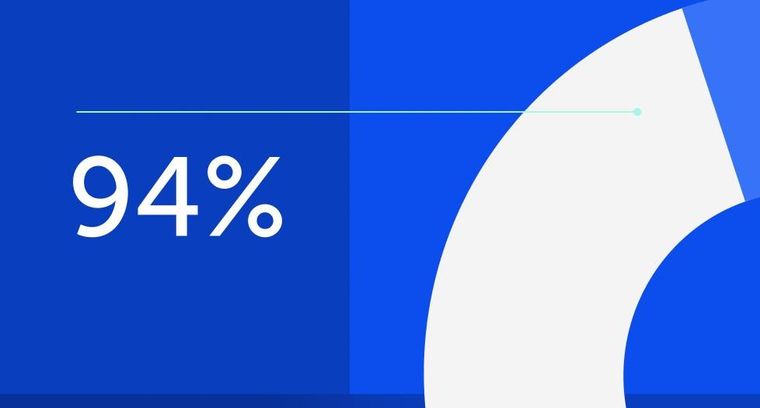
94% of researchers rate our articles as excellent or good
Learn more about the work of our research integrity team to safeguard the quality of each article we publish.
Find out more
ORIGINAL RESEARCH article
Front. For. Glob. Change, 21 July 2023
Sec. Forests and the Atmosphere
Volume 6 - 2023 | https://doi.org/10.3389/ffgc.2023.1145474
This article is part of the Research TopicDynamics of Asia's and Australasia's forests in a changing worldView all 22 articles
Soil respiration is a major pathway for CO2 emissions from ecosystems. Owing to its temperature dependency, the soil respiration rate is expected to increase due to global warming, particularly at high elevations. To clarify the effects of soil temperature and volumetric soil water content on soil respiration rates (RS), we examined seasonal changes in RS at five elevations of 1600–2800 m in subalpine coniferous forests in Japan for 5 years. The aboveground biomass of forest stands decreased from 282 to 29 Mg/ha as elevation increased. The monthly mean RS was lower at higher elevations from July to October. While RS was positively correlated with soil temperature at each elevation, the effect of soil water content on RS varied among the five elevations. Seasonal changes in RS could be reproduced from soil temperature and soil water content for each elevation in each year. RS at any temperature was lower at higher elevations because RS was also positively correlated with aboveground biomass. From 1600 to 2800 m, the annual RS was estimated to decrease from 2.79 to 0.74 kg CO2 year–1 m–2. The annual RS along the elevational gradient was predicted to increase by 9–12% and 30–42% under low and high greenhouse gas emission scenarios (annual mean temperature 0.76°C and 3.3°C increases), respectively, during 2095–2100 compared to the current period 2015–2020. Increased soil respiration rate will accelerate global warming via the positive feedback. Overall, our findings suggest that soil respiration evaluation is important not only for calculating the carbon balance of forest stands due to global warming but also for predicting global warming owing to the feedback of CO2 emission from soil to atmosphere.
Soil respiration is a major pathway for CO2 emissions from ecosystems (Bond-Lamberty and Thomson, 2010a). In forest ecosystems, soil contributes 60–80% of total ecosystem respiration (Law et al., 1999; Janssens et al., 2001). Soil respiration is the sum of the respiration of plant roots and microorganisms (Hanson et al., 2000). Notably, root respiration accounts for an average of 46% of total soil respiration in forests (Hanson et al., 2000). The annual soil respiration rate, on a global scale, correlates positively with the mean annual temperature, annual precipitation, soil organic matter content, and productivity (Raich, 1998; Chen et al., 2014; Zhao et al., 2017). Owing to the suppression of plant’s photosynthetic and organic matter decomposition rates in cold regions (Couteaux et al., 2002; Cornelissen et al., 2007; Takeda and Takahashi, 2020), global warming would increase the plants photosynthetic rate and consequently both root respiration rate and rate of plant detritus inputs to soil thereby increasing substrate availability or microbial decomposition/respiration. Notably, the soil respiration rate was shown to increase at a rate of 0.1 Pg C year–1 during 1989–2008 on a global scale (Bond-Lamberty and Thomson, 2010b). Cold regions, such as those at high latitudes and elevations, are expected to shift from carbon sinks to carbon sources (Moore et al., 1999; McGuire et al., 2002) because the temperature sensitivities of organic matter decomposition and soil respiration rates are high in cold regions (Hendrickson, 2003; Janssens and Pilegaard, 2003; Xu et al., 2015; Klimek et al., 2016; Zhao et al., 2017). Therefore, soil respiration evaluation is important in considering the carbon balance in the ecosystems of cold regions (Rustad et al., 2001). However, no studies have predicted future soil respiration rates at high elevations in mountainous areas—a topic examined in this study.
Soil respiration is usually reported to be lower at higher elevations because of lower air temperature (or soil temperature) (Niklińska and Klimek, 2007; Badraghi et al., 2021), and lower aboveground and belowground biomass of plant communities (Shibistova et al., 2002; Epron et al., 2006; Jiang et al., 2013; Zhao et al., 2017; Zhang et al., 2021). In general, forest biomass decreases with increasing elevation (Takahashi, 2021). Therefore, the decreased soil respiration rate at high elevations is due to low temperatures and declined plant biomass.
Further, soil respiration changes seasonally and is usually highest in the summer (Kang et al., 2003; Soe and Buchmann, 2005; Caprez et al., 2012). As the high photosynthetic rate of plants in summer affects seasonal changes in the soil respiration rate via root respiration (Högberg et al., 2001; Curiel Yuste et al., 2004), temperature can be used to explain many variations in seasonal changes in soil respiration (Soe and Buchmann, 2005; Zhang et al., 2010; Chen et al., 2013). However, the effect of soil water content often varies with elevation. For example, while the soil respiration rate correlated positively with soil water content at low elevations, it correlated negatively with the same variable at high elevations (Huang et al., 2017).
Because central Japan has many high mountains, estimating carbon uptake at the landscape level requires investigating soil respiration along elevational gradients. Further, examining the change in the soil respiration rate along elevational gradients is necessary to clarify the impact of global warming on the carbon balance of forest stands. However, soil respiration measurements along elevational gradients have not yet been made in Japan. Therefore, the purpose of this study is to answer the following questions:
(1) Does the soil respiration rate decrease with increasing elevation, and can the seasonal change in the soil respiration rate at each elevation be explained by soil temperature and soil water content?
(2) Can the mean annual temperature and stand biomass of trees explain the elevational variation in the annual soil respiration rate?
(3) How much will global warming increase soil respiration rates along the elevational gradient by the year 2100?
