- 1Department of Geographical Sciences, Taiyuan Normal University, Jinzhong, China
- 2Northwest Institute of Eco-Environment and Resources, Chinese Academy of Sciences (CAS), Lanzhou, Gansu, China
Leaf functional traits allow plant survival and maintain their ecosystem function. Salinity affects leaf functional traits, but coordination among leaf functional traits is poorly known and may depend on salt severity. To increase our understanding of the coordination of leaf functional traits under salt stress, we determined hydraulic, gas exchange, and physiological and biochemical parameters in Populus euphratica Oliv. (P. euphratica) grown under salinity treatments, as well as gas exchange parameters under different CO2 concentrations. We found that P. euphratica can reinforce its hydraulic capacity by increasing the water transfer efficiency of both its leaves and stems when a salinity threat occurs for a specific duration of stress. Its stems were more adaptable than leaves. The economic and hydraulic traits of P. euphratica leaves were consistent during the middle stages of salt stress, but inconsistent during the onset and late stages of salt stress. There was almost no biochemical limitation under severe salinity conditions, and CO2 enrichment of P. euphratica had a greater effect on leaf economic traits. The mechanism of toxic ion exclusion based on water availability and intracellular mechanisms in leaves contributed to salt tolerance when P. euphratica was exposed to salinity stress. There was also a coordination mechanism for the plants during increasing salt stress. The leaf intracellular traits of P. euphratica can coordinate with the leaf economic and hydraulic traits and form a defense mechanism to reduce salt damage and guarantee growth under saline conditions. In conclusion, P. euphratica, the main constructional species of riparian forests, adapts to saline environments by adjustment and coordination of leaf functional traits, ensuring survival. These results provide a scientific basis for riparian forest restoration.
1. Introduction
The area of salinized soil accounts for 7.6% of the global land area, and China is one of the major keepers of salinized land, distributed in the arid northwestern part of the country. Salinity stress is an important environmental factor that interferes with plant productivity and growth, causing decreased growth, productivity, and even death (Shamsi et al., 2020; Yang et al., 2021). Salt stress develops as a result of salt accumulation to toxic concentrations through evapotranspiration in the soil surface layer, particularly in semi-arid and arid areas (Deinlein et al., 2014; Yu et al., 2020).
Downstream areas of river basins in the arid area of northwestern China are known as green corridors due to the lush cover of riparian forests that are several miles wide. Populus euphratica (P. euphratica) is one of the few tall tree species that form forests in semiarid areas and maintain ecosystem functions in vulnerable oases (Chen et al., 2012). P. euphratica is a tree species with an extremely strong adaption to growth under an adverse environment where it can persist for decades and centuries (Si et al., 2014). Increases in soil salinization could further inhibit tree growth and increase the risk of plant mortality; however, plants change their functional traits to adapt to the environment (Zhang C. et al., 2022).
Leaf functional traits are key to the understanding of tree internal coordination and adaptation mechanisms to the environment; thus, coordination of leaf functional traits plays a critical role in realizing the physiological, ecological, and survival strategies of plants (Derroire et al., 2018; Zhang C. et al., 2022). Recent studies on tree response processes in the environment highlight the importance of hydraulic functional integrity for tree survival and that hydraulic failure can lead to tree mortality across multiple tree taxa (Adams et al., 2017; Powers et al., 2020). Leaves are a vital hydraulic choke point in the hydraulic system of plants, in which hydraulic resistance accounts for approximately 30–80% of the whole-plant resistance (Liu et al., 2014; Pan et al., 2016; Li et al., 2019).
The hydraulic function of a leaf is very sensitive to soil water potential (Pan et al., 2016), resulting in changes with drought and salt stress. Therefore, leaf hydraulic traits are essential indices of water conduction in plants that reflect the water transfer efficiency and plant safety condition under various environmental stress conditions. In addition, the physiological cause of tree mortality is a fatal depletion of non-structural carbohydrates resulting from CO2 fixation and carbon metabolism disorders, even carbon starvation (Anderegg et al., 2012). Moreover, the gas exchange level, a leaf economic trait, that reflects carbon investment and carbon allocation, can affect the construction of the hydraulic system (Petit et al., 2016; Dong and Jaume, 2018). It has been shown that organs with a short lifespan require lower economic investment and fewer resources, and thus, they could be easily rebuilt depending on the construction economics of plants (Pivovaroff et al., 2014). As stomata can link the water desorption and gas exchange processes, the leaf hydraulic and economic traits are associated at the leaf scale (Choat et al., 2018; Yin et al., 2018). There is a trade-off between the hydraulic and economic traits of trees at the leaf level, which affects plant survival and adaption to the environment (Liu et al., 2019; Yin et al., 2021). Therefore, leaf hydraulic and economic traits may exhibit coordination in adaption to saline environments.
A saline environment can have direct harmful effects on cell growth and intracellular traits; past research has shown that a physiological cause of tree mortality is lethal tissue dehydration with no shrinking ability (Anderegg et al., 2012). Osmotic adjustment is a common response to osmotic stress induced by high ion accumulation, which affects plant cell systolic traits involved in counteracting the loss of turgor by maintaining or increasing bulk intracellular compatible solutes (Per et al., 2017; Meena et al., 2019; Moukhtari et al., 2020). The contents of proline and soluble sugars, as vital intracellular compatible solutes in plants, are closely related to osmotic adjustment and the converse-succession-resistant capability of plants (Shamsi et al., 2020; Zelm et al., 2020; Afefe et al., 2021). In addition, the formation of reactive oxygen species (ROS) within plant cells caused by ion accumulation under salinity compromises cell contractility and disrupts the metabolic processes (Flowers and Colmer, 2015; Hussain et al., 2015; Ma et al., 2019). Plants can protect themselves from ROS-induced oxidative damage through enzymatic defense mechanisms, and the activation of antioxidant enzymes is critical for suppressing toxic ROS levels within cells (Hishida et al., 2014; You and Chan, 2015; AbdElgawad et al., 2016). Therefore, intracellular regulatory mechanisms including enzymatic defense and osmotic adjustment are important to the understanding of leaf intracellular traits.
Earlier studies showed that leaf hydraulic traits are under the influence of changes in cell shrinkage, including permeability of extravascular water pathways (Pou et al., 2013; Scoffoni et al., 2014). In addition, stomatal behavior affects not only gas exchange and water desorption but also osmotic regulation and ROS accumulation (Cao et al., 2014; Hartmann and Trumbore, 2016; Karst et al., 2017). Therefore, there appears to be a coordination of leaf hydraulic, economic, and intracellular traits for plant acclimatization to saline environments. Understanding the coordination of leaf functional traits in saline environments is essential for a holistic perception of plant resistance mechanisms to adverse conditions.
Differences in the physiological and biochemical responses of riparian plants to salt stress shed light on adaptive traits (Li et al., 2013, 2019; Si et al., 2014; Rajput et al., 2015; Pan et al., 2016) and help reveal the mechanisms of the converse-succession-resistant capability of P. euphratica. Some reports indicate that P. euphratica has the physiological ability to tolerate salinity stresses, but little is known about the physiological aspects and coordination among leaf functional traits to adapt to salt stress. The growth mechanisms of P. euphratica under such conditions are not fully explained because leaf functional traits have not been addressed in previous research studies. Nevertheless, P. euphratica survives saline conditions and forms forests, indicating that its leaf functional traits adapt to stressful environments. Therefore, a greater understanding of the adaptation and coordination of leaf functional traits in P. euphratica is essential for understanding salt stress adaptation. The goal of the present study is to elucidate the effects of salinity stress on the leaf hydraulic, economic, and intracellular traits and salt tolerance of P. euphratica. Specifically, we aimed to answer the following questions: (1) how do the leaf hydraulic traits of P. euphratica respond to changes in a salinity gradient and stress duration? (2) how do the leaf economic traits of P. euphratica respond to changes in a salinity gradient and stress duration? and (3) how do intracellular traits of P. euphratica respond to changes in a salinity gradient and stress duration?
2. Materials and methods
2.1. Study sites
The study was carried out in the Alxa Desert Eco-Hydrology Experimental Research Station in Ejina oasis in northwestern China (41°40′ N−42°40′ N, 100°15′ E−101°15′ E), which is a part of the Heihe River Basin and located downstream (Figure 1). In this area, 75% of precipitation occurs during June, July, and August, and the annual precipitation averages 38 mm. Evaporation is very strong at 3,390 mm annually, exceeding precipitation by more than 90 times. The area belongs to the extremely arid regions in China, with an annual average temperature of 8.2°C and high temperatures usually occurring from June to August (Si et al., 2014). Local groundwater is the main source of water for plants in this region and it is derived mostly from the discharge in the middle and upper streams of the Heihe River (Zhou et al., 2013). The soil in this region originates from fluvial sediments; the soil salinity is up to 33% (Si et al., 2014).
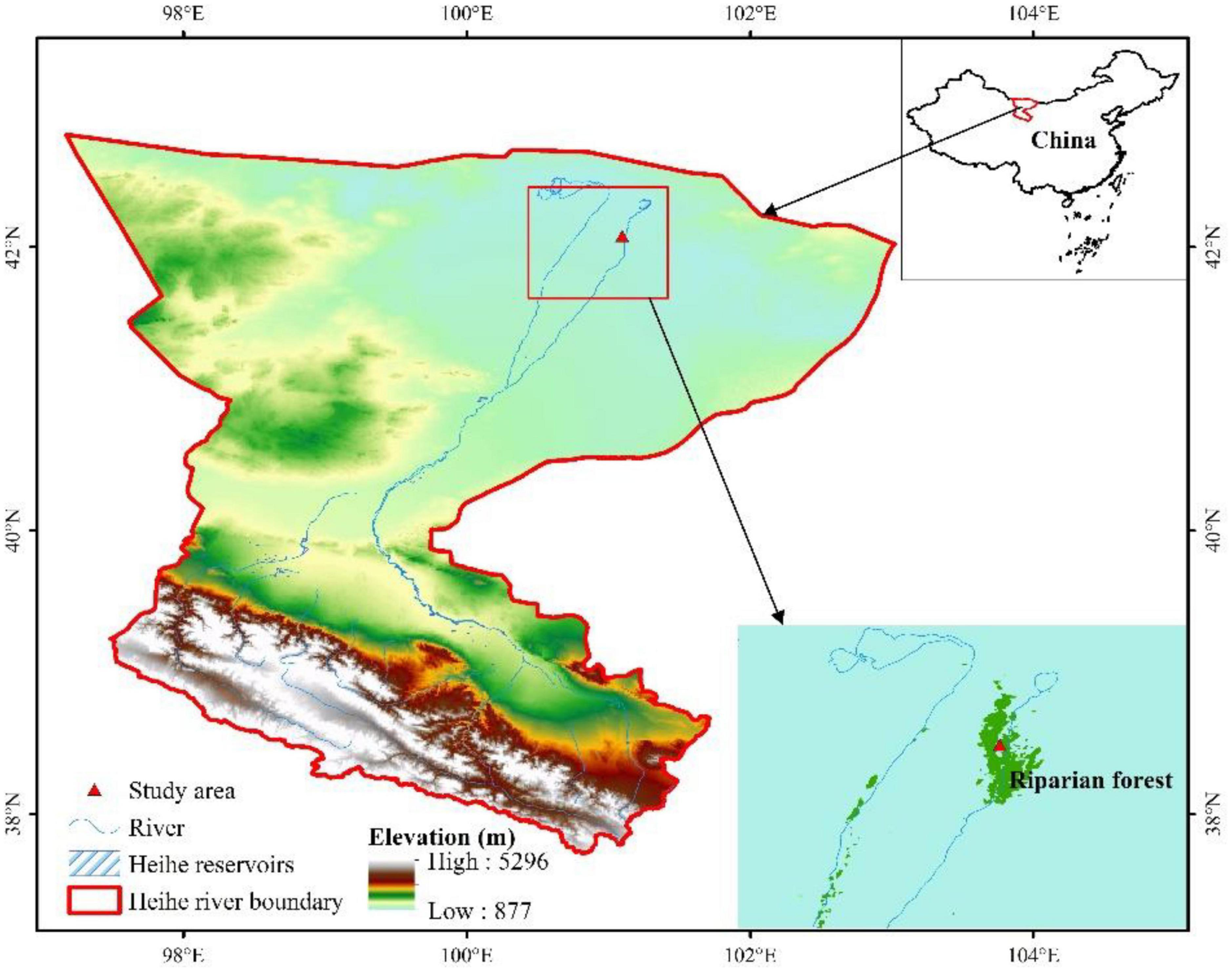
Figure 1. Location and elevation of the study area in the Heihe River Basin in the arid inland region of China.
