- 1School of Natural Resources and the Environment, University of Arizona, Tucson, AZ, United States
- 2United States Geological Survey, Alaska Science Center, Anchorage, AK, United States
- 3Southwestern Region, USDA Forest Service, Albuquerque, NM, United States
- 4School of Geography, Development and Environment, University of Arizona, Tucson, AZ, United States
Tree loss is increasing rapidly due to drought- and heat-related mortality and intensifying fire activity. Consequently, the fate of many forests depends on the ability of juvenile trees to withstand heightened climate and disturbance anomalies. Extreme climatic events, such as droughts and heatwaves, are increasing in frequency and severity, and trees in mountainous regions must contend with these landscape-level climate episodes. Recent research focuses on how mortality of individual tree species may be driven by drought and heatwaves, but how juvenile mortality under these conditions would vary among species spanning an elevational gradient—given concurrent variation in climate, ecohydrology, and physiology–remains unclear. We address this knowledge gap by implementing a growth chamber study, imposing extreme drought with and without a compounding heatwave, for juveniles of five species that span a forested life zones in the Southwestern United States. Overall, the length of a progressive drought required to trigger mortality differed by up to 20 weeks among species. Inclusion of a heatwave hastened mean time to mortality for all species by about 1 week. Lower-elevation species that grow in warmer ambient conditions died earlier (Pinus ponderosa in 10 weeks, Pinus edulis in 14 weeks) than did higher-elevation species from cooler ambient conditions (Picea engelmannii and Pseudotsuga menziesii in 19 weeks, and Pinus flexilis in 30 weeks). When exposed to a heatwave in conjunction with drought, mortality advanced significantly only for species from cooler ambient conditions (Pinus flexilis: 2.7 weeks earlier; Pseudotsuga menziesii: 2.0 weeks earlier). Cooler ambient temperatures may have buffered against moisture loss during drought, resulting in longer survival of higher-elevation species despite expected drought tolerance of lower-elevation species due to tree physiology. Our study suggests that droughts will play a leading role in juvenile tree mortality and will most directly impact species at warmer climate thresholds, with heatwaves in tandem with drought potentially exacerbating mortality especially of high elevation species. These responses are relevant for assessing the potential success of both natural and managed reforestation, as differential juvenile survival following episodic extreme events will determine future landscape-scale vegetation trajectories under changing climate.
Introduction
Droughts and heatwaves are expected to increase in frequency and magnitude with changing climate (IPCC, 2021), acting in concert to increase tree mortality in forests around the globe (Allen et al., 2015; Hammond et al., 2022). Prolonged regional drought and warming temperatures are expected to lengthen atmospheric dry periods and lead to earlier spring snowmelt, soil moisture deficits, and increased plant evapotranspiration (Abatzoglou and Williams, 2016; IPCC, 2021; Williams et al., 2022). Heatwaves (defined as three or more consecutive days of elevated temperature above the 90th percentile of the seasonal mean) are also projected to become more frequent and intense (Steffen et al., 2014). Globally, one to six extra heatwave days have occurred each decade since 1950 (Perkins-Kirkpatrick and Lewis, 2020). Longest duration heatwaves have also increased, from 5 days in 1950 to an average of 7 days in 2017 (Figure 3B in Perkins-Kirkpatrick and Lewis, 2020). Evidence of tree die-off events related to droughts occurring under warmer baseline temperatures have been observed globally (Allen et al., 2015; Hammond et al., 2022). Additionally, evidence from field studies and plant physiology reviews indicate that heatwaves amplify tree mortality under drought conditions (Niinemets, 2010; Matusick et al., 2018; Ruthrof et al., 2018; López et al., 2022; Werner, 2022).
Within North America, drought and warming are likely to be particularly pronounced in the Southwestern United States as warming temperatures and increased vapor pressure deficit continue to deplete soil moisture and contribute to multi-decadal megadroughts (Cook et al., 2016). This region is currently in a 23-year-long megadrought of anomalously low soil moisture, which is comparable to the longest and most severe drought event in the region since 800 CE (Williams et al., 2020, 2022). Short-duration droughts are more frequent than longer duration droughts, although low precipitation and reduced soil moisture can span weeks to months (Lauenroth and Bradford, 2009). Droughts lasting approximately 5 weeks in duration have occurred more than 60 times in the last 100 years (Figure 3 in Adams et al., 2009). Yet soil moisture can even remain low for an entire year, such as during the 2002 drought in Southwestern woodlands where soil moisture content was measured over 15 years (Figure A1 in Breshears et al., 2009). As drought duration and severity in the Southwest are expected to increase, the same is expected for heatwaves (Figure 3 in IPCC, 2021). End-of-century climate projections in the Southwest (from 10 regionally downscaled CMIP5 models of a 4°C temperature increase) show that heatwave probabilities are expected to shift toward hotter events, with extreme temperatures of about 10°C above current means possible (Figure 3i in Guirguis et al., 2018).
Co-occurring climate stressors such as drought and heatwaves, and associated shifts in fire regimes (along with indirect impacts from insects and pathogens; Simler-Williamson et al., 2019) have the potential to cause tree mortality across broad regions. Large-scale tree mortality events in the Southwest have been observed in response to drought alone (Allen and Breshears, 1998) and to drought and chronic warming (Breshears et al., 2005). Wildfires are an additional, substantial cause of observed tree mortality, with potential for increasing mortality as climate changes heighten wildfire intensity and severity (Allen, 2007, 2014; Falk, 2013; Coop et al., 2016; Singleton et al., 2019). Fire and associated regional drought and warming temperatures have contributed to unprecedented forest loss in historically fire-tolerant ecosystems (Van Mantgem et al., 2013; Abatzoglou and Williams, 2016; Parks and Abatzoglou, 2020; Guiterman et al., 2022). Landscape-scale models incorporating ecosystem-fire processes predict extensive forest loss across landscapes by the end of the century (Loehman et al., 2020), with forested area expected to decline in the coming decades in many areas across the Southwest (McDowell et al., 2016; O’Connor et al., 2020).
Tree mortality at young growth stages is especially relevant to forest recovery following adult tree mortality from wildfires (Stevens-Rumann et al., 2022) and drought-related die-off (Redmond et al., 2018; Batllori et al., 2020). Drought-related tree mortality can alter forest age structure at a broad scale and reset successional progression, opening up resources for competing species and potentially causing woodland to savannah conversions (Clifford et al., 2011) or the replacement of mesic trees with more xeric tree and shrub communities (Batllori et al., 2020). Forest recovery depends first on successful recruitment of new seedlings, and then on the survival of those seedlings to achieve reproductive age (Falk et al., 2022). Successful recruitment of new seedlings can be limited by lack of seed sources or inhospitable growing conditions, which can be especially prevalent in post-disturbance landscapes such as after high-severity fires (Ibáñez et al., 2021; Nolan et al., 2021; Stewart et al., 2021). Establishing seedlings must also outcompete with post-disturbance successional plant communities for nutrients, light, and water (Fenner, 2000). Recent analyzes of forest plots spanning the western U.S. found declining recruitment in post-fire settings, with high sensitivities to water deficits, warmer and drier conditions, and climate extremes (Davis et al., 2023).
Even with successful seedling establishment, post-disturbance tree survival can be precarious for multiple years (White, 1985), and survival may be further impeded by subsequent climate extremes (Kemp et al., 2019; Urli et al., 2023). Survival of juvenile trees can be highly sensitive to temperature-related factors of microclimate, such as vapor pressure deficit (Crockett and Hurteau, 2022) and heat load index (Marsh et al., 2022; Jung et al., 2023). Previous studies have addressed seedling recruitment under changing climate (Davis et al., 2019; Kemp et al., 2019; Bailey et al., 2021; Nelson et al., 2021; Stewart et al., 2021), yet few have examined drivers of mortality in subsequently established juvenile trees (e.g., Rother and Veblen, 2016). In Southwestern woodlands where large-scale tree dieback events have occurred, survival of juvenile trees improved woodland regeneration capacity (Redmond et al., 2018). Focusing of juvenile survival in the face of recurring climate disturbances can further inform the recovery potential of tree species.
Across a landscape, juvenile tree species with differing physiologies occupy different climatic settings, and their responses to climate extremes are dependent on many factors, three of which are intertwined across elevation: (1) species and their ecophysiological adaptations, (2) ecohydrological influences, and (3) temperature influences. First, species vary across an elevational gradient and have specific physiological adaptations to survive in their bioclimatic range. These adaptations, such as stomatal regulation to avoid hydraulic failure or carbon starvation (McDowell et al., 2008), allow species to respond differentially to potential mortality drivers (Koepke et al., 2010). Second, ecohydrological influences refers to how soil moisture links climate and vegetation to describe ecological patterns which vary across landscapes (Rodriguez-Iturbe, 2000). Of particular interest here is how tree structure impacts subcanopy evaporative water loss at an individual tree scale (Royer et al., 2012). Third, temperature varies across elevation, with lower elevations broadly corresponding to warmer temperatures and increased evaporation rates (Haire et al., 2022). Individuals of a species that are acclimated to growing in warm, dry environments are expected to have a broader tolerance for climate extremes such as heat and drought (Ahrens et al., 2021). Yet, other studies suggest that such adaptations do not afford species greater tolerance to heatwaves (Notarnicola et al., 2021; López et al., 2022). Interactions among these concurrently varying factors make predicting responses among multiple species difficult to assess.
Uncertainty in managing forest recovery has prompted extensive studies of seedling regeneration in post-fire landscapes, whether from natural regeneration or replanting from nursery stock (Roccaforte et al., 2012; Chambers et al., 2016; Owen et al., 2017; Ziegler et al., 2017). Without successful tree regeneration, high-severity burned patches in post-fire landscapes are vulnerable to reorganization, including vegetation type conversion, at a landscape scale (Savage et al., 2013; Falk et al., 2022). Outplanting of juvenile trees that have been raised as nursery stock has been implemented strategically to reforest disturbed landscapes in the Southwest (Stevens et al., 2021). Land managers, reforesting landscapes, may be especially interested in juvenile tree responses to climate extremes, considering how outplanting success relates to tree size and species stress tolerance (Andivia et al., 2021). To address how landscapes will respond to disturbances of adult tree die-off from drought and wildfire, we need to understand how juveniles of multiple species that span those landscapes will fare in subsequent expected extreme events, likely including droughts and heatwaves.
To address these issues, our objective was to experimentally assess the relative tolerance of five tree species, at the juvenile growth stage, to the imposition of drought and heatwaves in a controlled environment. The main purpose of this study is to evaluate how different tree species across a landscape gradient will tolerate environmental extremes, measured by their time to mortality. We focused on a suite of species found across elevations in the Jemez Mountains of New Mexico, a model study area for vegetation change in response to drought and warming (Allen and Breshears, 1998; Breshears et al., 2005; McDowell et al., 2019) and wildfire (Haire and McGarigal, 2010; Allen, 2014; Haire et al., 2017; Guiterman et al., 2018, 2022; Coop et al., 2020; Coop, 2023). We designed an experiment to evaluate how prolonged drought, with and without heatwave exposure, may influence juvenile tree survival for five tree species that occur in this area. More specifically, we hypothesized that a protracted drought could trigger differential mortality of tree species, as occurred in 2002 in the Southwest (Gitlin et al., 2006). We asked two primary questions: (1) what are the differences in time to mortality among species under prolonged drought; and (2) does the additional imposition of a heatwave alter the time to mortality within these species? We hypothesized that: (1) species characteristic of different elevation zones would have different responses to drought stress due to a combination of species ecophysiology, ecohydrological influences, and temperature influences; and (2) within species, individuals exposed to a co-occurring stressor of a heatwave in combination with drought would die more quickly than individuals without heatwave exposure. Our approach was to implement growth chamber studies where we imposed extreme drought with and without a compounding heatwave for multiple species spanning an elevational gradient.
Methods
Study area and species selection
We selected species and climate conditions local to the Jemez Mountains, of northern New Mexico, United States (36°N, 106°W), a region that has been the site of numerous studies on drought-and heat-related tree mortality (Allen and Breshears, 1998; Breshears et al., 2005; Rich et al., 2008; Breshears et al., 2009; McDowell et al., 2019). The landscape has also experienced extensive change from uncharacteristic high-severity wildfires over the past several decades (Allen, 2007; Stevens et al., 2021; Guiterman et al., 2022). The Jemez Mountains have a semi-arid continental climate: across the Jemez Mountains, mean annual precipitation (1991–2020 norm) is 564 mm with a largest proportion (214 mm) falling in the summer monsoon season from July to September (Hegewisch and Abatzoglou, 2022). Highest average summer temperatures (7.8 to 24.3°C) and lowest rainfall (27.4 mm) are in June, the most likely time for prolonged drought and heatwave conditions (Hegewisch and Abatzoglou, 2022). Forest soils are composed of volcanic rock and gravel with a loam texture, dating mostly to Pleistocene eruptions that created the dominant physiographic feature of the range, a prominent crater known as the Valles Caldera (Soil Survey Staff, 2002; Muldavin and Tonne, 2003). The elevational range of the Jemez Mountains extends from 1,800 m to 3,500 m, supporting ecological communities across elevations from piñon-juniper woodlands to dense spruce-fir forests (Johnson, 2001). Ecological communities across the mountain range have been exposed to an extensive history of low-intensity fires, documented by tree ring records across the Jemez (Dewar et al., 2021), before being interrupted in the late 1800s by a century of overgrazing and fire suppression (Margolis and Malevich, 2016). Fire deficit in this region and subsequent accumulation of woody fuels, in combination with drier and hotter climate, increased the risk of severe fires (Parks et al., 2015; Singleton et al., 2019). High-severity fires have characterized the landscape in recent decades, raising uncertainty about patterns of forest regrowth and recovery (Stevens et al., 2021; Guiterman et al., 2022).