This study was conducted at five elevations (1600, 2000, 2300, 2500, and 2800 m) in a subalpine coniferous forest on the eastern slope of Mt. Norikura (36°06′N, 137°33′E, summit elevation 3026 m) in Chubu Sangaku National Park, Japan. Mt. Norikura is a volcano, but the last eruption was approximately 9000 years ago (Nakano and Uto, 1995). The soil substrate of the study site of 2800 m was scoria deposition with thin topsoil, while the study site of 1600 m was brown forest soil. An elevation of 1600 m is the lowest range limit of the subalpine zone. The treeline ecotone (sensu Körner, 2012) is located between 2500 and 2850 m (Takahashi and Yoshida, 2009; Takahashi et al., 2012). The mean annual temperatures of the study sites were estimated from the Nagawa Observatory (1068 m), which is the closest to the study sites, during the past 30 years (1981–2010) with the standard lapse rate (-0.6°C/100 m). The mean annual temperatures were 5.1°C at 1600 m, 2.7°C at 2000 m, 0.9°C at 2300 m, –0.3°C at 2500 m, and –2.1°C at 2800 m.
Four evergreen conifers (Abies veitchii Lindl., A. mariesii Mast., Tsuga diversifolia Mast., and Picea jezoensis var. hondoensis Rehder) were distributed between 1600 and 2500 m (Miyajima et al., 2007). However, the abundances of the four conifers were not uniform along the elevational gradient. A. veitchii dominates between 1600 m and 2200 m and A. mariesii dominates between 2000 m and 2500 m. The abundances of T. diversifolia and P. jezoensis var. hondoensis were lower than those of the two Abies species. Subordinate trees were all deciduous broad-leaved trees, such as Betula ermanii Cham. and Sorbus commixta Hedland. Dwarf pine Pinus pumila Regel dominated the treeline ecotone between 2500 and 2850 m (Miyajima et al., 2007), i.e., the study sites of 2500 and 2800 m were the lower and upper distribution limits of P. pumila, respectively. Dwarf bamboo Sasa senanensis Rehder is patchily distributed at 1600 m (Miyajima et al., 2007). The canopy height decreased with increasing elevation and was about 20 m at 1600 and 2000 m, about 10 m at 2300 m, 2 m at 2500 m, and 1 m at 2800 m (Miyajima and Takahashi, 2007; Takahashi and Yoshida, 2009).
Plots of 1.0, 0.6, 1.0, 0.05, and 0.004 ha were established at elevations of 1600, 2000, 2300, 2500, and 2800 m on the eastern slope of Mt. Norikura, respectively (Takeda and Takahashi, 2020). Soil respiration was measured at each plot in each elevation from July 2, 2015 to November 28, 2019. Measurements were performed once a week on sunny or cloudy days at each elevation after snow melting (May–July) to the end of October each year. In addition, measurements were taken once a month during the snow cover period (December–April) at 1600 m from 2017 onward (i.e., two winter seasons). Soil respiration was measured on the snow surface. Measurements were conducted at three points in each elevation. No soil collar was installed at each elevation, but measurements were taken at the same three points at each elevation. Soil respiration rates were measured for 5 min at each point using a closed static chamber (a volume of 8.83 liter, base area of 0.05 m2), equipped with a portable CO2 sensor (Vaisala, GMP343, Helsinki) and a data logger (Vaisala, MI70, Helsinki). The CO2 concentration was recorded at 15-s intervals, and the soil respiration rate (Rs, g CO2 h–1 m–2) was calculated using Equation 1 (Bekku et al., 1995).
where α is the time rate of change in CO2 concentration in the chamber (ppm/min), ρ is the density of CO2 (mg/m3), V is the volume of the chamber (m3), and S is the basal area of the chamber (m2). Furthermore, the air temperature, volumetric soil water content, and soil temperature at a depth of 5 cm were measured along with the soil respiration measurement, except for the snow period at 1600 m. Air temperature was measured using a bar mercury thermometer. Soil water content and soil temperature were measured using a soil moisture sensor (EC-5, Meter Group, Inc., Pullman) and a digital thermometer (D619, Tateyama Kagaku Co., Ltd., Toyama), respectively. The soil temperature and soil water content at a depth of 5 cm were manually measured three times for each point of the soil respiration measurement, and the mean values of the three measurements were used for statistical analysis.
In addition to the manual measurements of soil temperature and soil water content at the time of the measurements of soil respiration rates, the soil water content and soil temperature at a depth of 5 cm were automatically recorded at hourly intervals during the 5 years from 2015 to 2019 near the soil respiration measurement site at each elevation, using five soil moisture sensors (EC-5, Meter Group, Inc., Pullman) equipped with a data logger (Em50, Meter Group, Inc., Pullman) and a thermometer (TR-52i, T & D Corporation, Matsumoto), respectively. The values of the five soil water contents of each elevation were averaged for each hour. Hourly automatic measurement data of the soil temperature and soil water content at a depth of 5 cm were used for the snow period at 1600 m because the soil temperature and soil water content could not be measured manually during the snow period.
A generalized linear model (GLM) was used to analyze the effects of the soil water content, soil temperature at a depth of 5 cm, and the observation year on the soil respiration rate for each elevation as follows:
where ln is the natural logarithm, Rs is the soil respiration rate (g CO2 h–1 m–2), Ts is the soil temperature (°C), WS is the volumetric soil water content (%), Yi is the observation year i, a0 is the constant, and a1–a3 are regression coefficients. The squared value of WS was included as an independent variable because the soil respiration rate may be at its peak at a certain WS. The observation year was added as an explanatory variable because the soil respiration rate may be affected by unknown factors specific to the observation year (i.e., human error and interannual meteorological variation) other than the soil temperature and soil water content at the time of measurement. The observation year was used as a categorical variable. The effect of each observation year i between 2016 and 2019 on the soil respiration rate was expressed as the difference from that of 2015 (i.e., the constant value of Y2015 is zero).
Data of non-snow periods at the five elevations and those of the snow period at 1600 m after 2017 were also included in the dataset. The GLM analysis was performed for each elevation because the stand biomass of the trees differed along the elevational gradient. The number of measurements of soil respiration rates used for the statistical analysis were 376, 274, 239, 235, and 217 at 1600, 2000, 2300, 2500, and 2800 m, respectively. The selection of explanatory variables was performed by the Akaike Information Criteria (AIC) for Equation 2, and the model with the lowest AIC was selected as the best.
Sensitivity analysis was performed to examine the effects of soil temperature and soil water content on the soil respiration rate. The soil respiration rate was calculated using Equation 2 (the year was set as 2015) for the actual observed range of soil water content (about 10–60%) and for four soil temperature conditions (0-5.0, 5.1-10.0, 10.1-15.0, and 15.1-20.0°C).
