- 1Theoretical Physics Division, Petersburg Nuclear Physics Institute, St. Petersburg, Russia
- 2Institute for Advanced Study, Technical University of Munich, Garching, Germany
- 3School of Life Sciences, Technical University of Munich, Freising, Germany
- 4Centro de Ciência do Sistema Terrestre, Instituto Nacional de Pesquisas Espaciais, São José dos Campos, São Paulo, Brazil
Along with the accumulation of atmospheric greenhouse gases, particularly carbon dioxide, the loss of primary forests and other natural ecosystems is a major disruption of the Earth's system and is causing global concern. Quantifying planetary warming from carbon emissions, global climate models highlight natural forests' high carbon storage potential supporting conservation policies. However, some model outcomes effectively deprioritize conservation of boreal and temperate forests by suggesting that increased albedo upon deforestation could cool the planet. A potential conflict of global cooling vs. regional forest conservation could harm environmental policies. Here we present theoretical and observational evidence to demonstrate that, compared to the carbon-related warming, modeling skills for assessing climatic impacts of deforestation is low. We argue that estimates for deforestation-induced global cooling result from the models' limited capacity to account for the global effect of cooling from evapotranspiration of intact forests. Specifically, transpiration of trees can change the greenhouse effect via small modifications of the vertical temperature profile. However, due to their convective parameterization (which postulates a certain critical temperature profile), global climate models do not properly capture this effect. This may lead to an underestimation of warming from the loss of forest evapotranspiration in both high and low latitudes. As a result, conclusions about deforestation-induced global cooling are not robust and could result in action that immediately worsened global warming. To avoid deepening the environmental crisis, these conclusions should not inform policies of vegetation cover management, especially as studies from multiple fields are accumulating that better quantify the stabilizing impact of natural ecosystems evolved to maintain environmental homeostasis. Given the critical state and our limited understanding of both climate and ecosystems, an optimal policy with immediate benefits would be a global moratorium on the exploitation of all natural forests.
1. Introduction
The Earth is suffering from climate destabilization and ecosystem degradation (Figure 1), and humanity seeks to stop both (IPBES, 2019; IPCC, 2021). Policies for global climate stabilization focus on decarbonization and are informed by the outcomes of global climate models that formalize our evolving understanding of the Earth's system—currently, by the model simulations from the 6th Coupled Model Intercomparison (CMIP6) for the 6th Assessment Report of the Intergovernmental Panel on Climate Change (IPCC, 2021). With the Intergovernmental Science-Policy Platform on Biodiversity and Ecosystem Services (IPBES) formed 24 years later than IPCC, the ecosystem preservation narrative is less formally developed (Wilhere, 2021). Proponents of ecosystem preservation often borrow from the decarbonization argumentation and invoke the carbon storage potential of natural forests as a major illustration of their climatic importance. For example, the ground-breaking proforestation initiative in the United States emphasizes how much carbon unexploited natural forests can remove from the atmosphere if allowed to develop to their full ecological potential (Moomaw et al., 2019; Faison et al., 2023).
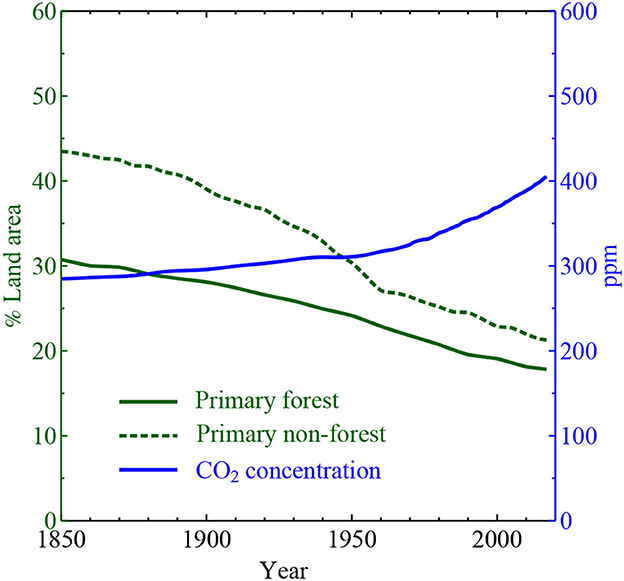
Figure 1. Decline of primary forest and non-forest ecosystems (left axis, data from Hurtt et al., 2020, their Figure 7) and increase of atmospheric CO2 (right axis, data downloaded from https://www.eea.europa.eu/data-and-maps/daviz/atmospheric-concentration-of-carbon-dioxide-5). During the industrial era, primary ecosystems have declined, and CO2 concentration has grown, by approximately one half.
At the same time, the carbon-storage argument for temperate and boreal forests is undermined by the fact that global climate models suggest that deforestation in these regions could cool the planet. Based on these models, increased albedo is estimated to overcome the warming caused by deforestation-induced carbon emissions (Jia et al., 2022, Figure 2.17) even if the latter can be underestimated (Schepaschenko et al., 2021). These model outcomes have been known for quite a while (e.g., Snyder et al., 2004), but recently these ideas clearly gained prominence and are even approaching implementation. A recent Science commentary warned that regrowing boreal forests would not make the Earth cooler (Pearce, 2022), a conclusion that is derived purely from global climate model simulations (e.g., De Hertog et al., 2022). The World Resources Institute's report “Not just carbon” noted that the increased albedo from deforestation would cool the Earth and emphasized that the positive climate role of boreal forests is only a local effect (Seymour et al., 2022a,b). Aligned with these modeling studies, a recent study in Nature Ecology and Evolution did not include primary boreal forests into Nature's critical assets (Chaplin-Kramer, 2023). One of the criteria for an ecoregion to be classified as a critical asset was its proximity to people—and primary boreal forests are often distant from any human settlements (which is a major reason for why they are still primary). Together, these models and mainstream messages not only de-emphasize the preservation of natural boreal and, to a lesser degree, temperate forests, but they implicitly prescribe and incentivize their destruction.
In this Perspective, we would like to ring an alarm bell by showing that this potentially biased picture of the role of natural forests, in particular boreal forests, for stabilizing Earth's climate is based on a few model assumptions that rule out important evapotranspiration feedbacks and can result in policies deepening rather than mitigating the climate crisis. We also outline a clear and possible path forward.
2. Global cooling from plant transpiration
2.1. Local vs. global cooling
We argue that the conclusion of a cooler Earth upon the loss of boreal forests stems from the limited capacity of global climate models to quantify another forest-related effect acting in the opposite direction: global cooling from forest transpiration. The ability of transpiring plants to provide local cooling is well-known (e.g., Alkama and Cescatti, 2016; Huryna and Pokorný, 2016; Bright et al., 2017; Ellison et al., 2017; Hesslerová et al., 2018, and see Figure 2). Instead of converting to heat, some of the absorbed solar energy is spent on breaking the intermolecular (hydrogen) bonds between the water molecules during evapotranspiration. As a result, the evaporating surface cools.