To compare juvenile tree vulnerability as an indicator of forest recovery potential, we selected five study species that are dominant or co-dominant in mid-to upper-elevation forest communities across the Jemez Mountains. Species were selected both for their relative dominance within elevational zones and for their availability in tree nurseries in the western United States, which are a primary source of plant material for active reforestation management (Landis et al., 2010). As a result, the trees were not sourced from our specific study area, and we could not evaluate the climate conditions of the seed source as a factor in our study. Our selected species were Pinus ponderosa Douglas ex Lawson & C. Lawson (ponderosa pine), Pinus edulis Engelmann (piñon pine), Pinus flexilis E. James (limber pine), Pseudotsuga menziesii Mirbel Franco (Douglas-fir), and Picea engelmanni Engelmann (Engelmann spruce).1 Within the Jemez Mountains, Picea engelmanni occurs most frequently in cooler, wetter sites at upper elevations of 3,000 meters (10,000 ft). Pinus flexilis and Pseudotsuga menziesii occur in slightly drier sites above 2,900 meters (9,500 ft). At the lowest forest elevational range, Pinus ponderosa and Pinus edulis occur in open forests and forest-woodland ecotones, at mean elevations of 2,590 meters (8,400 ft) (Muldavin and Tonne, 2003). Pinus edulis and Pseudotsuga menziesii were purchased from the University of Idaho’s Franklin H. Pitkin Forest Nursery in Moscow Idaho, United States. Picea engelmanni, Pinus ponderosa, and Pinus flexilis were purchased from the Colorado State Forest Service Nursery in Fort Collins Colorado, United States. Trees had well-established root systems and are consequently referred to in this study as juveniles, which represent individuals that have progressed beyond the seedling stage (first one to 2 years of initial growth following seed germination and initial root and shoot development), but prior to the sapling stage (established trees >1 m tall) (Fenner, 2000; National Park Service, 2003; Burrill, 2021). Juvenile trees used in this experiment averaged 4 ± 0.9 years old at the beginning of the experiment (Table 1).
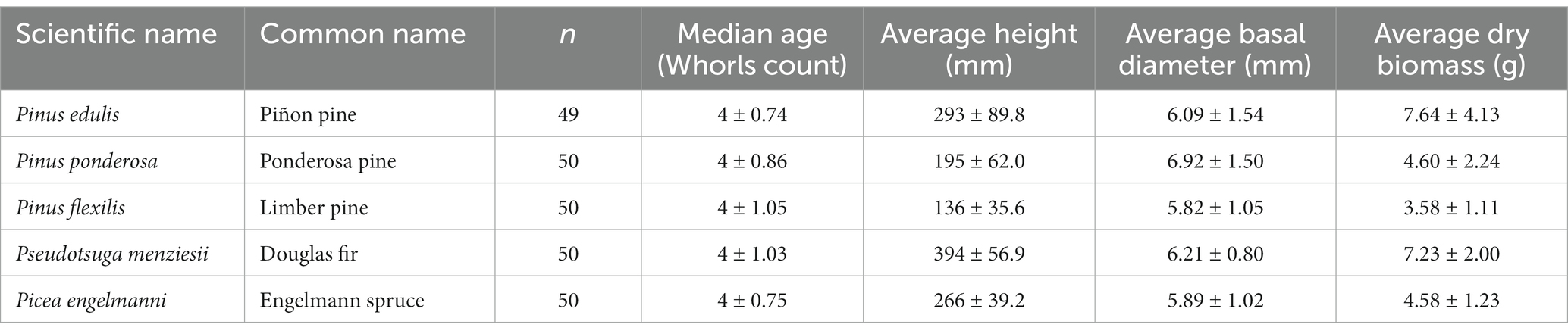
Table 1. Summary of sample size, age, height, basal diameter, and dry biomass of juvenile trees, by species.
Growth chamber parameters
We developed temperature profiles for each species to establish ambient temperature conditions for experimental growth chambers. To calibrate these, we used detailed 1987 vegetation surveys across the Jemez landscape (Allen, 1989) to determine a precise latitudes, longitudes, and elevations for each species. These latitudes and longitudes were then entered into Prism Explorer to retrieve our estimates of the daily mean, minimum, and maximum temperatures of those locations (Climate PRISM Group, Oregon State University); using all the location occurrences in that survey, for each of the species, gave us acceptable estimates of daily temperature profiles that were mimicked in the growth chambers. The accepted 30-year historical baseline that PRISM weather data are modeled from werebetween the years 1970 to 2000, with a spatial resolution of 4 km, for the month of June, historically one of the warmest months for this landscape (Bowen, 1990). We defined our lower-elevation ambient temperature regime where Pinus ponderosa and Pinus edulis co-occur as warm, dry forest types in the Jemez. Where cooler, wetter forests support a mix of Picea engelmanni, Pseudotsuga menziesii, and Pinus flexilis, we defined our higher-elevation ambient temperature regime (Bowen, 1990). Experimental temperatures were set to fluctuate between minimum and maximum temperatures, following a diurnal pattern, and heatwaves were calculated as a 10°C increase over these ambient temperature patterns (Supplemental Figure S1).
Three temperature-controlled plant growth chambers (Conviron, Model E7/2, Pembina North Dakota, United States) were set to temperatures that matched the PRISM data for each species or species group (Table 2). The growth chambers maintained 12 h of visible light (400 to 700 nm wavelengths) at a total output of 7,180 lumens from Agrobrite compact fluorescent light bulbs in each 24-h diurnal cycle. The fourth chamber was used to implement a one-week heatwave for a subset of plants in each chamber (staggered in time as detailed below in Experimental Design). The high elevation group was divided into two chambers, one containing Pinus flexilis and Pseudotsuga menziesii, and the other containing Picea engelmanni. We calibrated chamber temperatures using two Kestrel weather instruments (Kestrel 5,500, Nielsen-Kellerman, United States) placed on either end of each chamber to measure temperature accurately and ensure consistency across chambers. Our Kestrel measurements show the average lower-elevation temperatures ranged from 11.3°C (min) to 27.6°C (max), while the average higher-elevation temperatures ranged from 4.9°C (min) to 19.4°C (max). During the experimental heatwave of 10°C increase, the average lower-elevation temperatures ranged from 18.7°C (min) to 36.3°C (max), and the average higher-elevation temperatures ranged from 13.6°C (min) to 29.1°C (max). Both chambers achieved near identical temperature fluctuations and heatwave increases, with average temperatures deviating 0.3–0.5°C from the target temperatures. We maintained the heatwave chamber to +10°C (actual range 8.9–10°C) above each species’ ambient temperature.
We hypothesized that juveniles of different species would die at varying rates under climate conditions of prolonged drought, and that mortality rates would increase when exposed to an acute heatwave. Timing, duration, and magnitude of heatwave are all variables of potential interest but must be constrained within a single study. Our heatwave parameters (+10°C above ambient temperature for 7 days) are meant to test the extremes of modeled heatwave probabilities in the Southwest (Guirguis et al., 2018). The duration and intensity of our heatwave is consistent with experimental propositions to test heatwaves effects, severe enough to elicit responses in plant mortality but within the realm of realistic probability for expected heatwave events (Perkins-Kirkpatrick and Lewis, 2020; Breshears et al., 2021). We selected a magnitude of 10°C above ambient temperatures with a duration of 1 week, which has been proposed as a target for initial studies of heatwaves to enable cross-study comparisons (Breshears et al., 2021). Currently, heatwaves of this magnitude are occurring across elevations in the Southwest (Zachariah et al., 2023). We implement the heatwave 7 weeks into the study, which approximates a typical interval between peak soil moisture in late spring and when hotter summer temperatures begin to occur in the study area (e.g., for soil moisture dynamics in Pinus edulis woodlands; see Figure A1 in Breshears et al., 2009). This heatwave study expands upon previous studies that tested heatwaves of 5–7°C increase over ambient for 5–6 days (Notarnicola et al., 2021; Marchin et al., 2022). We expand our scope beyond these prior studies to explore heatwave effects on higher-elevation species with lower ambient temperatures (Table 2).
Species preparation
We acquired 50 individuals per species as a target sample size, plus additional plants to account for mortality during transportation and transplanting. All plants arrived in April 2021 and were placed into a temporary electric growth chamber (Conviron, Model CMP3000, Pembina North Dakota, United States) set to a constant 15°C with 12 h of fluorescent light per 24-h diurnal cycle. Plants were watered regularly to maintain non-limiting soil moisture. All individuals were moved from the growth chamber to the greenhouse in May 2021 with a daytime high temperature of 29°C and a nighttime low temperature of 10°C. After 1 month of acclimation to the greenhouse, the plants were repotted into 10 × 10 × 35 cm pots in June 2021. Soil was mixed to roughly replicate an intermediate textured soil type, in terms of sand, silt and clay composition, of the Jemez Mountains, using sandy loam soil (Acme Sand and Gravel, Tucson, AZ, United States) sieved to a gravel size of 2 mm and amended with 30% pure sand and 25% sphagnum peat moss based potting soil (Sunshine Mix #4, Sungro Horticulture, Agawam, MA, United States). To begin the experiment, plants were placed into the experimental growth chambers (Conviron, Model E7/2, Pembina North Dakota, United States) in August and September 2021, using a staggered-in-time design as described below.
Experimental design
To implement the experiment, 50 individual plants per species were assigned randomly to two treatment groups: a temperature treatment, and a water treatment. Within the temperature treatment group, 25 individuals per species were assigned randomly to absence or occurrence of a heatwave. Plants remained under ambient temperature conditions within their growth chamber, except that individuals within the heatwave treatment were exposed to a 10°C increase above ambient temperature for 7 days during week seven of the experiment before being returned to ambient temperature conditions. In each temperature treatment, the majority (n = 20) received no additional water for the duration of the experiment, while a subset of individuals (n = 5) was watered to saturation every 2 weeks to enable us to confirm that drought was driving juvenile mortality.
We used a staggered-in-time design to expose each species to a heatwave during week seven, given our restriction that only a single chamber was available for implementing heatwaves. The start of the experiment was staggered by 2 weeks between each of the other three chambers, meaning that “Week 1” of the experiment corresponds to different calendar dates for each chamber. This allowed sufficient time and space to prepare the heatwave chamber for each round of heatwaves. Plants were placed into the growth chambers in August and September 2021. All plants were watered and fertilized at the beginning of the experiment (Week 0), and measurements began on Week 1. Fertilizer was applied as instructed: about 29.6 grams of Liquinox All-Purpose Fish Fertilizer (NPK 5–1-1) mixed with 3.78 liters of water. Each plant was watered to saturation and then 0.3 liters of fertilizer mixture was sprayed onto the soil with a chemical sprayer. During Week 3, containers with 297 grams of calcium chloride desiccant (Damp Rid, W.M. Barr, Memphis, TN, United States) were used to offset the humidity increase associated with watering treatments. Two desiccant containers per species were placed in each chamber, monitored weekly and refilled as needed. At Week 5, the foliage of all plants was saturated with insecticidal soap (Bondie Products LLC, Oriskany, NY, United States), widely used in greenhouse and growth chamber experiments, to treat a mild occurrence of scale insect (Adelges sp.) [Hemiptera: Adelgidae] mostly confined to the Pinus ponderosa juveniles.
Measurements
Tree size was characterized by taking single measurements of height (mm), basal diameter (mm), and dry weight biomass (g); these were recorded over the course of the experiment (Table 1). Age was estimated using branch whorl counts (Husch et al., 2003). Height was measured from the soil surface to the apical bud, and basal stem diameter was measured with a metric caliper at the base of each tree. Biomass was measured at the end of the experiment once all trees had died. Individuals were clipped at the soil surface, placed into labeled paper bags, set in a drying oven (Yamato, Model DX 402, Santa Clara, California, United States) set at 75°C for 24 h, and weighed.
We took weekly measurements capturing three key potential indicators of plant stress: (1) water availability, (2) stomatal conductance, and (3) leaf browning assessed using ocular estimates and digital photography.
First, to measure water availability and soil water loss, we weighed plants gravimetrically (grams) each week (Eamus, 2006). Weight data was used to calculate the amount of water available to the plant each week, graphically represented as a curve showing water weight in grams over time. Water stress was calculated as an inflection point along this curve, reflecting an estimate of when water availability transitions from relatively non-limiting to relatively limiting (Van Genuchten, 1980). Inflection points were calculated using R to compute the second derivative of the curve to find changes in concavity. Curves were inspected visually to verify the date of the inflection point.
Second, we measured stomatal conductance (mmol m−2 s−1) weekly on the uppermost needles of a subset of juveniles (n = 3 watered, 10 droughted) using a leaf porometer (Model SC-1, Meter group, Pullman, Washington, USA) as an indicator of plant transpiration. Low conductance measurements indicate reduced plant transpiration (Marchin et al., 2022). Because conductance measurements of non-conducting tissue (dry filter paper) recorded a minimum value of 90 mmol m−2 s−1, we assumed that plants had reached permanent stomatal closure when conductance was consistently at or below this minimum value.
Third, to establish a mortality date, we visually inspected leaf color twice per week to record leaf browning. Species tolerance to mortality drivers can be detected observationally by leaf browning trends, reflecting chlorophyll degradation and loss of photosynthetic capacity (Myneni et al., 1995; Jong et al., 2012). As a metric of mortality, foliar color has been shown to relate to hydraulic failure (Blackman et al., 2019). Ocular estimates of 90% brown thresholds have been used as a benchmark to determine plant death in other mortality studies (Adams et al., 2009, 2017). We categorized percent brown as 10%, 25%, 50%, 75%, or 90% brown. Studies have also found that leaf browning among Pinus species can lag behind hydraulic failure-induced mortality, showing that leaf color change is a delayed indicator of tree death (Blackman et al., 2019; Hammond et al., 2019). We similarly observed that many plants retained their green color even while exhibiting other signs of severe tissue degradation. To best establish time of death, we supplemented our percent brown estimates with criteria related to needle loss and brittleness (needles that broke under slight pressure). Plants were declared dead when they reached a 90% threshold for percent brown, needle loss, or brittle needles. We continued to measure individuals for 2 weeks after their death date to confirm each mortality event; the experiment concluded when all droughted plants died, given that watered plants were not expected to die. As a visual record of plant death, photographs were taken weekly against a grey background in the same position and with similar lighting (white balance of 3,200–3,400 K) using a digital camera (Sony Alpha 6,000 mirrorless, 24.3-megapixel 16–50 mm zoom lens). Further image analysis might enable more consistent, quantitative, repeatable, and precise metrics to assess color continuum change from green to brown (e.g., Hammond et al., 2019).
