mean litterfall was recorded highest in Quercus-Machilus forest (5.94 ± 0.54 Mg ha−1 yr.−1), followed by Alnus forest (5.57 ± 0.31 Mg ha−1 yr.−1) and Macaranga forest (4.67 ± 0.39 Mg ha−1 yr.−1). The maximum mean annual litterfall was accounted for the summer season (3.00 ± 0.25 Mg ha−1 yr.−1) in Quercus-Machilus forest. However, it was observed at minimum during the winter season (0.76 ± 0.05 Mg ha−1 yr.−1) for Macaranga forest. The net primary productivity ranged from 12.76–14.79 Mg ha−1 yr.−1 in Macaranga forest to 12.93–14.99 Mg ha−1 yr.−1 in Alnus forest and 11.98–18.78 Mg ha−1 yr.−1 in Quercus-Machilus forest. Moreover, the biomass accumulation ratio varied between 20.12 and 57.75 for riverine forests in the sampled sites.
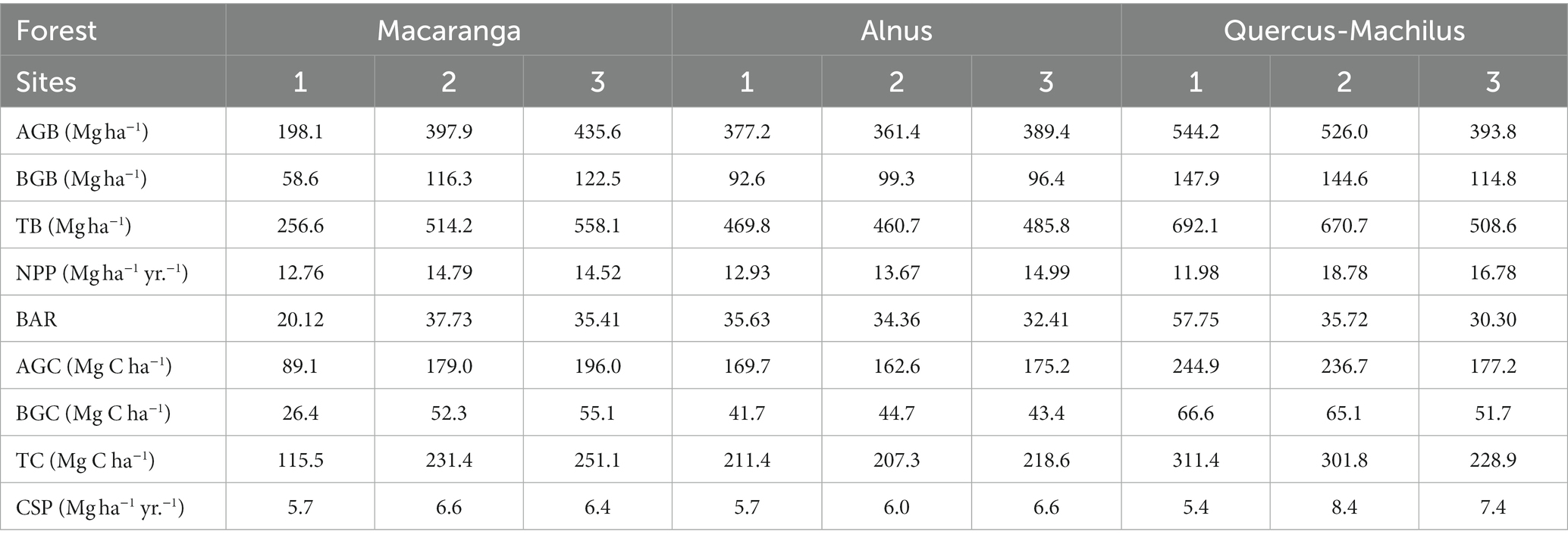
Table 4. Aboveground biomass (AGB), belowground biomass (BGB), total biomass (TB), net primary productivity (NPP), biomass accumulation ratio (BAR), aboveground carbon stock (AGC), belowground carbon stock (BGC), total carbon stock (TC), and carbon sequestration potential (CSP) along the altitudinal gradient in riverine forests.