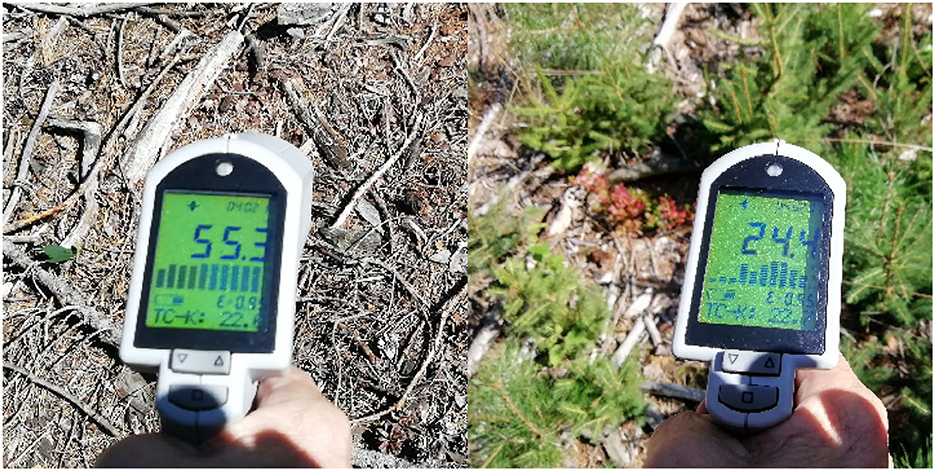
Figure 2. Local cooling from plant transpiration. With incoming solar radiation of about 1 kW m−2, dry area on the deforested plot (left) has temperature of 55.3°C. Young transpiring trees (right) lower the surface temperature by almost 30°C. Distance between the two spots is 1 m. Measurements and photo credit Jan Pokorný.
When more sunlight is reflected back to space, the planet receives less energy and it is intuitively clear that it must cool. In comparison, evaporation cools locally, but the captured energy does not disappear: it is released upon condensation elsewhere in the Earth's system. The methodology of explaining why the planet warms with increasing CO2 is well-developed (e.g., Benestad, 2017, and references therein). In contrast, how and whether loss of plant transpiration could warm or cool the planet remains conceptually unclear. While the IPCC reports recognize that global cooling from plant transpiration exists (Jia et al., 2022, Figure 2.17), its physical mechanism is not to be found in literature. Nevertheless, the environmental science is inherently transdisciplinary, and understanding this effect is important for the broader community of ecosystem researchers and conservationists, as it will enable a critical assessment of model outputs offered to guide large-scale vegetation management.
2.2. Methods
To illustrate the global thermal effect of transpiration, we will use a simple model of energy transfer (Figure 3). The greenhouse substances are represented by discrete layers that absorb all incoming thermal radiation and radiate all absorbed energy equally up and down. All the imaginary planets shown in Figure 3 are in a steady state: none is warming or cooling. In the absence of absorbers, the Earth's surface emits as much thermal radiation as it absorbs solar radiation (Figure 3A). Each layer of the greenhouse substances redirects part of the thermal radiation back to the Earth's surface. As a result, the planetary surface is warmer according to the greater the amount of absorbers (cf. Figures 3B, C).
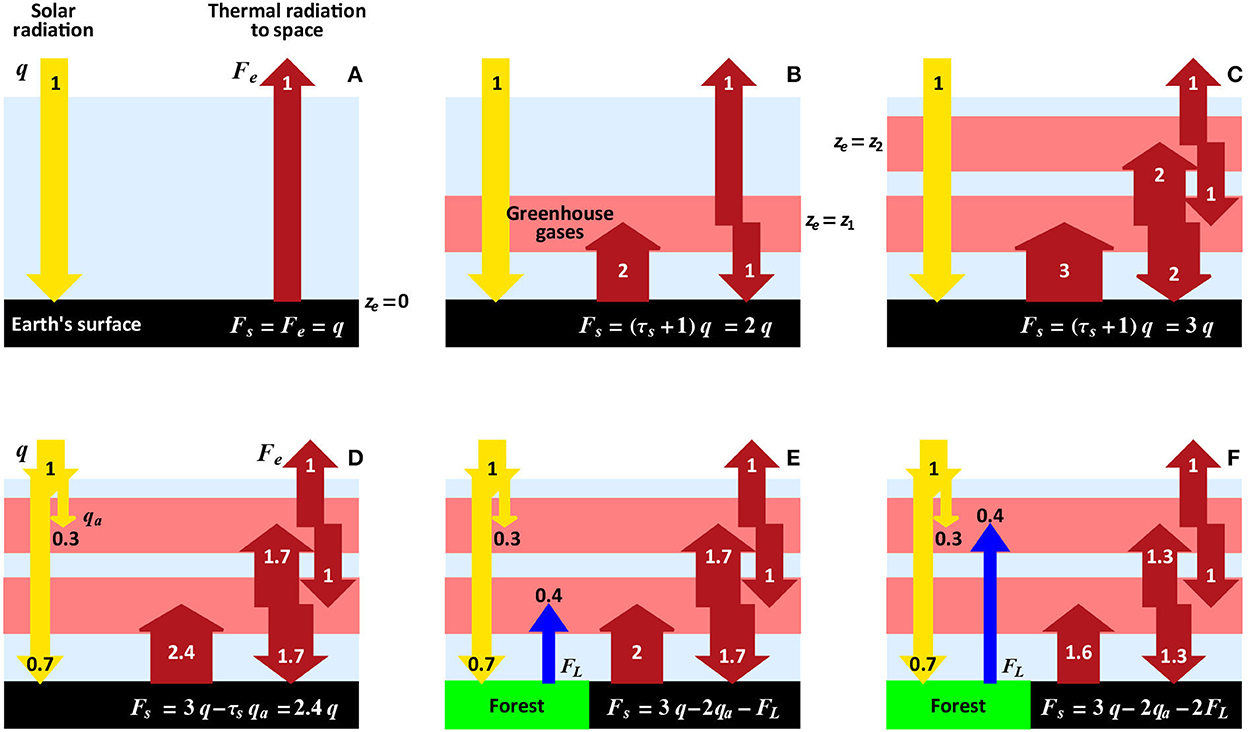
Figure 3. Scheme to illustrate the dependence of the planetary surface temperature on the amount of greenhouse substances (A–C) and on the magnitude and spatial distribution of the non-radiative energy fluxes (D–F). Thickness of each layer of the greenhouse substances corresponds to unit optical depth τ = 1 (one free path of thermal photons—the mean distance between two consecutive acts of absorption and re-emission by the absorber molecules); τs is the total number of layers: τs = 0 in (A), 1 in (B), and 2 in (C–F). A “gray” atmosphere is assumed, where absorption of thermal radiation is the same for all wavelengths (Ramanathan and Coakley, 1978; Makarieva and Gorshkov, 2001; Gorshkov et al., 2002). Thermal radiation of the planetary surface (W m−2) and of the upper radiative layer to space are related to surface temperature Ts and temperature of the upper radiative layer Te by Stefan–Boltzmann law, where σ = 5.7 × 10−8 W m−2 K−4 is the Stefan–Boltzmann constant. All energy fluxes are shown in the units of absorbed solar radiation q, which is in the steady state equal to thermal radiation emitted by the planet (Fe = q); in (D–F), qa = 0.3q is solar energy absorbed by the atmosphere (not to be confused with the reflected solar radiation (albedo), which is assumed to be constant and not shown); in (E, F), FL is the non-radiative heat flux accounting for both sensible and latent heat. Thermal radiation is emitted to space from mean height ze with temperature Te: ze = 0 in (A), ze = z1 > 0 in (B), and ze = z2 > z1 in (C–F).
When a certain part of the incoming solar radiation is absorbed in the upper atmosphere (for example, by aerosols or clouds), it escapes interaction with the absorbers beneath. Accordingly, in such a case the planetary surface is colder by an amount by which the absorbers would multiply this escaping part if it dissipated to thermal radiation at the surface (cf. Figures 3C, D). Figures 3C, D show that, with unchanged amount of greenhouse gases (e.g., carbon dioxide) and unchanged total flux q of absorbed solar energy, the planetary surface temperature depends on where solar energy dissipates to thermal radiation.
Similarly, in the presence of the non-radiative fluxes of sensible and latent heat, the amount of solar energy converted to thermal radiation at the surface diminishes—and so does the amount of thermal radiation redirected by the absorbers back to the surface. Thus, surface thermal radiation and temperature are smaller (cf. Figures 3D–F). The non-radiative fluxes “hide” a certain part of absorbed solar energy from the greenhouse substances easing its ultimate release to space. Convection, condensation and precipitation “deposit the latent heat removed from the surface above the level of the main water vapor absorbers, whence it is radiated to space” (Bates, 2003). This energy escaping partially from interaction with the absorbers is the physical mechanism behind global cooling from plant transpiration.