Although soil temperature and soil water content at a depth of 5 cm were automatically measured at hourly intervals for 5 years at each elevation, the year-round measurements of soil temperature and soil water content at the five elevations could be performed for only 2 years (2018 and 2019) because of measurement challenges at certain elevations each year. Therefore, annual soil respiration rates at the five elevations were estimated using soil temperature and soil water content for the 2 years (2018 and 2019) only. Hourly soil respiration rates at each elevation were calculated by substituting hourly soil temperature and soil water content in 2018 and 2019 into Equation 2, and the annual soil respiration rate of each elevation in each year was obtained by summing the hourly soil respiration rates.
The temperature sensitivity (Q10) of the soil respiration rate was calculated for each elevation in each year using Equations 3 and 4:
where RS is the soil respiration rate (g CO2 h–1 m–2), TS is the soil temperature, and b0 and b1 are the constant and exponent, respectively.
This study used the Agro-Meteorological Grid Square Data (AMGSD), the National Agriculture and Food Research Organization (NARO) in Japan (Ohno et al., 2016; Kominami et al., 2019) to predict the change in the soil respiration rate at each elevation by climate change. Two climate change scenarios were used in this study: representative concentration pathways (RCP) 2.6 and 8.5 of the atmosphere–ocean general circulation model, the Model for Interdisciplinary Research on Climate (MIROC5). RCP 2.6 and RCP 8.5 are low- and high-future greenhouse gas emission scenarios, respectively. Under the RCP 2.6 scenario, the temperature will rise by 0.70°C during 2055–2060 and by 0.76°C during 2095–2100 compared to the current period 2015–2020. Under the RCP 8.5 scenario, the temperature will rise by 1.5°C during 2055–2060 and by 3.3°C during 2095–2100 compared to the current period 2015–2020. The third-order mesh data (1 × 1 km resolution) of AMGSD were obtained from NARO, and we used daily mean air temperatures of the two climate change scenarios.
Soil respiration rates at the five elevations were calculated for 2015–2020, 2055–2060, and 2095–2100. The soil respiration rate at each elevation was calculated using Equation 5, with only the soil temperature and stand biomass of trees as explanatory variables:
where RS is soil respiration rate (g CO2 h–1 m–2), Ts is soil temperature (°C), B is aboveground biomass (Mg/ha), c0 is a constant, c1 and c2 are regression coefficients. The aboveground biomass values at 1600, 2000, 2300, 2500, and 2800 m were 282.4, 267.6, 195.3, 50.1, and 29.0 Mg/ha, respectively (Takeda and Takahashi, 2020). Soil water content was excluded from Equation 5 due to the difficulty of estimating it in the future, although it affects the soil respiration rate. Using the same dataset of the five elevations with which Equation 2 was analyzed, co-c2 of Equation 5 were estimated by GLM (n = 1341). The selection of explanatory variables was performed by AIC for Equation 5, and the model with the lowest AIC was selected as the best.
The daily mean soil temperature was estimated from the daily mean air temperature because soil temperature is omitted in AMGSD. Regression equations were made to estimate the soil temperature from the air temperature at the soil respiration measurement during the 5 years (2015–2019) for each elevation (Supplementary Table 1). Daily mean soil temperatures for 2015–2020, 2055–2060, and 2095–2100 were estimated by substituting the daily mean air temperature of AMGSD into the regression equation for each elevation. The soil temperature was almost 0°C in winter due to the insulation effect of snow cover from the results of year-round measurements of soil temperature. Therefore, this study assumed that soil temperature does not drop below 0°C even in winter. Furthermore, assuming that the aboveground biomass of each elevation is the same between the current period (2015–2020) and the two future periods (2055–2060, 2095–2100), this study calculated the soil respiration rates of the current and the two future periods from the aboveground biomass and the estimated daily mean soil temperature for each elevation. Because the soil respiration rate of Equation 5 is per hour, the daily soil respiration rate was calculated by multiplying this by 24. The annual soil respiration rate was estimated by summing the daily soil respiration rates for each year.
Soil respiration rates at the five elevations in 2019 are shown as examples of elevational and seasonal changes in soil respiration rates (Figure 1). The soil water content tended to be lower throughout the year at 2800 m than at the other elevations because of the scoria substrate at 2800 m. The soil respiration rate was highest during early August (day of the year 210–220) at each elevation. This period also corresponded to the highest soil temperatures (Figure 1). The monthly mean soil respiration rate was lower at higher elevations for each month from July to October (Pearson correlation test, P < 0.001 for each month, Figure 2). The soil respiration rate in 2016 (especially July) tended to be lower than in the other years at each elevation, except for 2000 m (Figure 2 and Table 1).
Figure 1. Seasonal changes in soil respiration rates (top row), soil temperature (middle row), volumetric soil water content (bottom row, lines) at a depth of 5 cm, and daily precipitation (bottom row, bars) at five elevations in 2019 on Mt. Norikura, central Japan. The elevation is 1600, 2000, 2300, 2500, and 2800 m from the left to the right for each row of five panels. The day of the year (X axis) is depicted between 100 and 350 for each panel.
Figure 2. Elevational changes in monthly mean soil respiration rates from July to October for 5 years (2015–2019). A Pearson correlation coefficient (R) is shown in each panel. Each correlation coefficient is significant (P < 0.001).
Seasonal changes in soil respiration rates at the five elevations could be reproduced using Equation 2 for each year (Table 1 and Supplementary Figure 1). While the soil respiration rate correlated positively with the soil temperature for each elevation (Figure 3 and Table 1), it also correlated positively with the soil water content at 1600 m but was uncorrelated at 2000 m (Table 1). The soil respiration rate correlated negatively with the square of soil water content at 2300, 2500, and 2800 m (Table 1). The sensitivity analysis using Equation 2 revealed that the higher the soil temperature, the higher the soil respiration rate at any elevation (Figure 4). Under higher soil temperature conditions, the soil respiration rate at 1600 m tended to increase more with increasing soil water content, although the variation of the soil respiration was large at any soil water content (Figure 4). The effect of soil water content on the soil respiration rate was not recognized at 2000 m (Figure 4). The soil respiration rate at 2300 m tended to decrease with increasing soil water content at any soil temperature (Figure 4). The soil respiration rates increased with increasing soil water content until peaking at 30 and 25% at 2500 and 2800 m, respectively, and then soil respiration rates decreased with increasing soil water content (Figure 4).
Figure 3. Relationships of soil respiration rates with soil temperature (left column) and soil water content (right column) at a depth of 5 cm at five elevations for 5 years (2015–2019). The elevations are 1600, 2000, 2300, 2500, and 2800 m from the top to the bottom for each column.
Figure 4. Changes in soil respiration rates with soil temperature and soil water content at a depth of 5 cm at five elevations (1600, 2000, 2300, 2500, and 2800 m). The soil respiration rate was calculated using Equation 2 in the main text for the three or four soil temperature conditions (0-5.0, 5.1-10.0, 10.1-15.0, and 15.1-20.0°C) at each elevation. See Table 1 for equation parameters (the measurement year was set as 2015). An average soil temperature was used to draw a regression line (Equation 2) for each soil temperature condition at each elevation (i.e., 3.6, 7.9, 12.5, 16.4°C at 1600 m, 8.0, 12.8, 16.2°C at 2000 m, 4.3, 7.7, 12.6°C at 2300 m, 3.5, 7.2, 11.7°C at 2500 m, and 3.5, 7.7, 11.6°C at 2800 m).