2.2. Plant materials
P. euphratica saplings were cultivated on the local forest farm in Ejina Banner for 2 years before transplantation. A total of 100 P. euphratica saplings were transplanted into pots for this study and placed outdoors in the natural environment in early April. We selected healthy, straight, non-stressed, and well growing saplings as samples for salt treatments when saplings grew in pots for more than 3 months. Samples were assigned to one of several salt treatments, each with four replications. The salinity gradient included NaCl at concentrations of 100, 200, 300, and 400 mmol/L. Each salt concentration treatment was given for the following durations: CK (0 days salinity), 7 days (salinity period lasted for 7 days), 14 days (salinity period lasted for 14 days), and 21 days (salinity period lasted for 21 days). The volume of the solution was 3 L per pot, which was poured at one time into pots for all treatments; subsequently, all treated pots were watered every 7 days for the rest of the time. All saplings were measured at once after the salt treatments. The starting day of treatment was varied to ensure that the required salinity duration was met on the same day for each group to avoid growth-induced differences in different salt treatments.
2.3. Measurement of hydraulic conductance
A high-pressure flow meter (HPFM-GEN3, Dynamax Inc., Houston, TX, USA) was used for determining the original hydraulic conductance using the water perfusion method (Tyree et al., 1995). Briefly, HPFM pushes distilled water into certain parts of plants and determines a homologous flow rate. As a result, the original hydraulic conductance is obtained from the relationship between flow rate and imposed pressure. Measurements of the original hydraulic conductance in shoots were determined with imposed pressure of approximately 300–350 kPa until the rate of water flow was stable. The determinations took approximately 10 min, and the original values had to be corrected to the values at 25°C to make up for water viscosity variations during each measurement.
The original hydraulic conductance of roots and shoots was determined as above and the specific steps were as follows: First, the stalk was excised from the main root at 3–4 cm above the soil surface. The original hydraulic conductance was obtained by attaching the ends of whole roots/shoots to the instrument for testing. In vitro measurement was performed for the whole shoot system, while in situ measurement was performed for the whole root system. Then, new values were obtained by removing the leaves—the original hydraulic conductance of the stem and then the original hydraulic conductance of the leaves were determined from the hydraulic pressure analog based on Ohm’s law (Alsina et al., 2011). Finally, the water transfer efficiency of different plant parts was expressed using the leaf-specific hydraulic conductance values—the hydraulic conductance per leaf area (K, kg m–2 s–1 MPa–1) was obtained from the corrected original hydraulic conductance that was adjusted for the leaf area. The hydraulic contribution of specific parts was reflected in the hydraulic resistance ratio through hydraulic segmentation, which was determined using the hydraulic resistance (the reciprocal of original hydraulic conductance) of specific parts relative to the whole plant, and expressed as a percentage. The formula for the relations among leaf-specific hydraulic conductance of different tissues is as follows:
where Kleaf is the leaf-specific hydraulic conductance of leaves, Ktwig is the leaf-specific hydraulic conductance of a fully-leaved twig, and Kstem is the leaf-specific hydraulic conductance of the bare stem.
2.4. Total leaf area
A total of 5–10 leaves per sapling from each group were placed on a grid paper and the edges were traced to obtain the leaf area. The total number of grids was counted in which the leaves occupied more than 50% of the grid; then, the area of a grid was multiplied by the number of square grids to obtain the leaf area. Subsequently, the leaves were washed and dried at 80°C and weighed to obtain biomass. The SLW (specific leaf weight) values of each sapling were calculated by dividing the leaf area by biomass. Fresh leaves were also stored in liquid nitrogen for measurements of physiological and biochemical parameters and their leaf area was measured as mentioned previously. The area of the remaining leaves was obtained from the dry weight divided by the SLW values, based on the assumption of the same SLW per sapling. The leaf area of the whole plant was obtained by adding the three parts of the leaf area calculated before.
2.5. Gas exchange
An LI-6400 portable photosynthesis system (LI-COR, Lincoln, NE, USA) was used to determine transpiration (E, mmolH2O⋅m–2⋅s–1), net photosynthetic rate (PN, μmolCO2⋅m–2⋅s–1), and stomatal conductance (gs, molH2O⋅m–2⋅s–1). Water use efficiency (WUE, μmolCO2⋅mmolH2O–1) was determined as the ratio of the net photosynthetic rate and the transpiration rate. Three or four fully expanded leaves from 3 to 4 branches on the top of each shoot system were used for the measurement. The LI-COR settings were 1,200 μmol m–2 s–1 for light intensity (with a 6400-02B red-blue LED Light Source) and 400 μmol mol–1 and 600 μmol mol–1 for CO2 concentration (using 6400-01 CO2 mixer). These measurements were conducted immediately after the salt treatments.
2.6. Physiological and biochemical parameters
Testing kits (Comin Biotechnology Co., Ltd., Suzhou, China) were used for measuring malondialdehyde content (MDA) and the activities of superoxide dismutase (SOD), peroxidase (POD) and catalase (CAT). A 0.1 g sample was ground in liquid nitrogen before weighing using an analytical balance. Then, the sample was homogenized in phosphate buffer with a pH of 7.8. Then MDA, SOD, POD, and CAT analyses were conducted after the homogenate was centrifuged at 12,000 rpm at 4°C for 15 min. The resulting supernatant was used for the determination of SOD, POD, and CAT activity by thiobarbituric acid chronometry, nitroblue tetrazolium, and guaiacol staining methods, respectively. MDA content was determined using ammonium molybdate colorimetry. After grinding in liquid nitrogen, a 0.1 g sample was weighed and extracted with 5 ml of 3% sulfosalicylic acid. The homogenate was placed in a centrifuge tube and extracted by vibrating in a hot water bath for 10 min at 95°C. After the homogenate was centrifuged at 10,000 rpm at 25° for 10 min, the content of proline was determined by colorimetry with acid ninhydrin a cooled homogenate. Then, 0.1–0.2 g samples were weighed and then ground with a small amount of distilled water. The volume of the homogenates was filled to 10 ml with deionized water and heated in a hot water bath for 30 min at 95°C; the supernatant was obtained by centrifuging at 3,000 rpm. Then, the anthrone colorimetric method was used for soluble sugar content determination in the supernatant.
2.7. Data treatment and statistical analysis
Analysis of variance (ANOVA) was used to test for differences in hydraulic values, net photosynthetic rate, stomatal conductance, water use efficiency, SOD activity, POD activity, CAT activity, MDA content, proline content, and soluble sugar content among different salinity treatments. We used the least significant difference test (LSD) based on post-hoc means. Pearson Product Moment Correlation was used to test the statistical significance of the correlations. A probability value of p < 0.05 was used to indicate statistically significant differences. Data were presented as means with standard error and statistically analyzed using SPSS 19.0. The figures were plotted using Origin 8.0.
3. Results
3.1. Variability in hydraulic characteristics
At the same concentration, leaf-specific hydraulic conductance in twigs (Ktwig) significantly increased with salinity duration from CK to 14 days for NaCl concentrations of 100, 200, and 300 mmol/L. The maximum values were 4.13 × 10–3, 3.54 × 10–3, and 3.33 × 10–3 kg m–2 s–1 MPa–1, respectively, at increasing concentrations. Subsequently, Ktwig significantly decreased when salinity stress lasted for 21 days and was 3.03, 1.59, and 1.11 times the value of CK treatment (no salt), respectively. The Ktwig value increased gradually to 1.36 × 10–3 kg m–2 s–1 MPa–1 with an increase in salinity duration from CK to 14 days, and then significantly decreased when the salt stress lasted for 21 days at a concentration of 400 mmol/L NaCl. Moreover, the Ktwig value decreased with increasing NaCl concentrations for the same duration (Figure 2A).
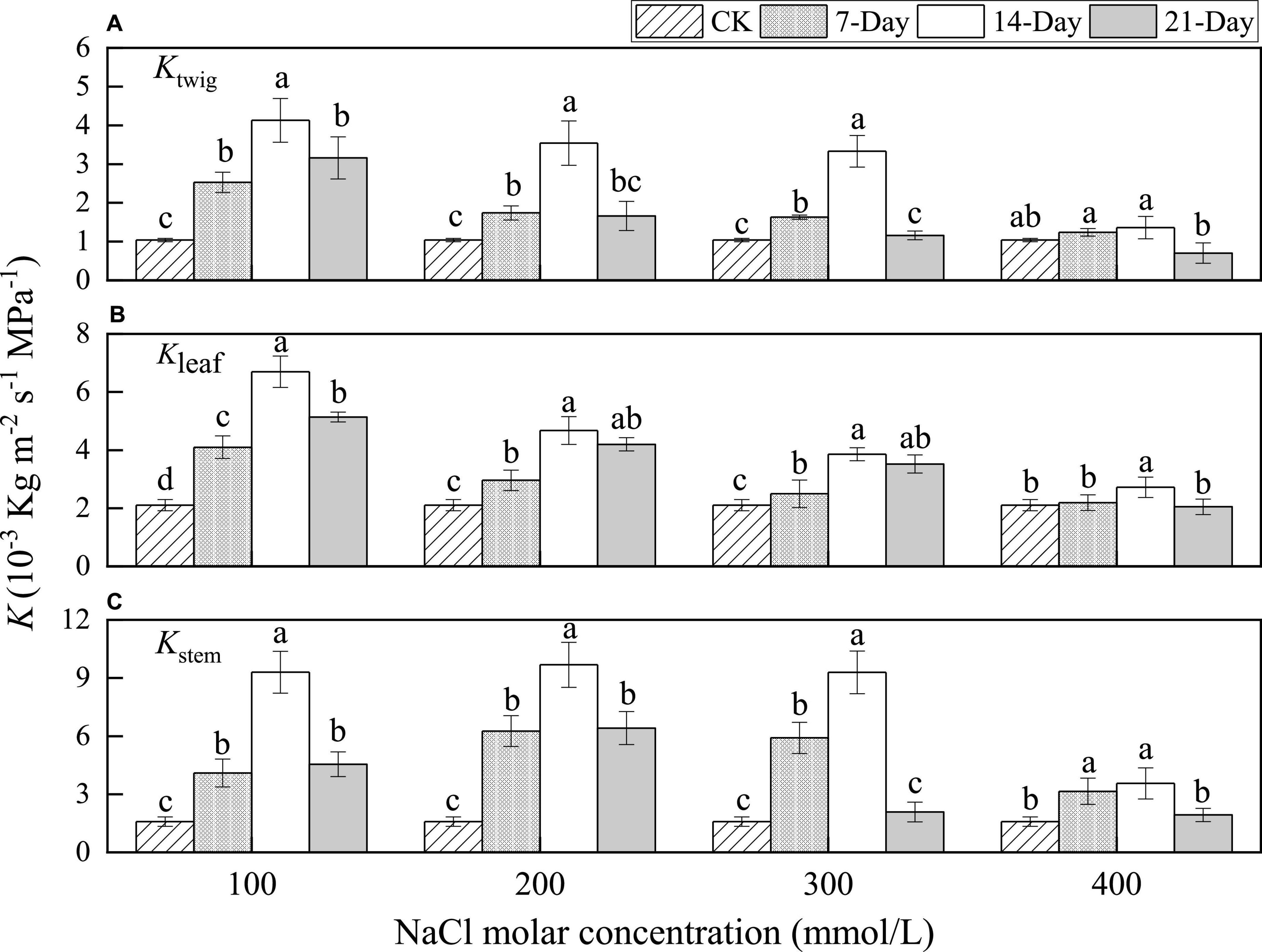
Figure 2. Changes in the leaf-specific hydraulic conductance values of whole twig (A), leaf (B), and stem (C) under different salinity concentrations and durations of stress. Different lower-case letters denote significance levels based on analysis of variance (ANOVA) post-hoc means with least significant difference test (LSD) analysis (p < 0.05). Data are means with standard error.