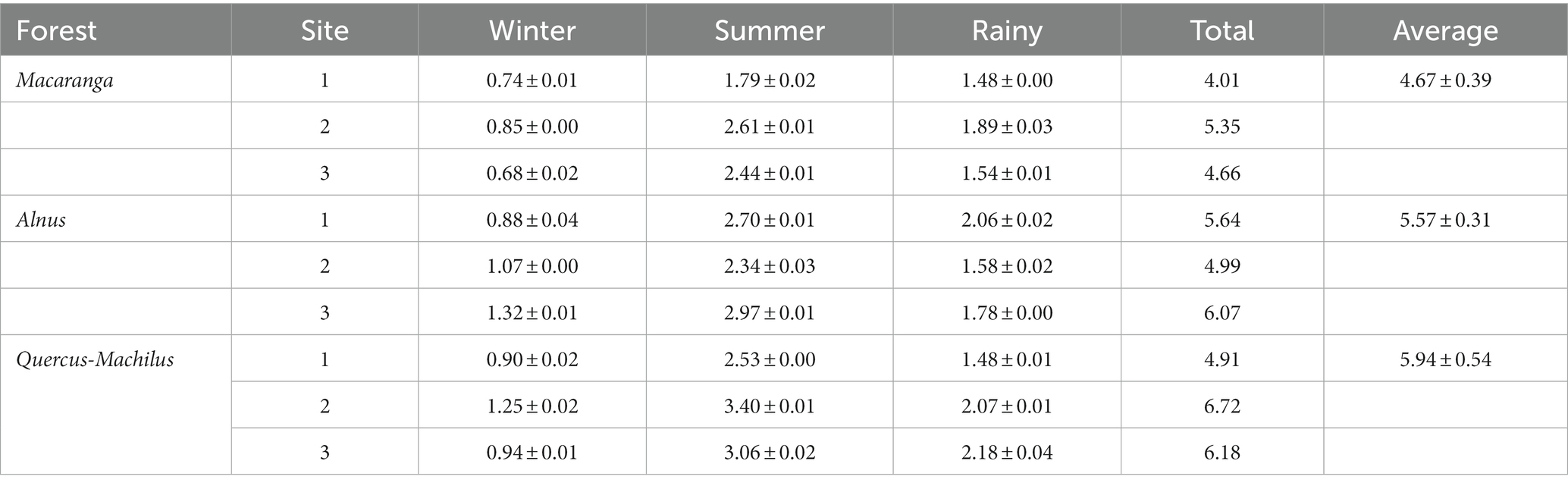
Table 5. Seasonal pattern of litterfall (Mg ha−1 yr.−1) in three distinct sites of different forests.
3.4. Carbon stock and sequestration potential
The carbon stock ranged from 115.5–251.1 Mg C ha−1 in Macaranga forest to 207.3–218.6 Mg C ha−1 in Alnus forest and 228.9–311.4 Mg C ha−1 in Quercus-Machilus forest. Of the total carbon stock, the aboveground carbon was estimated to be highest in Quercus-Machilus forest site 1 (244.9 Mg C ha−1) and lowest in Macaranga forest site 1 (89.1 Mg C ha−1). In addition, belowground carbon was recorded at the maximum in Quercus-Machilus forest site 1 (66.6 Mg C ha−1) and the minimum in Macaranga forest site 1 (26.4 Mg C ha−1). The carbon sequestration potential ranged from 5.7–6.6 Mg C ha−1 yr.−1 (Macaranga forest) to 5.7–6.6 Mg C ha−1 yr.−1 (Alnus forest) and 5.4–8.4 Mg C ha−1 yr.−1 (Quercus-Machilus forest).
4. Discussion
The dry matter dynamics and the carbon flux of the forest are influenced by the structural and functional characteristics of the forest stand. The tree density (r = 0.73) and total basal area (r = 0.71) increased with increasing altitude in the riverine forests. In the present study, the density and total basal area in riverine forests were reported to be higher in contrast to previous studies (Rawal and Pangtey, 1994; Dhar et al., 1997). The richness and diversity across the sites were observed to be lesser than the values reported by Dhar et al. (1997) in Kumaun Himalaya. A higher tree density in Alnus forest was recorded, while slightly lesser tree diversity and richness were recorded when compared with the values recorded by Gairola et al. (2011) in Garhwal Himalaya. In addition, lower tree density, richness, and diversity in broadleaf forest were recorded compared to the values observed by Gairola et al. (2011).
The physico-chemical characteristics of soil vary within space and time due to variation in topography, climate, weathering processes, vegetation cover, microbiological activity, season, and other biotic and abiotic factors (Bargali et al., 2019; Manral et al., 2020). Therefore, soil characteristics vary over relatively small distances throughout the Himalayan region, and species composition plays an important role in the formation of soil organic matter and influences soil-forming processes (Bargali et al., 2018). All the forest communities showed distinct soil physico-chemical characteristics in the present study. It was observed that soil organic carbon was significantly correlated (r = 0.80, p < 0.05) with the total basal area of the forest. The higher concentration of soil nutrients in Alnus forest was due to its nitrogen-fixing ability and nutrient-rich litter, which enhances the physico-chemical and biological characteristics of the soil (Awasthi et al., 2022). Alder, once established in degraded land, improves the physicochemical properties of soil (Perakis and Pett-Ridge, 2019; Joshi and Garkoti, 2021). Moreover, alder develops clustered roots that release phosphorus and carboxylates and other important elements available in the soil (Lambers et al., 2019). However, the soil in broadleaf forests is often deep and fertile and has a thick layer of humus.