Annual soil respiration rates at the five elevations were calculated by substituting hourly measurement data for soil temperature and soil water content in 2018 and 2019 into Equation 2. Seasonal changes in the soil respiration rate at each elevation did not differ significantly between the 2 years (Supplementary Figure 2). Annual soil respiration rates were lower at higher elevations, decreasing from 2.79 kg CO2 year–1 m–2 at 1600 m to 0.74 kg CO2 year–1 m–2 at 2800 m. The annual soil respiration rate correlated positively with the mean annual temperature (Pearson correlation test, R = 0.92, P < 0.001, n = 10) and the aboveground biomass (R = 0.93, P < 0.001, n = 10) (Figure 5).
Figure 5. Relationships of annual soil respiration rates with mean annual temperature (left) and aboveground biomass (right) at the five elevations (1600, 2000, 2300, 2500, and 2800 m) for the 2 years, 2018 (●) and 2019 (○). The annual soil respiration rate was estimated by substituting hourly soil temperature and soil water content into Equation 2 in the main text. See Table 1 for the equation parameters. The unit of Mg/ha is equivalent to × 0.1 kg/m2.
The temperature sensitivity (Q10) of the soil respiration rate showed no elevational trend, with the mean value ranging from 2.7 to 5.1 across the five elevations (Supplementary Figure 3). The effects of soil temperature (TS, °C) and aboveground biomass (B, Mg/ha) on the elevational change in the soil respiration rate (RS, g CO2 h–1 m–2) was analyzed using Equation 5. The constant (c0) and coefficients (c1, c2) of Equation 5 were estimated by GLM as below (P < 0.001 for each of c0-c2, n = 1341).
The soil respiration rate was higher at lower elevations with greater biomass even at the same soil temperature because of the two positive coefficients of TS and B (Figure 6).
Figure 6. Relationship between soil respiration rate and soil temperature at a depth of 5 cm at five elevations (1600, 2000, 2300, 2500, and 2800 m). The regression lines of elevations of 1600 and 2000 m markedly overlapped, such that they are indistinguishable. The soil respiration rate at each elevation was calculated by substituting aboveground biomass into Equation 6. The aboveground biomass was 282.4, 267.6, 195.3, 50.1, and 29.0 Mg/ha at 1600, 2000, 2300, 2500, and 2800 m, respectively.
Substituting the soil temperature estimated from the daily mean temperature of the climate change scenarios (RCP 2.6, RCP 8.5) into the soil temperature in Equation 6, the percent change in the annual soil respiration rate was estimated for the two future periods (2055–2060, 2095–2100) compared to the current period (2015–2020). The annual soil respiration rate along the elevational gradient was expected to increase by 9–12% and by 30–42% under the RCP 2.6 and RCP 8.5, respectively, during the future period (2095–2100) compared to the current period (2015–2020) (Figure 7). Under the two RCP scenarios, the percentage increase in the soil respiration rate was highest at 2000 m and slightly decreased with increasing elevation (Figure 7).
Figure 7. Percent increases in annual soil respiration rates for the two future periods, 2055–2060 and 2095–2100, relative to the current period (2015–2020) at the five elevations (1600, 2000, 2300, 2500, and 2800 m). Annual soil respiration rates were estimated using Equation 6 and two climate change scenarios (RCP 2.6 and RCP 8.5).
This study showed that the soil respiration rate was lower at higher elevations with lower soil temperatures and stand biomass. Many studies on seasonal changes in the soil respiration rate have found positive correlations among the soil respiration rate, soil temperature, and biomass (Rustad et al., 2001; Wieser, 2004; Epron et al., 2006; Zhao et al., 2017; Zhang et al., 2021). Therefore, the soil respiration rate is often reported to be lower in higher elevations (Niklińska and Klimek, 2007; Badraghi et al., 2021). In addition, the photosynthetic rate of plants is temperature-dependent (DeLucia and Smith, 1987; Mellander et al., 2004; Zaka et al., 2016), and the root respiration rate is thought to increase when the photosynthetic rate is high due to high air and soil temperatures (Sampson et al., 2007; Metcalfe et al., 2011). The photosynthetic rates of Abies veitchii, A. mariesii, and Pinus pumila, which are dominant in the sites of the present study, are decreased by low air and soil temperatures (Shimada and Takahashi, 2022, Suzuki and Takahashi, 2022). Therefore, lower soil respiration rates at higher elevations are undoubtedly caused by lower biomass and cooler temperatures.
Q10 has been shown to correlate negatively with temperature (Janssens and Pilegaard, 2003; Song et al., 2014; Xu et al., 2015) and is often reported to be greater at higher elevations and latitudes (Zhao et al., 2017; Badraghi et al., 2021). In addition, the Q10 value is usually approximately 2 at lowlands (Hashimoto, 2005; Meyer et al., 2018). Although no elevational trend in Q10 was found among the five elevations (1600–2800 m) studied, the average value of Q10 at each elevation ranged from 2.7 to 5.1. Therefore, Q10 was clearly higher at the five elevations in the subalpine zone than in the lowlands.
The effect of soil water content on the soil respiration rate differed among the five elevations. The soil respiration rate slightly increased with increasing soil water content at 1600 m when soil temperature was high but decreased at higher elevations when the soil water content exceeded the optimal soil water content, corroborating Huang et al.’s (2017) findings. This elevational change in the effects of soil water content on soil respiration rate is thought to correspond to the elevational change in meteorological conditions. In general, air temperature decreases while precipitation increases with increasing elevation in central Japan (Yamamura and Nakano, 1985; Yamada et al., 1995). Dendrochronological studies have often reported that climatic factors limiting tree growth change from less precipitation in summer at low elevations to low summer temperatures at high elevations (Adams and Kolb, 2005; Wang et al., 2005; Massaccesi et al., 2008; Peng et al., 2008). This is also true for the elevational gradient in the present study (Takahashi, 2003; Takahashi et al., 2003, 2005, 2011). Therefore, the positive correlation between the soil respiration rate and the soil water content in high soil temperature conditions at 1600 m indicates that the decrease in tree growth due to less precipitation (i.e., drought stress) reduces the root respiration rate and consequently the soil respiration rate.
During the 5-year measurement, the soil respiration rate in 2016 (especially July) was lower than in the other measurement years even at the same elevation, except for 2000 m. Although the mean monthly temperature in July 2016 was almost the same as in the other measurement years, monthly precipitation was 48–68% lower than in the other measurement years (Supplementary Figure 4), resulting in longer drought stress compared to the other measurement years. The soil respiration rate decreases under drought stress (Davidson et al., 1998; Epron et al., 2004; Lellei-Kovács et al., 2011). Therefore, the soil respiration rate was considered to be low in July 2016.