A related process is the atmospheric transport of heat from the equator to higher latitudes, where the water vapor concentration in the colder atmosphere is smaller. This transport likewise “hides” a certain part of solar energy absorbed at the equator from the abundant greenhouse substances (water vapor) in the warm tropical atmosphere. As a result, despite the amount of absorbers does not change, the globally averaged greenhouse effect diminishes and the planetary surface cools (Bates, 1999; Caballero, 2001). The potential of this effect was illustrated by Marvel et al. (2013), who modeled an idealized atmosphere with two strong circulation cells connecting the equator and the poles. With such a circulation, the Earth's surface became eleven degrees Kelvin cooler than the modern Earth (Marvel et al., 2013, their Figure 1e and Figure 3 bottom).
An increase in the non-radiative flux FL (from FL = 0 in Figure 3D to FL ≃ 0.4q > 0 in Figures 3E, F) decreases surface thermal radiation Fs by a magnitude proportional to the flux change ΔFL and to the number Δτ of absorbing layers beneath the height where this flux dissipates to thermal radiation (Δτ = 1 in Figure 3E and Δτ = 2 in Figure 3F). Historical deforestation affected about 13% of land area km2 (or 3.8% of planetary surface km2) (Figure 1). With the global mean latent flux of FL = 80 W m−2, if deforestation has reduced this flux by thirty per cent (ΔFL ~ −0.3FL), this could increase the surface radiation by −0.038ΔFLΔτ ~ 0.9 W m−2 (cf. Figures 3D, E, Δτ = 1) or twice that number (cf. Figures 3D, F, Δτ = 2), Table 1. Given an equilibrium climate sensitivity ε ~ 1 K/(W m−2) (Zelinka et al., 2020), the latter case corresponds to a warming of about two degrees Kelvin (Table 1). If the optical thickness of the atmosphere, and, accordingly, the magnitude of Δτ, is greater, the cooling can be proportionally larger.
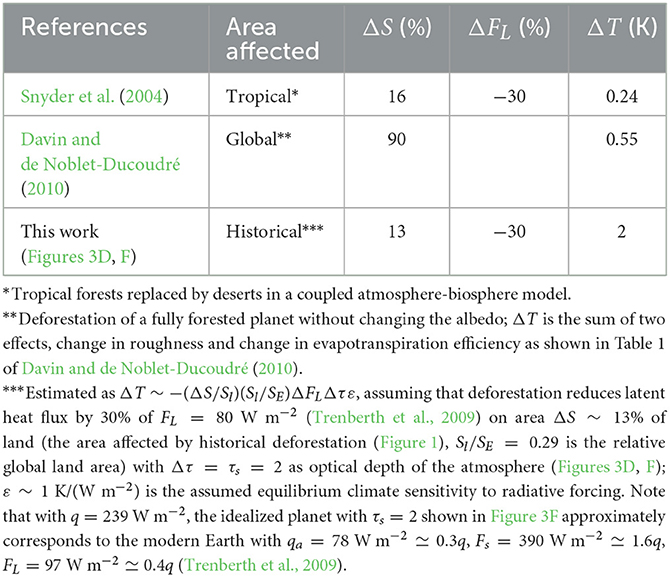
Table 1. Estimates of global warming from the loss of tree transpiration associated with deforestation; ΔFL (% of the global mean value FL) is the local reduction of latent heat on the deforested area ΔS (% of land area Sl); ΔT is the change of global surface temperature upon deforestation.
In a steady state with the unchanged amount of greenhouse substances, temperature Te and height ze of the upper radiative layer remain constant (e.g., Figures 3C–F). Therefore, surface warming caused by loss of plant transpiration should be manifested as an increase in the temperature difference between the surface and the upper radiative layer, i.e., the mean temperature lapse rate1 should grow (see discussion and Figure 5 in Section 2.4).
2.3. Dependence of global transpirational cooling on atmospheric circulation
The higher up in the air column that convection transports heat, the more pronounced global cooling it exerts. This is because the energy is radiated more directly to space from the upper atmospheric layer (cf. Figures 3E, F). In addition to the altitude, it matters how rapidly the cooled air descends. When the air rises and increases its potential energy in the gravitational field, its internal energy accordingly declines, and it cools. While evaporation cools the evaporating surface, the release of latent heat during condensation in the rising air partially offsets this decline of the internal energy of air molecules, making the rising air warmer than it would be without condensation. Radiating this extra thermal energy to space takes time. The more time spent by the air warmed by latent heat release in the upper atmosphere (above the main absorbers), the more energy is radiated unimpeded to space and the stronger the global transpirational cooling. With the characteristic radiative cooling rate of the order of 2 K day−1, it takes about 15–30 days to radiate the latent heat released by tropical moist convection (Goody, 2003).
Therefore, long-distance moisture transport (including the biotic pump run by forests, Makarieva and Gorshkov, 2007) enhances global transpirational cooling: moist air travels for many days, and thousands of kilometers from the ocean to land, where it ascends and latent heat is released. The dry air warmed by latent heat makes the same long way back in the upper atmosphere, thereby radiating energy to space (Figure 4). If, on the contrary, the warmed air descends rapidly and locally, then most heat is brought back to the surface before it is radiated, and the global power of the net cooling effect can be nullified. Therefore, disruptions in the long-distance moisture transport (e.g., by deforestation) and violent local rains should warm the Earth. In smaller convective clouds up to a quarter of ascending air descends locally at a relatively high vertical velocity (Heus and Jonker, 2008; Katzwinkel et al., 2014). These effects are not taken into account when assessing the temperature effects of land cover changes (e.g., Bright et al., 2017).
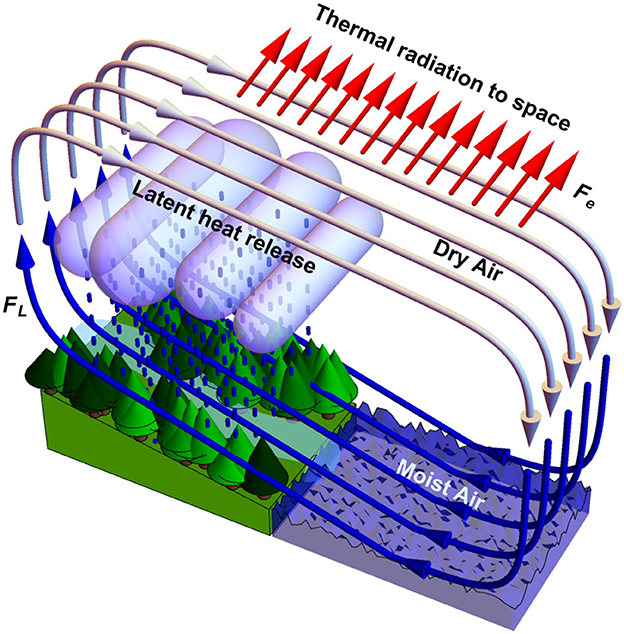
Figure 4. Visualization of the link between transpirational cooling and air circulation. After latent heat is released upon condensation, this energy can be radiated to space while the air travels back to the ocean in the upper troposphere.
Current global climate models do not correctly reproduce either the long-distance ocean-to-land moisture transport or the moisture transport over the ocean (Sohail et al., 2022). For example, the Amazon streamflow is underestimated by up to 50% (Marengo, 2006; Hagemann et al., 2011, their Figure 5). This corresponds to a 10% error in the global continental streamflow, the latter being of the same order as global continental evaporation. Similarly, global climate models do not correctly reproduce how the local diurnal cycle of convection changes upon deforestation by producing extreme low and high temperatures (Lejeune et al., 2017, their Figure 7). These are indirect indications of the models' limited capacity to reproduce global transpirational cooling. We will now discuss the cause of this limited capacity.