Litterfall serves as a conduit between the tree canopy and the soil below, contributing nutrients gathered from the biomass and affecting the productivity of the forest (Pitman, 2013). Seasonality plays a key role in the fluctuation of litterfall. Both anthropogenic and natural factors, including climate change, may have an impact on litterfall output and seasonal progression. In the present study, the lower values of litterfall were due to lower tree density (r = 0.69), lower total basal area (r = 0.72), and other site conditions (Table 6). The amount of litterfall in any ecosystem shows a linear relationship with the canopy cover, tree size, tree density, and basal area of the forest (Navarroa et al., 2013). With an increase in annual litterfall input, the soil organic carbon (r = 0.96) and soil carbon stock (r = 0.69) have significantly increased in the forest sites. The litter of Alnus is nitrogen rich, which promotes soil carbon (Bissonnette et al., 2014; Perakis and Pett-Ridge, 2019) and thus markedly benefits soil fertility. The annual litterfall in the present study was estimated to be higher than the values reported by Sharma and Ambasht (1987) in eastern Himalaya, due to higher values of tree density and total basal area. According to Chen et al. (2014), litterfall peaks in the monsoon and summer seasons for temperate broadleaf forests. The peak litterfall production during the summer season reported in this study is an indication of the tree’s physiological response to increased temperature.
Forest structure and composition had a substantial effect on biomass, carbon stock, and soil organic carbon. The tree biomass of the forest depends upon vegetation structure, species composition, and stand age (Bisht et al., 2022b). In terms of forest communities, Quercus-Machilus forest had the highest aboveground biomass, indicating a greater number of individuals with higher girth class. Moreover, Macaranga forest, with lower density and total basal area, had the lower aboveground biomass in the forest sites. The total biomass in Alnus and Quercus-Machilus forest was recorded to be higher compared to the values recorded by Singh and Singh (1987), Rikhari et al. (1997), Gairola et al. (2011), and Joshi and Garkoti (2021) in Uttarakhand Himalaya, which might be due to variation in species composition and girth class size. The value of tree biomass falls within the range reported by Sharma et al. (1998) in Alnus forest. Moreover, the tree biomass in the present study was recorded to be higher than the riverine Shorea robusta and riverine A. catechu forest of Sharma et al. (2010) and Sajad et al. (2021). In the Himalayan region, very few studies have been conducted on dry matter dynamics of riverine forests.
The net primary productivity is the key indicator of concern from the forest management and sustainability point of view. The productivity of the forest ecosystem depends upon the population dynamics, forest structure, species composition, growth traits, and rate of increment in given environmental and site conditions. The NPP reported for Alnus forest falls within the range reported by Singh and Singh (1987) and Rikhari et al. (1997). Furthermore, a key indicator of the potential age of the dominating species and the harshness of the environment, which measures the accumulation of primary persistent material, is the biomass accumulation ratio (Whittaker, 1975). The BAR in Quercus-Machilus forest was recorded at 41.3 ± 8.4, which was higher than the value (28.3 ± 1.9) reported by Singh et al. (1994) in Kumaun Himalaya. For Alnus plantation, the value falls within the range reported by Sharma et al. (1998). As the altitude rises, the mean value of the BAR increased due to larger amount of biomass production in the riverine forests.
The process of absorbing and exporting carbon varies considerably in forest ecosystems, depending on forest density, soil, and carbon stock. The carbon flux varies with the size, age, and species composition of trees in the forest ecosystem (Covey et al., 2012). The total carbon stock of the Alnus and Quercus-Machilus forest was higher than the values observed previously (Rikhari et al., 1997; Gairola et al., 2011). The carbon stock in the present study was higher than that of the riverine S. robusta and A. catechu forest recorded by Sharma et al. (2010). The significant relation between total basal area and carbon stock (r = 0.85) indicates that an increase in the stand basal area led to an increase in the carbon stock of the forest ecosystem. Carbon sequestration potential showed strong correlation with the tree density of the forest sites (r = 0.81, p < 0.01). According to Gera (2012), the variations in the sequestration potential can be attributed to the mean annual increment, which varies with forest site, age, density, and plantation. The present study reveals that soil is the largest pool of forest carbon, contributing 48.2–50.6%, followed by aboveground carbon (38.7–39.5%) and belowground carbon (10.1–11.6%, Figure 3).