In this study, the annual soil respiration rate decreased from 2.79 to 0.74 kg CO2 year–1 m–2 as elevation increased because the soil temperature and above ground biomass were lower at higher elevations. These annual respiration rates were within the range of reported values for the boreal ecosystem (Bond-Lamberty and Thomson, 2010a). The soil respiration rate at the five elevations (1600–2800 m) was expected to increase 9–12% (RCP 2.6) and 30–42% (RCP 8.5) by the year 2100 compared to the current period (2015–2020). The different results between the RCP 2.6 and RCP 8.5 scenarios were absolutely due to the difference in the temperature increase because concomitant changes in the soil water content and biomass were not taken into account. Compared to the current period (2015–2020), the global temperature will increase by 0.76°C and 3.3°C in 2095–2100 under the RCP 2.6 and RCP 8.5 scenarios, respectively. Therefore, global warming will increase the soil respiration rate, but the rate of the increase depends on how much temperature increases.
Unfortunately, there were no studies that estimated future soil respiration rates, so we cannot compare our estimates with previous studies. However, this study has some limitations in terms of predicting soil respiration rates by 2100. First, the soil respiration rate was predicted using soil temperature and biomass without considering soil water content. The increase in temperature possibly reduces the soil water content by increasing the evapotranspiration rate. Although a rise in soil temperature increases the soil respiration rate, this effect can be offset by a decrease in soil water content (Schindlbacher et al., 2012). Second, the current relationship between soil temperature and soil respiration rate was assumed to remain the same in the future, but this may change. As soil temperature increases, the organic matter decomposition rate increases (Trofymow et al., 2002; Cornelissen et al., 2007; Takeda and Takahashi, 2020), resulting in an increased supply rate of soil nutrients, which may increase plant growth rate, root respiration rate, and stand biomass. Third, it is possible that stand biomass will increase due to global warming, although this study estimated the future soil respiration rate under the assumption that stand biomass does not change from the current biomass. Increase of stand biomass will increase the soil respiration rate. Forth, as vegetation shifts to faster-growing tree species due to global warming, the nutrient concentration of litter supplied to the forest floor increases, and the heterotrophic respiration rate may increase (Metcalfe et al., 2011). Given the preceding four points, investigating the future effects of global warming on soil respiration rates is necessary using a dynamic vegetation model (cf. Sato et al., 2007).
This study showed that (1) the soil respiration rate was lower at higher elevations for each month from July to October, and the seasonal change in the soil respiration rate at each elevation could be reproduced by the soil temperature and soil water content, (2) the annual soil respiration rate correlated positively with the mean annual temperature and the aboveground biomass, and (3) the annual soil respiration rate will increase 9–12% and 30–42% under the RCP 2.6 and RCP 8.5 scenarios, respectively, during the future period (2095–2100) compared to the current period (2015–2020). As a result, global warming is expected to increase soil respiration along the elevational gradient in the future.
In general, in high elevations with cool thermal conditions, tree growth is limited more by low summer temperatures (Buckley et al., 1997; Hopton and Pederson, 2005; Wang et al., 2005; Savva et al., 2006; Levanič et al., 2009; Takahashi and Okuhara, 2013). The higher the elevation, the lower the net primary production (NPP) along the elevational gradient of the present study (Takeda and Takahashi, 2020). Global warming increases NPP because of the positive correlation between mean annual temperature and NPP from a meta-analysis (Piao et al., 2006). However, both NPP and soil respiration rates influence the carbon balance at the ecosystem level (Randerson et al., 2002; Bond-Lamberty et al., 2004; Reichstein et al., 2005). Therefore, our findings suggest that soil respiration evaluation is important not only for calculating the carbon balance of forest stands due to global warming but also for predicting global warming owing to the feedback of CO2 emission from soil to the atmosphere in mountain ecosystems.
The raw data supporting the conclusions of this article will be made available by the authors, without undue reservation.
KT conceived the study and led the writing of the manuscript. KT, RM, and ST collected the data and analyzed the data. NM and KT revised the manuscript. All authors contributed to the article and approved the submitted version.
This study was partially supported by the Japan Society for the Promotion of Science (26292081 and 21K06331) and the joint research program of the Institute for Cosmic Ray Research, University of Tokyo.
The authors declare that the research was conducted in the absence of any commercial or financial relationships that could be construed as a potential conflict of interest.
All claims expressed in this article are solely those of the authors and do not necessarily represent those of their affiliated organizations, or those of the publisher, the editors and the reviewers. Any product that may be evaluated in this article, or claim that may be made by its manufacturer, is not guaranteed or endorsed by the publisher.