2.4. Global transpirational cooling in global climate models
We have seen that, for a given amount of absorbers, surface temperature is determined by the vertical distribution of the non-radiative heat fluxes (Figures 3D–F). But these fluxes themselves depend on the vertical temperature gradient: if the air temperature declines with height faster than a certain critical lapse rate of air temperature, the atmosphere is unstable to convection. The non-radiative heat fluxes originate proportional to the difference between the actual and the critical temperature lapse rates (Ramanathan and Coakley, 1978).
Therefore, strictly speaking, it is not justified to freely vary where and how the non-radiative heat fluxes dissipate to thermal radiation, not paying attention to whether the resulting vertical temperature profile is physically consistent with the convective fluxes specified a priori. However, since the non-radiative (convective) and net radiative energy fluxes in the Earth's atmosphere are of the same order of magnitude (100 and 60 W m−2, respectively, Trenberth et al., 2009), a rough estimate of global transpirational cooling can be obtained from considering the radiative transfer alone as done in Figures 3D–F. (This would not be possible if the convective fluxes were an order of magnitude higher than the radiative flux). We emphasize that our goal here is not to obtain an accurate estimate of global transpirational cooling, but simply to present plausible arguments showing that it is overlooked and it can be large.
An exact estimate of what happens when the evapotranspiration and the latent heat flux are suppressed on a certain part of land area requires solving the problem simultaneously for the radiative-convective transfer and the temperature profile. This problem is too complicated for modern global climate models to address, therefore they apply the so-called convective parameterization. Convective parameterization in climate models postulates the (generally unknown) value of a critical temperature lapse rate instead of solving for it. While the numerical simulation is run, “whenever the radiative equilibrium lapse rate is greater than the critical lapse rate, the lapse rate is set equal to the critical lapse rate” (Ramanathan and Coakley, 1978). Therefore, by construction, global climate models cannot provide any independent information about the climatic effect of evapotranspirational cooling—that should be manifested as the change in the global mean lapse rate—besides what was fed into them a priori via convective parameterization.
The reason for this artificial constraint on the impact of evapotranspirational cooling is that global climate models have been built with a major goal of assessing radiative forcing from changing carbon dioxide concentrations. Accordingly, they do have this capacity: this forcing can be approximately estimated assuming an unchanged atmospheric temperature profile. It is under this assumption that Arrhenius (1896) obtained the first ever estimate of global warming from CO2 doubling2. But radiative forcing caused by the suppression of evapotranspiration is a conceptually different problem for which convective parameterization precludes a solution that would be non-zero in the first order. Therefore, in the models, global warming resulting from the loss of transpirational cooling is, for the same deforested area, at least one order of magnitude smaller than our estimate (Table 1). For example, according to global climate models, tropical deforestation on 16% of land area would produce a global warming of 0.2 K (Snyder et al., 2004), while converting about 90% of global land area from forest to grassland (with unchanged albedo) would warm the Earth by about half a degree Kelvin (Davin and de Noblet-Ducoudré, 2010), see Table 1.
As a further illustration of the lack of conceptual clarity with regard to global transpirational cooling, one can refer to the conclusion of Davin and de Noblet-Ducoudré (2010, their Table 1) that modeled global warming due to the loss of evapotranspiration is a “non-radiative” forcing. This conclusion is reached by noting that loss of evapotranspiration practically does not change the radiation balance at the top of the atmosphere: ΔFe → 0 such that ΔT/ΔFe ≫ ε. However, using this logic, CO2 increase would not be a radiative forcing either, because, once the planetary temperature equilibrates, CO2 increase per se (feedbacks absent) does not change the outgoing radiation at the top of the atmosphere. Indeed, Figure 3 illustrates how the steady-state planetary temperature changes due to the radiative forcing from an increased amount of greenhouse substances (Figures 3A–C) and due to the radiative forcing from changing non-radiative fluxes (Figures 3D–F). The incoming solar and outgoing longwave radiation remain the same in all cases (Fe = q and ΔFe = 0).
In current models, it is assumed that as the planet warms, the temperature lapse rate should slightly diminish following moist adiabat (the so-called lapse rate feedback, Sejas et al., 2021). While robust across models, this feature is not, however, supported by observations that indicate an increase in the lapse rate (Figure 5). The temperature difference between the surface and the upper radiative layer ze (located between 500 and 400 mb, Benestad, 2017) grows at approximately the same rate as the surface temperature itself. This effect is especially pronounced over land (Figures 5C, F) and is consistent with a radiative forcing imposed by changing non-radiative fluxes, including those due to the land cover change (Figures 3D–F). In addition to transpiration losses, the non-radiative fluxes of both latent and sensible heat are additionally reduced due to a decrease in surface roughness as the tree cover is removed during deforestation (Bright et al., 2017; Winckler et al., 2019).
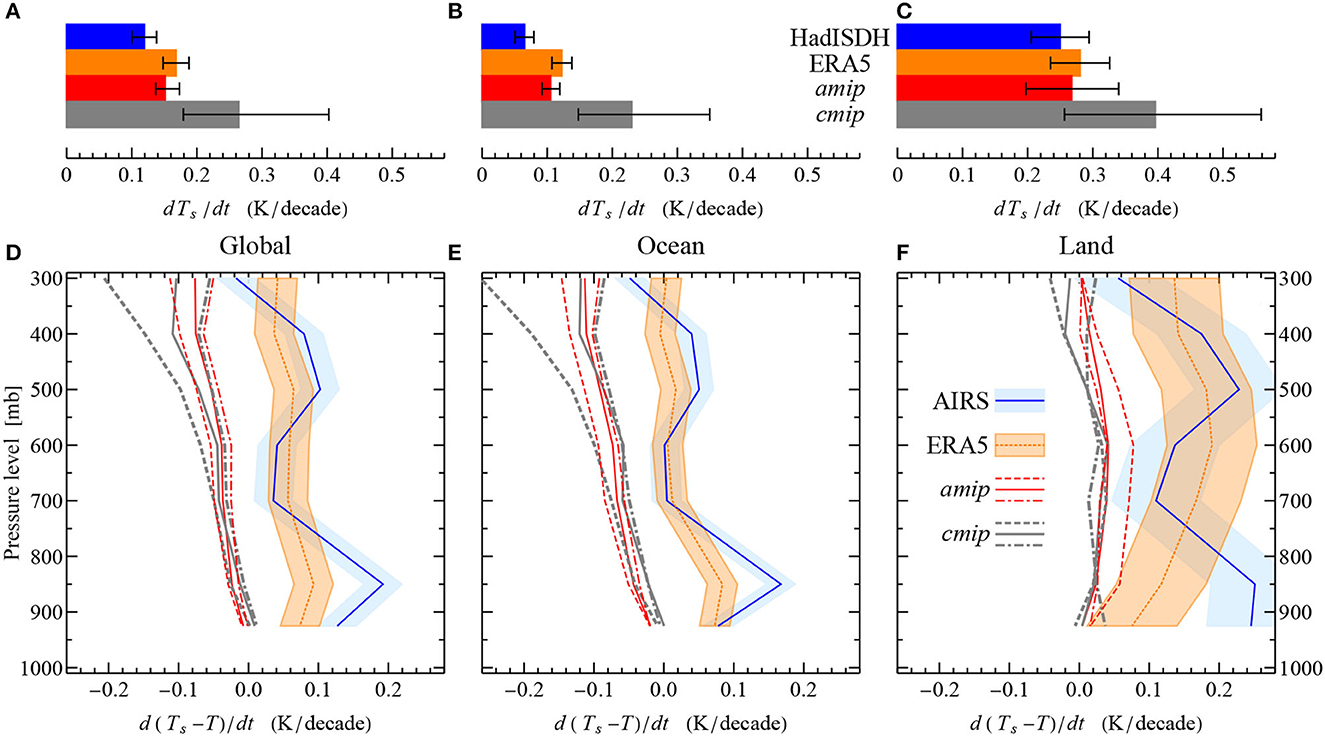
Figure 5. Mean trends of the surface temperature Ts (A–C) and of the temperature difference (Ts−T) between the surface and atmospheric pressure levels (D–F) for the planet as a whole (A, D), ocean (B, E), and land (C, F) over 1988–2014 in models (amip, cmip) vs. observations (AIRS, HadISDH, ERA5). In (A–C), whiskers for HadISDH and ERA5 indicate ± one standard deviation, for amip and cmip—the maximum and minimum values. Shading in (D–F) indicates ± one standard deviation. Dashed, solid and dash-dotted model curves in (D–F) were obtained, respectively, by using the maximum, median and minimum values of dTs/dt and dT/dt in the model ensembles (amip and cmip). Data from Supplementary Figure S4 of Allan et al. (2022): AIRS, Atmospheric Infrared Sounder satellite data (Tian et al., 2019); HadISDH, The Met Office Hadley Centre homogenized and quality controlled, integrated sub-daily data set (Willett et al., 2014); ERA5, the fifth generation European Center for Medium-range Weather Forecasts global reanalysis (Hersbach et al., 2020); amip, Atmospheric Model Intercomparison Project, atmosphere-only simulations (without the ocean-atmosphere feedbacks in the climate system) (Gates et al., 1999); cmip, Phase 6 of the Coupled Model Intercomparison Project (includes amip simulations as an integral part) (Eyring et al., 2016). See Allan et al. (2022) for further details. In (D–F), d(Ts−T)/dt for AIRS is calculated using HadISDH dTs/dt. Note that altitude z(p) of a given pressure level p increases slightly as the surface temperature grows, but for p <300 mb it is a minor effect compared to the increase of the temperature difference Ts−T(p).