The trend in leaves was the same as that in whole twigs. At the same concentration, leaf-specific hydraulic conductance in leaves (Kleaf) significantly increased from CK to 14 days and then decreased from 14 to 21 days for all NaCl concentrations. The values of Kleaf at 21 days were 2.43, 1.99, 1.67, and 1.18 times the value of CK treatment, respectively, for increasing concentrations. This trend of changes showed that leaves enhanced water transfer efficiency under salt stress. The Kleaf values decreased gradually with the increase in NaCl concentration for the same duration. The results exhibited that the water transfer efficiency of leaves was significantly reduced by salinity stress for the same duration (Figure 2B).
The maximum values of leaf-specific hydraulic conductance in stems (Kstem) were 9.31 × 10–3, 9.68 × 10–3, 9.29 × 10–3, and 3.56 × 10–3 kg m–2 s–1 MPa–1 at the salinity duration of 14 days, respectively, at increasing concentrations. At the same salinity duration, the values of Kstem increased initially and then decreased with the increase in NaCl concentrations, differing from those of Ktwig and Kleaf. Moreover, the maximum values of Kstem were higher at all other concentrations but sharply reduced for the concentration of 400 mmol/L NaCl. This indicated that stems were more adaptable than leaves under mild and moderate salt stress conditions (Figure 2C). This illustrated that both the leaves and stems of P. euphratica could enhance the water transfer efficiency upon increased durations of salt stress.
The leaf hydraulic resistance was 64% relative to that of the whole twig in the CK treatment. The values of leaf hydraulic resistance relative to that of the whole twig decreased from 71.4 to 64.5% after the 7 days treatment with increasing NaCl concentrations. The leaf hydraulic resistance ratio declined from 70.6 to 56.2% after the 14 days treatment with increasing NaCl concentrations. There was a significant decline in the leaf hydraulic resistance ratio from 67.5 to 39.4% after the 21 days treatment with increasing NaCl concentrations. This indicated that the hydraulic contribution of leaves in twigs was significantly enhanced by salinity stress over the same duration. The hydraulic resistance in leaves accounted for 39.4% of the whole twigs in the treatment for 21 days with 400 mmol/L NaCl, which was lower than that of bare stems for the first time (Figure 3). This showed that the hydraulic contribution of leaves in twigs was more than half.
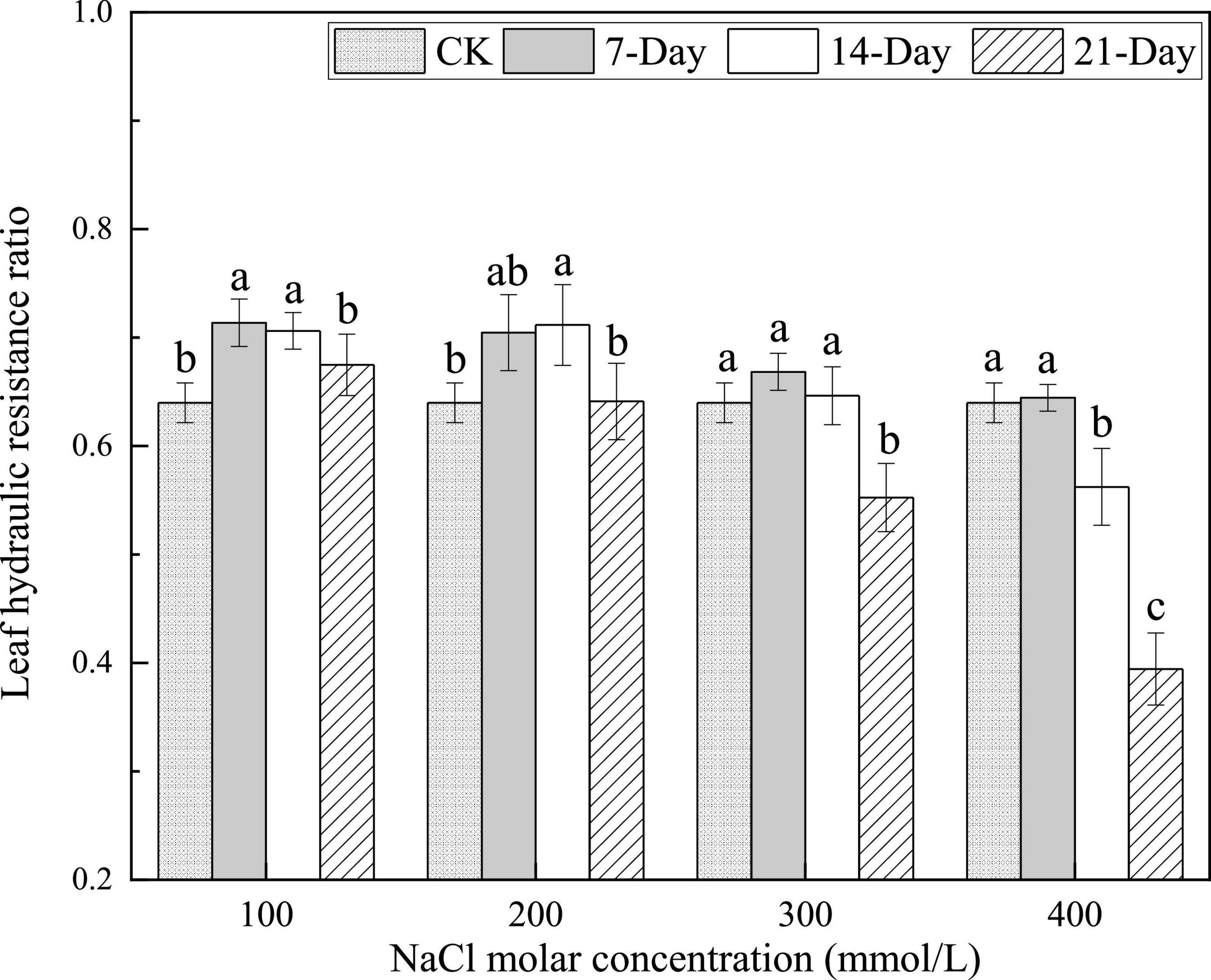
Figure 3. Changes in the leaf hydraulic resistance ratio under different salinity concentrations. Different lower-case letters denote significance levels based on ANOVA post-hoc means with LSD analysis (p < 0.05). Data are means with standard error.
At the same concentration, the leaf hydraulic resistance ratio first increased and then decreased with an increase in salinity duration for all NaCl concentrations. The leaf hydraulic resistance ratio values obtained at 21 days were not statistically significantly different from those obtained after the CK treatment for the 100 and 200 mmol/L NaCl concentrations, but were 13.6 and 38.4% lower for the concentrations 300 and 400 mmol/L NaCl (Figure 3). The decrease in the proportion of hydraulic resistance represents the increase in hydraulic transport contribution. This indicated that the hydraulic contributions of leaves in whole twigs first enhanced and then decreased depending on salinity duration for the same NaCl concentrations. The more severe the stress is, the greater the impact is.
3.2. Variability in gas-exchange characteristics
At the same concentrations, there was a sharp decline in the net photosynthetic rate (PN) between CK and 7 days of treatment, ranging from 50.8 to 8.6% of the value in CK, with increasing concentrations. This indicated that the higher the initial salt concentration, the greater the PN decreased in the short term. Subsequently, the value of PN significantly increased, indicating an adaptation to salt stress. Meanwhile, the more severe the salinity stress, the smaller the values of PN for the same duration (Figure 4A).
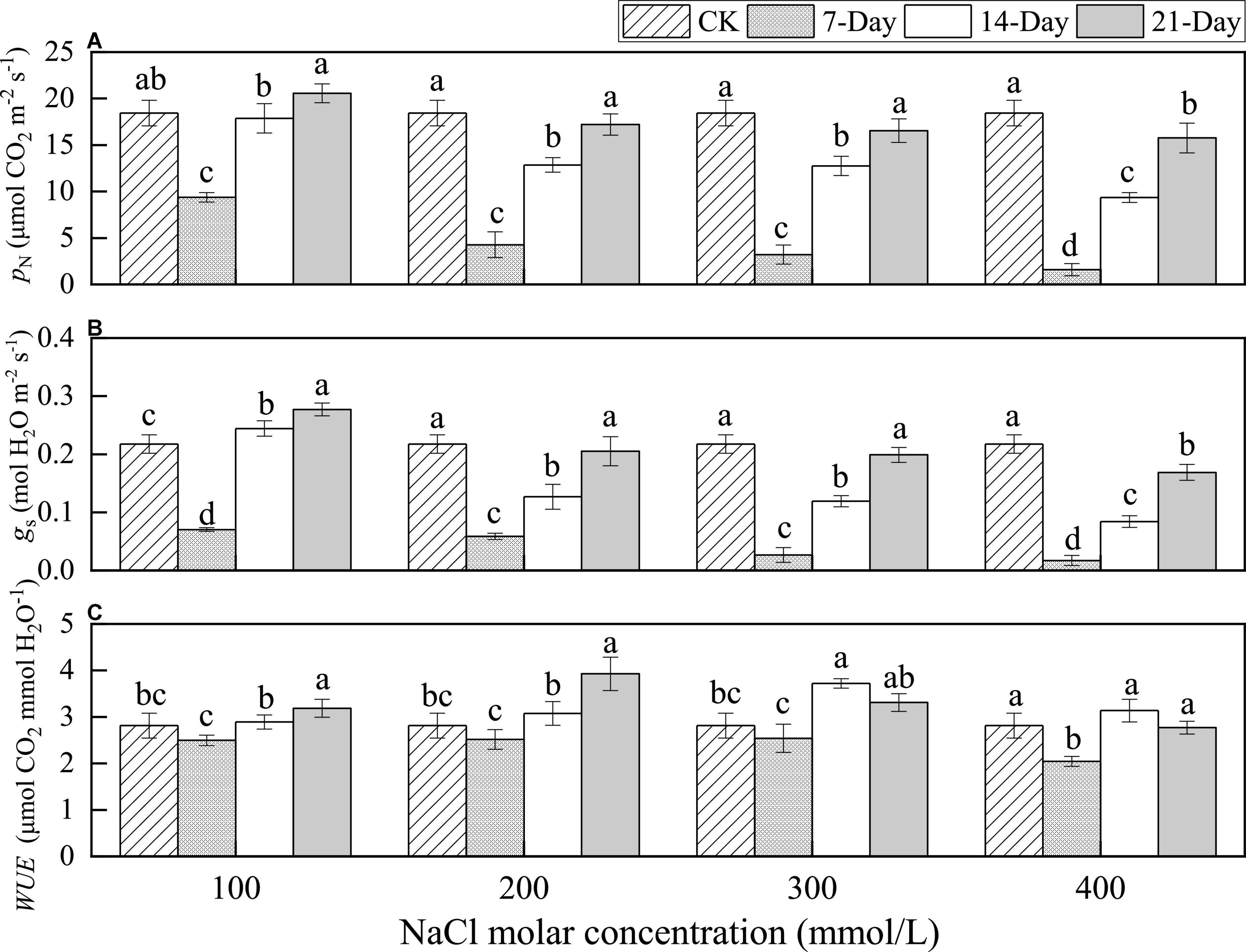
Figure 4. Changes in the net photosynthetic rate (A), stomatal conductance (B), and water use efficiency (C) under different salinity concentrations. Lowercase letters over the bars denote significance levels based on ANOVA post-hoc means using LSD analysis (p < 0.05).