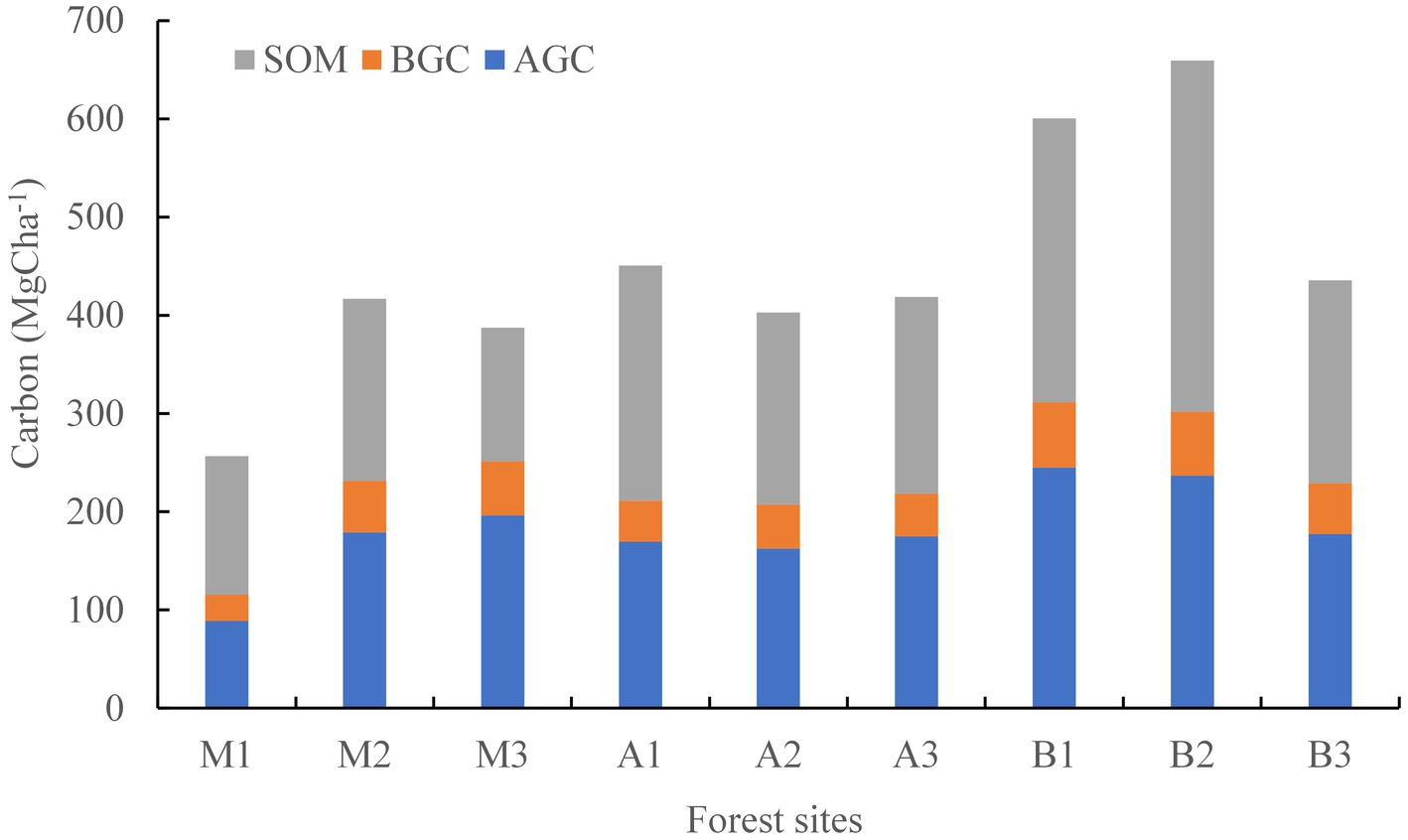
Figure 3. Density of carbon in soil and tree biomass in riverine forests of different sites (M-Macaranga forest, A-Alnus forest, B-Quercus-Machilus forest, and numeric after alphabet denotes site 1, 2, and 3 in each forest type, SOM-soil organic matter, AGC-aboveground carbon, and BGC-belowground carbon; for details, see Table 4).
5. Conclusion
It was observed that as altitude increased, there was a significant increase in both tree density and total basal area within riverine forests. The highest values for these parameters were documented in the Quercus-Machilus forest, while the lowest values were observed in the Macaranga forest. Similarly, tree biomass and productivity exhibited their peak values in the Quercus-Machilus forest (692.1 Mg ha−1) and their lowest values in the Macaranga forest (66.91 Mg ha−1). The annual litterfall demonstrated a seasonal variation, with higher levels during the summer (3.40 ± 0.01 Mg ha−1) and lower levels in the winter (0.74 ± 0.01 Mg ha−1), ranging from 4.01 Mg ha−1 in the Macaranga forest to 6.72 Mg ha−1 in the Quercus-Machilus forest. This investigation unveiled that the Quercus-Machilus forest not only stores a substantial amount of carbon (280.7 ± 26.0 Mg ha−1 yr.−1) but also sequesters carbon effectively (7.1 ± 0.9 Mg C ha−1 yr.−1) compared to the Macaranga and Alnus forests. In conclusion, the research indicates that a greater litterfall and higher forest density in riverine habitats exert a more pronounced influence on soil organic carbon content. Additionally, it was observed that Alnus forests significantly enhanced the nitrogen content in the forest ecosystem, positively affecting soil fertility.