3. Discussion and conclusions
Recognizing that for the ecological audience it could be difficult to assess the credibility of our quantitative estimates, we would like to emphasize two of the more unequivocal points. First, global climate models do indicate that the regional loss of forest evapotranspiration leads to global warming. Although the global effect is small (Table 1), it is of the opposite sign compared to the albedo-related cooling from deforestation that is invoked to argue that certain forests (boreal in particular) are not globally beneficial in the climate change context. Despite this obvious importance for policy-relevant model outcomes, a conceptual description of how evapotranspiration cools the Earth, and how its loss would lead to global warming, is absent from the meteorological literature. If and when conceptual understanding is lacking, how can one independently assess whether the models get the effect right?
Second, we have discussed that, from the first principles, we can expect global warming resulting from the loss of evapotranspiration to manifest itself as an increase in the vertical lapse rate of air temperature. Due to the convective parameterization, global climate models keep this lapse rate roughly constant as the planet warms (Held and Soden, 2006; Jeevanjee et al., 2022). However, this model feature does not agree with observations that accommodate a considerable increase in the temperature difference between the surface and the upper radiative layer (Figure 5).
Land and energy policies based on the model outcomes that we have criticized are being shaped right now and an evaluation/reevaluation that avoids harm is paramount. While the above arguments continue to percolate in the meteorological literature, readers from all disciplines should be interested in evaluating and discussing these concerns and can approach their colleagues in the field of meteorology to see how they respond to the above two challenges, thus getting an indirect confirmation (or disproval) of our argumentation.
At the most basic level, our results highlight the importance of a valid concept at the core of a model. The assumption of an a priori specified critical lapse rate in the convective parameterization yields a negligible global transpirational cooling, which translates into de-emphasizing the preservation of boreal forests. Concepts are powerful; incorrect concepts can be destructive. This brings us to the question, is there a concept that ecology could offer to put at the core of a global climate model, to adequately represent the biosphere?
From our perspective, it is the concept of environmental homeostasis, which is the capacity of natural ecosystems to compensate for environmental disturbances and stabilize a favorable for life environment and climate (Lovelock and Margulis, 1974; Gorshkov, 1995). Recent studies discuss how biotic control can be evident in the observed dynamics of the Earth's temperature (Leggett and Ball, 2020, 2021; Arnscheidt and Rothman, 2022). When the information about how the natural ecosystem influences environment is lacking, the best guess could be to assume that they provide a stabilizing feedback to the disturbance.
There was already a predicament in climate science that could have been facilitated by such an approach. It was the missing sink problem: when the rates of carbon accumulation in the atmosphere and the ocean became known with sufficient accuracy, it turned out that a significant part of fossil fuel emissions could not be accounted for. The enigmatic missing sink was later assigned to the terrestrial biota (Popkin, 2015). While now it is commonly referred to as plant CO2 fertilization, this is a response at the level of the ecological community as a whole: for there to be a net sink, as the plants synthesize more organic matter, heterotrophs must refrain from decomposing it at a higher rate under the warming conditions (cf. Wieder et al., 2013). Surprisingly, while the idea that ecological succession proceeds in the direction of the ecosystem attaining increased control of the environment and maximum protection from environmental perturbations was dominant in ecology (Odum, 1969), a community's stabilizing response to the CO2 disturbance was not predicted but rather opposed by ecologists on the basis that undisturbed ecosystems should have a closed matter cycle3 (Hampicke, 1980; Amthor, 1995). However, based on the premises of the biotic regulation concept (Gorshkov, 1995; Gorshkov et al., 2000), and long before the missing sink was assigned to the terrestrial biota, Gorshkov (1986, p. 946) predicted that the undisturbed ecosystems should perform a compensatory response to rising atmospheric CO2 by elevating synthesis of carbohydrates.
Today, climate science faces a new challenge. Global climate models with an improved representation of clouds display a higher sensitivity of the Earth's climate to CO2 doubling than models with a poorer representation of clouds (Zelinka et al., 2020; Kuma et al., 2023). This implies more dire projections for future climate change, but also poses the problem of how to account for the past temperature changes that are not affected by the model improvements and have been satisfactorily explained assuming a lower climate sensitivity. The concept of the environmental homeostasis and the biotic regulation of the environment provide a possible solution: the climate sensitivity may have been increasing with time—reflecting the decline of natural ecosystems and their global stabilizing impact (Figure 1).
Currently, climate model uncertainties are assessed by comparing outputs from models developed by different research centers (Zelinka et al., 2020). This provides a minimal uncertainty estimate, as the model development may follow universal principles sharing both progress and errors. A distinct approach would be to attempt building a model that departs significantly from the others in its core concept and see if such a model can be plausibly tuned to competitively describe observations. Success of such a model would force the range of model uncertainties to be extended. As global climate models are currently being used to elaborate strategies for the survival of humanity as a whole, such a stress test on their performance would not be superfluous.
Such an endeavor requires a plausible alternative concept, and we propose that a global climate model built around the stabilizing impact of natural ecosystems can become such an alternative. This will require an interdisciplinary effort and an account of global transpirational cooling, the role of natural ecosystems in the long-distance moisture transport (Makarieva and Gorshkov, 2007; van der Ent et al., 2010; Ellison et al., 2012; Poveda et al., 2014; Molina et al., 2019; Makarieva et al., 2023) and water cycle stabilization (Zemp et al., 2017; Baudena et al., 2021; O'Connor et al., 2021) and the distinct impact of ecosystems at different stages of ecological succession on the surface temperature and fire regime (e.g., Aleinikov, 2019; Baker and Spracklen, 2019; Lindenmayer et al., 2022) and the cloud cover (Cerasoli et al., 2021; Duveiller et al., 2021). Living systems function on the basis of solar energy that under terrestrial conditions can be converted to useful work with a near 100% efficiency. What processes are enacted with use of this energy, is determined by the genetic programs of all the organisms composing the ecological community. Randomly changing the species composition and morphological status of living organisms in the community—for example, by converting to developed land (removing natural elements altogether), replacing natural forest with a plantation, clearing large expanses for farmland, or forcing areas of forest to remain in an early successional or degraded state—disturbs the flow of environmental information and disrupts the ecosystem's capacity to respond to environmental disturbances (Makarieva et al., 2020; Kellett et al., 2023). We need a better understanding of this fundamental regulation and its tipping points.