Statistical analyzes
We analyzed significant differences in time to mortality within each species using two-sample t-tests in two contexts. First, we verified our control group to ensure that juvenile death was attributed to drought rather than chamber conditions, testing for differences between watered and droughted juveniles within each species. Second, we tested for differences in heatwave effects among droughted juveniles within each species. We visually inspected for normality and used Shapiro–Wilk tests to compare raw and transformed data; we determined that untransformed data met the assumptions of normality (Shapiro and Wilk, 1965). We performed statistical tests with and without outlier data points; because outliers did not change statistical conclusions, we included outliers in the final analysis. Using a two-sample t-test, we evaluated the null hypothesis that juveniles within a species would respond equally to drought, with or without heatwave exposure. The alternative hypothesis is that juveniles with exposure to a heatwave would have more rapid mortality rates. Because juveniles with heatwave exposure were expected to die more quickly on average, a one-sided value of p was used to evaluate significance (alpha = 0.05).
We used a one-way ANOVA to test significance of time to mortality among species. We compared individuals within drought-only treatment groups, and separately compared individuals within drought-heatwave treatment groups. We compared sample sizes and variance of data to determine that our data fit ANOVA assumptions. Data were analyzed using three measures of time to mortality, as indicators of drought stress: time to mortality (in weeks) since (1) water restriction, at the beginning of the experiment; (2) water stress, calculated from weight data at the water loss inflection point; and (3) permanent stomatal closure, when conductance data consistently reached a minimum. Across these three measures, we evaluated the null hypothesis that all species would respond equally to drought and have the same time to mortality. The alternative hypothesis is that at least one species mortality rate would differ. Given that mortality rates differed among species, further post-hoc analysis of species differences were tested using Tukey’s honest significant difference (HSD). We graphed predicted survival probability against observed values using the R package survival to produce a Kaplan–Meier curve, a nonparametric estimation of survival (Kaplan and Meier, 1958).
All statistical analyzes and visualizations were completed using R Statistical Software, version 4.2.1 (R Core Team, 2022; Therneau, 2022) and figures were produced using the package ggplot2 (Wickham, 2016). All code is available on publicly available GitHub pages.2
Results
Survival of watered juveniles
Our experimental focus was on drought effects with and without a heatwave, so we used our set of watered juveniles as a control group to confirm drought effects as the driver of mortality. We followed the progression of juvenile death until 100% of droughted individuals reached mortality. In the time that all droughted individuals had died, watered juveniles of four species (Picea engelmannii, Pinus edulis, Pinus flexilis, and Pseudotsuga menziesii) had 0% mortality. One of five watered Pinus ponderosa individuals died in both the ambient and heatwave groups. The number of juveniles that died during the experiment was statistically different between watered and droughted Pinus ponderosa juveniles (two-sample t-test, t48 = −12.39, one-sided p < 0.0001). Collectively, these results validate that conditions within the growth chambers were suitable for juvenile growth if water was not limiting.
Species differences in time-to-mortality during drought without a heatwave
We focused our primary analysis on species responses to prolonged drought. Under ambient temperatures and drought conditions, average time to mortality differed among species (analysis of variance F-test, F4,94 = 256.6, p < 0.0001). Pinus ponderosa tree juveniles died most quickly (ӯ = 10.7 weeks, SD = 1.71, n = 20), followed by Pinus edulis (ӯ = 14.6 weeks, SD = 2.17, n = 19), Picea engelmannii (ӯ = 19.2 weeks, SD = 1.51, n = 20), Pseudotsuga menziesii (ӯ = 19.4 weeks, SD = 2.31, n = 20), and Pinus flexilis (ӯ = 30.3 weeks, SD = 2.39, n = 20). All species were significantly different from each other, except for Picea engelmannii and Pseudotsuga menziesii (post-hoc Tukey HSD test; Figure 1). Using a Kaplan Meier survival function, we plotted a predicted survival probability curve against observed values for each species (Figure 1). These contrasts did not change when analyzing species under the drought-heatwave treatment (post-hoc Tukey HSD test; Supplemental Figure S2; Supplemental Table 1A), indicating that drought was the primary driver of mortality rather than heatwave exposure.
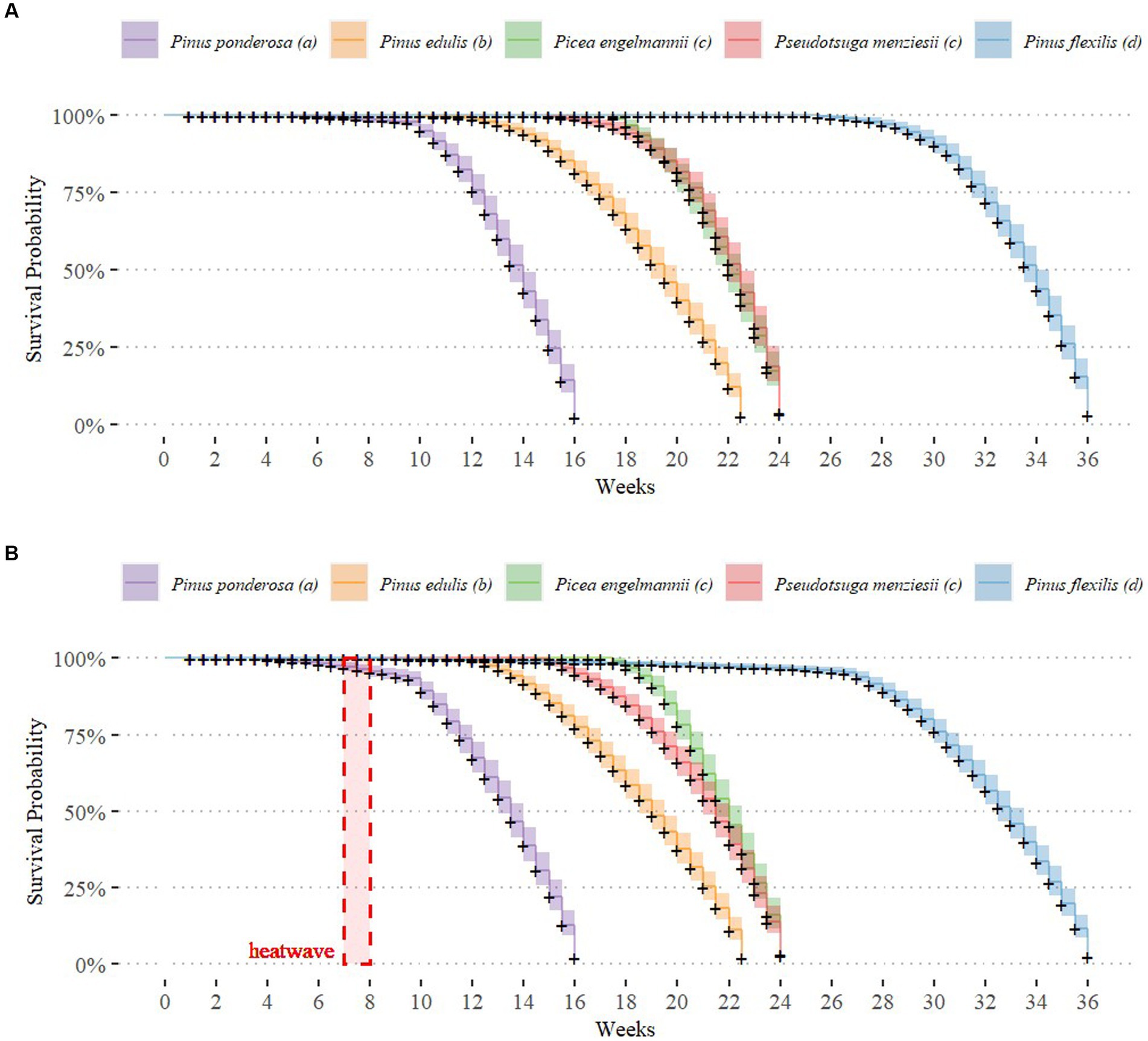
Figure 1. Kaplan Meier Survival Curve of Juvenile Survival Probability under Drought. Survival probability of each species under ambient (A) and heatwave (B) temperature treatments (n = 20 per species). Weeks show time since the start of the experiment, adjusted to account for staggered start times. Survival probability is estimated from observed data using a Kaplan Meier survival function. Letters in the legend (a, b, c, d) indicate significant differences among species (post-hoc Tukey HSD test, p < 0.05).
Heatwave effects
Our secondary analysis was focused on species responses to a weeklong heatwave event overlapping the experimentally imposed drought. Among all species, the mean time to mortality was earlier among juveniles with heatwave exposure (0.3 to 2.7 weeks earlier than drought-only juveniles), although this difference was not statistically significant for either of the lower-elevation species (Pinus ponderosa, Pinus edulis; Figure 2).
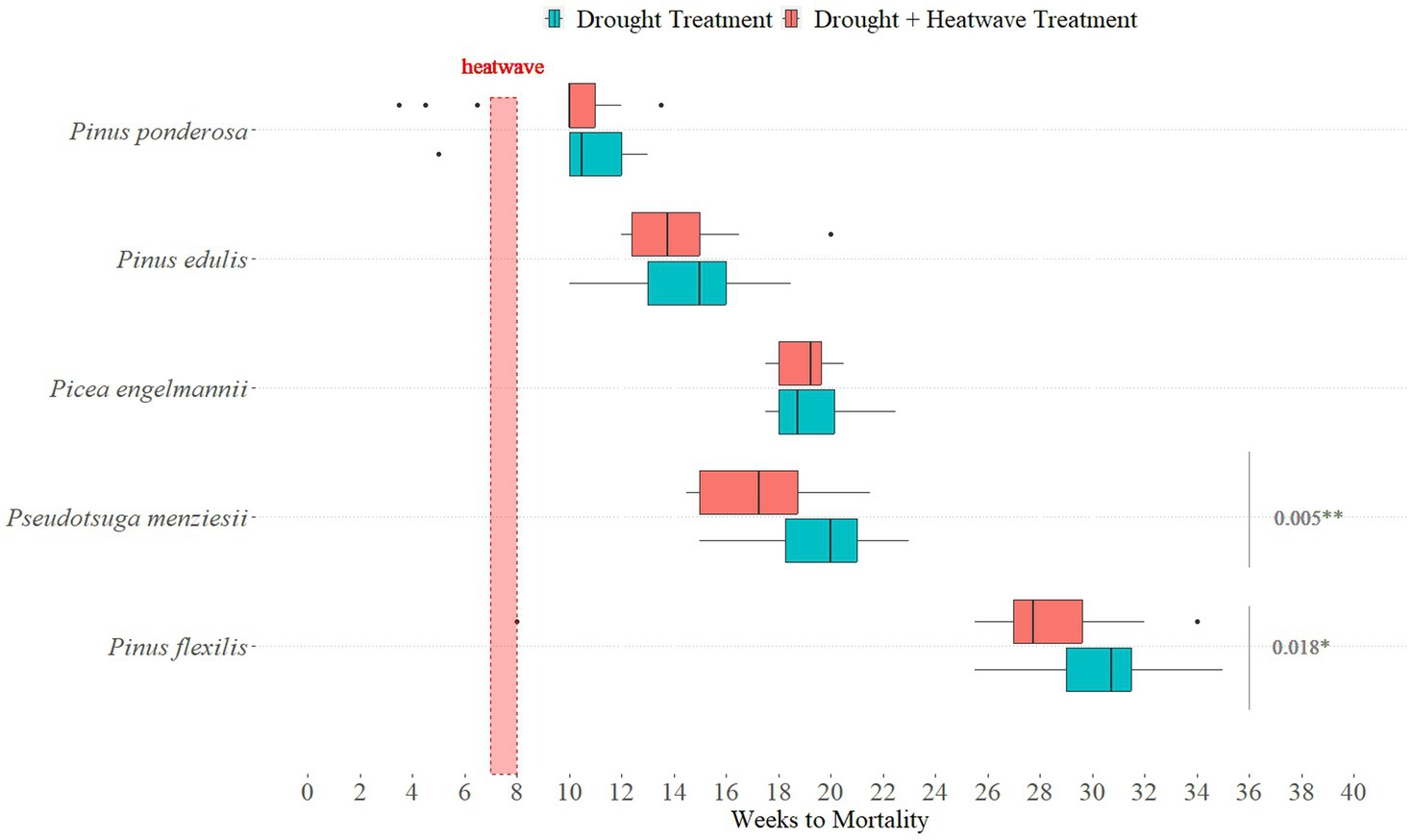
Figure 2. Heatwave Differences of Droughted Juveniles. Half the droughted individuals (n = 20 per species) were exposed to a week-long heatwave (indicated by the vertical red box). Boxplots show median time to mortality (rather than the mean). Grey bars on the right-hand side show value of ps of species which are significantly different in their mean time to mortality (two-sample t-test, p < 0.05).
Two of three higher-elevation species (Pinus flexilis, Pseudotsuga menziesii) exposed to a heatwave had a statistically significant shorter time to mortality (Table 3; Figure 2). Pinus flexilis individuals with a heatwave treatment (ӯ = 27.6, SD = 5.05, n = 20) died an average of 2.7 weeks before those with no heatwave treatment (ӯ = 30.3, SD = 2.39, n = 20; two-sample t-test, t38 = −2.16, one-sided p = 0.018), and Pseudotsuga menziesii juveniles with a heatwave treatment (ӯ = 17.4, SD = 2.39, n = 20) died an average of 2.0 weeks before those with no heatwave treatment (ӯ = 19.4, SD = 2.31, n = 20) (two-sample t-test, t38 = −2.69, one-sided p = 0.005).