At the same concentration, the trend in stomatal conductance (gs) was the same as that in PN but gs decreased by day 7 of the treatment; the decreasing amplitude range was from 67.7 to 92.2% of the value in CK with increasing concentrations, which was higher than that of PN. This indicated that the initial salt stress effect on gs was even greater than that on PN in the short term. Then gs increased between 7 and 21 days, and this indicated that both gs and PN could be enhanced after a short-term adaptation for certain levels of salt stress. Meanwhile, the more severe the salinity stress, the smaller the values of gs at the same duration. At day 21, the gs values were significantly higher than those at CK for 100 mmol/L NaCl treatment. There was no significant difference between these values and those at CK for 200 and 300 mmol/L NaCl treatment. The gs values were significantly lower than those at CK for 400 mmol/L NaCl treatment (Figure 4B). This indicated that the longer the stress duration, the slower the recovery of gs with increasing salinity.
Although there was a sharp decline in both the PN and gs values at CK and at day 7, there was no significant difference in the water use efficiency (WUE) between day 7 of the treatment and at CK. The WUE trend was the same as that for stomatal conductance for 100 and 200 mmol/L NaCl treatments, which had the maximum value after 21 days. The WUE first increased at days 7 and 14 and then decreased for 300 and 400 mmol/L NaCl treatments. This was not significantly different from that in the CK group after 21 days (Figure 4C). This indicated that P. euphratica could maintain the efficient usage of water under salt stress for a long time.
All values of PN increased when the CO2 concentrations increased from 400 to 600 μmol mol–1, which indicated that CO2 provision could enhance photosynthesis in P. euphratica under salt stress. The values of PN at days 7, 14, and 21 of the treatment increased by 37.9, 59.7, and 49.0%, respectively, with 100 mmol/L NaCl treatment (Figure 5A). They increased by 39.1, 43.1, and 33.1%, respectively, with 200 mmol/L NaCl treatment (Figure 5B) and by 75.5, 46.8, and 66.4%, respectively, with 300 mmol/L NaCl treatment (Figure 5C). The values increased by 97.5, 93.9, and 101.3%, respectively, with 400 mmol/L NaCl treatment (Figure 5D). This illustrated that the more severe the salt stress, the greater the enhancement effect of increased CO2 on photosynthesis for the same salinity duration.
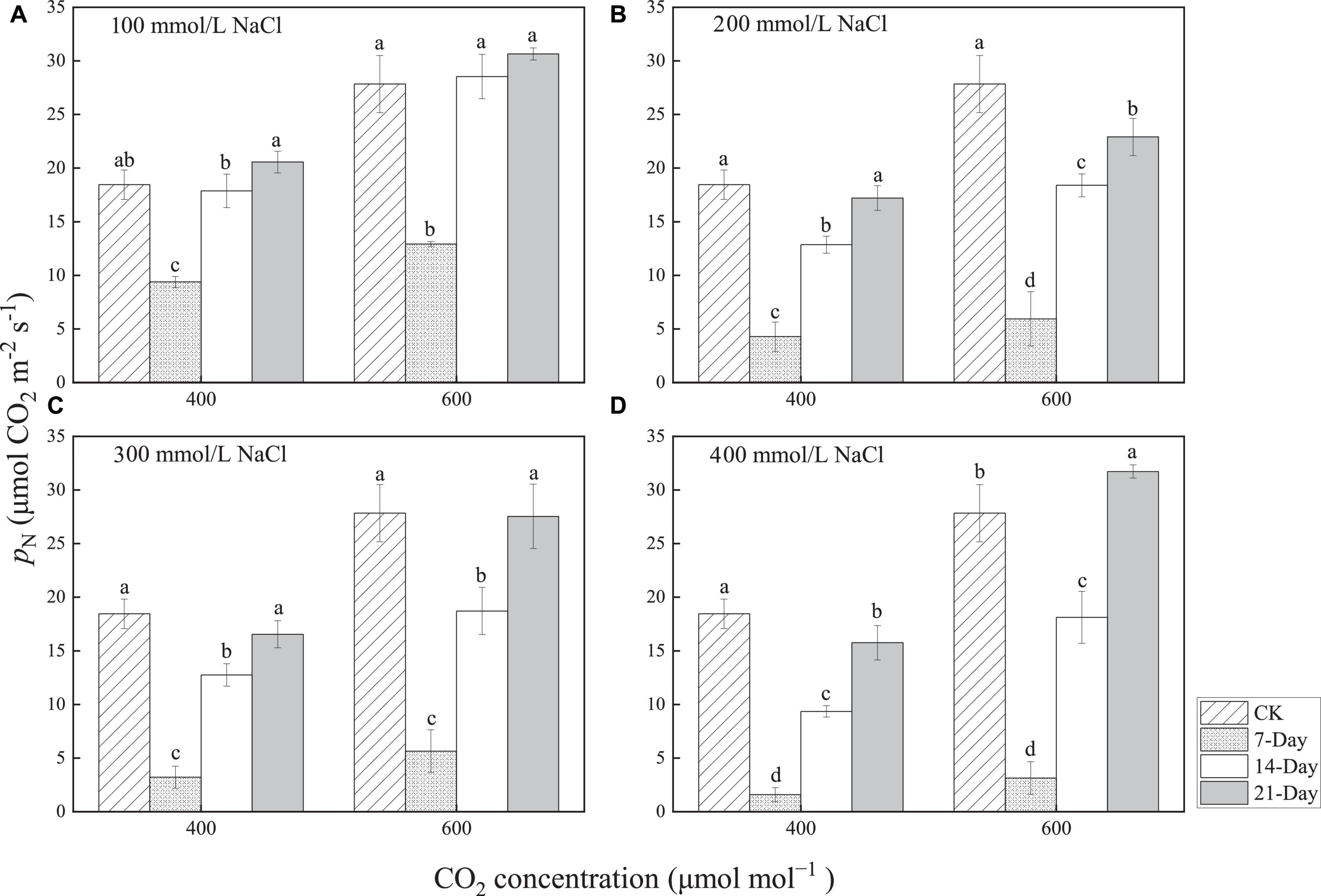
Figure 5. Changes in the net photosynthetic rate at 100 mmol/L NaCl (A), 200 mmol/L NaCl (B), 300 mmol/L NaCl (C), and 400 mmol/L NaCl (D) with the same CO2 concentration. Lowercase letters denote significance levels based on ANOVA post-hoc means using LSD analysis (p < 0.05). Data are means with standard error.
3.3. Variability in physiological and biochemical substances
The SOD activity was 156.5 U⋅g–1 at CK which increased with the durations of salt stress for all NaCl concentrations. Meanwhile, changes in salt stress during the early stages of salt stress were very slow with the durations of stress being relatively mild but rose rapidly under relatively severe salt stress. In addition, the SOD activity increased with increasing NaCl concentrations over the same salinity duration (Figure 6A).
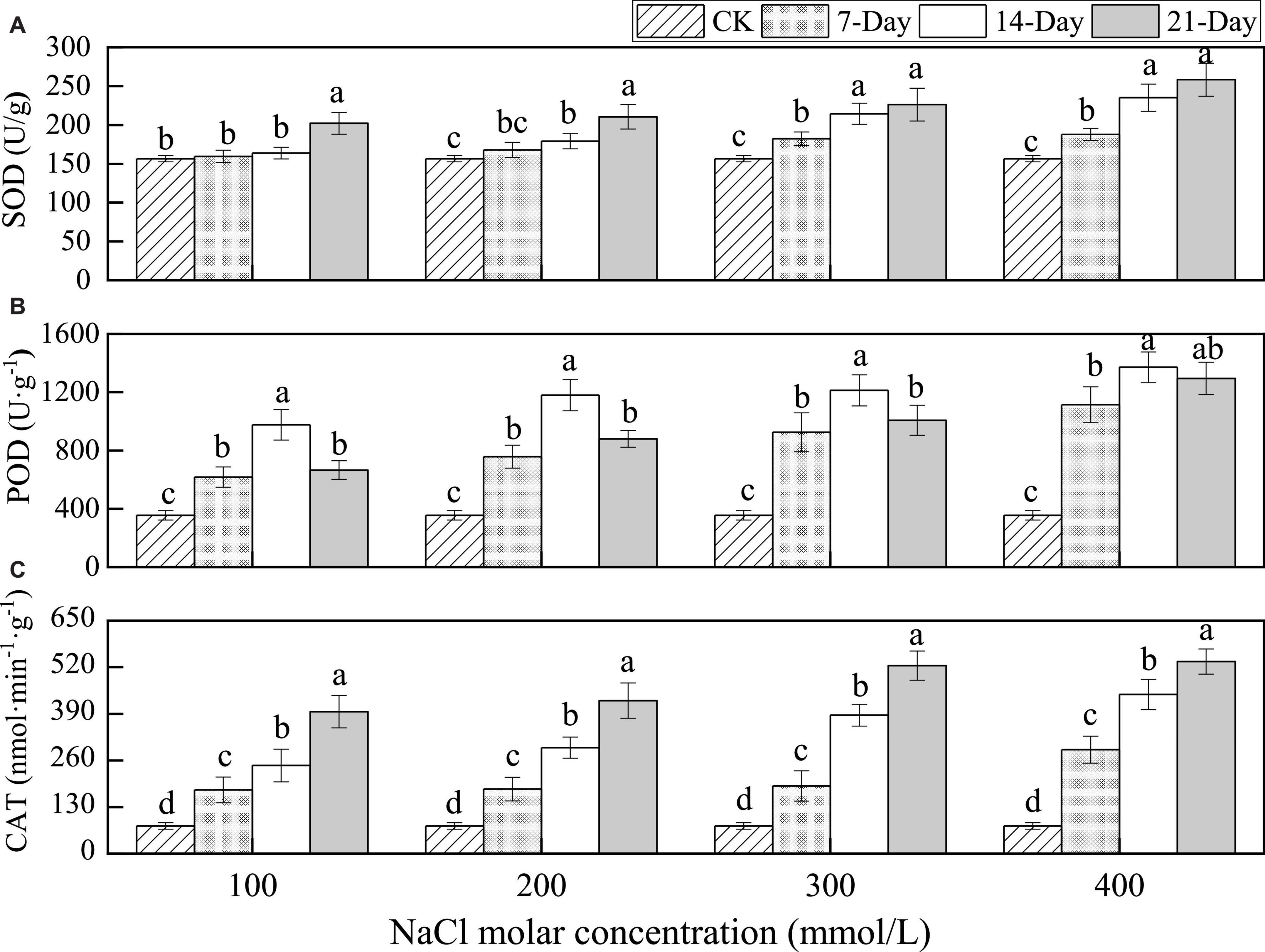
Figure 6. Changes in the superoxide dismutase (SOD) (A), peroxidase (POD) (B), and catalase (CAT) (C) under different salinity concentrations. Lowercase letters denote significance levels based on ANOVA post-hoc means using LSD analysis (p < 0.05). Data are means with standard error.