While fundamental science is being advanced, the precautionary principle should be strictly applied. Any control system increases its feedback as the perturbation grows. Therefore, as the climate destabilization deepens, the remaining natural ecosystems should be exerting an ever increasing compensatory impact per unit area. In other words, the global climate price of losing a hectare of natural forest grows as the climate situation worsens. We call for an urgent global moratorium on the exploitation of the remaining natural ecosystems and a broad application of the proforestation strategy to allow them to restore to their full ecological and climate-regulating potential.
Data availability statement
The original contributions presented in the study are included in the article/supplementary material, further inquiries can be directed to the corresponding author.
Author contributions
AM wrote the first draft of the manuscript. All authors contributed to conception and design of the study. All authors contributed to manuscript revision, read, and approved the submitted version.
Funding
The work of AM was partially funded by the Federal Ministry of Education and Research (BMBF) and the Free State of Bavaria under the Excellence Strategy of the Federal Government and the Länder, as well as by the Technical University of Munich – Institute for Advanced Study.
Acknowledgments
The authors are grateful to Jan Pokorný, David Ellison, Ugo Bardi, Jon Schull, Zuzana Mulkerin, and Susan Butler for inspiring discussions of the transpirational cooling, and to Jennifer Francis for constructive critical comments on the manuscript. We thank Richard P. Allan for kindly supplying the data for trends in atmospheric and near surface temperature in numerical form. Comments and suggestions from two anonymous referees are gratefully acknowledged.
Conflict of interest
The authors declare that the research was conducted in the absence of any commercial or financial relationships that could be construed as a potential conflict of interest.
Publisher's note
All claims expressed in this article are solely those of the authors and do not necessarily represent those of their affiliated organizations, or those of the publisher, the editors and the reviewers. Any product that may be evaluated in this article, or claim that may be made by its manufacturer, is not guaranteed or endorsed by the publisher.
Footnotes
1. ^Temperature lapse rate Γ is the absolute magnitude of the vertical temperature gradient, Γ≡−∂T/∂z.
2. ^If the lapse rate Γ is known, an alternative way to calculate how surface temperature rises with increasing concentration of greenhouse substances is to calculate the change of radiative height Δze = z2−z1 (cf. Figures 3B, C) using the hydrostatic equilibrium; then use ΔTs = ΓΔze.
3. ^This represents what can be called Odum's paradox, who thought that ecological succession culminates in ecosystem's maximum control of the environment (Odum, 1969). But if the ecosystem functions on the basis of closed matter cycles, its environmental impact (and, hence, environmental control) is zero by definition. The biotic regulation concept introduced the notion of directed openness of the matter cycles to compensate for environmental disturbances (Gorshkov, 1995).
References
Aleinikov, A. A. (2019). The fire history in pine forests of the plain area in the Pechora-Ilych Nature Biosphere Reserve (Russia) before 1942: Possible anthropogenic causes and long-term effects. Nat. Conserv. Res. 4, 21–34. doi: 10.24189/ncr.2019.033
Alkama, R., and Cescatti, A. (2016). Biophysical climate impacts of recent changes in global forest cover. Science 351, 600–604. doi: 10.1126/science.aac8083
Allan, R. P., Willett, K. M., John, V. O., and Trent, T. (2022). Global changes in water vapor 1979–2020. J. Geophys. Res. Atmos. 127, e2022JD036728. doi: 10.1029/2022JD036728
Amthor, J. S. (1995). Terrestrial higher-plant response to increasing atmospheric [CO2] in relation to the global carbon cycle. Glob. Change Biol. 1, 243–274. doi: 10.1111/j.1365-2486.1995.tb00025.x
Arnscheidt, C. W., and Rothman, D. H. (2022). Presence or absence of stabilizing Earth system feedbacks on different time scales. Sci. Adv. 8, eadc9241. doi: 10.1126/sciadv.adc9241
Arrhenius, S. (1896). XXXI. On the influence of carbonic acid in the air upon the temperature of the ground. Lond. Edinb. Dublin Philos. Mag. J. Sci. 41, 237–276. doi: 10.1080/14786449608620846
Baker, J. C. A., and Spracklen, D. V. (2019). Climate benefits of intact Amazon forests and the biophysical consequences of disturbance. Front. For. Glob. Change 2, 47. doi: 10.3389/ffgc.2019.00047
Bates, J. R. (1999). A dynamical stabilizer in the climate system: a mechanism suggested by a simple model. Tellus A: Dyn. Meteorol. Oceanogr. 51, 349–372. doi: 10.3402/tellusa.v51i3.13458
Bates, J. R. (2003). On Climate Stability, Climate Sensitivity and the Dynamics of the Enhanced Greenhouse Effect. DCESS Report No. 3. Department of Geophysics, University of Copenhagen, 1–38.
Baudena, M., Tuinenburg, O. A., Ferdinand, P. A., and Staal, A. (2021). Effects of land-use change in the Amazon on precipitation are likely underestimated. Glob. Change Biol. 27, 5580–5587. doi: 10.1111/gcb.15810
Benestad, R. E. (2017). A mental picture of the greenhouse effect. Theor. Appl. Climatol. 128, 679–688. doi: 10.1007/s00704-016-1732-y
Bright, R. M., Davin, E., O'Halloran, T., Pongratz, J., Zhao, K., and Cescatti, A. (2017). Local temperature response to land cover and management change driven by non-radiative processes. Nat. Clim. Change 7, 296–302. doi: 10.1038/nclimate3250
Caballero, R. (2001). Surface wind, subcloud humidity and the stability of the tropical climate. Tellus A: Dyn. Meteorol. Oceanogr. 53, 513–525. doi: 10.3402/tellusa.v53i4.12224
Cerasoli, S., Yin, J., and Porporato, A. (2021). Cloud cooling effects of afforestation and reforestation at midlatitudes. Proc. Natl. Acad. Sci. U.S.A. 118, e2026241118. doi: 10.1073/pnas.2026241118
Chaplin-Kramer, R., Neugarten, R. A., Sharp, R. P., Collins, P. M., Polasky, S., Hole, D., et al. (2023). Mapping the planet's critical natural assets. Nat. Ecol. Evol. 7, 51–61. doi: 10.1038/s41559-022-01934-5
Davin, E. L., and de Noblet-Ducoudré, N. (2010). Climatic impact of global-scale deforestation: radiative versus nonradiative processes. J. Clim. 23, 97–112. doi: 10.1175/2009JCLI3102.1
De Hertog, S. J., Havermann, F., Vanderkelen, I., Guo, S., Luo, F., Manola, I., et al. (2022). The biogeophysical effects of idealized land cover and land management changes in Earth system models. Earth Syst. Dyn. 13, 1305–1350. doi: 10.5194/esd-13-1305-2022
Duveiller, G., Filipponi, F., Ceglar, A., Bojanowski, J., Alkama, R., and Cescatti, A. (2021). Revealing the widespread potential of forests to increase low level cloud cover. Nat. Commun. 12, 4337. doi: 10.1038/s41467-021-24551-5
Ellison, D., Morris, C. E., Locatelli, B., Sheil, D., Cohen, J., Murdiyarso, D., et al. (2017). Trees, forests and water: cool insights for a hot world. Glob. Environ. Change 43, 51–61. doi: 10.1016/j.gloenvcha.2017.01.002
Ellison, D., Futter, M. N., and Bishop, K. (2012). On the forest cover–water yield debate: from demand- to supply-side thinking. Glob. Change Biol. 18, 806–820. doi: 10.1111/j.1365-2486.2011.02589.x
Eyring, V., Bony, S., Meehl, G. A., Senior, C. A., Stevens, B., Stouffer, R. J., et al. (2016). Overview of the Coupled Model Intercomparison Project Phase 6 (CMIP6) experimental design and organization. Geosci. Model Dev. 9, 1937–1958. doi: 10.5194/gmd-9-1937-2016
Faison, E. K., Masino, S. A., and Moomaw, W. R. (2023). The importance of natural forest stewardship in adaptation planning in the United States. Conserv. Sci. Pract. 5, e12935. doi: 10.1111/csp2.12935
Gates, W. L., Boyle, J. S., Covey, C., Dease, C. G., Doutriaux, C. M., Drach, R. S., et al. (1999). An overview of the results of the Atmospheric Model Intercomparison Project (AMIP I). Bull. Amer. Meteor. Soc. 80, 29–56. doi: 10.1175/1520-0477(1999)080<0029:AOOTRO>2.0.CO;2
Goody, R. (2003). On the mechanical efficiency of deep, tropical convection. J. Atmos. Sci. 60, 2827–2832. doi: 10.1175/1520-0469(2003)060<2827:OTMEOD>2.0.CO;2
Gorshkov, V., Makarieva, A., and Pujol, T. (2002). “Radiative-convective processes and changes of the flux of thermal radiation into space with increasing optical thickness of the atmosphere,” Proceedings of the XXXVI Winter School of Petersburg Nuclear Physics Institute (Nuclear and Particle Physics) (St. Petersburg), 499–525.