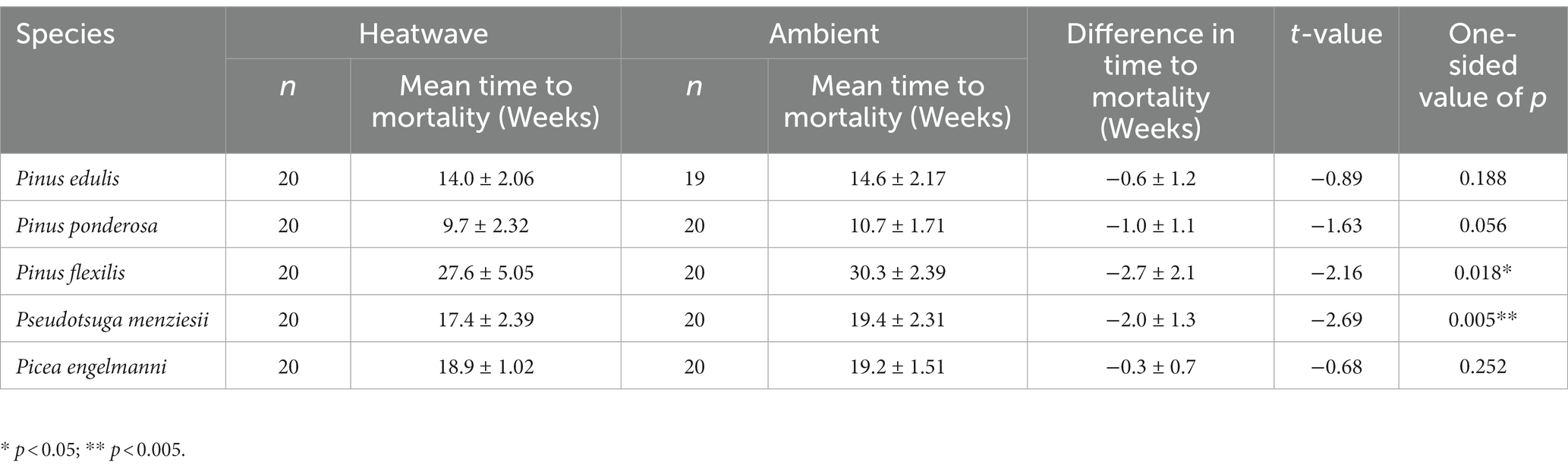
Table 3. Results from two-sample t-test of temperature effects on species (ambient and heatwave treatment groups).
Time to mortality adjusted to metrics of stress onset
Across all species, we found that water stress was the earliest stress indicator, followed by stomatal closure 2 to 4 weeks later (Figures 3, 4). Under ambient temperatures and drought conditions, time to mortality from water stress differed significantly among species (analysis of variance F-test, F4,94 = 103.1, p < 0.0001, post-hoc Tukey HSD test). Following the water stress inflection point, Pinus ponderosa juveniles died most quickly (ӯ = 6.5 weeks, SD = 1.77, n = 20), followed by Pinus edulis (ӯ = 10.5 weeks, SD = 2.89, n = 19), Picea engelmannii (ӯ = 11.7 weeks, SD = 1.41, n = 20), Pseudotsuga menziesii (ӯ = 11.9 weeks, SD = 1.45, n = 20), and Pinus flexilis (ӯ = 19.0 weeks, SD = 2.15, n = 20). Using a Kaplan Meier survival function, we plotted a predicted survival probability curve against observed values for each species (Supplemental Figure S2). The order species died did not change when adjusted for water stress, although Pinus edulis was not significantly different when compared to Picea engelmannii and Pseudotsuga menziesii (post-hoc Tukey HSD test; Supplemental Table 1B). By normalizing water stress, we expected species differences to diminish between lower-elevation species and higher-elevation species, anticipating that lower-elevation species would show greater drought tolerance. This effect is shown for Pinus edulis but not Pinus ponderosa, which maintained significantly earlier death dates compared to all other species.
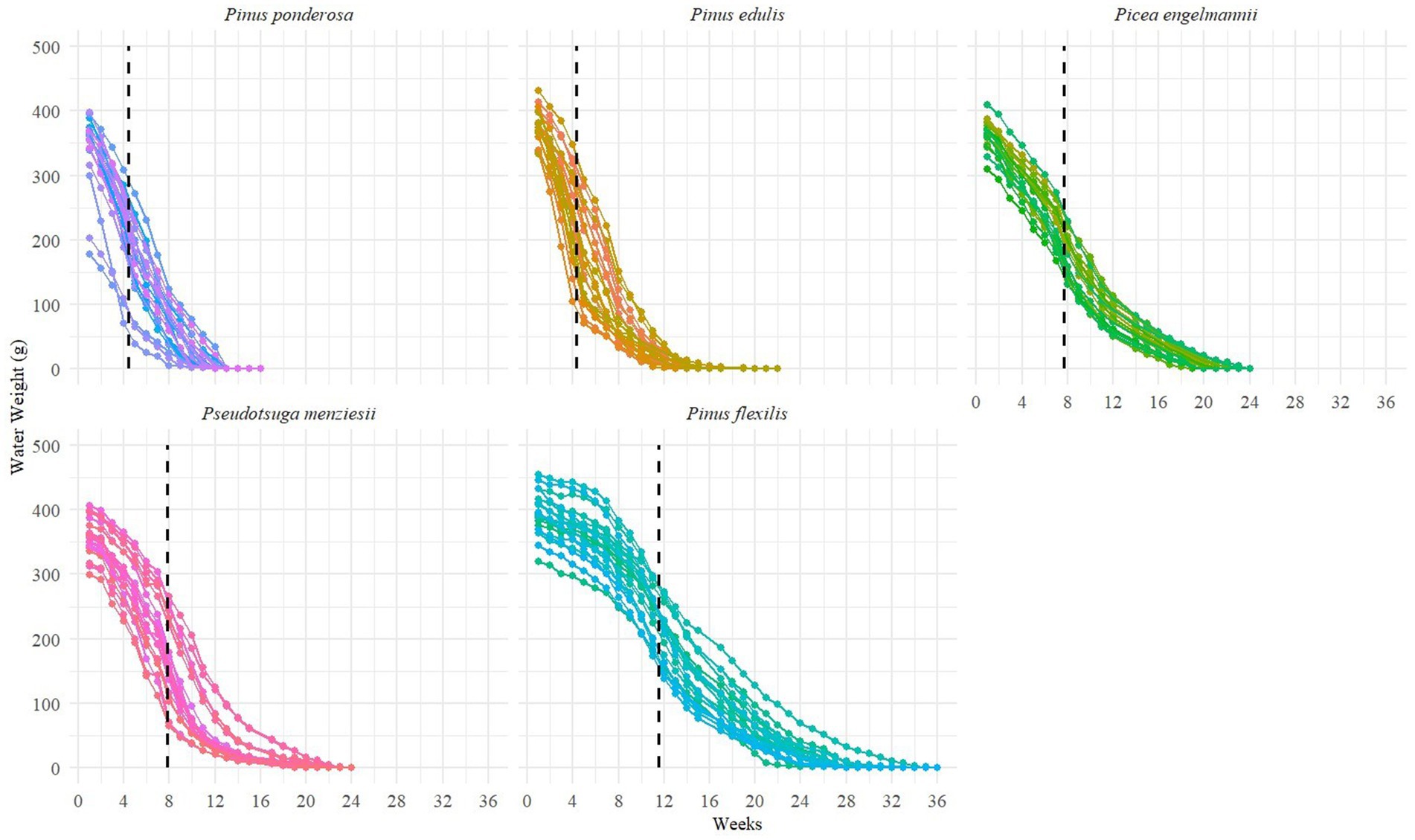
Figure 3. Water Weight of Juveniles under Droughted and Ambient Treatments. Curves were calculated using individual weight data (n = 20 per species). Black dotted lines show the average inflection point among all curves to show where concavity changes from concave up to concave down, reflecting the time when water availability transitioned from a non-limiting to a limiting resource.
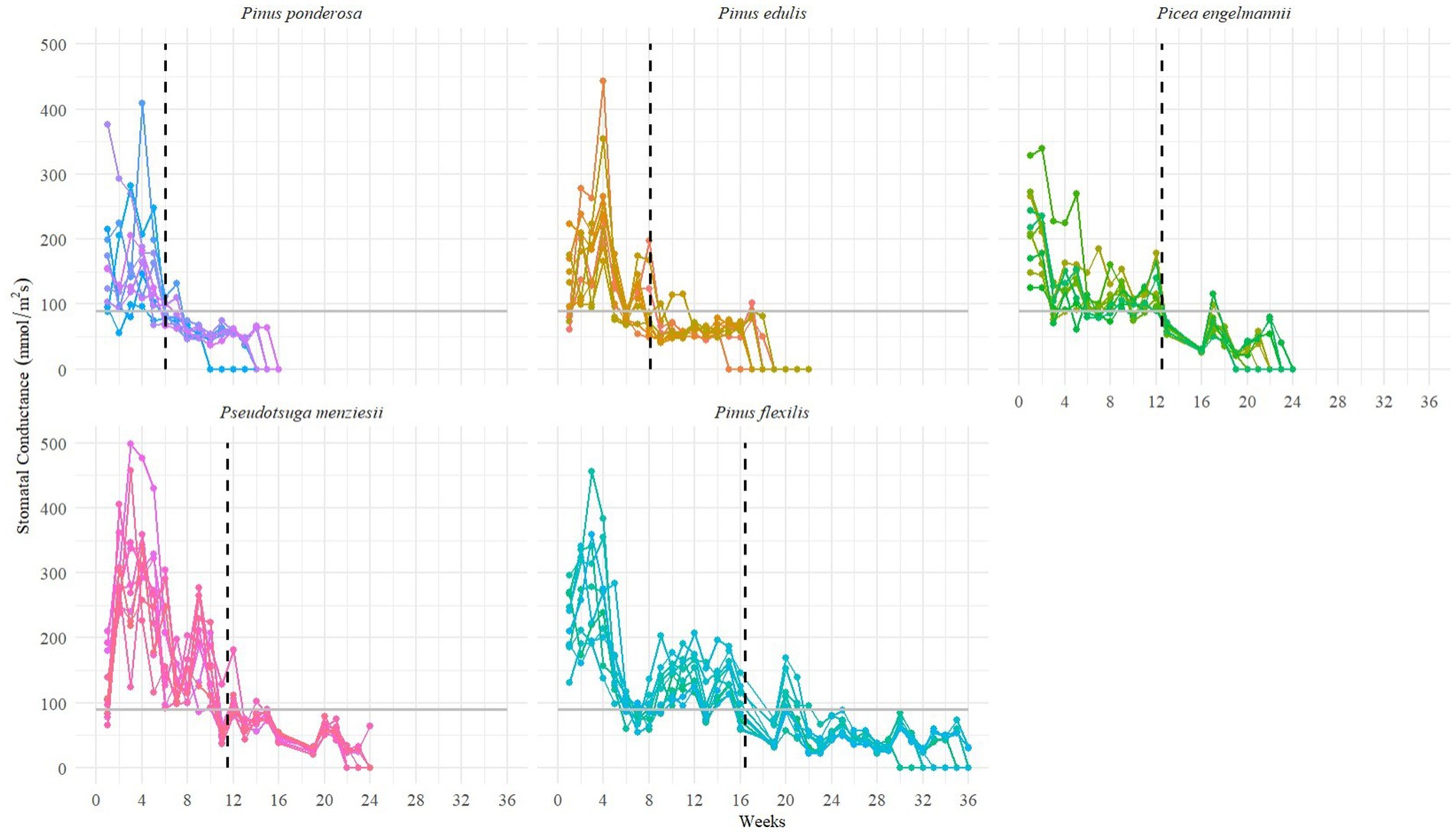
Figure 4. Stomatal Conductance of Juveniles under Droughted and Ambient Treatments. The black dotted line shows the average time of permanent stomatal closure (n = 10 per species), when measurements were consistently at or below a minimum value of 90 mmol m−2 s−1 (solid gray horizonal line).
Under ambient temperatures and drought conditions, the time to mortality from stomatal closure also differed significantly among species (analysis of variance F-test, F4,45 = 27.1, p < 0.0001). Pinus ponderosa tree juveniles died most quickly (ӯ = 5.0 weeks, SD = 1.83, n = 10), followed by Pinus edulis (ӯ = 6.8 weeks, SD = 2.44, n = 10), Picea engelmannii (ӯ = 7.0 weeks, SD = 1.94, n = 10), Pseudotsuga menziesii (ӯ = 8.1 weeks, SD = 1.29, n = 10), and finally Pinus flexilis (ӯ = 14.2 weeks, SD = 2.86, n = 10). Using a Kaplan Meier survival function, we plotted a predicted survival probability curve against observed values for each species (Supplemental Figure S3). The order in which species died did not change when adjusted for stomatal closure stress, although Pinus ponderosa became non-significantly different compared to Pinus edulis and Picea engelmannii (post-hoc Tukey HSD test; Supplemental Table 1C). By normalizing for stomatal closure stress, we expected species differences to diminish even further. This effect is shown for Pinus edulis and Pinus ponderosa as they became more indistinguishable from Picea engelmannii and Pseudotsuga menziesii. However, these four species remained significantly different from Pinus flexilis after we adjusted for metrics of stress onset.
Notably, species contrasts did not change when analyzing species under the drought-heatwave treatment (post-hoc Tukey HSD test; Supplemental Tables 1B,C). Except for stomatal closure stress comparisons between Pinus ponderosa and Pinus edulis, all significant species differences under drought conditions were also significant under heatwave conditions. This further indicates that drought was the primary driver of mortality rather than heatwave exposure.
Across all levels of analysis–with and without adjusting to time of onset of stress–Pinus ponderosa died significantly earlier, and Pinus flexilis died significantly later, than most other species (post-hoc Tukey HSD test; Supplemental Tables 1A,C). Adjusting the time to mortality by stress points or stomatal closure did not change the order in which species died. The species most vulnerable to drought was Pinus ponderosa, followed by Pinus edulis, Picea engelmannii, Pseudotsuga menziesii, and Pinus flexilis.
Discussion
Species differences in juvenile vulnerability to drought
The ability of juvenile trees to survive prolonged drought is a key determinant of forest regeneration capacity, informing future forest composition under extreme climate conditions and following severe disturbances (Tepley et al., 2017; Stevens-Rumann and Morgan, 2019; Stevens-Rumann et al., 2022). We implemented an experimental drought which lasted until all individuals had died, exploring the bounds of how long juvenile trees can persist under conditions of extended drought compounded by a heatwave. Our study indicates substantial differences in species vulnerabilities to drought at the juvenile life stage. The length of a lethal drought for lower-elevation species under warmer ambient conditions was short: 11 weeks for juvenile Pinus ponderosa and 14 weeks for Pinus edulis. Higher-elevation species under cooler ambient conditions did not reach mortality until much later: 20 weeks for Pseudotsuga menziesii and Picea engelmannii, and 30 weeks for Pinus flexilis.