The POD activity was 354.37 U⋅g–1 at CK and it increased between CK and 14 days after the treatment and then decreased for all NaCl concentrations. The values ranged from 1.9 to 3.7 times the value during CK treatment and on day 21, respectively, with increasing concentrations. The POD activity enhanced with increasing NaCl concentrations over the same salinity duration (Figure 6B).
The CAT activity significantly increased from 77.42 nmol⋅min–1⋅g–1 at CK to a maximum value at day 21 under the same salt stress duration for all NaCl concentrations. The CAT activity enhanced with increasing NaCl concentrations over the same salinity duration (Figure 6C). This indicated that SOD, POD, and CAT in the leaves of P. euphratica could provide strengthened defensive protection under salt stress conditions.
There were no significant differences in proline content over different salinity durations for 100 and 200 mmol/L NaCl treatment. The proline content increased gradually between CK and at day 21 from 29.85 to 35.62 μg⋅g–1 for 300 mmol/L NaCl treatment and significantly from 29.85 to 45.89 μg⋅g–1 for 400 mmol/L NaCl treatment. In addition, the proline content accumulated with increasing NaCl concentrations over the same salinity duration (Figure 7A).
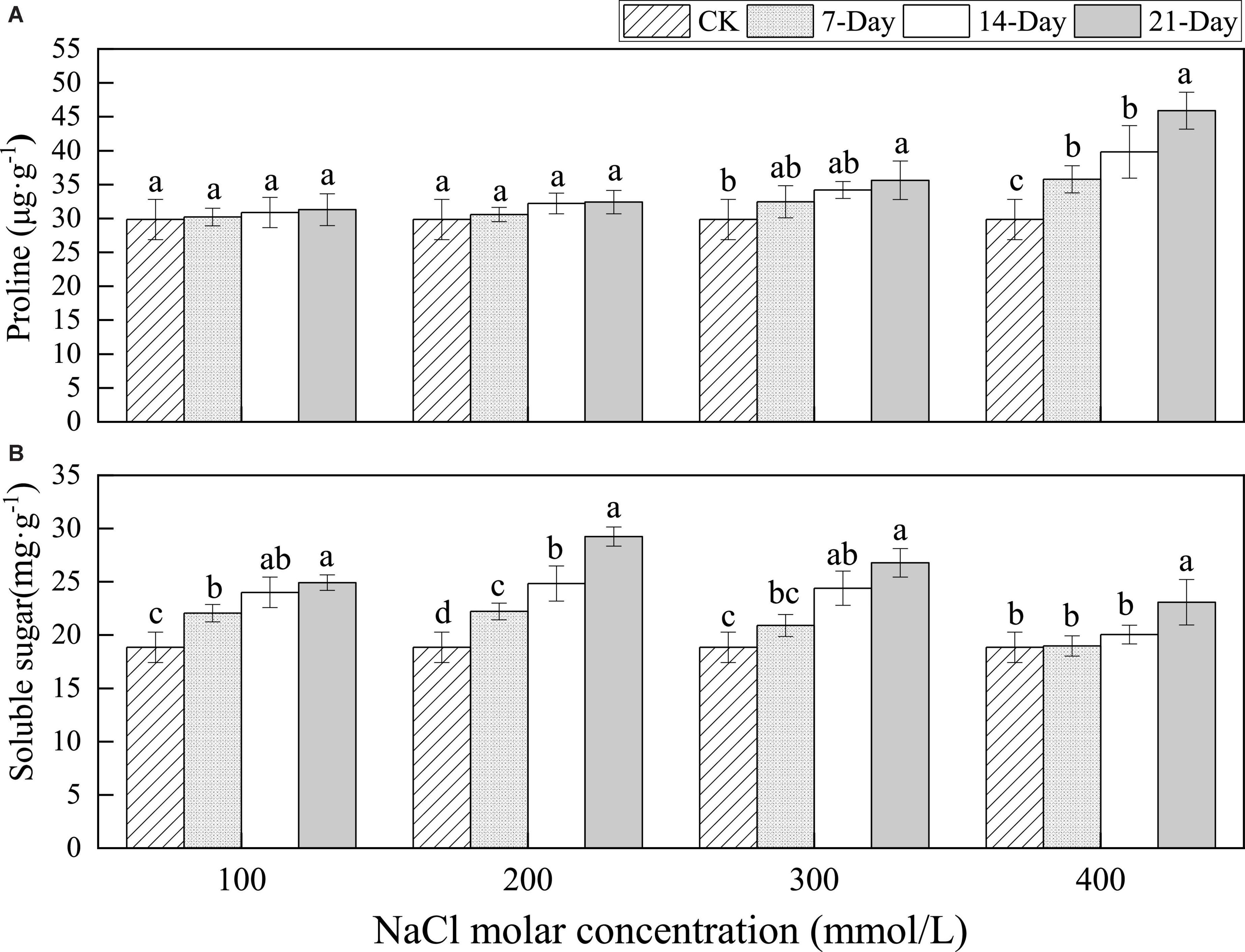
Figure 7. Changes in proline (A) and soluble sugar (B) with different salinity concentrations. Lowercase letters denote significance levels based on ANOVA post-hoc means using LSD analysis (p < 0.05). Data are means with standard error.
The soluble sugar content was 18.85 mg⋅g–1in CK, and it increased between CK and 21 days treatment at all NaCl concentrations. Meanwhile, changes in salt stress were very rapid and relatively mild with stress duration in the early stage but rose slowly under relatively severe salt stress. In addition, the soluble sugar content increased upon 100 to 200 mmol/L NaCl treatment and then decreased upon 200 to 400 mmol/L NaCl treatment under the same salinity duration (Figure 7B).
The MDA content was 37.64 nmol⋅g–1 in CK and it significantly declined between CK and day 7 and then increased on day 21 upon 100 and 200 mmol/L NaCl treatments. The values significantly declined between CK and day 14 and then increased at day 21 upon 300 mmol/L NaCl treatment. The values gradually decreased until day 21 upon 400 mmol/L NaCl treatment and the minimum values were at day 21. The time node at which the minimum values occurred at different salt concentrations indicated that the lighter the salt stress, the faster the recovery of the MDA content. The minimum values of MDA content were approximately 88 to 81% of the value in CK treatment for 100 to 400 mmol/L NaCl treatment, respectively, (Figure 8). This demonstrated that the MDA content could be maintained at a low level under salt stress, reflecting relatively light stress-induced damage to cell membranes.
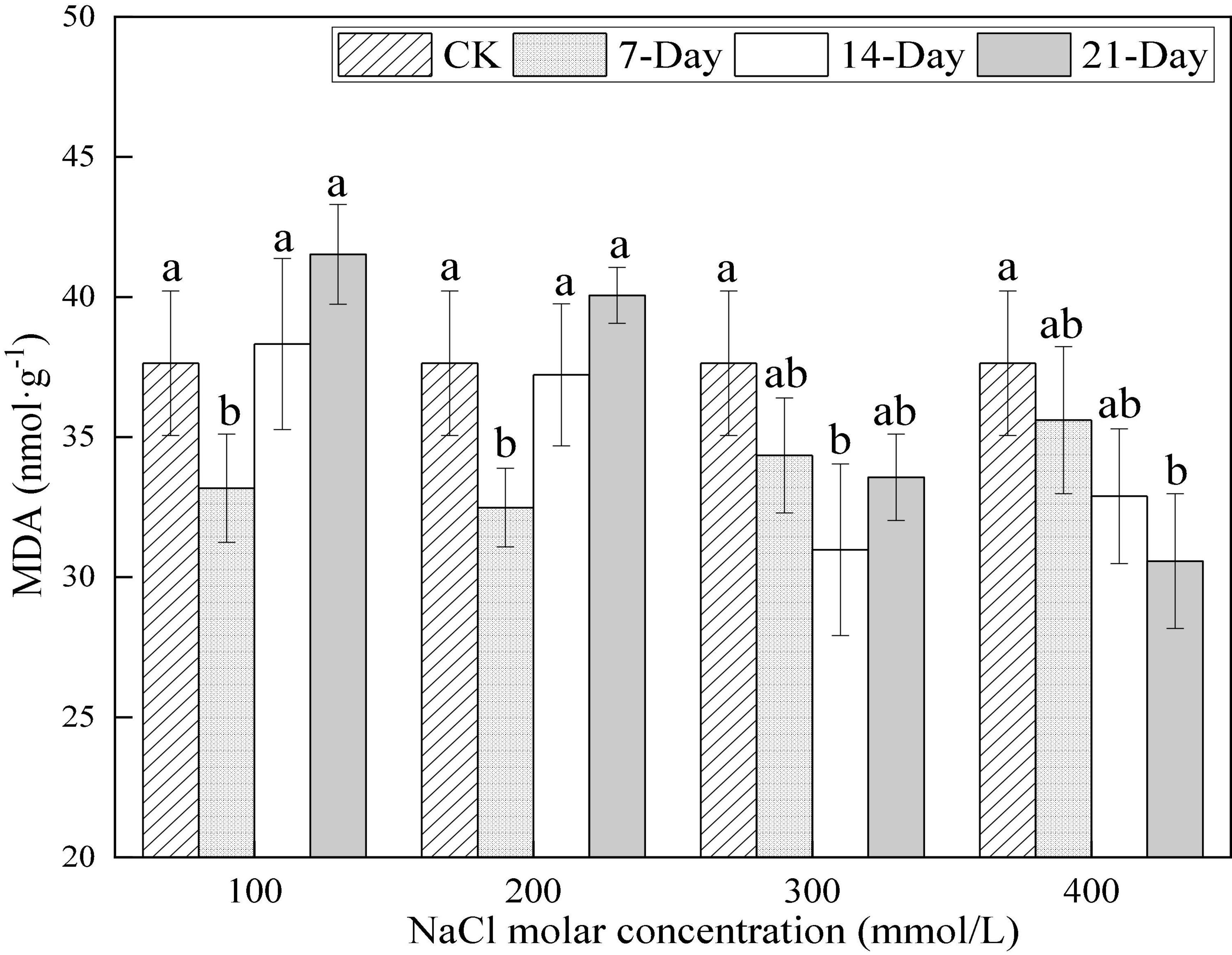
Figure 8. Changes in malondialdehyde (MDA) content under different salinity concentrations. Different lower-case letters denote the significance levels based on ANOVA post-hoc means with LSD analysis (p < 0.05). Data are means with standard error.
4. Discussion
4.1. Leaf hydraulic traits under salinity stress
The hydraulic capacities of both leaves and bare stems were higher than those of whole twigs as water in whole twigs needs to travel longer water channels. When the salt concentration did not exceed 400 mmol/L, the hydraulic conductance of both leaves and stems was enhanced with the duration of stress not exceeding 14 days. It showed that P. euphratica can reinforce its hydraulic capacity by increasing its water transfer efficiency when a salinity threat occurs for a specific duration. Meanwhile, the greater the salt concentration, the lower the increase in the hydraulic capacity of leaves over the same duration of stress. Our results also suggested that increasing salt concentrations attenuated the degree of enhanced water transport performance than prolonged stress durations for leaf hydraulic traits. Moreover, the salt concentration of 100 mmol/L made a great positive impact on the hydraulic capacity of leaves. In contrast, the salt concentrations of 200 and 300 mmol/L positively affected the hydraulic capacities of stems more than the 100 mmol/L concentration over the same duration of stress. These results indicated that stems in P. euphratica were more adaptable than leaves under mild and moderate salt stress, and the self-adaptive and regulatory capacity of water transfer was weakened under severe salt stress with a salt concentration of 400 mmol/L.