This study contributes to our comprehension of carbon storage and fluxes within riverine forests in the Western Himalaya. Carbon offset programs such as REDD+ offer abundant opportunities and resources for the conservation of these forests, allowing for the retention of carbon reserves while simultaneously delivering supplementary ecosystem services to local communities. Consequently, it is advisable to contemplate the utilization of riverine forests for the restoration of deteriorated sites in the Western Himalaya, and the implementation of alder-based restoration initiatives should be examined as a potential means to enhance carbon storage within ecosystems. This should be viewed as an integral element of climate change mitigation strategies and forest ecosystem management.
Data availability statement
The original contributions presented in the study are included in the article/supplementary material, further inquiries can be directed to the corresponding authors.
Author contributions
SB collected data, performed analysis, and prepared the manuscript. YR and SSB conceptualized. KB and GR finally reviewed the paper. All authors contributed to the article and approved the submitted version.
Funding
NMHS is duly acknowledged for providing funding for the project, Mainstreaming landscape approach for biodiversity conservation, improved livelihoods and ecosystem health in Kailash Sacred Landscape part of India, received by SB and GR.
Acknowledgments
The authors acknowledge the Director and Dean, Wildlife Institute of India, for providing the necessary facilities. The authors thank the Project Coordinator of the project “Mainstreaming Landscape Approach to Biodiversity Conservation, Livelihoods and Ecosystem Health in Kailash Sacred Landscape India” for encouragement. The authors are immensely grateful to the Uttarakhand State Forest Department for their permits to work in the high-altitude zones of the study area and are also thankful to the villagers and field assistants for their help.
Conflict of interest
The authors declare that the research was conducted in the absence of any commercial or financial relationships that could be construed as a potential conflict of interest.
Publisher’s note
All claims expressed in this article are solely those of the authors and do not necessarily represent those of their affiliated organizations, or those of the publisher, the editors and the reviewers. Any product that may be evaluated in this article, or claim that may be made by its manufacturer, is not guaranteed or endorsed by the publisher.
References
Ali, S. N., Biswas, R. H., Shukla, A. D., and Juyal, N. (2013). Chronology and climatic implications of late quaternary glaciations in the Goriganga valley, central Himalaya, India. Quat. Sci. Rev. 73, 59–76. doi: 10.1016/j.quascisrev.2013.05.016
Allan, J. D., Castillo, M. M., and Capps, K. A. (2021). “Carbon dynamics and stream ecosystem metabolism” in Stream ecology: structure and function of running Waters. eds. J. D. Allan, M. M. Castillo, and K. A. Capps (Heidelberg: Springer Netherlands), 421–452.
Anderson, F. (1971). “Methods and preliminary results of estimation of biomass and primary production in south Swedish mixed deciduous woodland” in Proceedings of Brussels symposium on productivity of forest ecosystems. ed. P. Duvigneaud (Paris: Unesco), 281–288.
Arasa-Gisbert, R., Vayreda, J., Rom an-Cuesta, R. M., Villela, S. A., Mayorga, R., and Retana, J. (2018). Forest diversity plays a key role in determining the stand carbon stocks of Mexican forests. For. Ecol. Manag. 415–416, 160–171. doi: 10.1016/j.foreco.2018.02.023
Awasthi, P., Bargali, K., Bargali, S. S., and Jhariya, M. (2022). Structure and functioning of Coraria nepalensis dominated shrublands in degraded hills of Kumaun Himalaya. I. Dry matter dynamics. Land Degrad. Dev. 33, 1474–1494. doi: 10.1002/ldr.4235
Bargali, K., Manral, V., Padalia, K., Bargali, S. S., and Upadhyay, V. P. (2018). Effect of vegetation type and season on microbial biomass carbon in central Himalayan forest soils, India. CATENA 171, 125–135. doi: 10.1016/j.catena.2018.07.001
Bargali, S. S., Padalia, K., and Bargali, K. (2019). Effects of tree fostering on soil health and microbial biomass under different land use systems in central Himalaya. Land Degrad. Dev. 30, 1984–1998. doi: 10.1002/ldr.3394
Becknell, J. M., and Powers, J. S. (2014). Stand age and soils as drivers of plant functional traits and aboveground biomass in secondary tropical dry forest. Can. J. For. Res. 44, 604–613. doi: 10.1139/cjfr-2013-0331
Bisht, S., and Adhikari, B. S. (2014). Dendrobium longicornu: an addition to the orchid flora of Western Himalaya. Richardiana 14, 157–168.