Droughts of this duration are within the range of climatic episodes that have occurred in recent decades (e.g., Late 2001–2002; Breshears et al., 2009), and are projected for an altered-climate future. A 10-week drought strong enough to induce widespread mortality of Pinus ponderosa in the Southwest occurred approximately 30 times in the last century (Figure 2B in Adams et al., 2017). Yet shorter droughts are more frequent than longer droughts, and a 30-week drought, such as needed to induce widespread mortality of our Pinus flexilis species, might occur once a century (Figure 2B in Lauenroth and Bradford, 2009; Adams et al., 2017). Thus, species such as Pinus ponderosa may be more vulnerable to drought-induced mortality due to the greater likelihood of shorter droughts, especially at the juvenile life stage. Notably, the drought conditions leading up to the die-off event documented in our study area for adult trees in 2002 (Breshears et al., 2005) had anomalously low precipitation for almost all 52 weeks of that year (Figure A1 in Breshears et al., 2009), highlighting the potential for a protracted drought as in our experiment. Droughts within a single year may additionally be compounded by interannual drought such as in the Southwest U.S., which is currently in a 23-year megadrought matching both the length and duration of the worst drought in the past 1,200 years (Williams et al., 2022).
Species differences in juvenile heatwave vulnerability
Heatwave impacts on juvenile trees in our study were relatively minor, yet consistent. Across all species, the average time to mortality was faster among individuals with heatwave exposure. For two high elevation species–Pinus flexilis and Pseudotsuga menziesii–this difference was statistically significant, accelerating average time to mortality by 2 to 3 weeks. Despite observations of heatwaves having initiated large tree mortality events (Ruthrof et al., 2018), crown dieback of trees (Matusick et al., 2018), and reduced productivity (Ciais et al., 2005; Young et al., 2022), this study found that the impact of a heatwave was small relative to the duration of the drought, perhaps because some individuals were already experiencing physiological stress from drought at that point. We initiated our heatwave at week seven during the dry-down process; at this time point, only Pinus ponderosa had progressed to stomatal closure, and the three higher-elevation species had not reached the soil moisture inflection point. Thus, our results suggest that the timing and duration of a heatwave could determine its effect on time to mortality among species (Breshears et al., 2021). A heatwave alone did not trigger mortality in watered juveniles.
Hotter temperatures during drought pose a triple threat to plants: amplification of atmospheric drought (or increased vapor pressure deficit), intensified soil drought, and direct effects of heat stress (Hammond et al., 2022). Related research on survival of juveniles in a post-fire landscape of the Jemez Mountains found that temperature-related metrics such as Heat Load Index were particularly influential in predicting seedling survival among species including Pinus ponderosa, Pinus edulis, and Pseudotsuga menziesii (Marsh et al., 2022). Other studies report that heatwaves do not directly damage leaf tissue, but instead intensify drought conditions (De Boeck et al., 2010; Salomón et al., 2022) which is consistent with our findings.
Concurrent influences of ecophysiology, ecohydrology, and site temperature
Differences in time to mortality among species at the juvenile stage are a complex emergent response resulting from a multitude of factors. Three intertwining factors–those which vary concurrently across elevation–deserve attention as to how they played a role in this study: (1) species-specific vulnerability to embolism during drought, (2) the associated ecohydrological response (for example, how that climate and the juvenile canopy structure affect soil evaporation), and (3) the climate conditions to which juvenile trees are exposed. Our experiment was not designed to isolate these three factors; rather, they inherently covary as part of our design and merit discussion.
Embolism in response to protracted drought is a key driver of plant mortality (Choat et al., 2012; Adams et al., 2017; McDowell et al., 2019, 2022). Drought-stressed plants may undergo cavitation in their xylem cells, disrupting their water transport system (Choat et al., 2018; McDowell et al., 2022). A common metric of drought tolerance is Ψ50, the water potential of a plant when 50% of its water transport system has been disrupted by cavitation (Choat et al., 2012; Klein, 2014). Species with a more negative Ψ50 value can tolerate more extreme drought conditions before losing hydraulic function. Research on Ψ50 values suggests that, among our study species, Pinus ponderosa would have the greatest vulnerability to drought, followed by Pseudotsuga menziesii, Pinus flexilis, Pinus edulis, and finally Picea engelmannii (Domec and Gartner, 2001; Choat et al., 2012). In our study, Pinus ponderosa had the shortest time to mortality, consistent with this prediction. However, time to mortality among the other four species did not match this expected embolism order, even when we adjusted our time to mortality based on stress metrics. A potentially co-occurring driver of mortality among plants is carbon starvation via stomatal closure (McDowell et al., 2008, 2022; Peltier et al., 2021). In this study, stomatal closure followed water stress points by 2 to 4 weeks across all species. The time from stomatal closure to mortality, on the other hand, varied widely across species. While most species reached mortality 5 to 8 weeks following stomatal closure, Pinus flexilis survived for 13 weeks. These differences in time to mortality could possibly be attributed to higher tolerance to carbon limitation or even greater carbon reserves to withstand longer periods of stomatal closure, although our study was not designed to elucidate this mechanism. The early mortality in Pinus ponderosa is consistent with mechanisms of both embolism and carbon starvation, but leaves open questions about the mechanisms of drought and temperature vulnerability in the other species at the juvenile stage.
Ecohydrological factors–how plant structure interacts with environmental conditions to influence water availability–may help to elucidate the role of evapotranspiration in this study (Rodriguez-Iturbe, 2000; D’Odorico et al., 2010). A field study in the Jemez of planted seedling survival under shrub canopy found that planting efforts were more successful in cooler microclimates where denser shrub canopy provided more shading for seedlings and reduced vapor pressure deficit (Crockett and Hurteau, 2022). These authors found that shading provided by denser shrub canopy compared to more open shrub canopy delayed the time to mortality among Pinus ponderosa, Pinus edulis, and Pseudotsuga menziesii by 2 weeks. Other studies have found that increased tree basal area is correlated with increased mortality, suggesting that thinning forests may reduce resource competition among adult trees (Bradford and Bell, 2017; Bradford et al., 2022; McCauley et al., 2022), although it is unclear how juvenile trees may interact with this competition given differences in plant-water availability at various soil depths (Breshears et al., 2009). More open tree canopy architecture can allow greater evapotranspiration and water loss, while closed canopy structures can shade soil and retain moisture (Royer et al., 2012). This effect may have been operating in our study at the individual tree scale, so that leaf arrangement interacted with evaporative loss in our potted plants. In our study, Pinus flexilis was observed to have more compact needles with branches concentrated near the soil, which may have led to greater water retention. Even after soil moisture became more limiting, lower evaporation rates may have extended the time Pinus flexilis was able to survive.
Current species elevational distribution, represented in this study with corresponding temperature ranges, substantially influenced differing mortality rates among species. We expected a priori that lower-elevation species growing in warmer and drier environments would be more adapted to withstand drought stress. Instead, we found that warm ambient temperatures led to high evapotranspiration rates and water loss, followed by more rapid species death. Cooler ambient temperatures of higher-elevation species, conversely, acted as a buffer against drought conditions presumably by slowing the loss of water through evaporation; longer droughts were necessary before higher-elevation species would reach water stress, perhaps overriding expected differences in drought tolerance due to tree physiology.
All three interacting factors of species physiology, ecohydrological influences, and temperature effects could influence our experimental results. Nonetheless, the outcomes of this study indicate the potential consequences of prolonged drought and heatwave exposure at the juvenile state across species, adding to our overall understanding of these species’ drought tolerances at critical demographic life stages.
Limitations and future work
We acknowledge several limitations in this preliminary study. First, we used growth chambers to implement a controlled heatwave event and normalize drought stress across species to compare stress tolerance, which would be difficult to implement in a replicated field setting. Second, as is typical with growth chamber experiments, individuals within each chamber may be spatially correlated in their responses, and results can be difficult to translate to the landscape scale. Third, conductance measurements are sensitive to fluctuations in humidity and temperature; to maintain measurement accuracy, we calibrated the porometer prior to each measurement period. Fourth, ecohydrological influences with landscape topography, microclimate, and the broader plant community can attenuate climate drivers of heat and drought, influencing water stress among species. Fifth, as is often the case in reforestation efforts, plant material did not originate from the specific study region, and regional species adaptations were therefore not reflected in this study. While there is mixed evidence about the effect of seed sources from different local climates on survival rates of planted seedlings (Pinto et al., 2023), local variation could be important when making predictions for how species might respond across elevational gradients in specific landscapes. Recent studies have suggested that epigenetic responses (heritable traits in juveniles in response to stresses experienced by the parent organism) may help mitigate localized stress to drought heat stress (Carneros et al., 2017; Amaral et al., 2020; Backs and Ashley, 2021). Overall, we employed temperature conditions within the typical elevation range for ecologically co-occurring species the Jemez Mountains, and this study design was appropriate for imposing consistent environmental conditions across species.
Potential limitations in our experimental design suggest promising future lines of research. More mechanistic methods to complement our insights into drought tolerance would require a larger sample size to allow for weekly destructive sampling (e.g., of biomass, plant water potential and non-structural carbohydrates) or rewatering plants at different time intervals to estimate when plants cannot recover (e.g., Hammond et al., 2019). Estimates of plant death and percent brown are consistent with other plant mortality studies as relative measures, yet these are still whole-plant subjective measurements with coarse definitions. We mitigated observer variation by having one technician consistently evaluating tree mortality metrics. Multispectral or hyperspectral image data include a wider and more finely resolved array of wavelengths beyond what would be detected by ocular estimates, such as near infrared wavelengths that are longer than the visible wavelengths. Although results have not been reported in this study, initial progress has been made to develop and test an objective, quantifiable, and repeatable method of image analysis using weekly photographs tracking plant color change.
To uncover the impacts of heatwave at various stress points, future growth chamber studies could adjust heatwave timing, duration (days), and intensity (degrees of increase) after the onset of drought. A differently parameterized heatwave experiment (e.g., Breshears et al., 2021) would help to explore the dimensions of heatwave impacts. Our drought experimental framework could be expanded to include re-watering of trees following varying intervals of drought, to examine the recovery potential of individuals. These results could be complemented with further studies of the effects of warming baseline temperatures on species vulnerability (e.g., Adams et al., 2009, 2017) for a more complete picture of future forest change. Additionally, exposing trees of multiple species to a range of ambient temperatures could help to differentiate proximate responses from the tolerance to environmental conditions. In this experiment, the co-occurrence of species in a single climate represents a narrow view of the area a species may occupy. For instance, the co-occurrence of Pinus ponderosa and Pinus edulis represents the lower elevational boundary of Pinus ponderosa and the upper elevational boundary of Pinus edulis. A more complete factorial experiment in which species are exposed to a gradient of conditions would further elucidate species tolerances across their elevational range.
Ecological and management implications
Drought and temperature regimes, and widespread disturbances, will almost certainly have a prominent role in shaping future species distributions (Thuiller, 2004; Loehman et al., 2018; Parks et al., 2019; Davis et al., 2023). Landscape-scale droughts and periodic heatwaves are likely to become persistent climate factors in the Southwest (Guirguis et al., 2018; Williams et al., 2020). The backdrop of drought, even within the context of species-specific climatic adaptations, will be a determining factor in the survival of tree species to a rapidly changing climate. In this dynamic environment, young trees represent a critical demographic stage forcing species range shifts, especially toward the leading edge of the species distribution (Talluto et al., 2017; Parks et al., 2019; Shriver et al., 2022). Young trees play an outsized role in post-fire restoration, where naturally occurring seed dispersal is either limited or absent (Shive et al., 2018; North et al., 2019; Stevens-Rumann and Morgan, 2019).
This study expands the scope of forest recovery beyond initial seedling establishment following disturbance, to include persistence against subsequent climate pressures during early tree development. Our results uncover substantial species-specific responses to drought and heat at the juvenile growth stage. When normalizing species to different levels of stress, Pinus flexilis survived three times longer than Pinus ponderosa at the juvenile growth stage, while Pinus edulis, Picea engelmannii, and Pseudotsuga menziesii had comparable tolerance with intermediate survival rates. We also saw a pronounced temperature effect, where warmer baseline temperatures allow drought conditions to be realized more quickly and cooler baseline temperatures delayed the onset of drought conditions. Thus, higher-elevation species at cooler temperatures may be able to withstand longer droughts. This could be a mechanism to draw species up in elevation over time in response to climate change (Monleon and Lintz, 2015).Importantly, our results complement observations of recruitment failure in post-fire landscapes that are projected to become more pronounced under future climate (Davis et al., 2023) by experimentally isolating the effects of drought with and without a heatwave.
The relatively high vulnerability of Pinus ponderosa to drought seems surprising given its dominance in dry landscapes across the Southwest (Oliver and Ryker, 1990). Pinus ponderosa is highly adapted to dry, fire-frequent landscapes, with traits such as thick bark and elevated open crowns to avoid fire damage, although these traits are expressed primarily in the adult stage (Pausas et al., 2017; Keeley and Pausas, 2022). As a mature tree, Pinus ponderosa can survive low intensity fire disturbance, but juveniles are considerably less fire resistant (Partelli-Feltrin et al., 2020). Juvenile regeneration in the species is episodic and highly dependent on climate (White, 1985; Mast et al., 1999); several years of favorable climate are needed to support juvenile survival and growth (Kemp et al., 2019). Although mature Pinus ponderosa may be favored at lower elevations due to its fire adaptations, juveniles facing climate extremes may be vulnerable in the warm-dry tails of their climatic range (Kolb et al., 2007; Remke et al., 2020). In contrast, Pinus flexilis has a relatively small abundance in the Jemez Mountains despite its drought tolerance (Muldavin and Tonne, 2003). Pinus flexilis is concentrated on rocky dry sites in cool environments where they hold a competitive advantage over other conifers (Menon et al., 2021; Hankin et al., 2023). In the southern Rocky Mountains, they are concentrated in the mixed conifer and spruce-fir zones, and are successionally replaced by spruce and fir species on most sites (Rebertus et al., 1991). In an experimental study of seedling emergence under low soil moisture conditions, Pinus flexilis had the highest emergence rates of the three high-elevation five-needle pines in the study (Hankin et al., 2023). As this study suggests, Pinus flexilis may gain a competitive advantage in sites where forest species must contend with lengthening droughts.