The construction costs in plants could be reflected in the values of hydraulic resistance, and it has been shown that organs with a short lifespan require lower economic investment owing to the stronger hydraulic resistance (Pivovaroff et al., 2014). Leaves with higher hydraulic resistance required higher construction costs than stems, and the leaf hydraulic resistance increased for all salt concentrations over a shorter duration, thus reflecting the decreased firmness of leaves. Zeng et al. (2009) showed that a salt elimination mechanism in P. euphratica operated by allocating large amounts of toxic salt ions into leaves that are ultimately abscised. There was also a significant decline ranging from 67.5 to 39.4% in leaf hydraulic resistance relative to that of whole twig under severe salinity stress over a longer duration, which was caused by the enhanced hydraulic resistance of the whole twig. These results also reflected weakened effectiveness of overall hydraulic capacity and decreased water availability ultimately. Our study revealed that the toxic ion exclusion mechanism in leaves was a contributing factor in salt tolerance based on water availability when P. euphratica was exposed to salinity stress.
4.2. Leaf economic traits under salinity stress
Water use efficiency could reflect the amount of dry matter produced by plants per unit of water consumed, which was adversely affected by other physiological and biochemical processes, but not photosynthesis, during the onset of salt stress (Minh et al., 2016). Enhanced water use efficiency occurs in some tree species, while some plants exhibit low water use efficiency under salinity conditions (Li et al., 2022; Zhang Y. et al., 2022). In general, a reduction in photosynthesis is a response to an initial osmotic shock, caused by some degree of salt stress, resulting from stomata closure (Li et al., 2013). Although the photosynthetic rate decreased sharply in the early stage of salt stress, P. euphratica tried to alleviate salinity stress by decreasing its stomatal conductance and increasing WUE; this indicated that changes in stomatal performance had a greater effect on water loss and WUE than photosynthesis, in agreement with past research (Rajput et al., 2015). It has been pointed out that when plants are grown in a saline environment, improved WUE is favorable to the survival and development of the species (Rajput et al., 2015). The results showed that P. euphratica can reinforce its water use efficiency accompanied by an increase in water transfer efficiency when a salinity threat occurs at a specific duration of stress. Water use efficiency began to decline with stress duration at relatively high salinity gradients, and comprehensive physiological and biochemical processes could ameliorate the negative effects of salinity through decreased water use efficiency even in a long term.
A large number of studies have shown that stomatal conductance decreases due to stomatal closure, which restrains the availability of CO2 and fixation of carbon in the leaves with an increase in salt concentration (Liu et al., 2014; Duan et al., 2018; Lawson and Matthews, 2020). Stomatal conductance was lower under saline conditions than in CK except for 100 mmol/L, which affected CO2 diffusion in leaves pre-dominantly triggered by salt stress (Liu and Suarez, 2021). Past findings in woody plants also indicated that increasing CO2 concentration in leaves and active sites of Rubisco ultimately enhanced light-saturated photosynthetic rates up to 2.8 times (Duan et al., 2015). Elevated CO2 compensated for the reduction in CO2 supply caused by stomatal limitation on leaf photosynthesis and improved leaf photosynthetic performance (Pérez-López et al., 2012; Eller et al., 2014). Our research showed that elevated CO2 could improve photosynthesis, providing evidence that the main limitation was a reduction of CO2 diffusion into leaves due to the stomatal effect rather than a biochemical limitation of CO2 assimilation, even under the high salinity concentration of 400 mmol/L. As a result, CO2 enrichment of P. euphratica had a greater effect on photosynthesis and leaf economic traits by CO2 assimilation and carbon fixation.
The significantly lower stomatal conductance exhibited a higher stomatal limitation upon sudden increases in salt stress at day 7 when leaf photosynthesis was eliminated by at least half. This indicated that it would take more than 7 days to recover leaf photosynthesis although no biochemical limitation to photosynthesis was observed under the circumstances. Salt-stressed leaves required higher ambient concentrations of CO2 to exhibit the same or higher photosynthesis than exhibited by leaves without salt stress at a maximum CO2 assimilation rate; elevated CO2 may render different results in different species under salt stress (Xu et al., 2016; Liu and Suarez, 2021). A 50% increase in carbon dioxide had a significant effect on photosynthesis in this study. In summary, the effects of salt stress on photosynthesis were eliminated by supplementing CO2 to leaves; this was due to alleviating the diffusion resistance for CO2 through reopened stomata or higher concentration gradient of atmospheric CO2 for P. euphratica with improved salt tolerance. Moreover, CO2 enrichment in P. euphratica under lower salt stress showed a relatively weak response but the effect was greater in plants in severe salinity conditions.
4.3. Coordination of hydraulic traits and economic traits under salinity stress
An intact water supply through the xylem pathway is a necessity for plant growth and survival to supplement water lost from stomata by transpiration (Sack and Scoffoni, 2013). The existing evidence shows that stomata performance is highly correlated with hydraulic systems, controlling the water supply capacity of plants (Zhang et al., 2013). Stomatal conductance, which is one of the major regulators of water flow in plants in the short term, could be impacted by enhanced water transfer efficiency (Liu et al., 2014; Pan et al., 2016). A lower leaf hydraulic capacity was found concomitantly with declining stomatal conductance in grasses and crops exposed to water stress (Corso et al., 2020). In this study, there was a significant decline in stomatal conductance at day 7 of stress but with increasing hydraulic capacity of leaves, the improved stomatal conductance of P. euphratica under mild and moderate salt stress was likely due to the water transfer efficiency in stems and sufficient water in leaves. Our study also showed a notable reduction in photosynthesis at day 7 under all salinity concentrations, with a rebound with longer periods of salinity. It has been previously reported that a decline in photosynthesis was an early response to salinity in P. euphratica seedlings, resulting from a decrease in osmotic potential and availability of water and nutrients due to the presence of salts in the soil (Li et al., 2013). Enhanced photosynthesis after a period of adaptation to salinity stress may be one of the adaptation strategies of P. euphratica. A rebound in photosynthesis reflected leaf economic traits that consisted of higher water transfer efficiency for leaf hydraulic traits. These results illustrated that the leaf economic traits in P. euphratica were consistent with the leaf hydraulic traits at the middle stages of salt stress.
There was an increase in stomatal conductance at day 21 of stress but with a decline in the hydraulic capacity of leaves. This suggested an inconsistent relationship between the hydraulic traits and economic traits of the leaf, which was consistent with earlier findings in subtropical and tropical forests in China (Li et al., 2015; Blackman et al., 2016). Li et al. (2015) observed that the hydraulic and economic traits of leaves were two functional subsystems involved in different mesophyll tissues located in different regions; leaf hydraulic and economic traits were related to spongy and palisade tissues, respectively, (Yin et al., 2018). Regulation of functional subsystems may not be synchronized, and the most likely causes of the differences were different conditions, in particular, water availability. The existing evidence showed that leaf stomatal regulation appeared more connected with water potential in stems and the xylem hydraulic system than in leaves (Quero et al., 2011; Zhang et al., 2013). These results showed consistency between leaf economic traits and leaf hydraulic traits in P. euphratica was a non-entity at late stages of salt stress.
4.4. Leaf intracellular traits under salinity stress
Salt stress exposed the chloroplasts to excessive excitation energy, which could result in an increase in the production of ROS and oxidative-induced stress (Ray et al., 2012). The generation of ROS is highly harmful to the integrity of a cell, and destruction by reactive oxygen species with increasing duration of salt stress (Ray et al., 2012; AbdElgawad et al., 2016). To dissipate these harmful molecules, peroxidase enzymes commence protecting trees against oxidative-induced stress, indicating increased production of ROS (Demidchik, 2015; Rajput et al., 2015). Foliar antioxidant enzyme activities were crucial in scavenging ROS and maintaining self-tolerance in coping with stress environments (Li et al., 2016a,b; Yu et al., 2020). In our study, the activity of SOD was stable at lower salinity treatment at a relatively shorter duration and then was highly enhanced. The activities of POD and CAT in P. euphratica leaves were enhanced with salinity stress, which illustrated that the enzymes POD and CAT played dominant roles in protection at lower salt stress. Higher activity of SOD, POD, and CAT observed in higher salinity-treated plants indicated that P. euphratica had a higher capacity for scavenging ROS, indicating that this may be a defensive mechanism to weaken oxidative damage induced by severe salt stress; this is consistent with previous studies (Rajput et al., 2015).
Proline and total soluble sugar accumulation may offer leaves an osmotic adjustment, which is a common response in some herbaceous and woody plant species to osmotic stress induced by high ion accumulation in soils (Shamsi et al., 2020; Afefe et al., 2021). Accumulating organic compounds, such as sugars and amino acids in the cytoplasm, eventually lead to the restoration of cellular homeostasis, detoxification, and therefore survival under stress (Parihar et al., 2015). The magnitude of change in proline content in different species under salt stress may differ; thus, proline rapidly accumulated in mangrove and Australian wild rice under salt stress (Afefe et al., 2021; Nguyen et al., 2021), but it decreased in both pearl millet and wheat (Yadav et al., 2020). In this study, proline content was stable at lower salinity levels for a relatively short period but significantly accumulated with a higher salinity treatment. Proline content was low in roots of P. euphratica grown under NaCl stress but high in leaves of in vitro shoots (Watanabe et al., 2000); this was probably because the integrity of the plant can be properly regulated by osmotic adjustment through a low level of proline accumulation. Soluble sugars may act as a form of storage and be supplied to achieve plant survival under salinity stress conditions (Choat et al., 2018; Yu et al., 2020), such as in osmoregulation and xylem restoration (Secchi and Zwieniecki, 2011; Martínez-Vilalta et al., 2016). Previous studies have demonstrated that soluble sugars contribute to maintaining the water potential gradient between soil and plant during osmotic stress by osmotic adjustment (Baerdemaeker et al., 2017). Soluble sugars in this study were basically stable with higher salinity treatment at a relatively short duration but significantly accumulated with lower salinity treatment. The accumulation of proline and total soluble sugar in leaves may be connected with osmotic mechanisms and saline stress tolerance, consistent with the conclusions of previous studies. In addition, the accumulation of sugars in P. euphratica may contribute to osmotic adjustment more than the accumulation of proline (Watanabe et al., 2000; Moukhtari et al., 2020).
Malondialdehyde content could reflect the final level of cell membrane disruption, the generation of MDA will be lower, indicating a lower level of cell membrane disruption (Shamsi et al., 2020; Bezerra-Neto et al., 2022). Lower values of MDA caused by a combination of enzymatic defense and osmotic adjustment in P. euphratica remained stable to avoid membrane damage in salt stress. This showed that low MDA levels may significantly affect plant adaption to saline environments, as proposed by several researchers for some species with salt-tolerant mechanisms (Sergio et al., 2012). Intracellular regulatory mechanisms including enzymatic defense and osmotic adjustment in leaves were contributing factors in salt tolerance when P. euphratica was exposed to salinity stress. This illustrated that there was a coordination mechanism for plants during increasing salt stress, leaf intracellular traits in P. euphratica can coordinate with leaf hydraulic and with leaf economic traits, reduce salt damage, and guarantee the survival and growth of P. euphratica under salinity conditions.
5. Conclusion
Adaptation and coordination of leaf functional properties in P. euphratica under salt stress were determined with hydraulic parameters, gas exchange parameters, and physiological and biochemical factors. The range of deleterious effects of salt stress on plant leaf functional traits depends on salinity levels. Functional properties of the leaf, which link the external environment and plants, have a significant effect on the improvement of plant performance in changing environments. Changes in leaf functional traits reflect the resistance and fitness of riparian plants under different salt stress conditions in arid areas. We found that the leaf economic traits in P. euphratica were consistent with the leaf hydraulic traits at a medium level of salt stress, with inconsistent relationships during the onset and late stages of salt stress. There was almost no biochemical limitation under severe salinity conditions and the CO2 enrichment of P. euphratica had a greater effect on leaf economic traits. Toxic ion exclusion based on water availability and the intracellular mechanisms in leaves were contributing factors in salt tolerance when P. euphratica was exposed to salinity. Leaf intracellular traits can coordinate with leaf economic traits and leaf hydraulic traits, then form a defense mechanism to reduce salt damage and guarantee the growth of P. euphratica under saline conditions.