The Supplementary Material for this article can be found online at: https://www.frontiersin.org/articles/10.3389/ffgc.2023.1145474/full#supplementary-material
Adams, H. D., and Kolb, T. E. (2005). Tree growth response to drought and temperature in a mountain landscape in northern Arizona, USA. J. Biogeogr. 32, 1629–1640. doi: 10.1111/j.1365-2699.2005.01292.x
Badraghi, A., Ventura, M., Polo, A., Borruso, L., Giammarchi, F., and Montagnani, L. (2021). Soil respiration variation along an altitudinal gradient in the Italian Alps: Disentangling forest structure and temperature effects. PLoS One 16, e0247893. doi: 10.1371/journal.pone.0247893
Bekku, Y., Koizumi, H., Nakadai, T., and Iwaki, H. (1995). Measurement of soil respiration using closed chamber method: An IRGA technique. Ecol. Res. 10, 369–373. doi: 10.1007/BF02347863
Bond-Lamberty, B., and Thomson, A. (2010a). A global database of soil respiration data. Biogeoscience 7, 1915–1926. doi: 10.5194/bg-7-1915-2010
Bond-Lamberty, B., and Thomson, A. (2010b). Temperature-associated increases in the global soil respiration record. Nature 464, 579–583. doi: 10.1038/nature08930
Bond-Lamberty, B., Wang, C., and Gower, S. T. (2004). Net primary production and net ecosystem production of a boreal black spruce wildfire chronosequence. Glob. Change Biol. 10, 473–487. doi: 10.1111/j.1529-8817.2003.0742.x
Buckley, B. M., Cook, E. R., Peterson, M. J., and Barbetti, M. (1997). A changing temperature response with elevation for Lagarostrobos franklinii in Tasmania, Australia. Clim. Change 36, 477–498. doi: 10.1023/A:1005322332230
Caprez, R., Niklaus, P. A., and Körner, C. (2012). Forest soil respiration reflects plant productivity across a temperature gradient in the Alps. Oecologia 170, 1143–1154. doi: 10.1007/s00442-012-2371-3
Chen, S., Zou, J., Hu, Z., Chen, H., and Lu, Y. (2014). Global annual soil respiration in relation to climate, soil properties and vegetation characteristics: Summary of available data. Agr. For. Meteorol. 19, 335–346. doi: 10.1016/j.agrformet.2014.08.020
Chen, W., Jia, X., Zha, T., Wu, B., Zhang, Y., Li, C., et al. (2013). Soil respiration in a mixed urban forest in China in relation to soil temperature and water content. Eur. J. Soil Biol. 54, 63–68. doi: 10.1016/j.ejsobi.2012.10.001
Cornelissen, J. H. C., van Bodegom, P. M., Aerts, R., Callaghan, T. V., van Logtestijn, R. S. P., Alatalo, J., et al. (2007). Global negative vegetation feedback to climate warming responses of leaf litter decomposition rates in cold biomes. Ecol. Lett. 10, 619–627. doi: 10.1111/j.1461-0248.2007.01051.x
Couteaux, M. M., Sarmiento, L., Bottner, P., Acevedo, D., and Thiery, J. M. (2002). Decomposition of standard plant material along an altitudinal transect (65-3968 m) in the tropical Andes. Soil Biol. Biochem. 34, 69–78. doi: 10.1016/S0038-0717(01)00155-9
Curiel Yuste, J., Janssens, I. A., Carrara, A., and Ceulemans, R. (2004). Annual Q10 of soil respiration reflects plant phenological patterns as well as temperature sensitivity. Glob. Change Biol. 10, 161–169. doi: 10.1111/j.1529-8817.2003.00727.x
Davidson, E. A., Belk, E., and Boone, R. D. (1998). Soil water content and temperature as independent or confounded factors controlling soil respiration in a temperature mixed hardwood forest. Glob. Change Biol. 4, 217–227. doi: 10.1046/j.1365-2486.1998.00128.x
DeLucia, E. H., and Smith, W. K. (1987). Air and soil temperature limitations on photosynthesis in Engelmann spruce during summer. Can. J. For. Res. 17, 527–533. doi: 10.1139/x87-088
Epron, D., Bosc, A., Bonal, D., and Freycon, V. (2006). Spatial variation of soil respiration across a topographic gradient in a tropical rain forest in French Guiana. J. Trop. Ecol. 22, 565–574. doi: 10.1017/S0266467406003415
Epron, D., Nouvellon, Y., Roupsard, O., Mouvondy, W., Mabiala, A., Saint-André, L., et al. (2004). Spatial and temporal variations of soil respiration in a Eucalyptus plantation in Congo. For. Ecol. Manage. 202, 149–160. doi: 10.1016/j.foreco.2004.07.019
Hanson, P. J., Edwards, N. T., Garten, C. T., and Andrews, J. A. (2000). Separating root and soil microbial contributions to soil respiration: A review of methods and observations. Biogeochemistry 48, 115–146. doi: 10.1023/A:1006244819642
Hashimoto, S. (2005). Q10 values of soil respiration in Japanese forests. J. For. Res. 10, 409–413. doi: 10.1007/s10310-005-0164-9
Hendrickson, O. (2003). Influences of global change on carbon sequestration by agricultural and forest soils. Environ. Rev. 11, 161–192. doi: 10.1139/a04-001
Hopton, H. M., and Pederson, N. (2005). “Climate sensitivity of Atlantic white cedar at its northern range limit,” in Proceedings of the Arlington Echo Symposium, USDA Forest Service. Atlantic white cedar: ecology, restoration and management, eds M. K. Burke and P. Sheridan (Sheville, NC: USDA Forest Service Southern Research Station), 22–30.
Huang, Y. H., Hung, C. Y., Lin, I. R., Kume, T., Menyailo, O. V., and Cheng, C. H. (2017). Soil respiration patterns and rates at three Taiwanese forest plantations: Dependence on elevation, temperature, precipitation, and litterfall. Bot. Stud. 58:49. doi: 10.1186/s40529-017-0205-7
Högberg, P., Nordgren, A., Buchmann, N., Taylor, A. F. S., Ekblad, A., Höggerg, M. N., et al. (2001). Large-scale forest girdling shows that current photosynthesis drives soil respiration. Nature 411, 789–792. doi: 10.1038/35081058
Janssens, I. A., and Pilegaard, K. (2003). Large seasonal changes in Q10 of soil respiration in a beech forest. Glob. Change Biol. 9, 911–918. doi: 10.1046/j.1365-2486.2003.00636.x
Janssens, I. A., Lankreijer, H., Matteucci, G., Kowalski, A. S., Buchmann, N., Epron, D., et al. (2001). Productivity overshadows temperature in determining soil and ecosystem respiration across European forests. Glob. Change Biol. 7, 269–278. doi: 10.1046/j.1365-2486.2001.00412.x
Jiang, J., Zong, N., Song, M., Shi, P., Ma, W., Fu, G., et al. (2013). Responses of ecosystem respiration and its components to fertilization in an alpine meadow on the Tibetan Plateau. Eur. J. Soil Biol. 56, 101–106. doi: 10.1016/j.ejsobi.2013.03.001
Kang, S., Doh, S., Lee, D., Lee, D., Jin, V. L., and Kimball, J. S. (2003). Topographic and climatic controls on soil respiration in six temperate mixed-hardwood forest slopes, Korea. Glob. Change Biol. 9, 1427–1437. doi: 10.1046/j.1365-2486.2003.00668.x
Klimek, B., Jelonkiewicz, Ł, and Niklińska, M. (2016). Drivers of temperature sensitivity of decomposition of soil organic matter along a mountain altitudinal gradient in the Western Carpathians. Ecol. Res. 31, 609–615. doi: 10.1007/s11284-016-1369-4
Kominami, Y., Sasaki, K., and Ohno, H. (2019). User’s manual for the agro-meteorological grid square data, Ver.4. Tsukuba: NARO, 67pp (in Japanese).