Natural and managed forest regeneration is dependent on the ability of tree juveniles to survive expected climate anomalies. Many forest and woodland ecosystems in the Southwest are forecast to be highly vulnerable to vegetation conversion, based on species distributions and shifting climate envelope models (Triepke et al., 2019). Strategic tree planting, especially in the context of prolonged drought, is an active management technique that can mitigate regeneration failure (Field et al., 2020; Pinto et al., 2023). Planting in refugia, where climate conditions are most suitable (McDowell et al., 2019; Krawchuk et al., 2020) can facilitate regeneration among disturbed landscapes (Stevens et al., 2021). The juvenile age class, as examined in this study, is especially relevant to managed reforestation strategies (Landis et al., 2010).
Our study employed juvenile trees acquired from nurseries, and our results can thus inform managers concerned with potential success of outplanting under emerging climate conditions. This demographic stage is key to implementing mechanistic models of forest ecological resilience (Falk, 2017; Falk et al., 2022). In turn, ecological resilience is the foundation for emerging management and adaptation paradigms, including the Resist-Resilience-Transition (Peterson St-Laurent et al., 2021) or Resist-Accept-Direct (Thompson et al., 2021) management frameworks. Resisting change through recovery would be focused on planting a species according to its historic distribution, which may require ongoing active management to resist persistent drought conditions. On the other hand, planting species at higher elevations with cooler temperatures, beyond their typical climatic zones, would represent a transition approach, facilitating upward migration of species (Brusca et al., 2013; Parks et al., 2019). Other transition approaches might include planting more drought-tolerant species which are not currently dominant on the landscape but may become more widespread in the future. Adaptive management will be especially important in the face of uncertain climate futures (Jackson, 2021).
In conclusion, our incorporation of drought and temperature tolerance at the juvenile demographic stage fills a key information gap regarding potential species persistence, recovery, and range shifts over time. Future management actions and planting strategies can consider these experimental outcomes to anticipate landscape-level climate impacts on juvenile tree survival as the key to species adaption in a rapidly changing world.
Data availability statement
The datasets presented in this study can be found in online repositories. The names of the repository/repositories and accession number(s) can be found at: https://github.com/alexandralalor/HeatwaveProject.
Author contributions
AL collected and managed data. DL and JF were responsible for experimental setup and assisted in data collection. DB and DF formulated the ideas for this manuscript with input from all authors. AL analyzed data and wrote the manuscript with substantial contributions from DB, DF, RL, and FT providing critical edits. DL, JF, and GB-G contributed to further manuscript edits and provided access to needed equipment. RL and FT provided fundamental insight in framing the manuscript. All authors contributed to the article and approved the submitted version.
Funding
Funding for this research was provided by the USGS SW Climate Adaptation Science Center (G18AC00320), with Regents Professor Funds used to acquire plants.
Acknowledgments
We are grateful for data access and professional insight on the topics of this study by Craig Allen, Kay Beeley, and Bill Hammond. Many faculty members at the University of Arizona provided technical assistance with this project. Devin Bayly assisted in developing code related to photo analysis and color change of seedlings over time. Ellen Bledsoe provided foundational training in R programing to organize data and graphically represent results. Robert Steidl provided crucial guidance on statistical analysis methods considering our experimental setup. And to the 250 trees which gave their lives to science, teaching us that drought has the potential to change species composition across landscapes.
Conflict of interest
The authors declare that the research was conducted in the absence of any commercial or financial relationships that could be construed as a potential conflict of interest.
Publisher’s note
All claims expressed in this article are solely those of the authors and do not necessarily represent those of their affiliated organizations, or those of the publisher, the editors and the reviewers. Any product that may be evaluated in this article, or claim that may be made by its manufacturer, is not guaranteed or endorsed by the publisher.
Supplementary material
The Supplementary material for this article can be found online at: https://www.frontiersin.org/articles/10.3389/ffgc.2023.1198156/full#supplementary-material
Footnotes
1. ^Flora of North America Editorial Committee, eds. 1993+. Flora of North America North of Mexico. 22+ vols. New York and Oxford. Available at: http://beta.floranorthamerica.org Accessed November 17 2022.
References
Abatzoglou, J. T., and Williams, A. P. (2016). Impact of anthropogenic climate change on wildfire across western US forests. Proc. Natl. Acad. Sci. 113, 11770–11775. doi: 10.1073/pnas.1607171113
Adams, H. D., Barron-Gafford, G. A., Minor, R. L., Gardea, A. A., Bentley, L. P., Law, D. J., et al. (2017). Temperature response surfaces for mortality risk of tree species with future drought. Environ. Res. Lett. 12:115014. doi: 10.1088/1748-9326/aa93be
Adams, H. D., Guardiola-Claramonte, M., Barron-Gafford, G. A., Villegas, J. C., Breshears, D. D., Zou, C. B., et al. (2009). Temperature sensitivity of drought-induced tree mortality portends increased regional die-off under global-change-type drought. Proc. Natl. Acad. Sci. 106, 7063–7066. doi: 10.1073/pnas.0901438106
Ahrens, C. W., Challis, A., Byrne, M., Leigh, A., Nicotra, A. B., Tissue, D., et al. (2021). Repeated extreme heatwaves result in higher leaf thermal tolerances and greater safety margins. New Phytol. 232, 1212–1225. doi: 10.1111/nph.17640
Allen, C. D. (1989). Changes in the landscape of the Jemez Mountains, New Mexico (dissertation). Berkeley, CA: University of California.
Allen, C. D. (2007). Interactions across spatial scales among Forest dieback, fire, and Erosion in northern New Mexico landscapes. Ecosystems 10, 797–808. doi: 10.1007/s10021-007-9057-4
Allen, C. D. (2014). “Forest ecosystem re-organization underway in the southwestern United States: a preview of widespread forest changes in the Anthropocene” in RMRS-P-71. Presented at the Forest conservation and Management in the Anthropocene: Conference proceedings. eds. V. A. Sample, R. P. Bixler, and C. Miller (Fort Collins, CO: USDA Forest Service Rocky Mountain Research Station), 103–123.
Allen, C. D., and Breshears, D. D. (1998). Drought-induced shift of a forest-woodland ecotone: rapid landscape response to climate variation. Proc. Natl. Acad. Sci. 95, 14839–14842. doi: 10.1073/pnas.95.25.14839
Allen, C. D., Breshears, D. D., and McDowell, N. G. (2015). On underestimation of global vulnerability to tree mortality and forest die-off from hotter drought in the Anthropocene. Ecosphere 6:art129. doi: 10.1890/ES15-00203.1
Amaral, J., Ribeyre, Z., Vigneaud, J., Sow, M. D., Fichot, R., Messier, C., et al. (2020). Advances and promises of epigenetics for Forest trees. Forests 11:976. doi: 10.3390/f11090976
Andivia, E., Villar-Salvador, P., Oliet, J. A., Puértolas, J., Dumroese, R. K., Ivetić, V., et al. (2021). Climate and species stress resistance modulate the higher survival of large seedlings in forest restorations worldwide. Ecol. Appl. 31:e02394. doi: 10.1002/eap.2394
Backs, J. R., and Ashley, M. V. (2021). Quercus conservation genetics and genomics: past, present, and future. Forests 12:882. doi: 10.3390/f12070882
Bailey, S. N., Elliott, G. P., and Schliep, E. M. (2021). Seasonal temperature–moisture interactions limit seedling establishment at upper treeline in the southern Rockies. Ecosphere 12:3568. doi: 10.1002/ecs2.3568
Batllori, E., Lloret, F., Aakala, T., Anderegg, W. R. L., Aynekulu, E., Bendixsen, D. P., et al. (2020). Forest and woodland replacement patterns following drought-related mortality. Proc. Natl. Acad. Sci. 117, 29720–29729. doi: 10.1073/pnas.2002314117
Blackman, C. J., Creek, D., Maier, C., Aspinwall, M. J., Drake, J. E., Pfautsch, S., et al. (2019). Drought response strategies and hydraulic traits contribute to mechanistic understanding of plant dry-down to hydraulic failure. Tree Physiol. 39, 910–924. doi: 10.1093/treephys/tpz016
Bradford, J. B., and Bell, D. M. (2017). A window of opportunity for climate-change adaptation: easing tree mortality by reducing forest basal area. Front. Ecol. Environ. 15, 11–17. doi: 10.1002/fee.1445
Bradford, J. B., Shriver, R. K., Robles, M. D., McCauley, L. A., Woolley, T. J., Andrews, C. A., et al. (2022). Tree mortality response to drought-density interactions suggests opportunities to enhance drought resistance. J. Appl. Ecol. 59, 549–559. doi: 10.1111/1365-2664.14073
Breshears, D. D., Cobb, N. S., Rich, P. M., Price, K. P., Allen, C. D., Balice, R. G., et al. (2005). Regional vegetation die-off in response to global-change-type drought. Proc. Natl. Acad. Sci. 102, 15144–15148. doi: 10.1073/pnas.0505734102
Breshears, D. D., Fontaine, J. B., Ruthrof, K. X., Field, J. P., Feng, X., Burger, J. R., et al. (2021). Underappreciated plant vulnerabilities to heat waves. New Phytol. 231, 32–39. doi: 10.1111/nph.17348
Breshears, D. D., Myers, O. B., and Barnes, F. J. (2009). Horizontal heterogeneity in the frequency of plant-available water with woodland intercanopy–canopy vegetation patch type rivals that occuring vertically by soil depth. Ecohydrology 2, 503–519. doi: 10.1002/eco.75
Brusca, R. C., Wiens, J. F., Meyer, W. M., Eble, J., Franklin, K., Overpeck, J. T., et al. (2013). Dramatic response to climate change in the southwest: Robert Whittaker’s 1963 Arizona Mountain plant transect revisited. Ecol. Evol. 3, 3307–3319. doi: 10.1002/ece3.720
Burrill, E. A.. (2021). The Forest inventory and analysis database: Database description and user guide for phase 2 (version 9.0.1) 1026.
Carneros, E., Yakovlev, I., Viejo, M., Olsen, J. E., and Fossdal, C. G. (2017). The epigenetic memory of temperature during embryogenesis modifies the expression of bud burst-related genes in Norway spruce epitypes. Planta 246, 553–566. doi: 10.1007/s00425-017-2713-9
Chambers, M. E., Fornwalt, P. J., Malone, S. L., and Battaglia, M. A. (2016). Patterns of conifer regeneration following high severity wildfire in ponderosa pine – dominated forests of the Colorado front range. For. Ecol. Manag. 378, 57–67. doi: 10.1016/j.foreco.2016.07.001
Choat, B., Brodribb, T. J., Brodersen, C. R., Duursma, R. A., López, R., and Medlyn, B. E. (2018). Triggers of tree mortality under drought. Nature 558, 531–539. doi: 10.1038/s41586-018-0240-x
Choat, B., Jansen, S., Brodribb, T. J., Cochard, H., Delzon, S., Bhaskar, R., et al. (2012). Global convergence in the vulnerability of forests to drought. Nature 491, 752–755. doi: 10.1038/nature11688
Ciais, P., Reichstein, M., Viovy, N., Granier, A., Ogée, J., Allard, V., et al. (2005). Europe-wide reduction in primary productivity caused by the heat and drought in 2003. Nature 437, 529–533. doi: 10.1038/nature03972
Clifford, M. J., Cobb, N. S., and Buenemann, M. (2011). Long-term tree cover dynamics in a pinyon-Juniper woodland: climate-change-type drought resets successional clock. Ecosystems 14, 949–962. doi: 10.1007/s10021-011-9458-2
Cook, B. I., Cook, E. R., Smerdon, J. E., Seager, R., Williams, A. P., Coats, S., et al. (2016). North American megadroughts in the common era: reconstructions and simulations. WIREs Clim. Change 7, 411–432. doi: 10.1002/wcc.394
Coop, J. D. (2023). Postfire futures in southwestern forests: climate and landscape influences on trajectories of recovery and conversion. Ecol. Appl. 33:e2725. doi: 10.1002/eap.2725
Coop, J. D., Parks, S. A., McClernan, S. R., and Holsinger, L. M. (2016). Influences of prior wildfires on vegetation response to subsequent fire in a reburned southwestern landscape. Ecol. Appl. 26, 346–354. doi: 10.1890/15-0775
Coop, J. D., Parks, S. A., Stevens-Rumann, C. S., Crausbay, S. D., Higuera, P. E., Hurteau, M. D., et al. (2020). Wildfire-driven Forest conversion in Western North American landscapes. Bioscience 70, 659–673. doi: 10.1093/biosci/biaa061
Crockett, J. L., and Hurteau, M. D. (2022). Post-fire early successional vegetation buffers surface microclimate and increases survival of planted conifer seedlings in the southwestern United States. Can. J. For. Res. 52, 416–425. doi: 10.1139/cjfr-2021-0221
D’Odorico, P., Laio, F., Porporato, A., Ridolfi, L., Rinaldo, A., and Rodriguez-Iturbe, I. (2010). Ecohydrology of terrestrial ecosystems. Bioscience 60, 898–907. doi: 10.1525/bio.2010.60.11.6
Davis, K. T., Dobrowski, S. Z., Higuera, P. E., Holden, Z. A., Veblen, T. T., Rother, M. T., et al. (2019). Wildfires and climate change push low-elevation forests across a critical climate threshold for tree regeneration. Proc. Natl. Acad. Sci. 116, 6193–6198. doi: 10.1073/pnas.1815107116
Davis, K. T., Robles, M. D., Kemp, K. B., Higuera, P. E., Chapman, T., Metlen, K. L., et al. (2023). Reduced fire severity offers near-term buffer to climate-driven declines in conifer resilience across the western United States. Proc. Natl. Acad. Sci. 120:e2208120120. doi: 10.1073/pnas.2208120120
De Boeck, H. J., Dreesen, F. E., Janssens, I. A., and Nijs, I. (2010). Whole-system responses of experimental plant communities to climate extremes imposed in different seasons. New Phytol. 189, 806–817. doi: 10.1111/j.1469-8137.2010.03515.x
Dewar, J. J., Falk, D. A., Swetnam, T. W., Baisan, C. H., Allen, C. D., Parmenter, R. R., et al. (2021). Valleys of fire: historical fire regimes of forest-grassland ecotones across the montane landscape of the Valles caldera National Preserve, New Mexico, USA. Landsc. Ecol. 36, 331–352. doi: 10.1007/s10980-020-01101-w
Domec, J.-C., and Gartner, B. L. (2001). Cavitation and water storage capacity in bole xylem segments of mature and young Douglas-fir trees. Trees 15, 204–214. doi: 10.1007/s004680100095
Eamus, D. (2006). Ecohydrology: Vegetation function, water and resource management. Clayton, Australia: CSIRO Publishing.