Bisht, S., Bargali, K., Bargali, S. S., Rawat, P. S., and Rawat, Y. S. (2022a). Carbon Pool in Quercus lanuginosa (D. Don) Forest of Askot wildlife sanctuary, Western Himalaya in relation to stand attributes. Indian Forester 148, 709–717. doi: 10.36808/if/2022/v148i7/168610
Bisht, S., Bargali, S. S., Bargali, K., Rawat, G. S., Rawat, Y. S., and Fartyal, A. (2022b). Influence of anthropogenic activities on Forest carbon stocks-a case study from Gori Valley, Western Himalaya. Sustainability 14:16918. doi: 10.3390/su142416918
Bisht, S., Rawat, G. S., Bargali, S. S., Rawat, Y. S., and Mehta, A. (2023). Forest vegetation response to anthropogenic pressures: a case study from Askot wildlife sanctuary, Western Himalaya. Environ. Dev. Sustain. 1, 1–25. doi: 10.1007/s10668-023-03130-2
Bissonnette, C., Fahlman, B., Peru, K. M., Khasa, D. P., Greer, C. W., Headley, J. V., et al. (2014). Symbiosis with Frankia sp. benefits the establishment of Alnus viridis spp. Crispa and Alnus incana spp. Rugosa in tailings sand from the Canadian oil sands industry. Ecol. Eng. 68, 167–175. doi: 10.1016/j.ecoleng.2014.03.06
Bookhagen, B., and Burbank, D. W. (2010). Toward a complete Himalayan hydrological budget: spatiotemporal distribution of snowmelt and rainfall and their impact on river discharge. J. Geophys. Res. 115:F03019. doi: 10.1029/2009JF001426
Brown, S. L., Schroeder, P., and Kern, J. S. (1999). Spatial distribution of biomass in forests of the eastern USA. For. Ecol. Manag. 123, 81–90. doi: 10.1016/S0378-1127(99)00017-1
Cairns, M. A., Brown, S., Helmer, E. H., and Baumgardner, G. A. (1997). Root biomass allocation in the world’s upland forests. Oecologia 111, 1–11. doi: 10.1007/s004420050201
Champion, H. G., and Seth, S. K. (1968). A revised survey of the Forest types of India. New Delhi: Goverment of India Publications.
Chen, H., Gurmesa, G. A., Liu, L., Zhang, T., Fu, S., Liu, Z., et al. (2014). Effects of litter manipulation on litter decomposition in a successional gradient of tropical forests in southern China. PLoS One 9:e99018. doi: 10.1371/journal.pone.0099018
Covey, K. R., Orefice, J., and Lee, X. (2012). “The physiological ecology of carbon science in Forest stands” in Managing Forest carbon in a changing climate. eds. M. S. Ashton, M. L. Tyrrell, D. Spalding, and B. Gentry (Heidelberg: Springer Netherlands), 31–49.
Crisafulli, C. M., Swanson, F. J., Halvorson, J. J., and Clarkson, B. D. (2015). “Volcano ecology: disturbance characteristics and assembly of biological communities” in The encyclopedia of volcanoes. ed. H. Sigurdsson. 2nd ed (Cambridge, MA: Academic Press), 1265–1284.
Curtis, J. T., and McIntosh, R. P. (1950). The interrelations of certain analytical and synthetic phytosociological characters. Ecology 31, 434–455. doi: 10.2307/1931497
Dhar, U., Rawal, R. S., and Samant, S. S. (1997). Structural diversity and representativeness of forest vegetation in a protected area of Kumaun Himalaya, India: implications for conservation. Biodivers. Conserv. 6, 1045–1062. doi: 10.1023/A:1018375932740
Dimri, S., Baluni, P., and Sharma, C. M. (2017). Biomass production and carbon storage potential of selected old-growth temperate forests in Garhwal Himalaya, India. Proc. Natl. Acad. Sci. India B Biol. Sci. 87, 1327–1333. doi: 10.1007/s40011-016-0708-0
Dumka, R. K. (2011). Determination of crustal strain field in Kumaun Himalaya using global positioning system (GPS). Geodesy 13, 978–973.
FSI. (1996). Volume equations for forests of India, Forest Survey of India, Ministry of Environment and Forests, Government of India: Nepal and Bhutan.
FSI. (2021). The state of forest report. Forest survey of India. Dehradun: Ministry of Environment and Forests.
Gairola, S., Sharma, C. M., Ghildiyal, S. K., and Suyal, S. (2011). Live tree biomass and carbon variation along an altitudinal gradient in moist temperate valley slopes of the Garhwal Himalaya (India). Curr. Sci. 100, 1862–1870.