Körner, C. (2012). Alpine treelines – functional ecology of the global high elevation tree limits. Berlin: Springer. doi: 10.1007/978-3-0348-0396-0
Law, B. E., Ryan, M. G., and Anthoni, P. M. (1999). Seasonal and annual respiration of a ponderosa pine ecosystem. Glob. Change Biol. 5, 169–182. doi: 10.1046/j.1365-2486.1999.00214.x
Lellei-Kovács, E., Kovács-Láng, E., Botta-Dukát, Z., Kalapos, T., Emmett, B., and Beier, C. (2011). Thresholds and interactive effects of soil moisture on the temperature response of soil respiration. Eur. J. Soil Biol. 47, 247–255. doi: 10.1016/j.ejsobi.2011.05.004
Levanič, T., Gričar, J., Gagen, M., Jalkanen, R., Loader, N. J., McCarroll, D., et al. (2009). The climate sensitivity of Norway spruce [Picea abies (L.) Karst.] in the southeastern European Alps. Trees 23, 169–180. doi: 10.1007/s00468-008-0265-0
Massaccesi, G., Roig, F. A., Pastur, G. J. M., and Barrera, M. D. (2008). Growth patterns of Nothofagus pumilio trees along altitudinal gradients in Tierra del Fuego, Argentina. Trees 22, 245–255. doi: 10.1007/s00468-007-0181-8
McGuire, A. D., Wirth, C., Apps, M., Beringer, J., Clein, J., Epstein, H., et al. (2002). Environmental variation, vegetation distribution, carbon dynamics and water/energy exchange at high latitudes. J. Veg. Sci. 13, 301–314. doi: 10.1111/j.1654-1103.2002.tb02055.x
Mellander, P. E., Bishop, K., and Lundmark, T. (2004). The influence of soil temperature on transpiration: A plot scale manipulation in a young Scots pine stand. For. Ecol. Manage. 195, 15–28. doi: 10.1016/j.foreco.2004.02.051
Metcalfe, D. B., Fisher, R. A., and Wardle, D. A. (2011). Plant communities as drivers of soil respiration: Pathways, mechanisms, and significance for global change. Biogeosciences 8, 2047–2061. doi: 10.5194/bg-8-2047-2011
Meyer, N., Welp, G., and Amelung, W. (2018). The temperature sensitivity (Q10) of soil respiration: Controlling factors and spatial prediction at regional scale based on environmental soil classes. Glob. Biogeochem. Cycles 32, 306–323. doi: 10.1002/2017GB005644
Miyajima, Y., and Takahashi, K. (2007). Changes with altitude of the stand structure of temperate forests on Mount Norikura, central Japan. J. For. Res. 12, 187–192. doi: 10.1007/s10310-007-0002-3
Miyajima, Y., Sato, T., and Takahashi, K. (2007). Altitudinal changes in vegetation of tree, herb and fern species on Mount Norikura, central Japan. Veg. Sci. 24, 29–40.
Moore, D. J., Nowak, R. S., and Tausch, R. J. (1999). Gas exchange and carbon isotope discrimination of Juniperus osteosperma and Juniperus occidentalis across environmental gradients in the Great Basin of western North America. Tree Physiol. 19, 421–433. doi: 10.1093/treephys/19.7.421
Nakano, S., and Uto, K. (1995). Volcanic history of Norikura Volcano based on K-Ar dating. Program. Abst. Volcanol. Soc. Japan 2, 91.
Niklińska, M., and Klimek, B. (2007). Effect of temperature on the respiration rate of forest soil organic layer along an elevation gradient in the Polish Carpathians. Biol. Fertil. Soils 43, 511–518. doi: 10.1007/s00374-006-0129-y
Ohno, H., Sasaki, K., Ohara, G., and Nakazono, K. O. U. (2016). Development of grid square air temperature and precipitation data compiled from observed, forecasted, and climatic normal data. Clim. Biosphere 16, 71–79. doi: 10.2480/cib.J-16-028
Peng, J., Gou, X., Chen, F., Li, J., Liu, P., and Zhang, Y. (2008). Altitudinal variability of climate-tree growth relationships along a consistent slope of Anyemaqen Mountains, northeastern Tibetan Plateau. Dendrochronologia 26, 87–96. doi: 10.1016/j.dendro.2007.10.003
Piao, S., Fang, J., and He, J. (2006). Variations in vegetation net primary production in the Qinghai-Xizang Plateau, China, from 1982 to 1999. Clim. Change 74, 253–267. doi: 10.1007/s10584-005-6339-8
Raich, J. W. (1998). Aboveground productivity and soil respiration in three Hawaiian rain forests. For. Ecol. Manage. 107, 309–318. doi: 10.1016/S0378-1127(97)00347-2
Randerson, J. T., Chapin, F. S. III, Harden, J. W., Neff, J. C., and Harmon, M. E. (2002). Net ecosystem production: A comprehensive measure of net carbon accumulation by ecosystems. Ecol. Appl. 12, 937–947. doi: 10.1890/1051-0761(2002)012[0937:NEPACM]2.0.CO;2
Reichstein, M., Falge, E., Baldocchi, D., Papale, D., Aubinet, M., Berbigier, P., et al. (2005). On the separation of net ecosystem exchange into assimilation and ecosystem respiration: Review and improved algorithm. Glob. Change Biol. 11, 1424–1439. doi: 10.1111/j.1365-2486.2005.001002.x
Rustad, L. E., Campbell, J. L., Marion, G. M., Norby, R. J., Mitchell, M. J., Hartley, A. E., et al. (2001). A meta-analysis of the response of soil respiration, net nitrogen mineralization, and aboveground plant growth to experimental ecosystem warming. Oecologia 126, 543–562. doi: 10.1007/s004420000544
Sampson, D. A., Janssens, I. A., Curiel Yuste, J., and Ceulemans, R. (2007). Basal rates of soil respiration are correlated with photosynthesis in a mixed temperate forest. Glob. Change Biol. 13, 2008–2017. doi: 10.1111/j.1365-2486.2007.01414.x
Sato, H., Itoh, A., and Kohyama, T. (2007). SEIB-DGVM: A new dynamic global vegetation model using a spatially explicit individual-based approach. Ecol. Model. 200, 279–307. doi: 10.1016/j.ecolmodel.2006.09.006
Savva, Y., Oleksyn, J., Reich, P. B., Tjoelker, M. G., Vaganov, E. A., and Modrzynski, J. (2006). Interannual growth response of Norway spruce to climate along an altitudinal gradient in the Tatra Mountains, Poland. Trees 20, 735–746. doi: 10.1007/s00468-006-0088-9
Schindlbacher, A., Wunderlich, S., Borken, W., Kitzler, B., Zechmeister-Boltenstern, S., and Jandl, R. (2012). Soil respiration under climate change: Prolonged summer drought offsets soil warming effects. Glob. Change Biol. 18, 2270–2279. doi: 10.1111/j.1365-2486.2012.02696.x
Shibistova, O., Lloyd, J., Evgrafova, S., Savushkina, N., Zrazhevskaya, G., Arneth, A., et al. (2002). Seasonal and spatial variability in soil CO2 efflux rates for a central Siberian Pinus sylvestris forest. Tellus 54B, 552–567. doi: 10.3402/tellusb.v54i5.16687
Shimada, R., and Takahashi, K. (2022). Diurnal and seasonal variations in photosynthetic rates of dwarf pine Pinus pumila at the treeline in central Japan. Arc. Antarc. Alp. Res. 54, 1–12. doi: 10.1080/15230430.2021.2022995