Falk, D. A.. (2013). Are Madrean ecosystems approaching tipping points? Anticipating interactions of landscape disturbance and climate change, in: RMRS-P-67. Presented at the merging science and management in a rapidly changing world: Biodiversity and management of the Madrean archipelago III. Tucson, AZ: Rocky Mountain Research Station, p. 8.
Falk, D. A. (2017). Restoration ecology, resilience, and the axes of change. Ann. Mo. Bot. Gard. 102, 201–216. doi: 10.3417/2017006
Falk, D. A., Van Mantgem, P. J., Keeley, J. E., Gregg, R. M., Guiterman, C. H., Tepley, A. J., et al. (2022). Mechanisms of forest resilience. For. Ecol. Manag. 512:120129. doi: 10.1016/j.foreco.2022.120129
Fenner, M. (2000). Seeds: The ecology of regeneration in plant communities, 2nd Edn.. New York: CABI Pub, Oxon.
Field, J. P., Breshears, D. D., Bradford, J. B., Law, D. J., Feng, X., and Allen, C. D. (2020). Forest management under Megadrought: urgent needs at finer scale and higher intensity. Front. For. Glob. Change 3:502669. doi: 10.3389/ffgc.2020.502669
Gitlin, A. R., Sthultz, C. M., Bowker, M. A., Stumpf, S., Paxton, K. L., Kennedy, K., et al. (2006). Mortality gradients within and among dominant plant populations as barometers of ecosystem change during extreme drought. Conserv. Biol. 20, 1477–1486. doi: 10.1111/j.1523-1739.2006.00424.x
Guirguis, K., Gershunov, A., Cayan, D. R., and Pierce, D. W. (2018). Heat wave probability in the changing climate of the southwest US. Clim. Dyn. 50, 3853–3864. doi: 10.1007/s00382-017-3850-3
Guiterman, C. H., Gregg, R. M., Marshall, L. A. E., Beckmann, J. J., Van Mantgem, P. J., Falk, D. A., et al. (2022). Vegetation type conversion in the US southwest: frontline observations and management responses. Fire Ecol 18, 1–16. doi: 10.1186/s42408-022-00131-w
Guiterman, C. H., Margolis, E. Q., Allen, C. D., Falk, D. A., and Swetnam, T. W. (2018). Long-term persistence and fire resilience of oak Shrubfields in dry conifer forests of northern New Mexico. Ecosystems 21, 943–959. doi: 10.1007/s10021-017-0192-2
Haire, S., Coop, J., and Miller, C. (2017). Characterizing spatial neighborhoods of Refugia following large fires in northern New Mexico USA. Land 6:19. doi: 10.3390/land6010019
Haire, S. L., and McGarigal, K. (2010). Effects of landscape patterns of fire severity on regenerating ponderosa pine forests (Pinus ponderosa) in New Mexico and Arizona, USA. Landsc. Ecol. 25, 1055–1069. doi: 10.1007/s10980-010-9480-3
Haire, S. L., Villarreal, M. L., Cortés-Montaño, C., Flesch, A. D., Iniguez, J. M., Romo-Leon, J. R., et al. (2022). Climate refugia for Pinus spp. in topographic and bioclimatic environments of the Madrean sky islands of México and the United States. Plant Ecol. 223, 577–598. doi: 10.1007/s11258-022-01233-w
Hammond, W. M., Williams, A. P., Abatzoglou, J. T., Adams, H. D., Klein, T., López, R., et al. (2022). Global field observations of tree die-off reveal hotter-drought fingerprint for Earth’s forests. Nat. Commun. 13:1761. doi: 10.1038/s41467-022-29289-2
Hammond, W. M., Yu, K., Wilson, L. A., Will, R. E., Anderegg, W. R. L., and Adams, H. D. (2019). Dead or dying? Quantifying the point of no return from hydraulic failure in drought-induced tree mortality. New Phytol. 223, 1834–1843. doi: 10.1111/nph.15922
Hankin, L. E., Leger, E. A., and Bisbing, S. M. (2023). Reforestation of high elevation pines: direct seeding success depends on seed source and sowing environment. Ecol. Appl. 33:e2897. doi: 10.1002/eap.2897
Hegewisch, K. C., and Abatzoglou, J. T. (2022). “Historical Climograph” web tool. Climate Toolbox. Available at: https://climatetoolbox.org/.
Husch, B., Beers, T., and Kershaw, J. J.. (2003). Forest Mensuration. Hoboken, NJ: John willey and Sons, Inc.
Ibáñez, T. S., Wardle, D. A., Gundale, M. J., and Nilsson, M.-C. (2021). Effects of soil abiotic and biotic factors on tree seedling regeneration following a boreal Forest wildfire. Ecosystems 25, 471–487. doi: 10.1007/s10021-021-00666-0
IPCC. (2021). Summary for policymakers. Climate change 2021: the physical science basis (working group I), Sixth assessment report of the intergovernmental panel on climate change. Cambridge: Cambridge University Press.
Jackson, S. T. (2021). Transformational ecology and climate change. Science 373, 1085–1086. doi: 10.1126/science.abj6777
Jong, R., Verbesselt, J., Schaepman, M. E., and Bruin, S. (2012). Trend changes in global greening and browning: contribution of short-term trends to longer-term change. Glob. Change Biol. 18, 642–655. doi: 10.1111/j.1365-2486.2011.02578.x
Jung, C. G., Keyser, A. R., Remy, C. C., Krofcheck, D., Allen, C. D., and Hurteau, M. D. (2023). Topographic information improves simulated patterns of post-fire conifer regeneration in the Southwest United States. Glob. Change Biol 29, 4342–4353. doi: 10.1111/gcb.16764
Kaplan, E. L., and Meier, P. (1958). Nonparametric estimation from incomplete observations. J. Am. Stat. Assoc. 53, 457–481. doi: 10.1080/01621459.1958.10501452
Keeley, J. E., and Pausas, J. G. (2022). Evolutionary ecology of fire. Annu. Rev. Ecol. Evol. Syst. 53, 203–225. doi: 10.1146/annurev-ecolsys-102320-095612
Kemp, K. B., Higuera, P. E., Morgan, P., and Abatzoglou, J. T. (2019). Climate will increasingly determine post-fire tree regeneration success in low-elevation forests, northern Rockies, USA. Ecosphere 10:e02568. doi: 10.1002/ecs2.2568
Klein, T. (2014). The variability of stomatal sensitivity to leaf water potential across tree species indicates a continuum between isohydric and anisohydric behaviours. Funct. Ecol. 28, 1313–1320. doi: 10.1111/1365-2435.12289
Koepke, D. F., Kolb, T. E., and Adams, H. D. (2010). Variation in woody plant mortality and dieback from severe drought among soils, plant groups, and species within a northern Arizona ecotone. Oecologia 163, 1079–1090. doi: 10.1007/s00442-010-1671-8
Kolb, T. E., Agee, J. K., Fulé, P. Z., McDowell, N. G., Pearson, K., Sala, A., et al. (2007). Perpetuating old ponderosa pine. For. Ecol. Manag. 249, 141–157. doi: 10.1016/j.foreco.2007.06.002
Krawchuk, M. A., Meigs, G. W., Cartwright, J. M., Coop, J. D., Davis, R., Holz, A., et al. (2020). Disturbance refugia within mosaics of forest fire, drought, and insect outbreaks. Front. Ecol. Environ. 18, 235–244. doi: 10.1002/fee.2190
Landis, T. D., Dumroese, R. K., and Haase, D. L. (2010). The container tree nursery manual: Volume 7, Seedling processing, storage, and outplanting 208.
Lauenroth, W. K., and Bradford, J. B. (2009). Ecohydrology of dry regions of the United States: precipitation pulses and intraseasonal drought. Ecohydrology 2, 173–181. doi: 10.1002/eco.53
Loehman, R., Flatley, W., Holsinger, L., and Thode, A. (2018). Can land management buffer impacts of climate changes and altered fire regimes on ecosystems of the southwestern United States? Forests 9:192. doi: 10.3390/f9040192
Loehman, R. A., Keane, R. E., and Holsinger, L. M. (2020). Simulation modeling of complex climate, wildfire, and vegetation dynamics to address wicked problems in land management. Front. For. Glob. Change 3:3. doi: 10.3389/ffgc.2020.00003
López, R., Ramírez-Valiente, J. A., and Pita, P. (2022). How plants cope with heatwaves in a drier environment. Flora 295:152148. doi: 10.1016/j.flora.2022.152148
Marchin, R. M., Backes, D., Ossola, A., Leishman, M. R., Tjoelker, M. G., and Ellsworth, D. S. (2022). Extreme heat increases stomatal conductance and drought-induced mortality risk in vulnerable plant species. Glob. Change Biol. 28, 1133–1146. doi: 10.1111/gcb.15976
Margolis, E. Q., and Malevich, S. B. (2016). Historical dominance of low-severity fire in dry and wet mixed-conifer forest habitats of the endangered terrestrial Jemez Mountains salamander (Plethodon neomexicanus). For. Ecol. Manag. 375, 12–26. doi: 10.1016/j.foreco.2016.05.011
Marsh, C., Crockett, J. L., Krofcheck, D., Keyser, A., Allen, C. D., Litvak, M., et al. (2022). Planted seedling survival in a post-wildfire landscape: from experimental planting to predictive probabilistic surfaces. For. Ecol. Manag. 525:120524. doi: 10.1016/j.foreco.2022.120524
Mast, J. N., Fulé, P. Z., Moore, M. M., Covington, W. W., and Waltz, A. E. M. (1999). Restoration of Presettlement age structure of an Arizona Ponderosa pine Forest. Ecol. Appl. 9, 228–239. doi: 10.1890/1051-0761(1999)009[0228:ROPASO]2.0.CO;2
Matusick, G., Ruthrof, K. X., Kala, J., Brouwers, N. C., Breshears, D. D., and Hardy, G. E. S. (2018). Chronic historical drought legacy exacerbates tree mortality and crown dieback during acute heatwave-compounded drought. Environ. Res. Lett. 13:095002. doi: 10.1088/1748-9326/aad8cb
McCauley, L. A., Bradford John, B., Robles, M. D., Shriver, R. K., Woolley, T. J., and Andrews, C. A. (2022). Landscape-scale forest restoration decreases vulnerability to drought mortality under climate change in Southwest USA ponderosa forest. For. Ecol. Manag. 509:120088. doi: 10.1016/j.foreco.2022.120088
McDowell, N. G., Grossiord, C., Adams, H. D., Pinzón-Navarro, S., Mackay, D. S., Breshears, D. D., et al. (2019). Mechanisms of a coniferous woodland persistence under drought and heat. Environ. Res. Lett. 14:045014. doi: 10.1088/1748-9326/ab0921
McDowell, N., Pockman, W. T., Allen, C. D., Breshears, D. D., Cobb, N., Kolb, T., et al. (2008). Mechanisms of plant survival and mortality during drought: why do some plants survive while others succumb to drought? New Phytol. 178, 719–739. doi: 10.1111/j.1469-8137.2008.02436.x
McDowell, N. G., Sapes, G., Pivovaroff, A., Adams, H. D., Allen, C. D., Anderegg, W. R. L., et al. (2022). Mechanisms of woody-plant mortality under rising drought, CO2 and vapour pressure deficit. Nat. Rev. Earth Environ. 3, 294–308. doi: 10.1038/s43017-022-00272-1
McDowell, N. G., Williams, A. P., Xu, C., Pockman, W. T., Dickman, L. T., Sevanto, S., et al. (2016). Multi-scale predictions of massive conifer mortality due to chronic temperature rise. Nat. Clim. Chang. 6, 295–300. doi: 10.1038/nclimate2873
Menon, M., Bagley, J. C., Page, G. F. M., Whipple, A. V., Schoettle, A. W., Still, C. J., et al. (2021). Adaptive evolution in a conifer hybrid zone is driven by a mosaic of recently introgressed and background genetic variants. Commun. Biol. 4:160. doi: 10.1038/s42003-020-01632-7
Monleon, V. J., and Lintz, H. E. (2015). Evidence of tree species’ range shifts in a complex landscape. PLoS One 10:e0118069. doi: 10.1371/journal.pone.0118069
Muldavin, E., and Tonne, P. (2003). A vegetation survey and preliminary ecological assessment of Valles caldera national preserve, New Mexico (Final Report No. Cooperative Agreement No. 01CRAG0014). University of New Mexico.
Myneni, R. B., Hall, F. G., Sellers, P. J., and Marshak, A. L. (1995). The interpretation of spectral vegetation indexes. IEEE Trans. Geosci. Remote Sens. 33, 481–486. doi: 10.1109/TGRS.1995.8746029
National Park Service. (2003). Fire monitoring handbook. National Interagency Fire Center, Fire Management Program Center No. 274.
Nelson, K. N., O’Dean, E., Knapp, E. E., Parker, A. J., and Bisbing, S. M. (2021). Persistent yet vulnerable: resurvey of an Abies ecotone reveals few differences but vulnerability to climate change. Ecology 102:3525. doi: 10.1002/ecy.3525
Niinemets, Ü. (2010). Responses of forest trees to single and multiple environmental stresses from seedlings to mature plants: past stress history, stress interactions, tolerance and acclimation. For. Ecol. Manag. 260, 1623–1639. doi: 10.1016/j.foreco.2010.07.054
Nolan, R. H., Collins, L., Leigh, A., Ooi, M. K. J., Curran, T. J., Fairman, T. A., et al. (2021). Limits to post-fire vegetation recovery under climate change. Plant Cell Environ. 44, 3471–3489. doi: 10.1111/pce.14176
North, M. P., Stevens, J. T., Greene, D. F., Coppoletta, M., Knapp, E. E., Latimer, A. M., et al. (2019). Tamm review: reforestation for resilience in dry western U.S. forests. For. Ecol. Manag. 432, 209–224. doi: 10.1016/j.foreco.2018.09.007
Notarnicola, R. F., Nicotra, A. B., Kruuk, L. E. B., and Arnold, P. A. (2021). Tolerance of warmer temperatures does not confer resilience to heatwaves in an alpine herb. Front. Ecol. Evol. 9:615119. doi: 10.3389/fevo.2021.615119
O’Connor, C. D., Falk, D. A., and Garfin, G. M. (2020). Projected climate-fire interactions drive Forest to Shrubland transition on an Arizona Sky Island. Front. Environ. Sci. 8:137. doi: 10.3389/fenvs.2020.00137
Oliver, W. W., and Ryker, R. A. (1990). Pinus ponderosa Dougl. Ex Laws. Ponderosa pine, silvics of North America. United States Department of Agriculture, Forest Service.