Plants can mitigate stress damage and sustain their existence through protective mechanisms. Plants could acclimatize to abiotic conditions with an adjustment in adaptive functional traits as further evidence of survival for P. euphratica in the extreme zone. In conclusion, riparian forests of P. euphratica exhibit a survival strategy to adapt to the saline environment by adjusting and coordinating leaf functional traits. Changes in the functional properties of the leaf in riparian plants under different salt stress environments also offer a scientific basis for riparian plant restoration and protection of riparian forests. Future studies should address the functional traits of P. euphratica grown in the wild combined with the local specific hydrological environment to improve the understanding of the internal acclimation mechanism under salt conditions.
Data availability statement
The original contributions presented in this study are included in the article/supplementary material, further inquiries can be directed to the corresponding author.
Author contributions
DL and JS collected the experimental data, analyzed it, and wrote the manuscript. XR and JL participated equally in conceptualization, data analysis, and manuscript preparation and review. All authors contributed to the article and approved the submitted version.
Funding
This research was funded by the Science and Technology Innovation Project of Colleges and Universities in Shanxi Province (No. 2022L414), the Inner Mongolia Special Fund Project for the Transformation of Scientific and Technological Achievements (No. 2021CG0046), and the Open Fund Project of Xinjiang Production and Construction Corps Key Laboratory of Tarim Basin Biological Resources Protection and Utilization (No. BRZD2202).
Acknowledgments
We thank the support received from the forest farm of Ejina Banner in the Alashan League. We also record our sincere appreciation for the helpful and constructive comments made by the reviewers of the draft manuscript.
Conflict of interest
The authors declare that the research was conducted in the absence of any commercial or financial relationships that could be construed as a potential conflict of interest.
Publisher’s note
All claims expressed in this article are solely those of the authors and do not necessarily represent those of their affiliated organizations, or those of the publisher, the editors and the reviewers. Any product that may be evaluated in this article, or claim that may be made by its manufacturer, is not guaranteed or endorsed by the publisher.
References
AbdElgawad, H., Zinta, G., Hegab, M. M., Pandey, R., Asard, H., and Abuelsoud, W. (2016). High salinity induces different oxidative stress and antioxidant responses in maize seedlings organs. Front. Plant Sci. 7:580. doi: 10.3389/fpls.2016.00276
Adams, H. D., Zeppel, M. J., Anderegg, W. R., Hartmann, H., Landhäusser, S. M., Tissue, D. T., et al. (2017). A multi-species synthesis of physiological mechanisms in drought-induced tree mortality. Nat. Ecol. Evol. 1, 1285–1291. doi: 10.1038/s41559-017-0248-x
Afefe, A. A., Khedr, A., Abbas, M. S., and Soliman, A. S. (2021). Responses and tolerance mechanisms of mangrove trees to the ambient salinity along the Egyptian red sea coast. Limnol. Rev. 21, 3–13. doi: 10.2478/limre-2021-0001
Alsina, M. M., Smart, D. R., Bauerle, T., Herralde, F d, Biel, C., Stockert, C., et al. (2011). Seasonal changes of whole root system conductance by a salt-tolerant grape root system. J. Exp. Bot. 62, 99–109. doi: 10.1093/jxb/erq247
Anderegg, W. R., Berry, J. A., Smith, D. D., Sperry, J. S., Anderegg, L. D., and Field, C. B. (2012). The roles of hydraulic and carbon stress in a widespread climate-induced forest die-off. Proc. Natl. Acad. Sci. U.S.A. 109, 233–237. doi: 10.1073/pnas.1107891109
Baerdemaeker, N. J., Salomón, R. L., Roo, L. D., and Steppe, K. (2017). Sugars from woody tissue photosynthesis reduce xylem vulnerability to cavitation. New Phytol. 216, 720–727. doi: 10.1111/nph.14787
Bezerra-Neto, E., Machado Coelho, J., Jarma-Orozco, A., Rodríguez-Páez, L., and Pompelli, M. (2022). Modulation of photosynthesis under salinity and the role of mineral nutrients in Jatropha curcas L. J. Agron. Crop Sci. 208, 314–334. doi: 10.1111/jac.12583
Blackman, C. J., Aspinwall, M. J., Resco de Dios, V., Smith, R. A., and Tissue, D. T. (2016). Leaf photosynthetic, economics and hydraulic traits are decoupled among genotypes of a widespread species of eucalypt grown under ambient and elevated CO2. Funct. Ecol. 30, 1491–1500. doi: 10.1111/1365-2435.12661
Cao, X., Jia, J., Zhang, C., Li, H., Liu, T., Jiang, X., et al. (2014). Anatomical, physiological and transcriptional responses of two contrasting poplar genotypes to drought and re-watering. Physiol. Plant. 151, 480–494. doi: 10.1111/ppl.12138
Chen, Y., Xu, C., and Li, W. (2012). Groundwater depth affects the daily course of gas exchange parameters of Populus euphratica in arid areas. Environ. Earth Sci. 66, 433–440. doi: 10.1007/s12665-011-1250-2
Choat, B., Brodribb, T. J., Brodersen, C. R., Duursma, R. A., López, R., and Medlyn, B. E. (2018). Triggers of tree mortality under drought. Nature 558, 531–539. doi: 10.1038/s41586-018-0240-x
Corso, D., Delzon, S., Lamarque, L. J., Cochard, H., Torres-Ruiz, J. M., King, A., et al. (2020). Neither xylem collapse, cavitation, or changing leaf conductance drive stomatal closure in wheat. Plant Cell Environ. 43, 854–865. doi: 10.1111/pce.13722
Deinlein, U., Stephan, A. B., Horie, T., Luo, W., Xu, G., and Schroeder, J. I. (2014). Plant salt-tolerance mechanisms. Trends Plant Sci. 19, 371–379. doi: 10.1016/j.tplants.2014.02.001
Demidchik, V. (2015). Mechanisms of oxidative stress in plants: From classical chemistry to cell biology. Environ. Exp. Bot. 109, 212–228. doi: 10.1016/j.envexpbot.2014.06.021
Derroire, G., Powers, J., Hulshof, C., Cárdenas, V. L., and Healey, J. (2018). Contrasting patterns of leaf trait variation among and within species during tropical dry forest succession in Costa Rica. Sci. Rep. 8:285. doi: 10.1038/s41598-017-18525-1
Dong, L., and Jaume, F. (2018). Leaf economics spectrum in rice: Leaf anatomical, biochemical and physiological trait trade-offs. J. Exp. Bot. 69, 599–609.
Duan, H., Ma, Y., Liu, R., Li, Q., Yang, Y., and Song, J. (2018). Effect of combined waterlogging and salinity stresses on euhalophyte Suaeda glauca. Plant Physiol. Biochem. 127, 231–237. doi: 10.1016/j.plaphy.2018.03.030
Duan, H., O’Grady, A., Duursma, R., Choat, B., Huang, G., Smith, R., et al. (2015). Drought responses of two gymnosperm species with contrasting stomatal regulation strategies under elevated [CO2] and temperature. Tree Physiol. 35, 756–770. doi: 10.1093/treephys/tpv047
Eller, F., Lambertini, C., Nguyen, L. X., and Brix, H. (2014). Increased invasive potential of non-native Phragmites australis: Elevated CO2. Glob. Change Biol. 20, 531–543. doi: 10.1111/gcb.12346
Flowers, T. J., and Colmer, T. D. (2015). Plant salt tolerance: Adaptations in halophytes. Ann. Bot. 115, 327–331. doi: 10.1093/aob/mcu267
Hartmann, H., and Trumbore, S. (2016). Understanding the roles of nonstructural carbohydrates in forest trees-from what we can measure to what we want to know. New Phytol. 211, 386–403. doi: 10.1111/nph.13955
Hishida, M., Ascencio-Valle, F., Fujiyama, H., Orduño-Cruz, A., Endo, T., and Larrinaga-Mayoral, J. Á (2014). Antioxidant enzyme responses to salinity stress of Jatropha curcas and J. cinerea at seedling stage. Russian J. Plant Physiol. 61, 53–62. doi: 10.1134/S1021443714010063
Hussain, M. I., Lyra, D. A., Farooq, M., Nikoloudakis, N., and Khalid, N. (2015). Salt and drought stresses in safflower: A review. Agron. Sustain. Dev. 36, 1–32. doi: 10.1007/s13593-015-0344-8
Karst, J., Gaster, J., Wiley, E., and Landhäusser, S. M. (2017). Stress differentially causes roots of tree seedlings to exude carbon. Tree Physiol. 37, 154–164. doi: 10.1093/treephys/tpw090
Lawson, T., and Matthews, J. (2020). Guard cell metabolism and stomatal function. Annu. Rev. Plant Biol. 71, 273–302. doi: 10.1146/annurev-arplant-050718-100251
Li, D., Si, J., Zhang, X., Gao, Y., Wang, C., Luo, H., et al. (2019). Hydraulic characteristics of Populus euphratica in an arid environment. Forests 10:407. doi: 10.3390/f10050407
Li, J., Zhao, C., Li, J., Yan, Y. Y., Yu, B., and Han, M. (2013). Growth and leaf gas exchange in Populus euphratica across soil water and salinity gradients. Photosynthetica 51, 321–329. doi: 10.1007/s11099-013-0028-z
Li, L., McCormack, M. L., Ma, C., Kong, D., Zhang, Q., Chen, X., et al. (2015). Leaf economics and hydraulic traits are decoupled in five species-rich tropical-subtropical forests. Ecol. Lett. 18, 899–906. doi: 10.1111/ele.12466
Li, Q., Liu, R., Li, Z., Fan, H., and Song, J. (2022). Positive effects of NaCl on the photoreaction and carbon assimilation efficiency in Suaeda salsa. Plant Physiol. Biochem. 177, 32–37. doi: 10.1016/j.plaphy.2022.02.019
Li, Y., Chen, W., Chen, J., and Shi, H. (2016a). Vulnerability to salt-induced cavitation in shoots of two typical shrubs in the southern Mu Us Sandy Land, China. J. Arid Land 8, 125–137. doi: 10.1007/s40333-015-0056-6
Li, Y., Duan, B., Chen, J., Korpelainen, H., Niinemets, Ü, and Li, C. (2016b). Males exhibit competitive advantages over females of Populus deltoides under salinity stress. Tree Physiol. 36, 1573–1584. doi: 10.1093/treephys/tpw070
Liu, C., Li, Y., Xu, L., Chen, Z., and He, N. (2019). Variation in leaf morphological, stomatal, and anatomical traits and their relationships in temperate and subtropical forests. Sci. Rep. 9:5803. doi: 10.1038/s41598-019-42335-2
Liu, J., Equiza, M. A., Navarro-Rodenas, A., Lee, S. H., and Zwiazek, J. J. (2014). Hydraulic adjustments in aspen (Populus tremuloides) seedlings following defoliation involve root and leaf aquaporins. Planta 240, 553–564. doi: 10.1007/s00425-014-2106-2
Liu, X., and Suarez, D. L. (2021). Lima bean growth. Leaf stomatal and nonstomatal limitations to photosynthesis, and C-13 discrimination in response to saline irrigation. J. Am. Soc. Horticult. Sci. 146, 132–144. doi: 10.21273/JASHS04996-20