Gera, M. (2012). Poplar culture for speedy carbon sequestration in India: A case study from Terai region of Uttarakhand. ENVIS Forest. Bull. 12, 75–83.
Joshi, R. K., and Garkoti, S. C. (2021). Dynamics of ecosystem carbon stocks in a chronosequence of nitrogen-fixing Nepalese alder (Alnus nepalensis D. Don.) forest stands in the Central Himalayas. Land Degrad. Dev. 32, 4067–4086. doi: 10.1002/ldr.3901
Lal, R. (2015). Restoring soil quality to mitigate soil degradation. Sustainability 7, 5875–5895. doi: 10.3390/su7055875
Lambers, H., Nascimento, D. L., Oliveira, R. S., and Shi, J. (2019). Do cluster roots of red alder play a role in nutrient acquisition from bedrock? Proc. Natl. Acad. Sci. 116, 11575–11576. doi: 10.1073/pnas.1905336116
Manhas, R. K., Negi, J. D. S., Kumar, R., and Chauhan, P. S. (2006). Temporal assessment of growing stock, biomass and carbon stock of Indian forests. Clim. Chang. 74, 191–221. doi: 10.1007/s10584-005-9011-4
Manral, V., Bargali, K., Bargali, S. S., and Shahi, C. (2020). Changes in soil biochemical properties following replacement of Banj oak forest with Chir pine in central Himalaya, India. Ecol. Proc. 9:30. doi: 10.1186/s13717-020-00235-8
Marwah, G., Bisht, S., and Rawat, G. S. (2021). Patterns of distribution among epiphytic orchids and environmental factors in Gori valley, western Himalaya. Orchid Soc. India 35, 159–167.
Merawi, E. (2016). Identification of ecological threats, pressure and their relative severity of Temcha riverine forest, north west, Ethiopisa. Am. J. Agric. For. 4, 64–68. doi: 10.11648/j.ajaf.20160403.12
Mfilinge, P., Atta, N., and Tsuchiya, M. (2002). Nutrient dynamics and leaf litter decomposition in a subtropical mangrove forest at Oura Bay, Okinawa, Japan. Trees 16, 172–180. doi: 10.1007/s00468-001-0156-0
Mueller-Dombois, D., and Ellenberg, E. (1974). Aims and methods of vegetation ecology. New York: John Wiley and Sons.
Naiman, R. J., Bilby, R. E., and Bisson, P. A. (2000). Riparian ecology and management in the Pacific coastal rain forest. Bioscience 50, 996–1011. doi: 10.1641/0006-3568(2000)050[0996:REAMIT]2.0.CO;2
Navarroa, F. B., Romero-Freireb, A., Del Castillob, T., Forondac, A., Jiméneza, M. N., Ripolla, M. A., et al. (2013). Effects of thinning on litterfall were found after years in a Pinus halepensis afforestation area at tree and stand levels. For. Ecol. Manage. 289, 354–362. doi: 10.1016/j.foreco.2012.09.026
Ogino, K. (1977). A beech forest at Ashiu-biomass, its increment and net production. Primary Productivity of Japanese Forests, 172–186.
Olsen, S., Cole, C., Watanabe, F., and Dean, L. (1954). Estimation of available phosphorus in soils by extraction with sodium bicarbonate. U.S.: Department of Agriculture Circular.
Orlov, A. J. (1968). Development and life duration of pine feeding roots. Productivity Studies in Root Systems and Rhizosphere Organisms. USSR, Leningrad, 139–145.
Pan, Y., Birdsey, R. A., Fang, J., Houghton, R., Kauppi, P. E., Kurz, W. A., et al. (2011). A large and persistent carbon sink in the World’s forests. Science 333, 988–993. doi: 10.1126/science.1201609
Perakis, S. S., and Pett-Ridge, J. C. (2019). Nitrogen-fixing red alder trees tap rock-derived nutrients. Proc. Natl. Acad. Sci. 116, 5009–5014. doi: 10.1073/pnas.1814782116
Pitman, R. M. (2013). “Litterfall–biomass, chemistry, leaf area, and links with wider ecosystem functioning” in Developments in environmental science. eds. M. Ferretti and R. Fischer (Amsterdam, Netherlands: Elsevier), 251–264.
Rajpara, M. N., Rajparb, A. H., and Zakaria, M. (2022). Riverine forest as a significant habitat to harbor a wide range of bird species. Braz. J. Biol. 84:e256160. doi: 10.1590/1519-6984.256160
Rawal, R. S., and Pangtey, Y. S. P. (1994). High altitude forest in a part of Kumaun, central Himalaya. Proc. Indian Natl. Sci. Acad. B60, 557–564.