Owen, S. M., Sieg, C. H., Sánchez Meador, A. J., Fulé, P. Z., Iniguez, J. M., Baggett, L. S., et al. (2017). Spatial patterns of ponderosa pine regeneration in high-severity burn patches. For. Ecol. Manag. 405, 134–149. doi: 10.1016/j.foreco.2017.09.005
Parks, S. A., and Abatzoglou, J. T. (2020). Warmer and drier fire seasons contribute to increases in area burned at high severity in Western US forests from 1985 to 2017. Geophys. Res. Lett. 47:9858. doi: 10.1029/2020GL089858
Parks, S. A., Dobrowski, S. Z., Shaw, J. D., and Miller, C. (2019). Living on the edge: trailing edge forests at risk of fire-facilitated conversion to non-Forest. Ecosphere 10:e02651. doi: 10.1002/ecs2.2651
Parks, S. A., Miller, C., Parisien, M.-A., Holsinger, L. M., Dobrowski, S. Z., and Abatzoglou, J. (2015). Wildland fire deficit and surplus in the western United States, 1984–2012. Ecosphere 6:art275. doi: 10.1890/ES15-00294.1
Partelli-Feltrin, R., Johnson, D. M., Sparks, A. M., Adams, H. D., Kolden, C. A., Nelson, A. S., et al. (2020). Drought increases vulnerability of Pinus ponderosa saplings to fire-induced mortality. Fire 3:56. doi: 10.3390/fire3040056
Pausas, J. G., Keeley, J. E., and Schwilk, D. W. (2017). Flammability as an ecological and evolutionary driver. J. Ecol. 105, 289–297. doi: 10.1111/1365-2745.12691
Peltier, D. M. P., Guo, J., Nguyen, P., Bangs, M., Gear, L., Wilson, M., et al. (2021). Temporal controls on crown nonstructural carbohydrates in southwestern US tree species. Tree Physiol. 41, 388–402. doi: 10.1093/treephys/tpaa149
Perkins-Kirkpatrick, S. E., and Lewis, S. C. (2020). Increasing trends in regional heatwaves. Nat. Commun. 11:3357. doi: 10.1038/s41467-020-16970-7
Peterson St-Laurent, G., Oakes, L. E., Cross, M., and Hagerman, S. (2021). R-R-T (resistance-resilience-transformation) typology reveals differential conservation approaches across ecosystems and time. Commun. Biol. 4:39. doi: 10.1038/s42003-020-01556-2
Pinto, J. R., Sloan, J. L., Ervan, G., and Burney, O. T. (2023). Physiological and morphological responses of Pinus ponderosa seedlings to moisture limitations in the nursery and their implications for restoration. Front. Plant Sci. 14:1127656. doi: 10.3389/fpls.2023.1127656
R Core Team. (2022). R: A language and environment for statistical computing. Available at: https://www.R-project.org/
Rebertus, A. J., Burns, B. R., and Veblen, T. T. (1991). Stand dynamics of Pinus flexilis-dominated subalpine forests in the Colorado front range. J. Veg. Sci. 2, 445–458. doi: 10.2307/3236026
Redmond, M. D., Weisberg, P. J., Cobb, N. S., and Clifford, M. J. (2018). Woodland resilience to regional drought: dominant controls on tree regeneration following overstorey mortality. J. Ecol. 106, 625–639. doi: 10.1111/1365-2745.12880
Remke, M. J., Hoang, T., Kolb, T., Gehring, C., Johnson, N. C., and Bowker, M. A. (2020). Familiar soil conditions help Pinus ponderosa seedlings cope with warming and drying climate. Restor. Ecol. 28:13144. doi: 10.1111/rec.13144
Rich, P. M., Breshears, D. B., and White, A. B. (2008). Phenology of mixed woody-herbaceous ecosystems following extreme events: net and differential responses. Ecology 89, 342–352. doi: 10.1890/06-2137.1
Roccaforte, J. P., Fulé, P. Z., Chancellor, W. W., and Laughlin, D. C. (2012). Woody debris and tree regeneration dynamics following severe wildfires in Arizona ponderosa pine forests. Can. J. For. Res. 42, 593–604. doi: 10.1139/x2012-010
Rodriguez-Iturbe, I. (2000). Ecohydrology: a hydrologic perspective of climate-soil-vegetation dynamics. Water Resour. Res. 36, 3–9. doi: 10.1029/1999WR900210
Rother, M. T., and Veblen, T. T. (2016). Limited conifer regeneration following wildfires in dry ponderosa pine forests of the Colorado front range. Ecosphere 7:1594. doi: 10.1002/ecs2.1594
Royer, P. D., Breshears, D. D., Zou, C. B., Villegas, J. C., Cobb, N. S., and Kurc, S. A. (2012). Density-dependent Ecohydrological effects of Piñon–Juniper Woody canopy cover on soil microclimate and potential soil evaporation. Rangel. Ecol. Manag. 65, 11–20. doi: 10.2111/REM-D-11-00007.1
Ruthrof, K. X., Breshears, D. D., Fontaine, J. B., Froend, R. H., Matusick, G., Kala, J., et al. (2018). Subcontinental heat wave triggers terrestrial and marine, multi-taxa responses. Sci. Rep. 8:13094. doi: 10.1038/s41598-018-31236-5
Salomón, R. L., Peters, R. L., Zweifel, R., Sass-Klaassen, U. G. W., Stegehuis, A. I., Smiljanic, M., et al. (2022). The 2018 European heatwave led to stem dehydration but not to consistent growth reductions in forests. Nat. Commun. 13:28. doi: 10.1038/s41467-021-27579-9
Savage, M., Mast, J. N., and Feddema, J. J. (2013). Double whammy: high-severity fire and drought in ponderosa pine forests of the southwest. Can. J. For. Res. 43, 570–583. doi: 10.1139/cjfr-2012-0404
Shapiro, S. S., and Wilk, M. B. (1965). An analysis of variance test for normality (complete samples). Biometrika 52:591. doi: 10.2307/2333709
Shive, K. L., Preisler, H. K., Welch, K. R., Safford, H. D., Butz, R. J., O’Hara, K. L., et al. (2018). From the stand scale to the landscape scale: predicting the spatial patterns of forest regeneration after disturbance. Ecol. Appl. 28, 1626–1639. doi: 10.1002/eap.1756
Shriver, R. K., Yackulic, C. B., Bell, D. M., and Bradford, J. B. (2022). Dry forest decline is driven by both declining recruitment and increasing mortality in response to warm, dry conditions. Glob. Ecol. Biogeogr. 31, 2259–2269. doi: 10.1111/geb.13582
Simler-Williamson, A. B., Rizzo, D. M., and Cobb, R. C. (2019). Interacting effects of global change on Forest Pest and pathogen dynamics. Annu. Rev. Ecol. Evol. Syst. 50, 381–403. doi: 10.1146/annurev-ecolsys-110218-024934
Singleton, M. P., Thode, A. E., Sánchez Meador, A. J., and Iniguez, J. M. (2019). Increasing trends in high-severity fire in the southwestern USA from 1984 to 2015. For. Ecol. Manag. 433, 709–719. doi: 10.1016/j.foreco.2018.11.039
Soil Survey Staff. (2002). Official soil series descriptions - Jemez series. Natural Resources Conservation Service, United States Department of Agriculture. Available at: https://soilseries.sc.egov.usda.gov/OSD_Docs/J/JEMEZ.html.
Steffen, W. L., Hughes, L., and Perkins, S. (2014). Heatwaves: Hotter, longer, more often. Sydney: Climate Council of Australia Limited.
Stevens, J. T., Haffey, C. M., Coop, J. D., Fornwalt, P. J., Yocom, L., Allen, C. D., et al. (2021). Tamm review: Postfire landscape management in frequent-fire conifer forests of the southwestern United States. For. Ecol. Manag. 502:119678. doi: 10.1016/j.foreco.2021.119678
Stevens-Rumann, C. S., and Morgan, P. (2019). Tree regeneration following wildfires in the western US: a review. Fire Ecol. 15:15. doi: 10.1186/s42408-019-0032-1
Stevens-Rumann, C. S., Prichard, S. J., Whitman, E., Parisien, M.-A., and Meddens, A. J. H. (2022). Considering regeneration failure in the context of changing climate and disturbance regimes in western North America. Can. J. For. Res. 52, 1281–1302. doi: 10.1139/cjfr-2022-0054
Stewart, J. A. E., Young, D. J. N., Shive, K. L., Preisler, H. K., Das, A. J., Stephenson, N. L., et al. (2021). Effects of postfire climate and seed availability on postfire conifer regeneration. Ecol. Appl. 31:2280. doi: 10.1002/eap.2280
Talluto, M. V., Boulangeat, I., Vissault, S., Thuiller, W., and Gravel, D. (2017). Extinction debt and colonization credit delay range shifts of eastern North American trees. Nat. Ecol. Evol. 1:0182. doi: 10.1038/s41559-017-0182
Tepley, A. J., Thompson, J. R., Epstein, H. E., and Anderson-Teixeira, K. J. (2017). Vulnerability to forest loss through altered postfire recovery dynamics in a warming climate in the Klamath Mountains. Glob. Change Biol. 23, 4117–4132. doi: 10.1111/gcb.13704
Therneau, T. (2022). A package for survival analysis in R. Available at: https://CRAN.R-project.org/package=survival.
Thompson, L. M., Lynch, A. J., Beever, E. A., Engman, A. C., Falke, J. A., Jackson, S. T., et al. (2021). Responding to ecosystem transformation: resist, accept, or direct? Fisheries 46, 8–21. doi: 10.1002/fsh.10506
Thuiller, W. (2004). Patterns and uncertainties of species’ range shifts under climate change: SPECIES’ RANGE SHIFTS. Glob. Change Biol. 10, 2020–2027. doi: 10.1111/j.1365-2486.2004.00859.x
Triepke, F. J., Muldavin, E. H., and Wahlberg, M. M. (2019). Using climate projections to assess ecosystem vulnerability at scales relevant to managers. Ecosphere 10:2854. doi: 10.1002/ecs2.2854
Urli, M., Périé, C., Thiffault, N., Coyea, M. R., Pepin, S., Logan, T., et al. (2023). Experimental drier climates affect hydraulics and induce high mortality of seedlings of three northern conifer species. For. Ecol. Manag. 544:121127. doi: 10.1016/j.foreco.2023.121127
Van Genuchten, M. T. (1980). A closed-form equation for predicting the hydraulic conductivity of unsaturated soils. Soil Sci. Soc. Am. J. 44, 892–898. doi: 10.2136/sssaj1980.03615995004400050002x
Van Mantgem, P. J., Nesmith, J. C. B., Keifer, M., Knapp, E. E., Flint, A., and Flint, L. (2013). Climatic stress increases forest fire severity across the western United States. Ecol. Lett. 16, 1151–1156. doi: 10.1111/ele.12151
Werner, C. (2022). Extreme droughts and heatwaves endanger temperate forests. Plant Biol. 24, 1091–1092. doi: 10.1111/plb.13488
White, A. S. (1985). Presettlement regeneration patterns in a southwestern ponderosa pine stand. Ecology 66, 589–594. doi: 10.2307/1940407
Williams, A. P., Cook, B. I., and Smerdon, J. E. (2022). Rapid intensification of the emerging southwestern North American megadrought in 2020–2021. Nat. Clim. Chang. 12, 232–234. doi: 10.1038/s41558-022-01290-z
Williams, A. P., Cook, E. R., Smerdon, J. E., Cook, B. I., Abatzoglou, J. T., Bolles, K., et al. (2020). Large contribution from anthropogenic warming to an emerging North American megadrought. Science 368, 314–318. doi: 10.1126/science.aaz9600
Young, A. L., Bloodworth, K. J., Frost, M. D. T., Green, C. E., and Koerner, S. E. (2022). Heatwave implications for the future of longleaf pine savanna understory restoration. Plant Ecol. 223, 339–351. doi: 10.1007/s11258-021-01212-7
Zachariah, M., Philip, S., Pinto, I., Vahlberg, M., Singh, R., Otto, F., et al. (2023). Extreme heat in North America, Europe and China in July 2023 made much more likely by climate change.
Keywords: climate change, drought, establishment, forest regeneration, heatwave, landscape gradient, survival, tree mortality
Citation: Lalor AR, Law DJ, Breshears DD, Falk DA, Field JP, Loehman RA, Triepke FJ and Barron-Gafford GA (2023) Mortality thresholds of juvenile trees to drought and heatwaves: implications for forest regeneration across a landscape gradient. Front. For. Glob. Change. 6:1198156. doi: 10.3389/ffgc.2023.1198156
Edited by:
YanLong Guo, Institute of Tibetan Plateau Research (CAS), ChinaReviewed by:
Lisa McCauley, The Nature Conservancy (United States), United StatesZhongsheng He, Fujian Agriculture and Forestry University, China
Copyright © 2023 Lalor, Law, Breshears, Falk, Field, Loehman, Triepke and Barron-Gafford. This is an open-access article distributed under the terms of the Creative Commons Attribution License (CC BY). The use, distribution or reproduction in other forums is permitted, provided the original author(s) and the copyright owner(s) are credited and that the original publication in this journal is cited, in accordance with accepted academic practice. No use, distribution or reproduction is permitted which does not comply with these terms.
*Correspondence: Alexandra R. Lalor, YWxsaWVsYWxvckBnbWFpbC5jb20=