Ma, F., Barrett-Lennard, E. G., and Tian, C. Y. (2019). Changes in cell size and tissue hydration (‘succulence’) cause curvilinear growth responses to salinity and watering treatments in euhalophytes. Environ. Exp. Bot. 159, 87–94. doi: 10.1016/j.envexpbot.2018.12.003
Martínez-Vilalta, J., Sala, A., Asensio, D., Galiano, L., Hoch, G., Palacio, S., et al. (2016). Dynamics of non-structural carbohydrates in terrestrial plants: a global synthesis. Ecol. Monogr. 86, 495–516. doi: 10.1002/ECM.1231
Meena, M., Divyanshu, K., Kumar, S., Swapnil, P., Zehra, A., Shukla, V., et al. (2019). Regulation of L-proline biosynthesis, signal transduction, transport, accumulation and its vital role in plants during variable environmental conditions. Heliyon 5:2952. doi: 10.1016/j.heliyon.2019.e02952
Minh, L., Khang, D., Ha, P., Tuyen, P. T., Minh, T. N., Quan, N. V., et al. (2016). Effects of salinity stress on growth and phenolics of rice (Oryza sativa L.). Int. Lett. Nat. Sci. 57, 1–10. doi: 10.56431/p-f1p658
Moukhtari, A. E., Cabassa-Hourton, C., Farissi, M., and Savour, A. (2020). How does proline treatment promote salt stress tolerance during crop plant development? Front. Plant Sci. 11:1127. doi: 10.3389/fpls.2020.01127
Nguyen, H. T., Bhowmik, S. D., Long, H., Cheng, Y., Mundree, S., and Hoang, L. T. (2021). Rapid accumulation of proline enhances salinity tolerance in Australian wild rice Oryza australiensis domin. Plants 10:2044. doi: 10.3390/plants10102044
Pan, Y., Chen, Y., Chen, Y., Wang, R., and Ren, Z. (2016). Impact of groundwater depth on leaf hydraulic properties and drought vulnerability of Populus euphratica in the Northwest of China. Trees 30, 2029–2039. doi: 10.1007/s00468-016-1430-5
Parihar, P., Singh, S., Singh, R., Singh, V. P., and Prasad, S. M. (2015). Effect of salinity stress on plants and its tolerance strategies: A review. Environ. Sci. Pollut. Res. 22, 4056–4075. doi: 10.1007/s11356-014-3739-1
Per, T. S., Khan, N. A., Reddy, P. S., Masood, A., Hasanuzzaman, M., Khan, M. I., et al. (2017). Approaches in modulating proline metabolism in plants for salt and drought stress tolerance: Phytohormones, mineral nutrients and transgenics. Plant Physiol. Biochem. 115, 126–140. doi: 10.1016/j.plaphy.2017.03.018
Pérez-López, U., Robredo, A., Lacuesta, M., Mena-Petite, A., and Muñoz-Rueda, A. (2012). Elevated CO2 reduces stomatal and metabolic limitations on TI Elevated CO2 reduces stomatal and metabolic limitations on photosynthesis caused by salinity in Hordeum vulgare. Photosynth. Res. 111, 269–283. doi: 10.1007/s11120-012-9721-1
Petit, G., Savi, T., Consolini, M., Anfodillo, T., and Nardini, A. (2016). Interplay of growth rate and xylem plasticity for optimal coordination of carbon and hydraulic economies in Fraxinus ornus trees. Tree Physiol. 36, 1310–1319. doi: 10.1093/treephys/tpw069
Pivovaroff, A., Lawren, S., and Santiago, L. (2014). Coordination of stem and leaf hydraulic conductance in southern California shrubs: A test of the hydraulic segmentation hypothesis. New Phytol. 203, 842–850. doi: 10.1111/nph.12850
Pou, A., Medrano, H., Flexas, J., and Tyerman, S. D. (2013). A putative role for TIP and PIP aquaporins in dynamics of leaf hydraulic and stomatal conductances in grapevine under water stress and re-watering. Plant Cell Environ. 36, 828–843. doi: 10.1111/pce.12019
Powers, J. S., Vargas G, G., Brodribb, T. J., Schwartz, N. B., Pérez-Aviles, D., Smith-Martin, C. M., et al. (2020). A catastrophic tropical drought kills hydraulically vulnerable tree species. Glob. Change Biol. 26, 3122–3133. doi: 10.1111/gcb.15037
Quero, J. L., Sterck, F. J., Martínez-Vilalta, J., and Villar, R. (2011). Water-use strategies of six co-existing Mediterranean woody species during a summer drought. Oecologia 166, 45–57. doi: 10.1007/s00442-011-1922-3
Rajput, V., Chen, Y., and Ayup, M. (2015). Effects of high salinity on physiological and anatomical indices in the early stages of Populus euphratica growth. Russian J. Plant Physiol. 62, 229–236. doi: 10.1134/S1021443715020168
Ray, P., Huang, B., and Tsuji, Y. (2012). Reactive oxygen species (ROS) homeostasis and redox regulation in cellular signaling. Cell. Signal. 24, 981–990. doi: 10.1016/j.cellsig.2012.01.008
Sack, L., and Scoffoni, C. (2013). Leaf venation: Structure, function, development, evolution, ecology and applications in the past, present and future. New Phytol. 198, 983–1000. doi: 10.1111/nph.12253
Scoffoni, C., Vuong, C., Diep, S., Cochard, H., and Sack, L. (2014). Leaf shrinkage with dehydration: coordination with hydraulic vulnerability and drought tolerance. Plant Physiol. 164, 1772–1788. doi: 10.1104/pp.113.221424
Secchi, F., and Zwieniecki, M. A. (2011). Sensing embolism in xylem vessels: The role of sucrose as a trigger for refilling. Plant Cell Environ. 34, 514–524. doi: 10.1111/j.1365-3040.2010.02259.x
Sergio, L., Paola, A., Cantore, V., Pieralice, M., Cascarano, N. A., Bianco, V. V., et al. (2012). Effect of salt stress on growth parameters, enzymatic antioxidant system, and lipid peroxidation in wild chicory (Cichorium intybus L.). Acta Physiol. Plant. 34, 2349–2358. doi: 10.1007/s11738-012-1038-3
Shamsi, N. A., Hussain, M. I., and El-Keblawy, A. (2020). Physiological responses of the xerohalophyte Suaeda vermiculata to salinity in its hyper-arid environment. Flora Morphol. Distrib. Funct. Ecol. Plants 273:151705. doi: 10.1016/j.flora.2020.151705
Si, J., Feng, Q., Cao, S., Yu, T., and Zhao, C. (2014). Water use sources of desert riparian Populus euphratica forests. Environ. Monitor. Assess. 186, 5469–5477. doi: 10.1007/s10661-014-3796-4
Tyree, M. T., Patiño, S., Bennink, J., and Alexander, J. (1995). Dynamic measurements of roots hydraulic conductance using a high-pressure flowmeter in the laboratory and field. J. Exp. Bot. 46, 83–94. doi: 10.1093/jxb/46.1.83
Watanabe, S., Kojima, K., Ide, Y., and Sasaki, S. (2000). Effects of saline and osmotic stress on proline and sugar accumulation in Populus euphratica in vitro. Plant Cell Tissue Organ Cult. 63, 199–206. doi: 10.1023/A:1010619503680
Xu, Z., Jiang, Y., Jia, B., and Zhou, G. (2016). Elevated-CO2 response of stomata and its dependence on environmental factors. Front. Plant Sci. 7:657. doi: 10.3389/fpls.2016.00657
Yadav, T., Kumar, A., Yadav, R. K., Yadav, G., Kumar, R., and Kushwaha, M. (2020). Salicylic acid and thiourea mitigate the salinity and drought stress on physiological traits governing yield in pearl millet-wheat. Saudi J. Biol. Sci. 27, 2010–2017. doi: 10.1016/j.sjbs.2020.06.030
Yang, L., Shi, Y., Xiao, R., Wu, Q., Qu, A., Yu, M., et al. (2021). Salt interferences to metabolite accumulation, flavonoid biosynthesis and photosynthetic activity in Tetrastigma hemsleyanum. Environ. Exp. Bot. 194:104765. doi: 10.1016/j.envexpbot.2021.104765
Yin, H., Tariq, A., Zhang, B., Lv, G., Zeng, F., Graciano, C., et al. (2021). Coupling relationship of leaf economic and hydraulic traits of Alhagi sparsifolia shap in a hyper-arid desert ecosystem. Plants 10:1867. doi: 10.3390/plants10091867
Yin, Q., Wang, L., Lei, M., Dang, H., Quan, J., Tian, T., et al. (2018). The relationships between leaf economics and hydraulic traits of woody plants depend on water availability. Sci. Total Environ. 621, 245–252. doi: 10.1016/j.scitotenv.2017.11.171
You, J., and Chan, Z. (2015). ROS regulation during abiotic stress responses in crop plants. Front. Plant Sci. 6:1092. doi: 10.3389/fpls.2015.01092
Yu, L., Dong, H., Li, Z., Han, Z., Korpelainen, H., and Li, C. (2020). Species-specific responses to drought, salinity and their interactions in Populus euphratica and P. pruinosa seedlings. J. Plant Ecol. 13, 563–573. doi: 10.1093/jpe/rtaa043
Zelm, E., Zhang, Y., and Testerink, C. (2020). Salt tolerance mechanisms of plants. Annu. Rev. Plant Biol. 71, 403–433. doi: 10.1146/annurev-arplant-050718-100005
Zeng, F., Yan, H., and Arndt, S. K. (2009). Leaf and whole tree adaptations to mild salinity in field grown Populus euphratica. Tree Physiol. 29, 1237–1246. doi: 10.1093/treephys/tpp055
Zhang, C., Liu, H., Huang, N., Zhang, F., Meng, Y., Wang, J., et al. (2022). Coordination of leaf hydraulic and economic traits in Cinnamomum camphora under impervious pavement. BMC Plant Biol. 22:347. doi: 10.1186/s12870-022-03740-4
Zhang, Y., Kaiser, E., Li, T., and Marcelis, L. F. (2022). NaCl affects photosynthetic and stomatal dynamics by osmotic effects and reduces photosynthetic capacity by ionic effects in tomato. J. Exp. Bot. 73, 3637–3650. doi: 10.1093/jxb/erac078
Zhang, Y., Meinzer, F. C., Qi, J., Goldstein, G., and Cao, K. (2013). Midday stomatal conductance is more related to stem rather than leaf water status in subtropical deciduous and evergreen broadleaf trees. Plant Cell Environ. 36, 149–158. doi: 10.1111/j.1365-3040.2012.02563.x
Keywords: Populus euphratica, leaf economic traits, leaf hydraulic traits, leaf intracellular traits, salt stress
Citation: Li D, Si J, Ren X and Li J (2023) Coordination in functional traits of Populus euphratica leaves under salt stress. Front. For. Glob. Change 6:1144079. doi: 10.3389/ffgc.2023.1144079
Received: 13 January 2023; Accepted: 29 March 2023;
Published: 24 April 2023.
Edited by:
Di Yang, University of Wyoming, United StatesReviewed by:
Dejin Wang, Kunming University of Science and Technology, ChinaFuping Zhang, Shaanxi Normal University, China
Shawn M. McKenzie, McMaster University, Canada
Copyright © 2023 Li, Si, Ren and Li. This is an open-access article distributed under the terms of the Creative Commons Attribution License (CC BY). The use, distribution or reproduction in other forums is permitted, provided the original author(s) and the copyright owner(s) are credited and that the original publication in this journal is cited, in accordance with accepted academic practice. No use, distribution or reproduction is permitted which does not comply with these terms.
*Correspondence: Jianhua Si, jianhuas@lzb.ac.cn