Rawat, G. S. (2017). “The Himalayan vegetation along horizontal and vertical gradients” in Bird Migration in the Himalaya. eds. H. T. Prins and T. Namgail (Cambridge, UK: Cambridge University Press), 189–204.
Rikhari, H. C., Adhikari, B. S., and Rawat, Y. S. (1997). Woody species composition of temperate forests along an elevational gradient in Indian central Himalaya. J. Trop. For. Sci. 10, 197–211.
Sagar, R., and Singh, J. S. (2005). Structure, diversity, and regeneration of tropical dry deciduous forest of northern India. Biodivers. Conserv. 14, 935–959. doi: 10.1007/s10531-004-0671-6
Sajad, S., Haq, S. M., Yaqoob, U., Calixto, E. S., and Hassan, M. (2021). Tree composition and standing biomass in forests of the northern part of Kashmir Himalaya. Vegetos 34, 857–866. doi: 10.1007/s42535-021-00234-w
Shannon, C. E., and Weaver, W. (1949). The mathematical theory of communication. University of Illinois Press, Urbana, USA.
Sharma, E., and Ambasht, R. S. (1987). Litterfall, decomposition and nutrient release in an age sequence of Alnus nepalensis plantation stands in the eastern Himalaya. J. Ecol. 75, 997–1010. doi: 10.2307/2260309
Sharma, C. M., Baduni, N. P., Gairola, S., Ghildiyal, S. K., and Suyal, S. (2010). Tree diversity and carbon stocks of some major forest types of Garhwal Himalaya, India. For. Ecol. Manag. 260, 2170–2179. doi: 10.1016/j.foreco.2010.09.014
Sharma, E., Sharma, R., and Pradhan, M. (1998). Ecology of Himalayan alder (Alnus nepalensis D. Don). Proc. Indian Natl. Sci. Acad. 64, 59–78.
Singh, S. P., Adhikari, B. S., and Zobel, D. B. (1994). Biomass, productivity, leaf longevity, and Forest structure in the central Himalaya. Ecol. Monogr. 64, 401–421. doi: 10.2307/2937143
Singh, S. P., Rawat, Y. S., and Garkoti, S. C. (1997). Failure of brown oak (Quercus semecarpifolia) to regenerate in central Himalaya: a case of environmental semisurprise. Curr. Sci. 73, 371–374.
Singh, J. S., and Singh, S. P. (1987). Forest vegetation of the Himalaya. Bot. Rev. 53, 80–192. doi: 10.1007/BF02858183
Valdiya, K. S. (1976). Himalayan transverse faults and folds and their parallelism with subsurface structures of north Indian plains. Tectonophysics 32, 353–386. doi: 10.1016/0040-1951(76)90069-X
Verma, S., and Jain, A. (2017). Carbon stock in biomass and litter in different plantation of tropical Forest research institute, Jabalpur. J. Tree Sci. 36, 29–33. doi: 10.5958/2455-7129.2017.00003.6
Walkley, A., and Black, C. A. (1934). An examination of Degtjareff methods for determining soil organic matter and a proposed modification of the chronic acid titration methods. Soil Sci. 37, 29–38. doi: 10.1097/00010694-193401000-00003
Wani, Z. A., and Pant, S. (2023). Assessment of floristic diversity and community characteristics of Gulmarg wildlife sanctuary, Kashmir Himalaya. Geol. Ecol. Landsc. 1–21, 1–21. doi: 10.1080/24749508.2023.2196767
Keywords: biomass, litterfall, productivity, riverine, sequestration, volume equation
Citation: Bisht S, Bargali SS, Bargali K, Rawat YS and Rawat GS (2023) Dry matter dynamics and carbon flux along riverine forests of Gori valley, Western Himalaya. Front. For. Glob. Change. 6:1206677. doi: 10.3389/ffgc.2023.1206677
Edited by:
Zishan Ahmad Wani, Baba Ghulam Shah Badshah University, IndiaReviewed by:
Zeeshan Ahmad, Quaid-i-Azam University, PakistanShreekar Pant, Baba Ghulam Shah Badshah University, India
Copyright © 2023 Bisht, Bargali, Bargali, Rawat and Rawat. This is an open-access article distributed under the terms of the Creative Commons Attribution License (CC BY). The use, distribution or reproduction in other forums is permitted, provided the original author(s) and the copyright owner(s) are credited and that the original publication in this journal is cited, in accordance with accepted academic practice. No use, distribution or reproduction is permitted which does not comply with these terms.
*Correspondence: Gopal Singh Rawat, Z3NyYXdhNTlAZ21haWwuY29t; Surendra Singh Bargali, c3VyZW5kcmFraXJhbkByZWRpZmZtYWlsLmNvbQ==