Soe, A. R. B., and Buchmann, N. (2005). Spatial and temporal variations in soil respiration in relation to stand structure and soil parameters in an unmanaged beech forest. Tree Physiol. 25, 1427–1436. doi: 10.1093/treephys/25.11.1427
Song, X., Peng, C., Zhao, Z., Zhang, Z., Guo, B., Wang, W., et al. (2014). Quantification of soil respiration in forest ecosystems across China. Atmos. Environ. 94, 546–551. doi: 10.1016/j.atmosenv.2014.05.071
Suzuki, R., and Takahashi, K. (2022). Responses of photosynthetic rates to temperature in two conifers dominating at different elevations. Landsc. Ecol. Eng. 18, 389–395. doi: 10.1007/s11355-022-00500-2
Takahashi, K. (2003). Effects of climatic conditions on shoot elongation of alpine dwarf pine (Pinus pumila) at its upper and lower altitudinal limits in central Japan. Arc. Antarc. Alp. Res. 35, 1–7. doi: 10.1657/1523-0430(2003)035[0001:EOCCOS]2.0.CO;2
Takahashi, K. (2021). Productivity does not decrease at the climate extremes of tree ranges in the Japanese archipelago. Oecologia 197, 259–269. doi: 10.1007/s00442-021-05015-5
Takahashi, K., and Okuhara, I. (2013). Forecasting the effects of global warming on radial growth of subalpine trees at the upper and lower distribution limits in central Japan. Clim. Change 117, 273–287. doi: 10.1007/s10584-012-0547-9
Takahashi, K., and Yoshida, S. (2009). How the scrub height of Pinus pumila decreases at the treeline. Ecol. Res. 24, 847–854. doi: 10.1007/s11284-008-0558-1
Takahashi, K., Azuma, H., and Yasue, K. (2003). Effects of climate on the radial growth of tree species in the upper and lower distribution limits of an altitudinal ecotone on Mt. Norikura, central Japan. Ecol. Res. 18, 549–558. doi: 10.1046/j.1440-1703.2003.00577.x
Takahashi, K., Hirosawa, T., and Morishima, R. (2012). How the timberline formed: Altitudinal changes in stand structure and dynamics around the timberline in central Japan. Ann. Bot. 109, 1165–1174. doi: 10.1093/aob/mcs043
Takahashi, K., Okuhara, I., Tokumitsu, Y., and Yasue, K. (2011). Responses to climate by tree-ring widths and maximum latewood densities of two Abies species at upper and lower altitudinal distribution limits in central Japan. Trees 25, 745–753. doi: 10.1007/s00468-011-0552-z
Takahashi, K., Tokumitsu, Y., and Yasue, K. (2005). Climatic factors affecting the tree-ring width of Betula ermanii at the timberline on Mount Norikura, central Japan. Ecol. Res. 20, 445–451. doi: 10.1007/s11284-005-0060-y
Takeda, S., and Takahashi, K. (2020). Elevational variation in abundance of coarse woody debris in subalpine forests, central Japan. For. Ecol. Manage. 473, 118295. doi: 10.1016/j.foreco.2020.118295
Trofymow, J. A., Moore, T. R., Titus, B., Prescott, C., Morrison, I., Siltanen, M., et al. (2002). Rates of litter decomposition over 6 years in Canadian forests: Influence of litter quality and climate. Can. J. For. Res. 32, 789–804. doi: 10.1139/x01-117
Wang, T., Ren, H., and Ma, K. (2005). Climatic signals in tree ring of Picea schrenkiana along an altitudinal gradient in the central Tianshan Mountains, northwestern China. Trees 19, 735–741. doi: 10.1007/s00468-005-0003-9
Wieser, G. (2004). Seasonal variation of soil respiration in a Pinus cembra forest at the upper timberline in the Central Austrian Alps. Tree Physiol. 24, 475–480. doi: 10.1093/treephys/24.4.475
Xu, Z., Tang, S., Xiong, L., Yang, W., Yin, H., Tu, L., et al. (2015). Temperature sensitivity of soil respiration in China’s forest ecosystems: Patterns and controls. Appl. Soil Ecol. 93, 105–110. doi: 10.1016/j.apsoil.2015.04.008
Yamada, T., Hibino, T., Araki, T., and Nakatsugawa, M. (1995). Statistical characteristics of rainfall in mountainous basins. Proc. Japan Soc. Civil. Eng. II 33, 1–13. doi: 10.2208/jscej.1995.527_1
Yamamura, R., and Nakano, H. (1985). Relationships between annual water losses and annual precipitation and altitude in forested watershed. Bull. Fac. Agr. Shinshu Univ. 22, 139–147.
Zaka, S., Frak, E., Julier, B., Gastal, F., and Louarn, G. (2016). Intraspecific variation in thermal acclimation of photosynthesis across a range of temperatures in a perennial crop. AoB Plants 8:lw035. doi: 10.1093/aobpla/plw035
Zhang, J., Liu, S., Liu, C., Wang, H., Luan, J., Liu, X., et al. (2021). Different mechanisms underlying divergent responses of autotrophic and heterotrophic respiration to long-term throughfall reduction in a warm-temperate oak forest. For. Ecosyst. 8:41. doi: 10.1186/s40663-021-00321-z
Zhang, L. H., Chen, Y. N., Zhao, R. F., and Li, W. H. (2010). Significance of temperature and soil water content on soil respiration in three desert ecosystems in Northwest China. J. Arid Environ. 74, 1200–1211. doi: 10.1016/j.jaridenv.2010.05.031
Keywords: CO2 emission, elevation, Q10, representative concentration pathways, soil respiration
Citation: Takeda S, Majima R, Makita N and Takahashi K (2023) Five-year measurement data along a 1200 m elevational gradient reveals that global warming increases soil respiration. Front. For. Glob. Change 6:1145474. doi: 10.3389/ffgc.2023.1145474
Received: 16 January 2023; Accepted: 05 July 2023;
Published: 21 July 2023.
Edited by:
Jeong-Wook Seo, Chungbuk National University, Republic of KoreaReviewed by:
David Ellison, University of Bern, SwitzerlandCopyright © 2023 Takeda, Majima, Makita and Takahashi. This is an open-access article distributed under the terms of the Creative Commons Attribution License (CC BY). The use, distribution or reproduction in other forums is permitted, provided the original author(s) and the copyright owner(s) are credited and that the original publication in this journal is cited, in accordance with accepted academic practice. No use, distribution or reproduction is permitted which does not comply with these terms.
*Correspondence: Koichi Takahashi, a29pY2hpdEBzaGluc2h1LXUuYWMuanA=
Disclaimer: All claims expressed in this article are solely those of the authors and do not necessarily represent those of their affiliated organizations, or those of the publisher, the editors and the reviewers. Any product that may be evaluated in this article or claim that may be made by its manufacturer is not guaranteed or endorsed by the publisher.
Research integrity at Frontiers
Learn more about the work of our research integrity team to safeguard the quality of each article we publish.