Gorshkov, V. G. (1986). Atmospheric disturbance of the carbon cycle: impact upon the biosphere. Nuov. Cim. C 9, 937–952. doi: 10.1007/BF02891905
Gorshkov, V. G. (1995). Physical and Biological Bases of Life Stability: Man, Biota, Environment. Berlin; Heidelberg: Springer. doi: 10.1007/978-3-642-85001-1
Gorshkov, V. G., Gorshkov, V. V., and Makarieva, A. M. (2000). Biotic Regulation of the Environment: Key Issue of Global Change. Berlin: Springer.
Hagemann, S., Chen, C., Haerter, J. O., Heinke, J., Gerten, D., and Piani, C. (2011). Impact of a statistical bias correction on the projected hydrological changes obtained from three GCMs and two hydrology models. J. Hydrometeor. 12, 556–578. doi: 10.1175/2011JHM1336.1
Hampicke, U. (1980). The effect of the atmosphere-biosphere exchange on the global carbon cycle. Experientia 36, 776–781. doi: 10.1007/BF01978577
Held, I. M., and Soden, B. J. (2006). Robust responses of the hydrological cycle to global warming. J. Clim. 19, 5686–5699. doi: 10.1175/JCLI3990.1
Hersbach, H., Bell, B., Berrisford, P., Hirahara, S., Horányi, A., Muñoz-Sabater, J., et al. (2020). The ERA5 global reanalysis. Q. J. Roy. Meteor. Soc. 146, 1999–2049. doi: 10.1002/qj.3803
Hesslerová, P., Huryna, H., Pokorný, J., and Procházka, J. (2018). The effect of forest disturbance on landscape temperature. Ecol. Eng. 120, 345–354. doi: 10.1016/j.ecoleng.2018.06.011
Heus, T., and Jonker, H. J. J. (2008). Subsiding shells around shallow cumulus clouds. J. Atmos. Sci. 65, 1003–1018. doi: 10.1175/2007JAS2322.1
Hurtt, G. C., Chini, L., Sahajpal, R., Frolking, S., Bodirsky, B. L., Calvin, K., et al. (2020). Harmonization of global land use change and management for the period 850–2100 (LUH2) for CMIP6. Geosci. Model Dev. 13, 5425–5464. doi: 10.5194/gmd-13-5425-2020
Huryna, H., and Pokorný, J. (2016). The role of water and vegetation in the distribution of solar energy and local climate: a review. Folia Geobot. 51, 191–208. doi: 10.1007/s12224-016-9261-0
IPBES (2019). Global Assessment Report on Biodiversity and Ecosystem Services of the Intergovernmental Science-Policy Platform on Biodiversity and Ecosystem Services. IPBES Secretariat, Bonn. doi: 10.5281/zenodo.6417333
IPCC (2021). Climate Change 2021: The Physical Science Basis. Contribution of Working Group I to the Sixth Assessment Report of the Intergovernmental Panel on Climate Change. Cambridge University Press, Cambridge, UK; New York, NY. doi: 10.1017/9781009157896
Jeevanjee, N., Held, I., and Ramaswamy, V. (2022). Manabe's radiative-convective equilibrium. Bull. Amer. Meteor. Soc. 103, E2559–E2569. doi: 10.1175/BAMS-D-21-0351.1
Jia, G., Shevliakova, E., Artaxo, P., De Noblet-Ducoudré, N., Houghton, R., House, J., et al. (2022). “Chapter 2: Land-climate interactions,” in Climate Change and Land: An IPCC Special Report on Climate Change, Desertification, Land Degradation, Sustainable Land Management, Food Security, and Greenhouse Gas Fluxes in Terrestrial Ecosystems, eds P. R. Shukla, J. Skea, E. Calvo Buendia, V. Masson-Delmotte, H. O. Pörtner, D. C. Roberts, P. Zhai, R. Slade, S. Connors, R. van Diemen, M. Ferrat, E. Haughey, S. Luz, S. Neogi, M. Pathak, J. Petzold, J. Portugal Pereira, P. Vyas, E. Huntley, K. Kissick, M. Belkacemi, and J. Malley (Cambridge, UK: Cambridge University Press), 131–248. doi: 10.1017/9781009157988.004
Katzwinkel, J., Siebert, H., Heus, T., and Shaw, R. A. (2014). Measurements of turbulent mixing and subsiding shells in trade wind cumuli. J. Atmos. Sci. 71, 2810–2822. doi: 10.1175/JAS-D-13-0222.1
Kellett, M. J., Maloof, J. E., Masino, S. A., Frelich, L. E., Faison, E. K., Brosi, S. L., et al. (2023). Forest-clearing to create early-successional habitats: questionable benefits, significant costs. Front. For. Glob. Change 5, 1073677. doi: 10.3389/ffgc.2022.1073677
Kuma, P., Bender, F. A.-M., Schuddeboom, A., McDonald, A. J., and Seland, Ø. (2023). Machine learning of cloud types in satellite observations and climate models. Atmos. Chem. Phys. 23, 523–549. doi: 10.5194/acp-23-523-2023
Leggett, L. M. W., and Ball, D. A. (2020). Observational evidence that a feedback control system with proportional-integral-derivative characteristics is operating on atmospheric surface temperature at global scale. Tellus A: Dyn. Meteorol. Oceanogr. 72, 1–14. doi: 10.1080/16000870.2020.1717268
Leggett, L. M. W., and Ball, D. A. (2021). Empirical evidence for a global atmospheric temperature control system: physical structure. Tellus A: Dyn. Meteorol. Oceanogr. 73, 1–24. doi: 10.1080/16000870.2021.1926123
Lejeune, Q., Seneviratne, S. I., and Davin, E. L. (2017). Historical land-cover change impacts on climate: comparative assessment of LUCID and CMIP5 multimodel experiments. J. Clim. 30, 1439–1459. doi: 10.1175/JCLI-D-16-0213.1
Lindenmayer, D. B., Bowd, E. J., Taylor, C., and Likens, G. E. (2022). The interactions among fire, logging, and climate change have sprung a landscape trap in Victoria's montane ash forests. Plant Ecol. 223, 733–749. doi: 10.1007/s11258-021-01217-2
Lovelock, J. E., and Margulis, L. (1974). Atmospheric homeostasis by and for the biosphere: the gaia hypothesis. Tellus 26, 2–10. doi: 10.3402/tellusa.v26i1-2.9731
Makarieva, A. M., and Gorshkov, V. G. (2001). The greenhouse effect and the stability of the global mean surface temperature. Dokl. Earth Sci. 377, 210–214.
Makarieva, A. M., and Gorshkov, V. G. (2007). Biotic pump of atmospheric moisture as driver of the hydrological cycle on land. Hydrol. Earth Syst. Sci. 11, 1013–1033. doi: 10.5194/hess-11-1013-2007
Makarieva, A. M., Nefiodov, A. V., Morozov, V. E., Aleynikov, A. A., and Vasilov, R. G. (2020). Science in the vanguard of rethinking the role of forests in the third millennium: comments on the draft concept of the federal law “Forest code of the Russian Federation”. Forest Sci. Iss. 3. doi: 10.31509/2658-607x-2020-3-3-1-25
Makarieva, A. M., Nefiodov, A. V., Nobre, A. D., Baudena, M., Bardi, U., Sheil, D., et al. (2023). The role of ecosystem transpiration in creating alternate moisture regimes by influencing atmospheric moisture convergence. Glob. Change Biol. 29, 2536–2556. doi: 10.1111/gcb.16644
Marengo, J. A. (2006). On the hydrological cycle of the Amazon Basin: a historical review and current state-of-the-art. Rev. Bras. Meteorol. 21, 1–19.
Marvel, K., Kravitz, B., and Caldeira, K. (2013). Geophysical limits to global wind power. Nat. Clim. Change 3, 118–121. doi: 10.1038/nclimate1683
Molina, R. D., Salazar, J. F., Martínez, J. A., Villegas, J. C., and Arias, P. A. (2019). Forest-induced exponential growth of precipitation along climatological wind streamlines over the Amazon. J. Geophys. Res. Atmos. 124, 2589–2599. doi: 10.1029/2018JD029534
Moomaw, W. R., Masino, S. A., and Faison, E. K. (2019). Intact forests in the United States: proforestation mitigates climate change and serves the greatest good. Front. For. Glob. Change 2, 27. doi: 10.3389/ffgc.2019.00027
O'Connor, J. C., Dekker, S. C., Staal, A., Tuinenburg, O. A., Rebel, K. T., and Santos, M. J. (2021). Forests buffer against variations in precipitation. Glob. Change Biol. 27, 4686–4696. doi: 10.1111/gcb.15763
Odum, E. P. (1969). The strategy of ecosystem development: an understanding of ecological succession provides a basis for resolving man's conflict with nature. Science 164, 262–270. doi: 10.1126/science.164.3877.262
Poveda, G., Jaramillo, L., and Vallejo, L. F. (2014). Seasonal precipitation patterns along pathways of South American low-level jets and aerial rivers. Water Resour. Res. 50, 98–118. doi: 10.1002/2013WR014087
Ramanathan, V., and Coakley, J. A. Jr. (1978). Climate modeling through radiative-convective models. Rev. Geophys. 16, 465–489. doi: 10.1029/RG016i004p00465
Schepaschenko, D., Moltchanova, E., Fedorov, S., Karminov, V., Ontikov, P., Santoro, M., et al. (2021). Russian forest sequesters substantially more carbon than previously reported. Sci. Rep. 11, 12825. doi: 10.1038/s41598-021-92152-9
Sejas, S. A., Hu, X., Cai, M., and Fan, H. (2021). Understanding the differences between TOA and surface energy budget attributions of surface warming. Front. Earth Sci. 9, 725816. doi: 10.3389/feart.2021.725816
Seymour, F., Wolosin, M., and Gray, E. (2022a). Policies Underestimate Forests' Full Effect on the Climate. Available online at: https://www.wri.org/insights/how-forests-affect-climate
Seymour, F., Wolosin, M., and Gray, E. (2022b). Not Just Carbon: Capturing All the Benefits of Forests for Stabilizing the Climate from Local to Global Scales. Washington, DC, doi: 10.46830/wrirpt.19.00004
Snyder, P. K., Delire, C., and Foley, J. A. (2004). Evaluating the influence of different vegetation biomes on the global climate. Clim. Dyn. 23, 279–302. doi: 10.1007/s00382-004-0430-0
Sohail, T., Zika, J. D., Irving, D. B., and Church, J. A. (2022). Observed poleward freshwater transport since 1970. Nature, 602, 617–622. doi: 10.1038/s41586-021-04370-w
Tian, B., Fetzer, E. J., and Manning, E. M. (2019). The Atmospheric Infrared Sounder Obs4MIPs Version 2 data set. Earth Space Sci. 6, 324–333. doi: 10.1029/2018EA000508
Trenberth, K. E., Fasullo, J. T., and Kiehl, J. (2009). Earth's global energy budget. Bull. Am. Meteor. Soc. 90, 311–324. doi: 10.1175/2008BAMS2634.1
van der Ent, R. J., Savenije, H. H. G., Schaefli, B., and Steele-Dunne, S. C. (2010). Origin and fate of atmospheric moisture over continents. Water Resour. Res. 46, W09525. doi: 10.1029/2010WR009127
Wieder, W. R., Bonan, G. B., and Allison, S. D. (2013). Global soil carbon projections are improved by modelling microbial processes. Nat. Clim. Change 3, 909–912. doi: 10.1038/nclimate1951
Wilhere, G. F. (2021). A Paris-like agreement for biodiversity needs IPCC-like science. Glob. Ecol. Conserv. 28, e01617. doi: 10.1016/j.gecco.2021.e01617
Willett, K. M., Dunn, R. J. H., Thorne, P. W., Bell, S., de Podesta, M., Parker, D. E., et al. (2014). HadISDH land surface multi-variable humidity and temperature record for climate monitoring. Clim. Past 10, 1983–2006. doi: 10.5194/cp-10-1983-2014
Winckler, J., Reick, C. H., Bright, R. M., and Pongratz, J. (2019). Importance of surface roughness for the local biogeophysical effects of deforestation. J. Geophys. Res. Atmos. 124, 8605–8618. doi: 10.1029/2018JD030127
Zelinka, M. D., Myers, T. A., McCoy, D. T., Po-Chedley, S., Caldwell, P. M., Ceppi, P., et al. (2020). Causes of higher climate sensitivity in CMIP6 models. Geophys. Res. Lett. 47, e2019GL085782. doi: 10.1029/2019GL085782
Keywords: ecosystem stability, climate stability, primary forests, precipitation, evapotranspiration, convective parameterization
Citation: Makarieva AM, Nefiodov AV, Rammig A and Nobre AD (2023) Re-appraisal of the global climatic role of natural forests for improved climate projections and policies. Front. For. Glob. Change 6:1150191. doi: 10.3389/ffgc.2023.1150191
Received: 23 January 2023; Accepted: 03 July 2023;
Published: 20 July 2023.
Edited by:
William R. Moomaw, Tufts University, United StatesReviewed by:
Richard Birdsey, Woods Hole Research Center, United StatesSusan A. Masino, Trinity College, United States
Copyright © 2023 Makarieva, Nefiodov, Rammig and Nobre. This is an open-access article distributed under the terms of the Creative Commons Attribution License (CC BY). The use, distribution or reproduction in other forums is permitted, provided the original author(s) and the copyright owner(s) are credited and that the original publication in this journal is cited, in accordance with accepted academic practice. No use, distribution or reproduction is permitted which does not comply with these terms.
*Correspondence: Anastassia M. Makarieva, YW1tYWthcmlldmFAZ21haWwuY29t